- 1Department of Mechanical Engineering, Shiv Nadar University, Delhi, India
- 2Department of Mechanical Engineering, Shiv Nadar University, Delhi, India
Biomimetic and soft robotics research has been inspired by many biological organisms focusing on soft structural composites reinforced by stiff constituents. In the case of synthetic soft structural materials, hydrogels are very similar to the biological soft tissues/organs, owing to their “wet and soft” properties, their elastic performance, biodegradability, and biocompatibility. However, the current scope of hydrogel applications is usually hampered by their feeble mechanical properties. Although significant progress has been made in developing intense, challenging, and stretchable hydrogels, it is still challenging to realize the optimal magnitude of physical and mechanical properties altogether in synthetic hydrogels. This review briefly focuses on the hydrogel fibrous shape-morphing composite structure and its functionalities. The nature-inspired, fiber-reinforced soft hydrogel shape-morphing functional composite structure delivers them a cartilage-like anisotropic behavior. They could have broad potential applications in various areas, including tissue engineering, soft robotics, stretchable and bio-integrated electronics, microfluidics, and biomedical devices.
Introduction
Nature has advanced into an assortment of delicate materials that might alter their shapes, morphology, and valuable properties in reaction to the inside or natural signals (Capadona et al., 2008; Fratzl and Barth, 2009; Armon et al., 2011; Studart, 2015; van Rees et al., 2017). Actuators are any device that can generate mechanical force/motion. In the past decades, various soft actuating materials have been studied (Sahoo et al., 2005) for different applications. Propelled by such materials, analysts have created stimulus-responsive, shape-morphing materials utilizing shape-memory polymers, fluid crystalline polymers, hydrogels (Wu et al., 2013; Sydney Gladman et al., 2016; Goyal et al., 2019), etc.
Among these materials, hydrogels are a promising material for bio-inspired and biomedical applications, such as delicate mechanical autonomy, counterfeit muscles, and keen pharmaceuticals owing to their physical properties compared to organic, soft tissues (Kim et al., 2012; Wu et al., 2013; Sydney Gladman et al., 2016; Cangialosi et al., 2017).
Hydrogels are in the family of three-dimensional (3D) polymeric materials that have promising applications due to their superior biocompatibility, responsiveness to environmental stimuli, high water content, and tunable physicochemical and structural properties (Dawson et al., 1997; Reyssat and Mahadevan, 2009; Roche et al., 2017). Figure 1 highlights the research interest in these kinds of gels and their responsive ability when subjected to external stimuli and applicability in various scientific disciplines since the early 1990s.
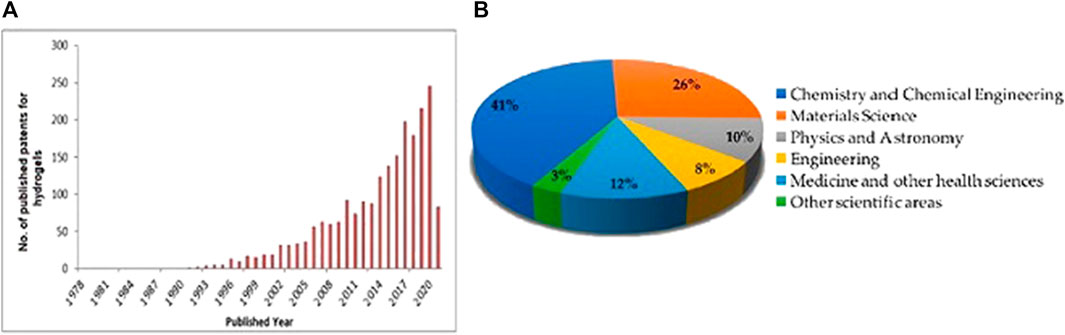
FIGURE 1. (A) Number of publications per year and (B) publication by discipline leading stimulus-responsive gel (Echeverria et al., 2018). Copyright 2018, gels MDPI.
Hydrogels with a large amount of water substance (more than 90%) can quickly alter their shape and morphology through swelling/deswelling in the polymer network with a change in the water content within the hydrogel, which can be stimulated by various external or internal ambient stimuli such as temperature, light, pH, chemicals, and biomolecules (Calvert, 2009; Sydney Gladman et al., 2016; Sano et al., 2018). The mechanical properties of the hydrogel matrices can be modified and optimized by altering the polymer matrix compositions adapting to the specific clinical needs (Peppas et al., 2006; Ionov, 2013). These features make the hydrogels the ideal candidate for implementing various actuation abilities in multiple applications, mainly in the biomedical field.
Additionally, these hydrogel-based polymer matrices are mechanically robust and compliant (Haraguchi and Takehisa, 2002; Yuk et al., 2017) and can interact with biological tissue/organs environment in a more safe and human-friendly way (Stanton et al., 2015). The hydrogels possess admirable diffusive properties due to their high water content and can behave similar to the extracellular morphology, thus can be widely used in various biomedicine areas such as regenerative medicine (Slaughter et al., 2009; Bettinger et al., 2014), drug delivery (Langer, 1990; Uhrich et al., 1999; Qiu and Park, 2001; Hamidi et al., 2008), artificial organs (Burczak et al., 1996; Hoffman, 2012), tissue engineering (Lee and Mooney, 2001; Drury and Mooney, 2003; Khademhosseini and Langer, 2016; Leijten et al., 2017), and related fields (Webber et al., 2016; Banerjee et al., 2018b). The hydrogel matrix can be easily customized in shape, form, and morphology via various fabrication and molding techniques (Carrico et al., 2017). This allows the hydrogel constituents to design sensors and actuators for different biomedical applications by mimicking the biological tissues/organs (Banerjee et al., 2018b). In general, gels and hydrogels have fascinated greater research attention in soft biomedical robotics.
Here, we limit our fundamental center to the hydrogel-based robust soft actuators/sensors for the most part from a mechanical characteristic perspective and its various applied applications, mainly in the area of the biomedical field. Material researchers are facing significant challenges in developing and fabricating soft, flexible, elastic yet robust and resilient soft hydrogel structures and characterizing new biomimetic soft polymer materials from a biocompatible, degradable, and environmentally sustainable perspective (Ilievski et al., 2011; Rossiter et al., 2016).
A polymer such as hydrogels can play a significant role in biomimetic research because of their stimulus-responsive ability, biodegradability, and biocompatibility. In addition to the stimulus-responsive behavior, their mechanical and functional properties can be enhanced by developing composites with the additive materials having toughness, strength, and stretchability. For example, chitosan-based hydrogels have already been used to mimic tissues and cells and their mechanical properties, such as wear and tear, which can be enhanced through structuring (Rafat et al., 2008; Fratzl and Barth, 2009; Tan et al., 2009).
