- 1Department of Chemical Engineering, Faculty of Engineering, Kyrgyz-Turkish Manas University, Bishkek, Kyrgyzstan
- 2Department of Chemical Engineering, Institute Natural and Applied Sciences, Van Yüzüncü Yıl University, Van, Turkey
In the recent two decades, tremendous devices and materials such as stents, biomimetic organs, scaffolds, and vessels have been developed for medical purposes. When such devices are utilized in the body, the side effects or biocompatibility of the materials have to be studied extensively. Interdisciplinary studies have reviled numerous strategies to overcome adverse body reactions against implanted devices. Besides naturally occurring materials such as collagen, chitosan, hyaluronic acid, and dextran, various synthetic and modified materials such as poly(lactic acid), poly(ethylene glycol), poly(vinyl alcohol), and poly(acrylamide) have been accomplished. In this context, progress in polymer science makes hydrogels a valuable candidate for those utilizations. Moreover, hydrogels received enormous attention as drug delivery devices because of their unique properties, such as soft structure and responsive capabilities based on the functional group attached. Particularly, the developments in synthetic materials have brought out numerous materials for medical and pharmaceutical applications. In recent studies, organo-hydrogels, a branch of hydrogels, have drawn considerable attention over hydrogels because of superior properties such as the coexistence of organic and aqueous phases and viscoelastic bi-phasic natures. They were prepared in bulk forms and nano-scale dimensions, which allow them to be utilized more extensively. These incredible structures provide them with extensive features to be utilized from head to toe in every aspect of health care application. In this short review, we will focus on some of the pioneering perspectives of organo-hydrogels particularly accomplished in clinical therapy and the use of their biodegradable, target-responsive properties as sensing components in novel microscale apertures.
Introduction
Developments in health care and pharmaceutical science are dependent on variations of novel drugs and rely significantly on drug carriers, which deliver drugs through the living organisms from the first step up to reach the targeted organs. In this perspective, drug carriers have drawn gradually increasing importance and had an enormous impact on interdisciplinary studies, as summarized in reviews on relevant areas (Sadtler et al., 2020; Bernhard and Tibbitt, 2021; Mitchell et al., 2021; Sadeghi et al., 2021). The complex structure of the drug delivery through the human body requires closer collaborations between disciplines such as clinical medicine, biology, immunology, chemistry, and chemical and biological engineering (Langer and Peppas, 1983; Peer et al., 2007; Sahiner et al., 2011; Garner et al., 2020; Alpaslan et al., 2021a). It is well known that each drug has an intake level above which it is toxic and below which it is insufficient, whereas available drug concentration in a body at a defined time is an important prescribed routine in the therapy. The drug delivery systems’ objective is the sum of the use of strategies and methodologies of synthesis, preparations, and modifications of drug carriers by the collaboration of chemistry, biology, and relevant engineering disciplines, which improve the fraction of drug that reaches its targeted tissues. Webber and Langer described the properties that drug delivery systems must have as follows: therapeutic efficacy, drug exposure/toxicity, dose frequency, therapeutic adherence, and patient-specific therapeutic function (Webber and Langer, 2017). From the clinicians and patients’ perspectives, maintaining the drug in the range and the required dosage is important. The utilization of the drug carriers for in vivo cases relies on many properties to be adopted by the complex physiology within living organisms, even though they were previously optimized throughout ex vivo studies (Langer and Peppas, 1981; Uhrich et al., 1999; Sagbas et al., 2015; Kakkar et al., 2017; Sahiner et al., 2018; Alpaslan et al., 2021b). Drug carriers need to overcome many tests prior to clinical implementation. As the first step, biocompatibility is only a very tiny part of those tests. Research has focused on case-sensitive delivery systems with responsive behavior to physiological environments, which must address biocompatibility, specific targeting, and transport. In the light of the above context, hydrogels and organogels have drawn considerable attention in the preparation of those materials (Peppas, 1982; Langer and Peppas, 1983; Jabbari and Peppas, 1994; Webber and Langer, 2017). Since the first time hydrogels appeared for biomedical applications in 1959 (Wichterle and Lim, 1960), they have drawn tremendous attention. Hundreds of studies are published every year on their utilization for specific purposes in medical applications. As described in common definitions, hydrogels are three-dimensional structures consisting of polymeric units and swollen in water or biological liquids (Uhrich et al., 1999; Koetting et al., 2015). Hydrogels have favorable properties that allow them to be used as biomaterials for drug delivery, implants, and engineered tissue platforms. Having a highly hydrophilic nature let them have water uptake properties that make them similar to cells in the biological environment and provide them with excellent properties to be used as biomaterials (Bell et al., 1995; Peppas et al., 2006; Koetting and Peppas, 2014; Sahiner et al., 2017; Zhang et al., 2020). Since Wichterle and Lim introduced gels prepared from polyvinyl alcohol or glycol monomethacrylate, which are exceptions among the polymers used in biomedical applications (Wichterle and Lím, 1960), numerous studies have been published to introduce novel materials in this context. Nowadays, hydrogels in bulk or particle form are used in numerous medical applications such as ophthalmology, biosensors, membranes, and drug carriers (Langer and Peppas, 1983; Bell et al., 1995; Hassan et al., 2000; Peppas et al., 2006; Suner et al., 2019; Sadtler et al., 2020; Vichare et al., 2020). Most known hydrogels and their utilization purposes in medicine are extensively studied and listed in review literature (Uhrich et al., 1999; Peppas et al., 2000). The polymeric backbone and the composition of the atomic structures are various, which makes those polymeric structures unique for the targeted applications. For instance, poly(vinyl alcohol) (PVA), polyacrylamide (PAAm), poly(N-vinyl pyrrolidone) (PNVP), poly(hydroxyethyl methacrylate) (PHEMA), poly(ethylene glycol) monomethyl ether (PEGME), and cellulose were used as intravascular carriers (Ronneberger et al., 1996; Ronneberger et al., 1997; Tsai et al., 2002; Dalsin et al., 2003; Anderson and Shive, 2012). Poly(hydroxyethyl methacrylate), methacrylic acid, butyl methacrylate, poly(hydroxyethyl methacrylate) and poly(ethylene terephthalate) are applied in contact lenses. Cellulose acetate, polyvinyl alcohol, thermo-polymers of HEMA, MMA, and p(HEMA-b-siloxane) were used for artificial tendons, artificial skin, and ophthalmic applications. Poly(glycolic acid), poly(lactic acid), chitosan, hyaluronate, and dextran are biodegradable hydrogels and used in controlled drug delivery (Draye et al., 1998; Vercruysse and Prestwich, 1998; Uhrich et al., 1999; Peppas et al., 2000; Borchard and Junginger, 2001; Cadée et al., 2001; Khor and Lim, 2003; Peppas et al., 2006; Sahiner et al., 2011; Uchegbu et al., 2011; Suner et al., 2018; Sahiner, 2021).
This short review focuses on recent advances of engineered organogels for medical, pharmaceutical, and drug delivery platforms and their utilization. Therefore, it will not cover important studies relevant to significant drug delivery materials and methods that have been tremendously studied recently.