Hydrogels with a significant amount of water content can transform their shapes, size, and morphology via altering the water content in the hydrogel polymer matrix network through their swelling/deswelling mechanism. The hydrogel polymer matrix can undergo volume–phase shape and morphology transformation via swelling or shrinking in response to physicochemical internal or external ambient environment stimuli such as temperature, light, electricity, humidity, pH value, and magnetic field (Chen and Dai, 2015).
In most applications, whenever there is a need to utilize a soft, flexible, manufactured polymer for creating deformable and shape-adaptable soft robotic structures, silicone elastomers are, for the most part, used broadly due to their primary and fast fabrication strategy (Shepherd et al., 2011; Tolley et al., 2014). In general, silicone elastomers with robust elastic properties come in natural/synthetic polymers. For instance, polysiloxane is a polymer functionalized with the chain of silicon and oxygen atoms (Boonstra et al., 1975; de Buyl, 2001; Colas and Curtis, 2004). Even though silicon-based elastomers have been utilized broadly in different applications, within the zone of soft robots and biomedical field (Goyal et al., 2019), in any case, a few issues such as biodegradability and biocompatibility remain challenging (Naji and Harmand, 1991; Kue et al., 1999). In addition to that, due to higher viscosity, a failure to scale down to the micro-/nano-administration, toxicity, and hydrophobicity threats (Kotzar et al., 2002) make them undesirable in many applications, especially in biomedical applications. On the other hand, due to the inherent biocompatibility, biodegradability, and customizability of hydrogels, researchers have been progressing toward their later use as biomaterials with different natural abilities (Otake et al., 2002; Discher, 2005). Therefore, it is beneficial to take advantage of the distinctive properties of elastomers and hydrogels to develop into a composite biomaterial through various methods such as cross-linking and free-radical copolymerization (Tobita and Hamielec, 1992; Hong et al., 2007). Table 1 highlights the main dissimilarities that have attracted hydrogels for being utilized, as restricted to their potential partners, such as silicone elastomer, especially in biological applications.
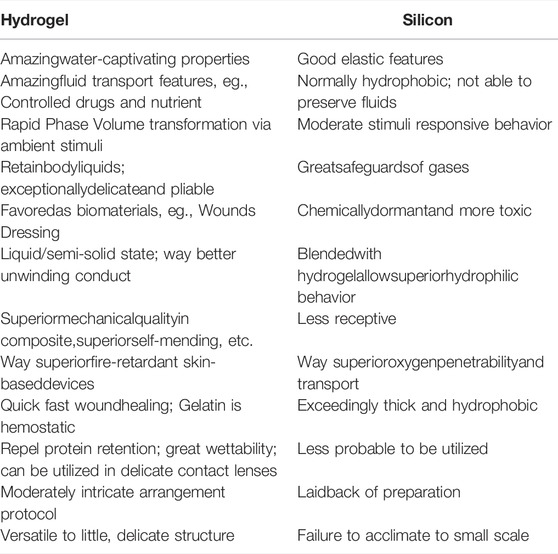
TABLE 1. Some key differences in properties between hydrogel and silicone elastomer (2018a). Copyright 2018, Biomimetic MDPI.
Bio-Inspired Shape-Morphing Actuators
Over millions of years, all living species have undergone different stages of advancement and adjusted to different natural reactions and components. An extraordinary sum of innovations has come about by closely watching nature’s manifestations and adjustments and by mirroring the answers for its everyday challenges. For example, a widespread extend of functional and morphological components in plants through their swelling/deswelling at the cellular level triggers the plants to incline toward daylight (Burgert and Fratzl, 2009; Fratzl and Barth, 2009).
Hydrogel-based sensors and actuators can be more fulfilled by using different stimulus-responsive hydrogels, which can perform various kinds of movement and shape and size deformation in response to ambient environmental stimuli. Excellent biodegradability and biocompatibility are some of the significant advantages of hydrogels, empowering them to perform way better than their partners (e.g., silicone-based elastomer) in the implementation of actuators for application in the bio-related area. So by gaining advantage from these valuable features, hydrogel-based actuators and sensors can interact with the human body’s internal environment in a more safe and human-friendly approach, and thus perform their task without any adverse impressions on human physiological activities. In addition to that, hydrogel materials claim to absorb polymer organize structures compared to those of local extracellular matrix (ECM) networks.
These properties further boost their applicability and feasibility in the bio-related areas, such as tissue engineering applications, even down to the cellular level (DeForest and Anseth, 2012; Caliari and Burdick, 2016). Furthermore, hydrogels’ biophysical, chemical, and mechanical properties can be effectively tuned by embracing distinctive manufacturing strategies with different composition extents. It provides the hydrogels with user-defined properties, which could meet practical demands in various areas such as soft robotic actuation tasks and could further expand its applicability in the biomedical application as hydrogel-based bio-actuators (Zhalmuratova and Chung, 2020).
Similarly, biomimetic actuators were motivated and created from the different highlights of nature, such as functions, movements, and morphology transformation of plants. Although seemingly stationary, plants can perform subtle approach movements such as nastic tropisms, such as a sensitive plant, Mimosa pudica, that can suddenly fold its leaves inward when touched. So by taking inspiration from Mimosa, researchers have, as of now, created a temperature-responsive reversible input-controlled bilayer hydrogel structure (Liu and Calvert, 2000). In other studies, by imitating the reversible development highlight of plants, for instance, Venus flytraps (Dionaea muscipula) and pine vines using the laminate movement and deformation of inhomogeneous hydrogels (Fusco et al., 2014). Additionally, the same component guideline can be amplified to fish and insects with unique features. Propelled by nature, the morpho butterfly includes biohybrid auxiliary color gels that can be valuable in planning explicit living materials (Hong et al., 2011; Fu et al., 2018). Some researchers focused on optically camouflage-based hydrogel actuators (hydrogel robotic fish) to realize high optical transparency in an aqueous environment by mimicking the natural camouflage fish such as leptocephalus (Löwenberg et al., 2017).
During the long-term investigation for appropriate materials aimed at soft robotic sensors and actuators and bio-related applications, the focus has been progressively shifted toward stimulus-responsive hydrogels due to their potential stimulus-responsive properties, superior biocompatibility, high water content, and tunable structural and physiochemical properties (Huang et al., 2017; Wei et al., 2017). In Figure 2, we appear to have a connection between biomimetic standards and nature-motivated actuators for delicate mechanical applications.
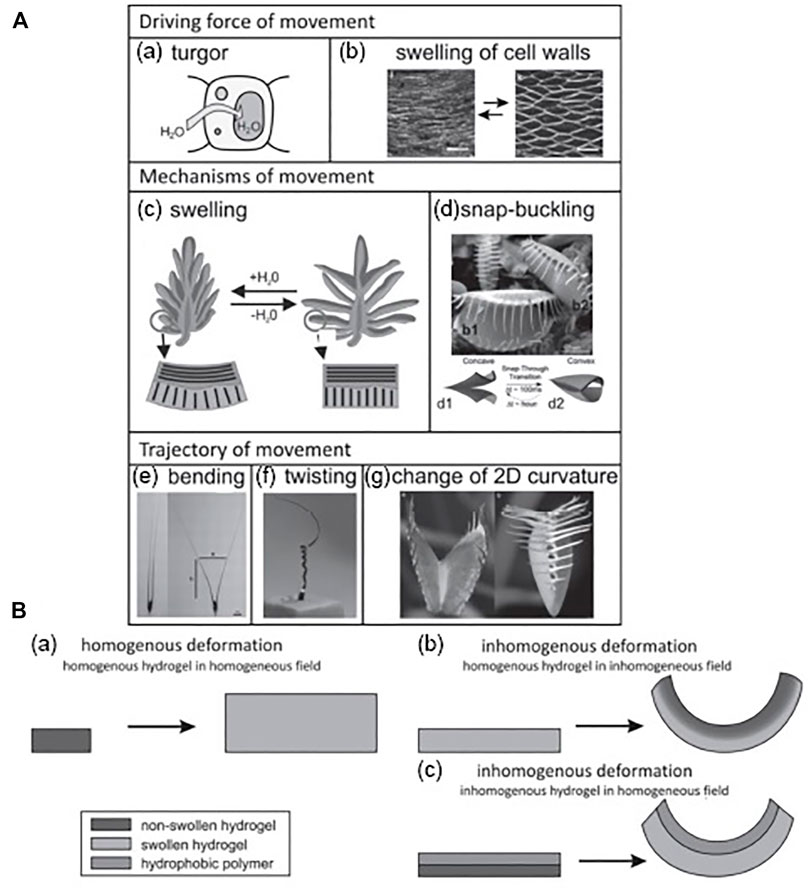
FIGURE 2. (A) Examples of two-way movement in plants with respect to the driving force: (a–g) turgor cell wall mechanism via swelling/shirking with snap-buckling, bending, twisting, and alteration of two-dimensional (2D) curvature. (B) Various setups of swelling/shrinking of hydrogels: (a–c) homogeneous and inhomogeneous distortion of homogeneous hydrogel and inhomogeneous distortion of inhomogeneous hydrogel (Mao et al., 2005; Bassil et al., 2008; Ismail et al., 2011; Takashima et al., 2012). Copyright 2013, John Wiley & Sons Inc.
The basic working principle of the hydrogel-based soft actuators is their ability to perform volumetric shape changes via swell/shrink kinetic mechanism, reliant on the water content in it, which permits it to perform different kinds of actuation movement and morphologies. The water content in the hydrogels is responsive to various external or internal ambient stimuli, such as temperature, light, electricity, magnetic field, pH, humidity, ionic strength, and molecular interactions. Therefore, there are applications in various areas for hydrogel-based shape-morphing actuators, although they may also have the impediment of performing only in watery media (Morales et al., 2014).
Recently, the design, development, and implementation of hydrogel-based devices, particularly in bio-related applications, are under development, and much research is anticipated for its wide practical use in biomedical applications. For instance, hydrogel-based actuators as artificial muscles and tissues (Ashley, 2003) are not yet developed fully due to the need for a solid and adaptable material/gel, which could be appealing as biological tissue-based actuators carrying different sorts of loads (Mao et al., 2005; Bassil et al., 2008; Ismail et al., 2011; Takashima et al., 2012). In Figure 3, we illustrate different delicate activation components based on the strain energy density function, which is a temperature-dependent strain response (Qiu et al., 2019). The design focus was to bio-mimic in a very close manner by accomplishing the hydrogel space as near to normal muscles as conceivable. In the subsequent sections, we attempt to cover various kinds of hydrogel polymer-based soft actuators on the premise of the nature of stimulus-responsive conduct and their various applications, mainly in the area of biomedical and soft robotics. More specifically, we cover the foremost broadly considered and developed hydrogel-based stimulus-responsive soft biomimetic actuators such as thermal, electric, magnetic, and pH-based actuators, and the moderately less inhabited light-based hydrogel actuators, among others, are also covered in a very concise way.
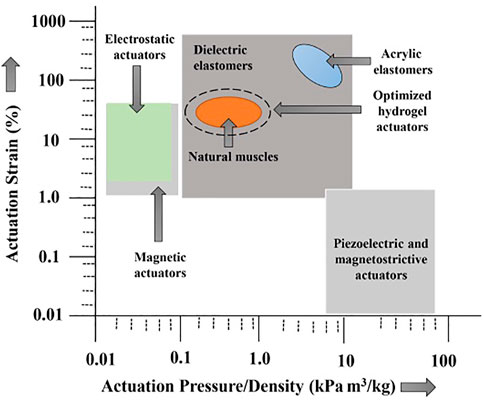
FIGURE 3. Various regimes of natural muscle and soft actuators; hydrogel-based actuators can closely mimic the profile of artificial muscles and tissue (Banerjee et al., 2018a). Copyright 2018, Biomimetic MDPI.
Various Stimulus-Responsive Hydrogels
The hydrogel-based structures have sufficient water content, provide stimulus-responsive features, and are biocompatible with human organs/tissues that allow predicting its conduct comparable to extracellular frameworks in its design. Hydrogels’ physiochemical and physio-mechanical properties can be responsive to various ambient environmental stimuli such as temperature, electricity, magnetic fields, light, and pH (Calvert, 2009).
Selecting the hydrogel materials with fitting stimulus-responsive characteristics is critical for designing bio-actuators and biosensors as the hydrogel-based actuator/sensor performance depends on its categorical response to its physical and chemical signals. The responsive behaviors can discover a seamless match with requests from various practical applications (Tokarev and Minko, 2009; Ionov, 2010). The physicochemical properties of innovative materials such as stimulus-responsive hydrogels can be easily tuned and managed via various methods and are instinctively adapted to varying ambient environments. Therefore, hydrogel-based innovative materials may give new and modern design openings in developing smart soft actuation systems such as soft actuators and soft robots. As illustrated in Figure 4, the actuation behavior of these smart hydrogels can be easily controlled by various external or internal ambient environment stimuli such as heat (temperature) (Yu et al., 2013; Fan et al., 2019), electrical current/voltage (Xue et al., 2016), magnetic fields (McCracken et al., 2019; Tang et al., 2019), pH value (Ma et al., 2014; Zhang et al., 2018), light (Iwaso et al., 2016; Cheng et al., 2019), and salinity (Liu et al., 2016; Xiao et al., 2017). Table 2 highlights the different kinds of hydrogel material and their main applications based on stimulus-responsive behavior such as thermal-responsive, electro-responsive, magnetic responsive, and photoresponsive.