Biocompatibility of Materials Used for Medication, Standard Tests, and Regulations
A living organism always reacts protectively regardless of the kind of material implanted, which is the natural reaction of all living organisms. As various controlled drug delivery devices are available commercially, it is important to consider the biocompatibility parameters for these systems. Since the acceptance of the Medical Devices Amendments in 1976, the biocompatibility of the materials for medical issues has been increasing (Park and Park, 1996). Biocompatibility is commonly defined as the absence of cytotoxicity of implant material and the material’s functionality, which provides it to support cell-implant material interactions where it is applied. In this regard, biocompatibility tests are the tests carried out at conditions similar to the biological environment to evidently predict whether a biomaterial or an implant presents a potential danger for the applied body. In this context, the first step to improving any material as an implant or drug delivery is to test its biocompatibility prior to studying its bio-adhesion behaviors through biological environments, as the body’s reaction to implanted devices adversely affects patients’ health. On top of all these, the body’s response to an antipathetic material in a particular application may not be antipathetic for that material in another application (Onuki et al., 2008).
In fact, an adverse response, for instance, inflammation, is induced when any foreign material such as an implant or biomaterial treats the living organisms (Fournier et al., 2003; Anderson et al., 2008). Moreover, the magnitude of the response varies according to the properties of the biomaterials and depends on chemical composition to the surface of the biomaterials, shape, morphology, sterility, and degradation of the materials (Peppas, 1982; Fournier et al., 2003; Sagbas et al., 2015). The body response is created after implantation, nonspecific absorption of blood and defensive tissue fluids is induced, and immune and inflammatory cells are produced by the body to protect it from biomaterials (Downes and Mishra, 2011). The 10993 protocol of the International Organization for Standardization (ISO) describes a series of standards for determining materials as biocompatible prior to initiating clinical investigations. European and Asian countries accepted the ISO 10993 standards, whereas FDA required more strict requirements in addition to the ISO requirements published in Blue Book Memorandum G95-1 (Required Biocompatibility Training and Toxicology Profiles for Evaluation of Medical Devices). These issues have been extensively discussed in the relevant literature (Onuki et al., 2008; Kohane and Langer, 2010; Bernard et al., 2018).
In the first step of improving a medical device, some inexpensive in vitro tests are applied to the materials to evaluate useful information prior to animal tests. These tests reduce risks and save time and resources early in choosing the best materials for in vivo studies. As the surface of a biomaterial is in contact with the cell at a molecular level, properties such as surface roughness, chemical structure, hydrophilicity, smoothness, swelling, tensile strength, hardness, durability, and interaction with proteins become more important (Angelova and Hunkeler, 1999; Wang et al., 2004; Thevenot et al., 2008; Akkas et al., 2013). Wettability is an important property of the materials as hydrophilic surfaces reduce interfacial free energy, making protein adsorption and cell contact easier. In this context, the hydrophilicity of the polymeric biomaterials is increased by various chemical and physical techniques. Several characterization techniques such as atomic force microscopy, X-ray diffraction, infrared spectroscopy, X-ray photoelectron spectroscopy, and scanning electron microscopy are widely accomplished to characterize the materials (Sagbas et al., 2015; Sahiner et al., 2019; Vichare et al., 2020). These tests generally consist of cytotoxicity, irritation, and hemocompatibility (Peppas and Sahlin, 1996; Onuki et al., 2008; Bernard et al., 2018).
Bernard et al. (2018) presented a detailed investigation on the biocompatibility of polymer-based biomaterials and clinical devices. Although the biocompatibility tests are in evaluation, useful studies are available to decide the final product. Regarding that study, biocompatibility assessment must be carried out in three steps as follow:
i) Polymer granules or bland: determining being extractable and leachable (antioxidants, plasticizers, stabilizers, pigment, lubricants, catalysts, and contaminants), toxicological evaluation, and degradation profile.
ii) Polymer films and sheets or generation by spin coating technique: surface functional group, microstructure (wettability, surface roughness, swelling, and electrostatic effect), protein adsorption, cell viability, cytotoxicity, primary evaluation of sterilization impacts, and chemical and lipid resistance.
iii) Specimen representative of the final product: protein adhesion, cell viability, cytotoxicity, hemocompatibility, in vivo studies, and being leachable (Bernard et al., 2018).
Organogels: Novel Biomaterials in Medication and Pharmaceutical Utilizations
There is evidence that medicinal plants and herbs were extensively used by primitive tribal shamans, mages, and sorcerers to treat disease or pain since ancient ages (Finlayson, 1893). Organogels are biomaterials produced by the combination of essential oils and polymeric hydrogels via chemical and physical methods (Alpaslan et al., 2021a; Alpaslan et al., 2021b; Alpaslan et al., 2021c). Additional to their natural abundance, organogels have superior properties in the eye of biocompatibility, making them valuable materials in controlled drug delivery and medical applications (Tokuyama and Kato, 2010; Alsaab et al., 2016; Esposito et al., 2018).
We investigate recent studies on the application of organogels; this area provides opportunities for future research on controlled drug delivery and medical utilizations (Table 1).
In the study on pluronic lecithin organogels (PLO gels), Alsaab et al. (2016) stated that, with the advancement of topical and transdermal drug delivery, organogels are one of the most effective bases for drugs that need to be administered orally or by injection. In the early years of PLO production, there was an increase in research into possible application areas. PLO gels are widely used as transdermal medicine for systemic and local effects. Several research articles have questioned the effectiveness of PLO gel-containing drugs in percutaneous absorption. This may be due to inappropriate synthesis and combination of transdermal PLO gels with drugs (an appropriate drug with ideal molecular weight, log p-value, and potency). PLO gels should be preferred primarily as transdermal application, and attention should be paid to the suitability of the drugs (physicochemical properties, dose, and application site) to be used. Alsaab et al. stated that further advanced research (based on blind and placebo-controlled studies and concrete results) should be conducted to evaluate the optimum efficacy of drug-loaded PLO gels for patient care and therapeutic applications of PLO gels (Alsaab et al., 2016). PLO and sorbitan monostearate organogels (SMO) containing ketorolac tromethamine were synthesized. Sustained-release ketorolac tromethamine (KT) niosomal organogels were synthesized to increase skin permeability and drug efficacy. There are unbiased variables in hydrophilic-lipophilic balance value (HLB), which is the ratio of cholesterol in total lipid (Chol. Ratio) and the ratio of total lipid to the drug (L:D ratio)) for sufficient concentrations. The response surface technique was used to investigate the effect of these variables on the cumulative release percentages. The capture efficiency of KT niosomes was 40.13%, whereas the cumulative release percentage of KT after 6 and 12 h reached 52.3% and 74.5%, respectively. The niosomal organogel showed an 85% pow edema inhibition effect, positively correlated with the in vitro results. The sustained-release niosomal organogel of KT is an effective alternative to oral-dosage forms with fewer systemic side effects and high drug bioavailability (Elsayed et al., 2019).
Studies reviewed by Esposito et al. (2018) indicate that physicochemical properties (thermodynamic behavior, viscoelasticity, and versatility) have important effects on the synthesis and stabilization of organogels. These properties can be easily adjusted with formulations. Organogels obtained by hybridization with the materials in the formulation become ideal matrices, providing effective drug concentration over a long period of time, thereby increasing the chances of patients adapting to therapeutic applications. Although the biocompatibility properties of organogels have been reported in recent studies, they have not been studied to a large extent compared to other gel systems. Further studies should also focus on very prolonged releases with biomolecules such as peptides, proteins, or immunoglobulins from organogel-forming matrices at the application site. Further research on solvent and electrolyte diffusion, matrix degradation, and by-product elimination is required for a more effective use of organogels in effective drug delivery systems (Zeng et al., 2021).