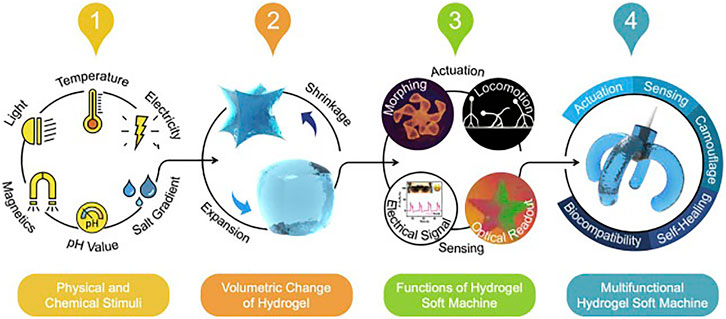
FIGURE 4. Schematic illustration of the hydrogel-based stimulus-responsive soft session and actuation features such as shape morphing (Fu et al., 2018), locomotion (DeForest and Anseth, 2012), sensing with electrical signal output (Morales et al., 2014), and sensing with optical readout (Ashley, 2003). Copyright 2020, Elsevier.

TABLE 2. Hydrogels based on kind of stimulus-responsive behavior and key features with their main applications, respectively (Calvert, 2009). Copyright 2018, Biomimetic MDPI.
Thermal-Responsive Hydrogel
Thermal-responsive hydrogels have the unique ability to undergo volumetric phase change such as shifting in their shape morphology when heated above or below a specific threshold temperature, called the lower critical solution temperature (LCST). Therefore, this critical solution temperature of hydrogels is generally considered the key to assessing the volumetric phase transition. The mechanism for getting the stimulus responsiveness in such gels is the boost-initiated change within the miscibility of arranging chain fragment and dissolvable. A standard illustration of this is often the lower critical solution temperature (LCST) of the thermal-responsive hydrogel such as N-isopropyl acrylamide (pNIPAM). For occurrence, below the LCST, pNIPAM segments are soluble in a watery medium. In contrast, in an environmental temperature higher than its LCST, it shrinks and precipitates in the aqueous solution due to the weakened hydrogen interchange bonding. This shape/volume altering property is precious for developing hydrogel-based actuators in soft robotics and biomedical applications such as artificial muscles and drug delivery systems. For instance, these thermal stimulus-responsive hydrogels, when composite with other kinds of biopolymers, can act as good biological actuation systems (Hsu et al., 2016; Nikouei et al., 2016; Suntornnond et al., 2016). Moreover, pNIPAM hydrogel, when combined with carbon nanoparticles (CNPs), can improve the response time of the thermal actuation manifold compared to unadulterated pNIPAM hydrogels driving to tunable thermally responsive actuator frameworks (Zhang et al., 2011).
In addition to pNIPAM, different kinds of other thermal-responsive hydrogels have been examined, such as poly(vinyl methyl ether) (PVME). These water-swollen thermal-responsive hydrogels have been widely developed. These hydrogels have been used to create various kinds of thermally stimulated devices such as counterfeit muscle, an automatic separation framework, and many other thermal and photosensitive devices (Kishi et al., 1993). Furthermore, hydrogels developed by photopolymerization for cross-linking can find their biomedical applications such as cell encapsulation materials and drug delivery systems (Nguyen and West, 2002; Oh et al., 2008; Chang et al., 2009). There has been growing attention toward developing thermal responsive tunable hydrogels via their molecular design in recent times. It can deliver new possibilities to create different kinds of soft machine systems to realize the requirement for other applications such as actuators (Lin et al., 2019), sensors (Hou et al., 2020), and drug delivery systems (Amoli-Diva et al., 2017).
Electro-Responsive Hydrogel
Electro-responsive hydrogels can convert applied electrical energy and transfer it to a few shape changes of mechanical vitality through their polymer arrangement dynamic framework deformation. One of the prime benefits of using these hydrogels is their compatibility with batteries and other electronics, making it simpler to supply a control source. Electro-responsive hydrogels come in the category of electro-active polymers (EAPs) that can swell or deswell in reaction to the applying electrical potential (e.g., voltage and current) (Ali et al., 2019). For instance, when an electric field/voltage is applied, it results in a non-uniform ion distribution across a layer of electro-responsive hydrogel, which causes the change in the curvature of the hydrogel layers due to the generation of the osmotic pressure difference between the layers. Generally, the EAPs such as electro-responsiveness of hydrogels can be precisely designed with different ionizable functional groups. The different kinds of developed EAPs are poly(acrylic acid) (PAA) (Moschou et al., 2004; Kim et al., 2005), poly(2-acrylamide-2-methyl propane sulfonic acid) (PAMPS) (Osada, 1991; Yang et al., 2017), poly(4-hydroxybutyl acrylate) [poly (4-HBA)] (Kwon et al., 2008, 2010), etc. The electro-responsive hydrogels, too, have the same basic working principle similar to that of thermal-responsive hydrogels. This sort of hydrogel actuator is often made of composite materials. It may be a grouping of hydrogel polymers in conjunction with other polymers such as conducting polymers (CPs) that can be prepared from polyelectrolytes. For instance, Jin et al. developed a 3-dimensional chemically cross-linked electron-conducting hydrogel electrolyte for quantum dot-sensitized solar cells (QDSSC) (Yang et al., 2018). So, by applying an electric signal, these hydrogel actuators swell/shrink reliant upon the material and the experimental setup.
Furthermore, to advance the elasticity of the material and its conductivity, there are various kinds of double-network (DN) hydrogels (Gong et al., 2003) and conductive gels such as oriented graphene hydrogels (OGHs) (Gao et al., 2015) that are more elastic and deformable and which can be developed into various thin films. It can be utilized in different applications, mainly in bio-related applications for which rigidity is not much concern (Shiga et al., 1993).