Moreover, organogels are applied in dermal application and cosmetic industry. Fatty acid ethyl and isopropyl esters (FAEs) and bis-(aminoalcohol) oxalamides (BAOAs) with different chain lengths (ethyl laurate, ethyl myristate, ethyl palmitate, isopropyl laurate, isopropyl myristate, isopropyl palmitate) are biocompatible biochemical utilized for those purposes while ibuprofen is widely used as a model drug. In pharmaceutical liquids, BOA gelators and bisphenylglycinol oxalamide do not have organogelation properties in FAEs. Others show good gelling ability with the same solvents. Organogelation depends on the nature of the amino alcohol moiety. Compounds containing the phenylalanine group bis(PhAlaOH)benzyl were good organogelators. Compounds with isobutyl, sec-butyl, and isopropyl groups have low thermal stability due to the sterically hindered branched alkyl groups, while having high stability in all gelling solvents tested and the lowest minimum gel concentrations. It is seen that bis(PhAhaOH)benzyl has high thermal stability and good gelling properties compared to other gelling solvents. The bis(PhAhaOH)benzyl/FAEs gel network also interacts with gelator molecules due to van der Waals interactions, the benzylic groups at the hydrogen bond, and amino alcohol moieties. The release rates of ibuprofen from organogels depend on the pH of their solutions and the amino alcohol groups in the oxalamide binder. In addition, ibuprofen release rates were dependent on gelator concentration and the fraction of drug (%w) in the gel matrix. BOA organogels may also be a viable alternative to human skin cancer if anti-cancer agents are delivered through them. The release of ibuprofen from organogels is compatible with Fickian diffusion control, and the retention of small drug molecules in the LMWG gel can be accomplished in simple ways (Uzan et al., 2016). Castor oil organogel loaded with two lipophilic compounds (ketoconazole or indomethacin) with different ionization behaviors was prepared by hot emulsification in water (above gelation temperature) and cooling at room temperature. It was stated that drug loading did not affect the particle size and stability of the dispersions and did not inhibit the gelation process. Encapsulation efficiency is also good for ketoconazole and indomethacin. Stability results have been reported, with very limited drug leakage occurring during storage. Dissolution of the drug appears to allow rapid diffusion from the vehicle. Thus, the gel network of 12-hydroxystearic acid fibers will not inhibit drug release. In addition, the increased solubility of the drug in the oil phase may lead to better bioavailability as more drug is available for diffusion. Although the sustained release was not achieved, immobilization of the oil in a gelled matrix was likely responsible for the improved stability of the dispersions and limited escape of drug during storage. These systems can provide alternatives to nanoemulsions or solid lipid nanoparticles for biocompatibility, stability, or delivery of lipophilic compounds. The stickiness of the lipid-based nanoparticle, which was determined to facilitate drug tissue permeability, shows the alternativity of organogel nanoparticles in the field of oral administration (Martin et al., 2017; Alpaslan et al., 2021b). For treating Candida albicans infections, organogels have been developed using an oil phase of oleic acid (OA) and an aqueous phase of poloxamer (PL) PL407, alone or in combination with PL188, and 0.25–1% sodium alginate (SA). Moreover, the voriconazole (VRC) release system is targeted intravaginal. For OA-PL407-188 systems, the inclusion of SA was observed to have a concentration-dependent effect on the modulation of the VRC release rate. OA-PL407-188-SA organogels showed promising in vitro performance due to their antifungal activities and low cytotoxicity against HeLa and Vero cell lines. In conclusion, the findings of this study indicated that OA-PL407-188-SA organogels could provide the basis for new VRC delivery systems for use in future vaginal applications (Querobino et al., 2019).
In another study using the quality by design (QbD) paradigm, egg oil-based (EO) organogels (SSD-EOOG) were synthesized and used for the distribution of silver sulfadiazine (SSD), an effective antibacterial agent in treating burns. The prepared organogels were evaluated for in vivo efficacy and stability. The developed formulations were characterized for particle size, drug content, morphology, in vitro drug release, skin safety studies, ex vivo permeation, skin retention, tissue analysis, and pharmacodynamic studies in a murine burn wound model. The optimized formulation exhibited improved permeability (72.33 ± 1.73%) and retention (541.20 ± 22.16 µg/cm2) across the skin barrier with a particle size of 256.5 nm compared to SSD-MKT. Pharmacodynamic results proved the superior therapeutic efficacy of SSD-EOOG in topical burn wounds caused by MRSA bacteria. Results showed a rate of wound shrinkage (78.23 ± 5.65%) and faster re-epithelialization in the SSD-EOOG-treated group. This study concluded that egg oil-based organogel supports the therapeutic efficacy of SSD for burn wound treatment (Thakur et al., 2020).
A new bigel was synthesized by mixing guar gum hydrogel and sorbitan monostearate-sesame oil-based organogel and evaluated as a controlled drug release system (Singh et al., 2014). Macro-scale deformation studies, viscometric analysis, shear-thinning, and viscoelastic structure of bigels were investigated. With the increase in oleogel content, bigels were smooth, stable, and biocompatible, with higher viscosity and hardness. In in vitro drug release experiments, it was observed that the amount of ciprofloxacin drug release increased with the decrease in organogel content. In addition, it has been found that drug release from all bigels conforms to the desired zero-order diffusion kinetics for a controlled release system. When drug-loaded bigels were examined in terms of antimicrobial activity, they had a good effect against Bacillus subtilis, and it was predicted that they could be considered a drug release system.
Zeng et al. (2021) found that the effective interfacial function of organogels can maintain low adhesion for months, even under harsh outdoor conditions. Superior surface property is critically dependent on the continuous renewal of the liquid layer from the matrix to the surface, creating unique micro-/nano-structures in the matrix, achieving a structural gradient, making them a superior surface for droplet manipulation. With their low modulus, organogels are widely researched for practical application as anti-icing and anti-fouling coatings. The biocompatibility, biodegradability, and functionality of organogelators and solvents enable the hybridization and stabilization of organogels with bioactive substances in microporous matrices, imparting thermodynamic behavior rheological properties for drug release and cosmetic potentials. Edible artificial organogels structured with vegetable oils for fat replacement in food processing have been developed by utilizing the solid-like physicochemical property. Organogels have an obvious disadvantage due to organic solvent. Its main component, the high-priced organic solvent, is costly in organogel production, leaving it out of various practical applications. Furthermore, interface interactions are considered weak if they act as interface material due to their low adhesive strength to substrates, especially organogels with low viscosity solvent. Amphiphilic organogels and the addition of a binder coating may be suitable options for strong binding to different materials. Poor mechanical performance limits organogels for use in various applications (Zeng et al., 2021).
A new in situ gel system was prepared using soybean oil, stearic acid, and N-methyl-2-pyrrolidinone (10:1:3, v/w/v). The gel was in a low viscosity sol when applied to the conjunctival sac and then quickly gelled in the eye, and in vivo experiments have shown that organogel-flunarizine hydrochloride has bioavailability, low ocular irritation, and biocompatibility, properties suitable for intraocular administration. The release of the organogel-flunarizine hydrochloride can be prolonged by 48 h. Pharmacokinetics in rats showed that the organogel helped maintain the effective organogel concentration in the body and slowed the rate of drug clearance compared to flunarizine hydrochloride solution. Organogel-flunarizine hydrochloride is a promising drug delivery system with therapeutic efficacy for treating brain diseases by intraocular administration (Dai et al., 2020).
An organogel-based nanoemulsion has been developed to effectively deliver hydrophobic drugs with organogel droplets that can gel in situ. A combination of G/W nanoemulsion, lipiodol, and organogelator 12-hydroxystearic acid (12-HSA) was used, stabilized with polyoxyethylene hydrogenated castor oil (HCO-60) as a surfactant. The prepared nanoemulsion has a high drug loading efficiency (∼97%) with an average diameter of 206 nm. This drug delivery system was novel in terms of organogel structure with thermo-reversible property and stability over a long period of time. The prepared nanoemulsion can effectively encapsulate and deliver hydrophobic drugs at the application site. The physicochemical properties of the organogel-based nanoemulsion were not affected by the storage temperature, and the in vitro study did not affect the mitochondrial and metabolic activities of primary rat hepatocytes. Both in vitro and in vivo studies determined the cytotoxicity of paclitaxel-containing organogel-based nanoemulsion against skin cancer. Therefore, organogel-based nanoemulsion can be a drug carrier in the body, and organogel-based nanoemulsion can be used to treat melanoma with fewer side effects and site-specificity (Fardous et al., 2021). A transdermal drug delivery system (TDDS) for circulatory organogels was recently developed with new evaluation in propylene glycol. The G′ value of the poly(VbNMDG)-Aq gel is 950 Pa, and the poly(VbNMDG)-PG gel is suitable for use as a transdermal depot. In permeability, the auxiliary aid limonene has a synergistic effect on limonene/PG. The poly(VbNMDG) gel can be evaluated for optimal testability for 24 h depending on the conditions. Overall, the poly(VbNMDG)-PG gel formed possible polymer–polymer interaction with the new organogel, which can improve the permeability of transdermal testosterone and be compatible with the penetration enhancing system (Charoensumran and Ajiro, 2020). The in situ organogels based on amino acid (the four amino-based gelator: N-icosanoyl-l-alanine methyl ester (C20-Ala-Me) N-icosanoyl-l-glutamate dimethyl ester (C20-Glu-Me), N-icosanoyl-l-serine methyl ester (C20-Ser-Me), and N-arachidonoyl-l-alanine ethyl ester (C20-Ala-Et)) derivatives were synthesized as biocompatible and biodegradable, the optimal formulation was investigated based on the central composite design experiments, and the risperidone-loaded organogel was prepared. It showed that risperidone-organogel could provide a release for up to 20 days. Compared with the drug oil solution, the drug concentration in the blood was prolonged from 1 day to 7 days, showing that the release of risperidone-organogel conforms to the Ritger–Peppas model and is a combined action of drug diffusion and erosion (Hu et al., 2020).
A new magnetic micro-organogel with a protein shell and oil core was prepared. The magnetic micro-organogel synthesized by utilizing the hydrophilicity and flexibility of the protein shell has a suitable dispersibility in water. In this study, superparamagnetism of OA-Fe3O4 in the oil core showed the easily accessible mobility of the magnetic micro-organogel for targeted drug delivery. The high loading efficiency of the magnetic micro-organogel for hydrophobic drugs is demonstrated by the high encapsulation rate of Coumarin 6. The magnetic micro-organogel exhibited thermosensitive and GSH-sensitive behavior. Release mechanisms included erosion, diffusion, and degradation. The magnetic micro-organogel was shown to be a potential material for high-efficiency delivery and stimuli-responsive release of hydrophobic drugs (Dong et al., 2021). The carrier property of organogel-based nanogel disperse (NGD) with 12-hydroxystearic acid and lipiodol for paclitaxel anti-cancer drug was investigated in vitro and in vivo. Organogel gel (G/W) nano-dispersion in water with 12-hydroxystearic acid and lipiodol was synthesized by the ultrasonication method and stabilized with a non-ionic surfactant. Paclitaxel-loaded NGDs were found to be biocompatible with mouse hepatocytes and fibroblast cells in vitro, while paclitaxel-loaded G/W nano-dispersion showed cytotoxicity (p < 0.05) against lung cancer (A549) cell lines. Intravenous administration of paclitaxel-loaded NGDs shows an anti-cancer effect against lung cancer in vivo with a significant reduction in tumor volume (p < 0.05). Paclitaxel-loaded NGDs may be a promising carrier for sustained drug release and chemotherapy agents (Fardous et al., 2022).
Sagiri et al. (2015) used the ionotropic gelation method to synthesize organogel-entrapped core-shell (organogel-alginate) type new microparticles. It has been used in controlled drug release applications. The encapsulation of the organogels was confirmed by microscopic, XRD, FTIR, and DSC studies. Encapsulation of the organogel in microparticles prevented the leakage of the inner phase and increased the efficiency of drug encapsulation. In in vitro drug release studies, ciprofloxacin was used as a model drug and was continuously released from the organogel containing microparticles. In addition, the biocompatibility of the microparticles was tested by hemocompatibility and cytocompatibility studies and found to have antimicrobial activity against Escherichia coli. Drug release data showed that the release occurred by super case-II diffusion. The developed core-shell type hybrid microparticles are thought to be promising for controlled drug release systems (Sagiri et al., 2015).
In a study by Kodela et al. (2017), bigels containing different ratios of agar gel and stearyl alcohol were prepared as oleogel type in the hydrogel. Thus, the inherent stability of bigel-based formulations, biphasic formulations, and gel-based formulations is combined. In addition, the increase in oleogel ratio caused the formation of microscopic structures similar to biphasic formulations, greatly reducing the hydrogen bonding in the formulations and the electrical stability of the formulations. The mechanical properties of bigels increased with the addition of oleogel into the agar hydrogel until the critical concentration was reached and started to decrease after reaching this critical point. The inherent electrical stability of bigels was found to be lower compared to hydrogels. Ciprofloxacin hydrochloride drug release from bigels was analyzed by the Korsmeyer–Pappas and Peppas–Sahlin models, and Fickian diffusion was found dominant in general. The results suggest that the mechanical, electrical, and drug-release properties of drugs can be improved by changes in their composition and can be used in food and pharmaceutical applications (Kodela et al., 2017).
In this study, bigels were synthesized using an oleogel based on stearic acid/rice bran oil and tamarind gum hydrogel. The oleogel portion was prepared by mixing stearic acid and rice bran oil, whereas the hydrogel portion was prepared from the mixture of tamarind gum and hydroethanol solution. Analysis showed that bigels exhibited the presence of stearic acid melting endotherm (oleogel associated) and water evaporation endotherm (hydrogel associated), and the hydrogel had the lowest bulk resistivity compared to other formulations. It has been observed that the bulk electrical resistance of bigels is controlled by the oleogel phase and there is an increase in the diffusion of moxifloxacin HCl from the formulations with the increase of the hydrogel ratio. It has been predicted that the bigels with the developed formulations can be used as a potential drug release material (Paul et al., 2018). A schematic representation of organogel preparation, drug loading step, and implementation in film and injectable forms are given in Figure 1.