Magnetic-Responsive Hydrogel
The magnetic field is a promising stimulus trigger for various applications explicit in the biomedical field due to its distant response behavior with a rapid response time. It may be a biocompatible energy source even at a high magnetic field strength. The stimulus-responsive behavior of these magnetic hydrogels is primarily realized by the combination of ferromagnetic or paramagnetic-added substances external fillers into the polymeric matrix framework, which contribute to empowering rapid and extensive actuating behaviors by applying some external magnetic fields. These magnetic additives can be categorized into three main types: metals and alloys such as iron (Fe) (Shankar et al., 2017), ferromagnetic, and iron platinum nanoparticle (FePt) (Zhang and Wang, 2012); different oxides such as ferrous ferric oxide (Fe3O4) (Zrínyi et al., 1996) and metal oxides such as ferric oxide (Fe2O3) (Roeder et al., 2012); and their functionalized derivatives. The motion of these magnetic fillers inside the entangled polymer chains could initiate the shape or volumetric deformation of the hydrogel structure via an external magnetic field (Hoare et al., 2009). Using magnetic-based hydrogel actuators, a relatively large actuation force is released upon generating the magnetic field. Another benefit of using magnetically responsive hydrogels is that they show quick response behavior than other hydrogel-based actuators.
Furthermore, they can be utilized in different kinds of mediums. The attraction contains a high degree of penetrability in another medium, making it conspicuous actuators used for biomedical applications within the near future. One of the prominent features of magnetic induction heating is the fast response time, which widely increases the applications of such magnetic responsive hydrogels in various applications such as soft actuators (Park et al., 2016; Wehner et al., 2016), biomedical devices (Fusco et al., 2014), and microfluidic systems (Satarkar et al., 2009).
Photoresponsive Hydrogel
Apart from the aforementioned stimulus-responsive behavior of hydrogels with a phase–volume transformation that reacts to another kind of incitement such as light have great potential for various purposes (Sidorenko et al., 2007; Nakahata et al., 2013). Light is a potential remote stimulus for the photoresponsive hydrogels to induce the volume, phase, and shape change transformations upon different light irradiations. For example, the realization of inaccessible and non-invasive remote activation with a high resolution by means of photostimulus has plenteous potential for various applications, primarily in the biomedical field (Wang et al., 2013). For instance, the dye-sensitized solar cells (DSSCs) exhibiting photo-responsive electrolytes have been developed by means of hybrid hydrogel, which is performed for power conversion efficiency up to 4.5% (Das et al., 2016).
The photoresponsive hydrogels can quickly respond to the illumination of a heat source such as near-infrared light, with sizeable volumetric deformation rapidly. Furthermore, hydrogels responsive to environmental humidity changes may find their usefulness in real-time ecological monitoring (Yin et al., 2013).
The development of photoresponsive hydrogels can find their applications in various areas such as soft sensors and actuators with distant control (Nakahata et al., 2013), self-healing hydrogels, soft molecular machines (Wang et al., 2013), and remote-controlled drug release (Park et al., 2016).
Mechanically Robust, Tougher, and Stretchable Hydrogels
Numerous biological delicate soft tissues, such as cartilage skeletal muscles in the human body, can be seen as a hydrogel framework comprising cells and fortifying elements such as reinforcing fibers. For instance, biological articular cartilage could be a composite structure with collagen fibrils fortifying a proteoglycan gel lattice (Peppas et al., 2006; Agrawal et al., 2013). The expansion to break a cartilage, which is around 100%, and the vast expansion to break permit the cartilage to stand up to tearing effect, even though the average quality is not exceptionally high (Tan et al., 2009). For the most part, the articular cartilage encompasses the quality of kPa, but this will go up to MPa in locales with large fiber substances.
The tendon and ligament, which have the highest collagen content among all the collagenous tissues, are another center of focus. The tendon associates a bone to the muscle, whereas the reason for ligaments is to interface bones together (Pradas and Calleja, 1990).
Moreover, ligaments and tendons encourage movement and keep joint soundness as they are subjected to various kinds of loadings along their length. Hence, it is not astounding that their fibrous variety of structures at diverse length scales are all adjusted in one course (Holzapfel and Gasser, 2001).
Most synthetic hydrogels have a shallow magnitude of toughness when completely swollen (Haraguchi et al., 2003). This could be credited to the thermodynamic driving constrain vital to solubilize the polymer networks, being restricted by the expansion of the cross-linked networks so that the arranged network is extended, and breaks can endanger the gel with small retention of vitality (Hong et al., 2007). The current research has depicted several courses to toughen the gels by presenting components that can retain vitality at even the break tip and closely resemble toughening instruments in ceramics and composites.
Recently, fiber-reinforced hydrogels can be moderately hardened but not as deformable as cartilages (Hassan and Peppas, 2000). Whereas profoundly stretchable and strong hydrogels have also been synthesized, their moduli and toughness are significantly second rate to those of cartilages. In expansion, the standard instrument to develop the tough and stretchable hydrogels is to actualize the manner for dissipating the mechanical energy and keeping up its high elasticity. For instance, robust and tough hydrogels that depend on long-chain linkages to preserve elevated flexibility and mechanical vitality by reversible cross-linking to dispel mechanical energy will be more powerless and fragile if the reversible cross-linkers in them are disposed of (Li et al., 2014).
The anisotropy also plays a critical role in biological organisms’ shape morphing and movements (Liao et al., 2013). For example, plants have advanced features that can change tissues’ anisotropic swelling and contract into different kinds of activities, such as, counting, twisting, bending, coiling, and turning (Pradas and Calleja, 1990; Shikinami et al., 2004). Additionally, natural tissues have neighborhood varieties in properties, particularly at tissue and bone interfacing, for instance, tendon-to-bone, ligament-to-bone, and cartilage-to-bone (Seidi et al., 2011). A characteristic tissue/cartilage shows a complex anisotropic behavior with viscoelastic and non-linear mechanical properties owing to complicated hierarchal architecture, making it alluring to imitate the structure (Yoda, 1998). The central feature of the mechanical property of soft tissues/organs could be the so-called “J-shaped” strain-stiffening mechanism, as illustrated in Figure 5A (Ma et al., 2017). This stress–strain mechanism is the instrument for nature’s self-defense against a different range of stresses, which may be applied onto delicate tissues. This mechanism is ascribed to pleated collagen strands inside the soft tissue that continuously coil and uncoil and straighten in the long run, driving to strength at higher strains and a high modulus, as shown in Figure 5B. So, the synthetic hydrogel, with its J-shaped feature could have potential applications in various areas, such as soft robotics, tissue engineering, and biomedical devices.
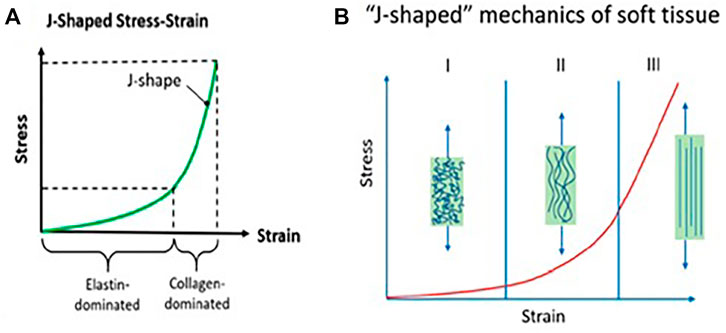
FIGURE 5. Physio-mechanical properties of biological tissues vital to mimic in composites structure: (A) J-shaped mechanical behavior, (B) mechanism to realize anisotropy via J-shaped stress−strain behavior (Ma et al., 2017) Copyright © 2020, American Chemical Society.
Subsequently, the development of tough, stiff, and flexible hydrogels in the current study depends on the composition of the hardened but stretchy fiber mesh and rigid and brittle hydrogel matrix (Figure 6A). In addition, reinforcing the fibers with the composite undoubtedly depends on the hierarchal architecture of numerous components over various length scales (Lin et al., 2014). One such type of hierarchal architecture featuring helicoidal arrangements can be seen in different species, incorporating bone, wood, fingernail skin, and the skeleton of glass wipes (Wagermaier et al., 2006). A comparative design is also seen within the wood cell, which is the central load-bearing component of trees. There, cellulose microfibrils shape a helicoidal design around the tube-like structure of the wood cell (Lichtenegger et al., 1999), as illustrated in Figure 6B (Ji and Kim, 2021).
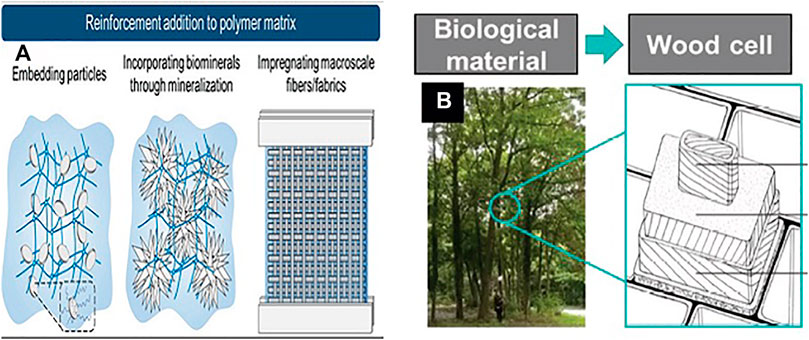
FIGURE 6. (A) Schematic outline of later methods pointed to develop stiff and stretchable tough hydrogels (Ji and Kim, 2021) Copyright 2021, John Wiley & Sons Inc. (B) Impression of the wood-inspired design model and mechanical performance of the fiber-reinforced composites comprising a multilayer cylindrical structure (Lichtenegger et al., 1999). Copyright 2018, John Wiley & Sons Inc.
Hydrogel-Based 3D Printing for Soft Robotics and Biomedical Application
Three-dimensional (3D) printing or additive manufacturing (AM) is a relatively new method that enables the fabrication of metallic and non-metallic material components directly from the computer-aided design (CAD) file to create a 3D object. In the additive manufacturing technique, the 3D objects, which can be of nearly any geometry or shape, are usually created utilizing the digital information data model from a 3D digital file or an additive manufacturing file (AMF).
The hydrogels are widely used to fabricate 3D-printed hydrogel-based actuators/sensors for various applications such as soft robotics and biomedical applications. The studies on 3D hydrogel printability are substantial in biomedical and bio-engineering. Consequently, further progress had been made in this area to test the properties such as reliability and printability of hydrogels amid the improvement of gel/ink-based printing (Malda et al., 2013; He et al., 2016). Hydrogel-based 3D printing can be categorized into three sorts: nozzle-, laser-, and inkjet-based printing. In most of the extrusion based 3D printing techniques, a store is utilized from which the ink of hydrogel is extruded through any of the over implies. In one research study, the 3D printability of hydrogels for human tissues such as cartilage and bone tissues is being carried out and its exceptionally tall request within biomedical region for the substitution or repair of harmed cells and tissues started by wounds was also examined (Hutmacher, 2000). The composite based on hydrogels (such as polyethylene glycol (PEG), gelatin, and hyaluronic acid) is superior for creating counterfeit bones and tissues than the natural hydrogels owing to their upgraded physical and mechanical properties. Still, at the same time, they make selection and printing for various requirements more challenging (De Mori et al., 2018).
As persistent enhancements are being made to develop highly soft and elastic hydrogels for soft robotics and biomedical applications, the development of 3D printing for hydrogel-based actuators and sensors has rapidly grown in interest shortly. For example, the development of customized bio-actuators for real-life applications with the 3D printed method was implemented for various external stimuli and controls (Zolfagharian et al., 2016). In another study, researchers had surveyed the humidity-compelled hydrogels actuation, developed by a 3D printing method via two-photon polymerization, in which swelling/shrinking driven by humidity was controlled by altering the cross-linking thickness voxels within the hydrogel microstructure (Lv et al., 2018). The development of a stimulus-responsive hydrogel such as temperature-responsive was 3D micro-printed by miniaturized projection stereolithography, a strategy in which fast photopolymerization of the hydrogel layers can be made by a streak of ultraviolet light at the microscale determination (Han et al., 2018). Furthermore, another study was conducted to perform the design and development of a 3D printing-based bio-fabrication system based on a skeletal muscle-powered bio-machine, which can be stimulated via environment ambient stimulus (Cvetkovic et al., 2014) as the 3D printing of hydrogel is becoming commonly utilized to develop different sensing devices such as physical sensors, chemical sensors, and biosensors. These can be 3D printed by printing the sensing/actuator components or printing the stages and printing molds (Ni et al., 2017). For example, researchers at MIT had developed thermo-responsive skin-based sensors by 3D printing of hydrogel (Cvetkovic et al., 2014), and 3D printed soft, nearly obscure, hydrogel-based soft robots can find various applications, such as in underwater surveillance (Yuk et al., 2017). Figure 7 illustrates multiple 3D printed models with different morphologies and textures with highly robust and compliant hydrogel composite structures.