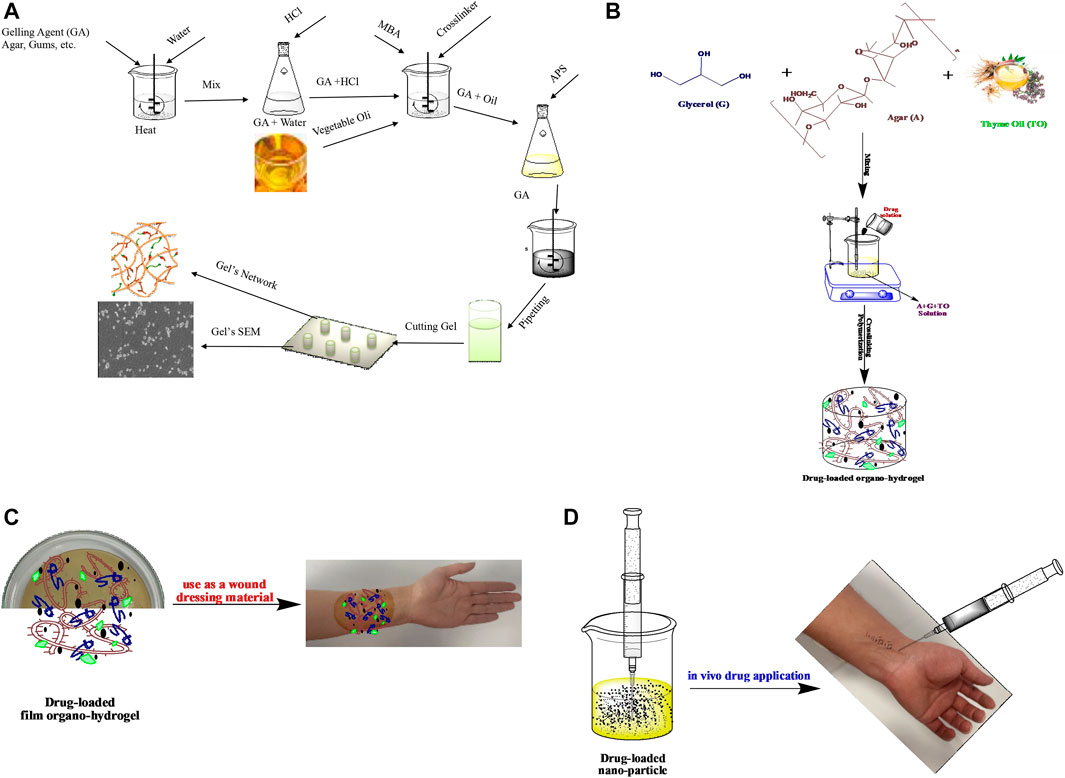
FIGURE 1. (A) Schematic representation of organogels preparation. (B) Schematic representation of drug loading to organogels. (C) Application of drug-loaded organogels in a film form. (D) Application of drug-loaded organogels in an injectable form (in nano-scale).
Conclusion
In this mini-review, we have focused on some recent developments of drug delivery platforms, particularly organogels based applications. We tried to give a point of view of state of the art. It is expected that organogels will be utilized at a gradually increasing level in the coming years because of their unique characteristics such as biocompatibility, being easily tunable, tailoring by chemical modifications. These properties enable organogels to be applied with enormous potential applications in drug delivery, anti-icing, anti-fouling, food processing, and more.
Author Contributions
All authors listed have made a substantial, direct, and intellectual contribution to the work and approved it for publication.
Conflict of Interest
The authors declare that the research was conducted in the absence of any commercial or financial relationships that could be construed as a potential conflict of interest.The reviewer AT declared a shared affiliation, with collaboration, with the authors to the handling editor at the time of the review.
Publisher’s Note
All claims expressed in this article are solely those of the authors and do not necessarily represent those of their affiliated organizations or those of the publisher, the editors, and the reviewers. Any product that may be evaluated in this article, or claim that may be made by its manufacturer, is not guaranteed or endorsed by the publisher.
References
Akkas, T., Citak, C., Sirkecioglu, A., and Güner, F. S. (2013). Which Is More Effective for Protein Adsorption: Surface Roughness, Surface Wettability or Swelling? Case Study of Polyurethane Films Prepared from castor Oil and Poly(ethylene Glycol). Polym. Int. 62 (8), 1202–1209. doi:10.1002/pi.4408
Alpaslan, D., Erşen Dudu, T. E., and Aktaş, N. (2021c). Synthesis and Characterization of Novel Organo-Hydrogel Based agar, Glycerol and Peppermint Oil as a Natural Drug Carrier/release Material. Mater. Sci. Eng. C 118, 111534. doi:10.1016/j.msec.2020.111534
Alpaslan, D., Erşen Dudu, T., and Aktas, N. (2021a). Development of Onion Oil-Based Organo-Hydrogel for Drug Delivery Material. J. Dispersion Sci. Technol., 1–13. doi:10.1080/01932691.2021.1974869
Alpaslan, D., Ersen Dudu, T., and Aktas, N. (2021b). Evaluation of poly(agar-co-glycerol-co-castor Oil) Organo-Hydrogel as a Controlled Release System Carrier Support Material. Polym. Bull., 9. doi:10.1007/s00289-021-03777-9
Alpaslan, D., Olak, T., Turan, A., Ersen Dudu, T., and Aktas, N. (2021d). A Garlic Oil-Based Organo-Hydrogel for Use in pH-Sensitive Drug Release. Chem. Pap. 75, 5759–5772. doi:10.1007/s11696-021-01760-2
Alpaslan, D., Olak, T., Turan, A., Ersen Dudu, T., and Aktas, N. (2021e). Use of Coconut Oil-Based Organo-Hydrogels in Pharmaceutical Applications. J. Polym. Environ. 30, 666–680. doi:10.1007/s10924-021-02219-x
Alsaab, H., Bonam, S. P., Bahl, D., Chowdhury, P., Alexander, K., and Boddu, S. H. (2016). Organogels in Drug Delivery: A Special Emphasis on Pluronic Lecithin Organogels. J. Pharm. Pharm. Sci. 19 (2), 252–273. doi:10.18433/J3V89W
Anderson, J. M., Rodriguez, A., and Chang, D. T. (2008). Foreign Body Reaction to Biomaterials. Semin. Immunol. 20 (2), 86–100. doi:10.1016/j.smim.2007.11.004
Anderson, J. M., and Shive, M. S. (2012). Biodegradation and Biocompatibility of PLA and PLGA Microspheres. Adv. Drug Deliv. Rev. 64, 72–82. doi:10.1016/j.addr.2012.09.004
Angelova, N., and Hunkeler, D. (1999). Rationalizing the Design of Polymeric Biomaterials. Trends Biotechnol. 17 (10), 409–421. doi:10.1016/s0167-7799(99)01356-6
Bell, C. L., and Peppas, N. A. (1995). “Biomedical Membranes from Hydrogels and Interpolymer Complexes,” in Biopolymers Ii. Editors N. A. Peppas&R, and S. Langer (Berlin/Heidelberg: Springer-Verlag), Advences in Polymer Science, Vol. 122. doi:10.1007/3540587888_15
Bernard, M., Jubeli, E., Pungente, M. D., and Yagoubi, N. (2018). Biocompatibility of Polymer-Based Biomaterials and Medical Devices - Regulations,in Vitroscreening and Risk-Management. Biomater. Sci. 6 (8), 2025–2053. doi:10.1039/c8bm00518d
Bernhard, S., and Tibbitt, M. W. (2021). Supramolecular Engineering of Hydrogels for Drug Delivery. Adv. Drug Deliv. Rev. 171, 240–256. doi:10.1016/j.addr.2021.02.002
Borchard, G., and Junginger, H. E. (2001). Modern Drug Delivery Applications of Chitosan. Adv. Drug Deliv. Rev. 52 (2), 103. doi:10.1016/s0169-409x(01)00188-0
Cadée, J. A., Brouwer, L. A., den Otter, W., Hennink, W. E., and van Luyn, M. J. (2001). A Comparative Biocompatibility Study of Microspheres Based on Crosslinked Dextran or Poly(lactic-Co-Glycolic)acid after Subcutaneous Injection in Rats. J. Biomed. Mater. Res. 56 (4), 600–609. doi:10.1002/1097-4636(20010915)56:4<600::aid-jbm1133>3.0.co;2-i