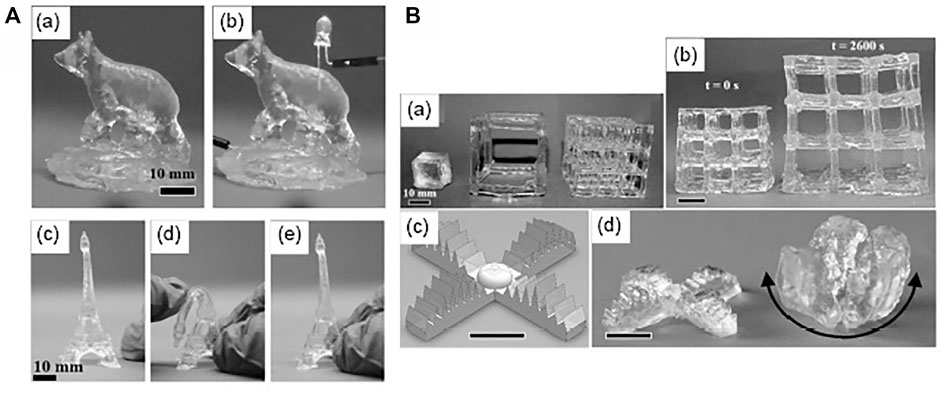
FIGURE 7. 3D printed hydrogel-based soft, conductive, elastic, and translucent composite structure. (A) (a,b) 3D printed conductive and translucent ionic composite hydrogel (c–e) Illustration of a 3D printed robust Eiffel tower with repeatable distortion. (B) (a-b) 3D printed ionic composite hydrogel structure of different aspect ratios with various shrinking/swelling phenomena with before and after water absorption. (c, d) Design of 3D printed multi-armed hydrogel-based gripper with swelling/shrinking in blue-dyed water (Cvetkovic et al., 2014). Copyright 2017, John Wiley & Sons Inc.
Conclusion and Future Challenges
Stimulus-responsive hydrogels have been developed to narrow the gap among delicate soft polymer-based actuators and sensors and their innovative applications, mainly in bio-related areas. These are an advanced bunch of materials for fabricating actuators and sensors, which can respond to various ambient environment stimuli such as temperature, electricity, light, pH, and magnetic field, with their phase and shape morphology transformation (Shi et al., 2019) and can find their application mainly in soft robotics and biomedical field.
However, there are still a few challenges that ought to be considered before the application of stimulus-responsive hydrogel-based actuators. To develop hydrogel-based actuators, one of the intrinsic challenges lies in the reliance on a wet environment as the activation phenomena of the stimulus-responsive hydrogel which is mainly based on the exchange of water molecules between the hydrogel polymer matrix framework and its immediate environment. In this review paper, the various critical mechanism to develop robost, flexible, and stretchable stimuli-responsive hydrogels with its various applications is being briefly discussed. In addition to this, the 3D printing of hydrogels and the related challenges confronting its application are also discussed.
Furthermore, irrespective of the development of hydrogel-based actuators that could sense different stimuli within a single device, they can expel particular stimulus-triggered hydrogel actuation impediments, for example, pH-/electro-, pH-/thermal-, thermal/magnetic-, and thermal/light-responsive hydrogel actuators. The consistency, dependability, and specificity of these multi-mode-triggered actuators have be examined, especially when considering the prospective cross-impact among unique stimulus-responsive phenomena. Despite these challenges, the development of stimulus-responsive hydrogels significantly rouses the advancement of soft actuators and sensors and their different applications, particularly in the biomedical field. The response time (currently several minutes) of the stimulus-responsive hydrogel is suggested to be achieved till the millisecond level, exclusively in the applications where a rapid response is essential such as artificial neurons or muscles. The growing potential of these stimulus-responsive hydrogels should be toward intending potential solutions to the prevailing challenges. For example, fast and quick reacting hydrogel-based actuators are anticipated to be fabricated and augment the responsive behavior under different ambient stimuli. A great way to address this objective could be toward enhancing the swelling/shrinking kinetic performance by modifying the cross-linking polymer structure of hydrogel molecules and realizing hydrogels with smaller sizes and thinner profiles. Additionally, the hydrogel molecular structure to create more mechanically strong and robust hybrid composites by combining other polymers’ properties is another advantage for their utility in the various applications (Zhao, 2017). In addition to this, other confinement of hydrogels, material synthesis is a slow-to-moderate multi-step polymerization technique, which is troublesome to control and modify the properties of hydrogels. However, it has been conquered as of late with the presentation of superior hydrogel synthesis strategies: one such is the one-pot synthesis method developed by Chen et al. (2013). One fundamental limitation to developing hydrogel-based devices is the inherent gel inhomogeneity present in hydrogels which hinders the strength and optical clarity. Apart from this, other limitations to be overcome in developing the hydrogel-based sensors or actuators are the non-adherence, high cost, and poor mechanical properties due to their high water content. However, most of the possibilities depicted here can possibly be connected within the range of soft robotics and biomedical application.
In conclusion, this review article provides a brief glimpse of hydrogels and their potential applications and challenges from a mechanical soundness perspective. However, to address the current biomedical challenges, many collaborative interdisciplinary efforts are still required for this technology to mature.
Author Contributions
RG has performed a rigorous review on the topic . As the main author of this review, my main contribution is to reading and understanding all the literature review articles related to the topic and consolidating them to prepare this brief review article. SM has given suggestions, support and guidance to publish this article in the prestigious journal.
Conflict of Interest
The authors declare that the research was conducted in the absence of any commercial or financial relationships that could be construed as a potential conflict of interest.
Publisher’s Note
All claims expressed in this article are solely those of the authors and do not necessarily represent those of their affiliated organizations, or those of the publisher, the editors, and the reviewers. Any product that may be evaluated in this article, or claim that may be made by its manufacturer, is not guaranteed or endorsed by the publisher.