Charoensumran, P., and Ajiro, H. (2020). Controlled Release of Testosterone by Polymer-Polymer Interaction Enriched Organogel as a Novel Transdermal Drug Delivery System: Effect of Limonene/PG and Carbon-Chain Length on Drug Permeability. Reactive Funct. Polym. 148, 104461. doi:10.1016/j.reactfunctpolym.2019.104461
Dai, M., Bai, L., Zhang, H., Ma, Q., Luo, R., Lei, F., et al. (2020). A Novel Flunarizine Hydrochloride-Loaded Organogel for Intraocular Drug Delivery In Situ: Design, Physicochemical Characteristics and Inspection. Int. J. Pharmaceutics 576, 119027. doi:10.1016/j.ijpharm.2020.119027
Dalsin, J. L., Hu, B.-H., Lee, B. P., and Messersmith, P. B. (2003). Mussel Adhesive Protein Mimetic Polymers for the Preparation of Nonfouling Surfaces. J. Am. Chem. Soc. 125 (14), 4253–4258. doi:10.1021/ja0284963
Dong, J., Du, X., Zhang, Y., Zhuang, T., Cui, X., and Li, Z. (2021). Thermo/glutathione-sensitive Release Kinetics of Heterogeneous Magnetic Micro-organogel Prepared by Sono-Catalysis. Colloids Surf. B: Biointerfaces 208, 112109. doi:10.1016/j.colsurfb.2021.112109
Downes, S., and Mishra, A. A. (2011). Tissue-biomaterial Interactions. Adv. Wound Repair Therapies, 174–185. doi:10.1533/9780857093301.2.174
Draye, J.-P., Delaey, B., Van de Voorde, A., Van Den Bulcke, A., De Reu, B., and Schacht, E. (1998). In Vitro and In Vivo Biocompatibility of Dextran Dialdehyde Cross-Linked Gelatin Hydrogel Films. Biomaterials 19 (18), 1677–1687. doi:10.1016/s0142-9612(98)00049-0
Elsayed, M. M. A., El Rasoul, S. A., Ramadan, A. E. h., and Hussein, A. K. (2019). Response Surface Methodology as a Useful Tool for Development and Optimization of Sustained Release Ketorolac Tromethamine Niosomal Organogels. J. Pharm. Innov. 15 (4), 664–677. doi:10.1007/s12247-019-09421-4
Ersen Dudu, T., Alpaslan, D., and Aktas, N. (2021). Application of Poly (Agar-Co-Glycerol-Co-Sweet Almond Oil) Based Organo-Hydrogels as a Drug Delivery Material. J. Polym. Environ. 30, 483–493. doi:10.1007/s10924-021-02212-4
Esposito, C. L., Kirilov, P., and Roullin, V. G. (2018). Organogels, Promising Drug Delivery Systems: an Update of State-Of-The-Art and Recent Applications. J. Controlled Release 271, 1–20. doi:10.1016/j.jconrel.2017.12.019
Fardous, J., Omoso, Y., Joshi, A., Yoshida, K., Patwary, M. K. A., Ono, F., et al. (2021). Development and Characterization of Gel-In-Water Nanoemulsion as a Novel Drug Delivery System. Mater. Sci. Eng. C 124, 112076. doi:10.1016/j.msec.2021.112076
Fardous, J., Omoso, Y., Yoshida, K., Ono, F., Patwary, M. K. A., and Ijima, H. (2022). Gel-in-water Nanodispersion for Potential Application in Intravenous Delivery of Anticancer Drugs. J. Biosci. Bioeng. 133, 174–180. doi:10.1016/j.jbiosc.2021.10.001
Finlayson, J. (1893). Ancient Egyptian Medicine: [A Bibliographical Demonstration in the Library of the Faculty of Physicians and Surgeons, Glasgow, January 12th, 1893.]. BMJ 1 (1684), 748–752. doi:10.1136/bmj.1.1684.748
Fournier, E., Passirani, C., Montero-Menei, C. N., and Benoit, J. P. (2003). Biocompatibility of Implantable Synthetic Polymeric Drug Carriers: Focus on Brain Biocompatibility. Biomaterials 24 (19), 3311–3331. doi:10.1016/s0142-9612(03)00161-3
Garner, I., Vichare, R., Paulson, R., Appavu, R., Panguluri, S. K., Tzekov, R., et al. (2020). Carbon Dots Fabrication: Ocular Imaging and Therapeutic Potential. Front. Bioeng. Biotechnol. 8, 8. doi:10.3389/fbioe.2020.573407
Hassan, C. M., and Peppas, N. A. (2000). “Structure and Applications of Poly(vinyl Alcohol) Hydrogels Produced by Conventional Crosslinking or by Freezing/thawing Methods,” in Biopolymers/Pva Hydrogels/Anionic Polymerisation Nanocomposites. Editor A. Abe (Cham, Switzerland: Springer International Publishing AG).
Hu, B., Yan, H., Sun, Y., Chen, X., Sun, Y., Li, S., et al. (2020). Organogels Based on Amino Acid Derivatives and Their Optimization for Drug Release Using Response Surface Methodology. Artif. Cell Nanomedicine, Biotechnol. 48 (1), 266–275. doi:10.1080/21691401.2019.1699833
Jabbari, E., and Peppas, N. A. (1994). Polymer-polymer Interdiffusion and Adhesion. J. Macromolecular Sci. C: Polym. Rev. 34 (2), 205–241. doi:10.1080/15321799408009635
Kakkar, A., Traverso, G., Farokhzad, O. C., Weissleder, R., and Langer, R. (2017). Evolution of Macromolecular Complexity in Drug Delivery Systems. Nat. Rev. Chem. 1 (8). doi:10.1038/s41570-017-0063
Khor, E., and Lim, L. Y. (2003). Implantable Applications of Chitin and Chitosan. Biomaterials 24 (13), 2339–2349. doi:10.1016/s0142-9612(03)00026-7
Kodela, S. P., Pandey, P. M., Nayak, S. K., Uvanesh, K., Anis, A., and Pal, K. (2017). Novel agar-stearyl Alcohol Oleogel-Based Bigels as Structured Delivery Vehicles. Int. J. Polymeric Mater. Polymeric Biomater. 66 (13), 669–678. doi:10.1080/00914037.2016.1252362
Koetting, M. C., and Peppas, N. A. (2014). Stimuli-responsive Hydrogels for Oral Delivery of High Isoelectric point-exhibiting Therapeutic Proteins. Abstr. Pap. Am. Chem. Soc. 247, 247.
Koetting, M. C., Peters, J. T., Steichen, S. D., and Peppas, N. A. (2015). Stimulus-responsive Hydrogels: Theory, Modern Advances, and Applications. Mater. Sci. Eng. R: Rep. 93, 1–49. doi:10.1016/j.mser.2015.04.001
Kohane, D. S., and Langer, R. (2010). Biocompatibility and Drug Delivery Systems. Chem. Sci. 1 (4), 441–446. doi:10.1039/c0sc00203h
Langer, R., and Peppas, N. (1983). Chemical and Physical Structure of Polymers as Carriers for Controlled Release of Bioactive Agents: A Review. J. Macromolecular Sci. C 23 (1), 61–126. doi:10.1080/07366578308079439
Langer, R., and Peppas, N. (1981). Present and Future Applications of Biomaterials in Controlled Drug Delivery Systems. Biomaterials 2 (4), 201–214. doi:10.1016/0142-9612(81)90059-4
Liu, D.-E., Chen, Q., Long, Y.-B., Ma, J., and Gao, H. (2018). A Thermo-Responsive Polyurethane Organogel for Norfloxacin Delivery. Polym. Chem. 9 (2), 228–235. doi:10.1039/c7py01803g
Martin, B., Brouillet, F., Franceschi, S., and Perez, E. (2017). Evaluation of Organogel Nanoparticles as Drug Delivery System for Lipophilic Compounds. Aaps Pharmscitech 18 (4), 1261–1269. doi:10.1208/s12249-016-0587-y
Mitchell, M. J., Billingsley, M. M., Haley, R. M., Wechsler, M. E., Peppas, N. A., and Langer, R. (2021). Engineering Precision Nanoparticles for Drug Delivery. Nat. Rev. Drug Discov. 20 (2), 101–124. doi:10.1038/s41573-020-0090-8
Olak, T., Turan, A., Alpaslan, D., Dudu, T. E., and Aktaş, N. (2020). Developing poly(Agar-co-Glycerol-co-Thyme Oil) Based Organo-Hydrogels for the Controlled Drug Release Applications. J. Drug Deliv. Sci. Technol. 60, 102088. doi:10.1016/j.jddst.2020.102088
Onuki, Y., Bhardwaj, U., Papadimitrakopoulos, F., and Burgess, D. J. (2008). A Review of the Biocompatibility of Implantable Devices: Current Challenges to Overcome Foreign Body Response. J. Diabetes Sci. Technol. 2 (6), 1003–1015. doi:10.1177/193229680800200610
Park, H., and Park, K. (1996). Biocompatibility Issues of Implantable Drug Delivery Systems. Pharm. Res. 13 (12), 1770–1776. doi:10.1023/a:1016012520276
Paul, S. R., Qureshi, D., Yogalakshmi, Y., Nayak, S. K., Singh, V. K., Syed, I., et al. (2018). Development of Bigels Based on Stearic Acid-Rice Bran Oil Oleogels and Tamarind Gum Hydrogels for Controlled Delivery Applications. J. Surfactants Deterg 21 (1), 17–29. doi:10.1002/jsde.12022
Peer, D., Karp, J. M., Hong, S., FaroKhzad, O. C., Margalit, R., and Langer, R. (2007). Nanocarriers as an Emerging Platform for Cancer Therapy. Nat. Nanotech 2 (12), 751–760. doi:10.1038/nnano.2007.387
Peppas, N. A., Hilt, J. Z., Khademhosseini, A., and Langer, R. (2006). Hydrogels in Biology and Medicine: From Molecular Principles to Bionanotechnology. Adv. Mater. 18 (11), 1345–1360. doi:10.1002/adma.200501612
Peppas, N. A., Huang, Y., Torres-Lugo, M., Ward, J. H., and Zhang, J. (2000). Physicochemical Foundations and Structural Design of Hydrogels in Medicine and Biology. Annu. Rev. Biomed. Eng. 2, 9–29. doi:10.1146/annurev.bioeng.2.1.9
Peppas, N. A., and Sahlin, J. J. (1996). Hydrogels as Mucoadhesive and Bioadhesive Materials: A Review. Biomaterials 17 (16), 1553–1561. doi:10.1016/0142-9612(95)00307-x
Peppas, N. A. (1982). Structure, Testing, and Applications of Biomaterials. Adv. Chem. Ser. 199, 465–473. doi:10.1021/ba-1982-0199.ch028
Querobino, S. M., de Faria, N. C., Vigato, A. A., da Silva, B. G. M., Machado, I. P., Costa, M. S., et al. (2019). Sodium Alginate in Oil-Poloxamer Organogels for Intravaginal Drug Delivery: Influence on Structural Parameters, Drug Release Mechanisms, Cytotoxicity and In Vitro Antifungal Activity. Mater. Sci. Eng. C 99, 1350–1361. doi:10.1016/j.msec.2019.02.036
Ronneberger, B., Kao, W. J., Anderson, J. M., and Kissel, T. (1996). In Vivo biocompatibility Study of ABA Triblock Copolymers Consisting of poly(L-Lactic-Co-Glycolic Acid) A Blocks Attached to central Poly(oxyethylene) B Blocks. J. Biomed. Mater. Res. 30 (1), 31–40. doi:10.1002/(sici)1097-4636(199601)30:1<31::aid-jbm5>3.0.co;2-s
Ronneberger, B., Kissel, T., and Anderson, J. M. (1997). Biocompatibility of ABA Triblock Copolymer Microparticles Consisting of poly(L-Lactic-Co-Glycolic-Acid) A-Blocks Attached to central Poly(oxyethylene) B-Blocks in Rats after Intramuscular Injection. Eur. J. Pharmaceutics Biopharmaceutics 43 (1), 19–28. doi:10.1016/s0939-6411(96)00006-9
Sadeghi, I., Byrne, J., Shakur, R., and Langer, R. (2021). Engineered Drug Delivery Devices to Address Global Health Challenges. J. Controlled Release 331, 503–514. doi:10.1016/j.jconrel.2021.01.035
Sadtler, K., Collins, J., Byrne, J. D., and Langer, R. (2020). Parallel Evolution of Polymer Chemistry and Immunology: Integrating Mechanistic Biology with Materials Design. Adv. Drug Deliv. Rev. 156, 65–79. doi:10.1016/j.addr.2020.06.021
Sagbas, S., Aktas, N., and Sahiner, N. (2015). Modified Biofunctional P(tannic Acid) Microgels and Their Antimicrobial Activity. Appl. Surf. Sci. 354, 306–313. doi:10.1016/j.apsusc.2015.06.163
Sagiri, S. S., Singh, V. K., Banerjee, I., Pramanik, K., Basak, P., and Pal, K. (2015). Core-shell-type Organogel-Alginate Hybrid Microparticles: A Controlled Delivery Vehicle. Chem. Eng. J. 264, 134–145. doi:10.1016/j.cej.2014.11.032
Sahiner, N., Ozay, O., and Aktas, N. (2011). 4-Vinylpyridine-Based Smart Nanoparticles with N-Isopropylacrylamide, 2-Hydroxyethyl Methacrylate, Acrylic Acid, and Methacrylic Acid for Potential Biomedical Applications. Cnano 7 (3), 453–462. doi:10.2174/157341311795542507
Sahiner, N., Sagbas, S., Sahiner, M., Blake, D. A., and Reed, W. F. (2018). Polydopamine Particles as Nontoxic, Blood Compatible, Antioxidant and Drug Delivery Materials. Colloids Surf. B: Biointerfaces 172, 618–626. doi:10.1016/j.colsurfb.2018.09.019
Sahiner, N., Sagbas, S., Sahiner, M., and Silan, C. (2017). P(TA) Macro-, Micro-, Nanoparticle-Embedded Super Porous P(HEMA) Cryogels as Wound Dressing Material. Mater. Sci. Eng. C 70, 317–326. doi:10.1016/j.msec.2016.09.025
Sahiner, N. (2021). Self-Crosslinked Ellipsoidal Poly(Tannic Acid) Particles for Bio-Medical Applications. Molecules 26 (9), 2429. doi:10.3390/molecules26092429
Sahiner, N., Suner, S. S., and Ayyala, R. S. (2019). Mesoporous, Degradable Hyaluronic Acid Microparticles for Sustainable Drug Delivery Application. Colloids Surf. B: Biointerfaces 177, 284–293. doi:10.1016/j.colsurfb.2019.02.015
Suner, S. S., Demirci, S., Yetiskin, B., Fakhrullin, R., Naumenko, E., Okay, O., et al. (2019). Cryogel Composites Based on Hyaluronic Acid and Halloysite Nanotubes as Scaffold for Tissue Engineering. Int. J. Biol. Macromolecules 130, 627–635. doi:10.1016/j.ijbiomac.2019.03.025
Suner, S. S., Sahiner, M., Sengel, S. B., Rees, D. J., Reed, W. F., and Sahiner, N. (2018). Responsive Biopolymer-Based Microgels/nanogels for Drug Delivery Applications. Stimuli Responsive Polymeric Nanocarriers Drug Deliv. Appl. Types Triggers 1, 453–500. doi:10.1016/b978-0-08-101997-9.00021-7
Thakur, K., Mahajan, A., Sharma, G., Singh, B., Raza, K., Chhibber, S., et al. (2020). Implementation of Quality by Design (QbD) Approach in Development of Silver Sulphadiazine Loaded Egg Oil Organogel: An Improved Dermatokinetic Profile and Therapeutic Efficacy in Burn Wounds. Int. J. Pharmaceutics 576, 118977. doi:10.1016/j.ijpharm.2019.118977
Thevenot, P., Hu, W., and Tang, L. (2008). Surface Chemistry Influences Implant Biocompatibility. Curr. Top. Med. Chem. 8 (4), 270–280. doi:10.2174/156802608783790901
Tokuyama, H., and Kato, Y. (2010). Preparation of Thermosensitive Polymeric Organogels and Their Drug Release Behaviors. Eur. Polym. J. 46 (2), 277–282. doi:10.1016/j.eurpolymj.2009.10.016
Tsai, W.-B., Grunkemeier, J. M., McFarland, C. D., and Horbett, T. A. (2002). Platelet adhesion to polystyrene-based surfaces preadsorbed with plasmas selectively depleted in fibrinogen, fibronectin, vitronectin, or von Willebrand's factor. J. Biomed. Mater. Res. 60 (3), 348–359. doi:10.1002/jbm.10048
Uchegbu, I. F., Schätzlein, A. G., Tetley, L., Gray, A. I., Sludden, J., Siddique, S., et al. (2011). Polymeric Chitosan-Based Vesicles for Drug Delivery. J. Pharm. Pharmacol. 50 (5), 453–458. doi:10.1111/j.2042-7158.1998.tb06185.x
Uhrich, K. E., Cannizzaro, S. M., Langer, R. S., and Shakesheff, K. M. (1999). Polymeric Systems for Controlled Drug Release. Chem. Rev. 99 (11), 3181–3198. doi:10.1021/cr940351u
Uzan, S., Barış, D., Çolak, M., Hoşgören, H., and Hosgoren, H. (2016). Organogels as Novel Carriers for Dermal and Topical Drug Delivery Vehicles. Tetrahedron 72 (47), 7517–7525. doi:10.1016/j.tet.2016.10.009
Vercruysse, K. P., and Prestwich, G. D. (1998). Hyaluronate Derivatives in Drug Delivery. Crit. Rev. Ther. Drug Carrier Syst. 15 (5), 513–555. doi:10.1615/critrevtherdrugcarriersyst.v15.i5.30
Vichare, R., Garner, I., Paulson, R. J., Tzekov, R., Sahiner, N., Panguluri, S. K., et al. (2020). Biofabrication of Chitosan-Based Nanomedicines and its Potential Use for Translational Ophthalmic Applications. Appl. Sci. 10 (12), 4189. doi:10.3390/app10124189
Wang, Y.-X., Robertson, J. L., Spillman, Jr., W. B., and Claus, R. O. (2004). Effects of the Chemical Structure and the Surface Properties of Polymeric Biomaterials on Their Biocompatibility. Pharm. Res. 21 (8), 1362–1373. doi:10.1023/b:pham.0000036909.41843.18
Webber, M. J., and Langer, R. (2017). Drug Delivery by Supramolecular Design. Chem. Soc. Rev. 46 (21), 6600–6620. doi:10.1039/c7cs00391a
Wichterle, O., and Lim, D. (1960). Hydrophilic Gels for Biological Use. Nature 185 (4706), 2. doi:10.1038/185117a0
Wichterle, O., and Lím, D. (1960). Hydrophilic Gels for Biological Use. Nature 185 (4706), 117–118. doi:10.1038/185117a0
Zeng, L., Lin, X., Li, P., Liu, F.-Q., Guo, H., and Li, W.-H. (2021). Recent Advances of Organogels: from Fabrications and Functions to Applications. Prog. Org. Coat. 159, 106417. doi:10.1016/j.porgcoat.2021.106417
Keywords: biomaterials, organogel, drug delivery, hydrogels, medical application, responsive polymers, smart materials
Citation: Aktas N, Alpaslan D and Dudu TE (2022) Polymeric Organo-Hydrogels: Novel Biomaterials for Medical, Pharmaceutical, and Drug Delivery Platforms. Front. Mater. 9:845700. doi: 10.3389/fmats.2022.845700
Received: 30 December 2021; Accepted: 24 February 2022;
Published: 18 March 2022.
Edited by:
Nafisa Gull, University of the Punjab, PakistanReviewed by:
Dursun Saraydin, Cumhuriyet University, TurkeyMusa Kamaci, Piri Reis University, Turkey
Abdullah Turan, Yüzüncü Yıl University, Turkey
Copyright © 2022 Aktas, Alpaslan and Dudu. This is an open-access article distributed under the terms of the Creative Commons Attribution License (CC BY). The use, distribution or reproduction in other forums is permitted, provided the original author(s) and the copyright owner(s) are credited and that the original publication in this journal is cited, in accordance with accepted academic practice. No use, distribution or reproduction is permitted which does not comply with these terms.
*Correspondence: Nahit Aktas, bmFoaXQuYWt0YXNAbWFuYXMuZWR1Lmtn