References
Agrawal, A., Rahbar, N., and Calvert, P. D. (2013). Strong Fiber-Reinforced Hydrogel. Acta Biomater. 9, 5313–5318. doi:10.1016/j.actbio.2012.10.011
Ali, I., Xudong, L., Xiaoqing, C., Zhiwei, J., Pervaiz, M., Weimin, Y., et al. (2019). A Review of Electro-Stimulated Gels and Their Applications: Present State and Future Perspectives. Mater. Sci. Eng. C 103, 109852. doi:10.1016/j.msec.2019.109852
Amoli-Diva, M., Sadighi-Bonabi, R., and Pourghazi, K. (2017). Switchable On/off Drug Release from Gold Nanoparticles-Grafted Dual Light- and Temperature-Responsive Hydrogel for Controlled Drug Delivery. Mater. Sci. Eng. C 76, 242–248. doi:10.1016/j.msec.2017.03.038
Armon, S., Efrati, E., Kupferman, R., and Sharon, E. (2011). Geometry and Mechanics in the Opening of Chiral Seed Pods. Science 333, 1726–1730. doi:10.1126/science.1203874
Banerjee, H., Suhail, M., and Ren, H. (2018a). Hydrogel Actuators and Sensors for Biomedical Soft Robots: Brief Overview with Impending Challenges. Biomimetics 3, 15–41. doi:10.3390/biomimetics3030015
Banerjee, H., Tse, Z. T. H., and Ren, H. (2018b). Soft Robotics with Compliance and Adaptation for Biomedical Applications and Forthcoming Challenges. Int. J. Robot. Autom. 33. doi:10.2316/Journal.206.2018.1.206-4981
Bassil, M., Davenas, J., and EL Tahchi, M. (2008). Electrochemical Properties and Actuation Mechanisms of Polyacrylamide Hydrogel for Artificial Muscle Application. Sensors Actuators B: Chem. 134, 496–501. doi:10.1016/j.snb.2008.05.025
Bettinger, C., Borenstein, J., and Langer, R. (2014). “Microfabrication Techniques in Scaffold Development,” in Nanotechnology and Regenerative Engineering (Boca Raton: CRC Press), 103–142. doi:10.1201/b17444-6
Boonstra, B. B., Cochrane, H., and Dannenberg, E. M. (1975). Reinforcement of Silicone Rubber by Particulate Silica. Rubber Chem. Technol. 48, 558–576. doi:10.5254/1.3539660
Burczak, K., Gamian, E., and Kochman, A. (1996). Long-term In Vivo Performance and Biocompatibility of Poly(vinyl Alcohol) Hydrogel Macrocapsules for Hybrid-type Artificial Pancreas. Biomaterials 17, 2351–2356. doi:10.1016/S0142-9612(96)00076-2
Burgert, I., and Fratzl, P. (2009). Actuation Systems in Plants as Prototypes for Bioinspired Devices. Phil. Trans. R. Soc. A. 367, 1541–1557. doi:10.1098/rsta.2009.0003
Caliari, S. R., and Burdick, J. A. (2016). A Practical Guide to Hydrogels for Cell Culture. Nat. Methods 13, 405–414. doi:10.1038/nmeth.3839
Calvert, P. (2009). Hydrogels for Soft Machines. Adv. Mater. 21, 743–756. doi:10.1002/adma.200800534
Cangialosi, A., Yoon, C., Liu, J., Huang, Q., Guo, J., Nguyen, T. D., et al. (2017). DNA Sequence-Directed Shape Change of Photopatterned Hydrogels via High-Degree Swelling. Science 357, 1126–1130. doi:10.1126/science.aan3925
Capadona, J. R., Shanmuganathan, K., Tyler, D. J., Rowan, S. J., and Weder, C. (2008). Stimuli-Responsive Polymer Nanocomposites Inspired by the Sea Cucumber Dermis. Science 319, 1370–1374. doi:10.1126/science.1153307
Carrico, J. D., Kim, K. J., and Leang, K. K. (2017). “3D-printed Ionic Polymer-Metal Composite Soft Crawling Robot,” in 2017 IEEE International Conference on Robotics and Automation (ICRA) (IEEE), 4313–4320. doi:10.1109/ICRA.2017.7989496
Chang, Y., Chen, W.-Y., Yandi, W., Shih, Y.-J., Chu, W.-L., Liu, Y.-L., et al. (2009). Dual-Thermoresponsive Phase Behavior of Blood Compatible Zwitterionic Copolymers Containing Nonionic Poly(N-Isopropyl Acrylamide). Biomacromolecules 10, 2092–2100. doi:10.1021/bm900208u
Chen, Q., Zhu, L., Zhao, C., Wang, Q., and Zheng, J. (2013). A Robust, One-Pot Synthesis of Highly Mechanical and Recoverable Double Network Hydrogels Using Thermoreversible Sol-Gel Polysaccharide. Adv. Mater. 25, 4171–4176. doi:10.1002/adma.201300817
Chen, X., and Dai, H.-H. (2015). Swelling and Instability of a Gel Annulus. Acta Mech. Sin. 31, 627–636. doi:10.1007/s10409-015-0496-4
Cheng, Y., Chan, K. H., Wang, X.-Q., Ding, T., Li, T., Lu, X., et al. (2019). Direct-Ink-Write 3D Printing of Hydrogels into Biomimetic Soft Robots. ACS Nano 13, 13176–13184. doi:10.1021/acsnano.9b06144
Colas, A., and Curtis, J. (2004). Silicone Biomaterials : History and Chemistry \\& Medical Applications of Silicones.
Cvetkovic, C., Raman, R., Chan, V., Williams, B. J., Tolish, M., Bajaj, P., et al. (2014). Three-dimensionally Printed Biological Machines Powered by Skeletal Muscle. Proc. Natl. Acad. Sci. 111, 10125–10130. doi:10.1073/pnas.1401577111
Das, S., Chakraborty, P., Shit, A., Mondal, S., and Nandi, A. K. (2016). Robust Hybrid Hydrogels with Good Rectification Properties and Their Application as Active Materials for Dye-Sensitized Solar Cells: Insights from AC Impedance Spectroscopy. J. Mater. Chem. A. 4, 4194–4210. doi:10.1039/C5TA08833J
Dawson, C., Vincent, J. F. V., and Rocca, A.-M. (1997). How pine Cones Open. Nature 390, 668. doi:10.1038/37745
de Buyl, F. (2001). Silicone Sealants and Structural Adhesives. Int. J. Adhes. Adhesives 21, 411–422. doi:10.1016/S0143-7496(01)00018-5
De Mori, A., Peña Fernández, M., Blunn, G., Tozzi, G., and Roldo, M. (2018). 3D Printing and Electrospinning of Composite Hydrogels for Cartilage and Bone Tissue Engineering. Polymers 10, 285. doi:10.3390/polym10030285
DeForest, C. A., and Anseth, K. S. (2012). Advances in Bioactive Hydrogels to Probe and Direct Cell Fate. Annu. Rev. Chem. Biomol. Eng. 3, 421–444. doi:10.1146/annurev-chembioeng-062011-080945
Discher, D. E., Janmey, P., and Wang, Y.-l. (2005). Tissue Cells Feel and Respond to the Stiffness of Their Substrate. Science 310, 1139–1143. doi:10.1126/science.1116995
Drury, J. L., and Mooney, D. J. (2003). Hydrogels for Tissue Engineering: Scaffold Design Variables and Applications. Biomaterials 24, 4337–4351. doi:10.1016/S0142-9612(03)00340-5
Echeverria, C., Fernandes, S., Godinho, M., Borges, J., and Soares, P. (2018). Functional Stimuli-Responsive Gels: Hydrogels and Microgels. Gels 4, 54–37. doi:10.3390/gels4020054
Fan, W., Shan, C., Guo, H., Sang, J., Wang, R., Zheng, R., et al. (2019). Dual-gradient Enabled Ultrafast Biomimetic Snapping of Hydrogel Materials. Sci. Adv. 5, eaav7174. doi:10.1126/sciadv.aav7174
Fratzl, P., and Barth, F. G. (2009). Biomaterial Systems for Mechanosensing and Actuation. Nature 462, 442–448. doi:10.1038/nature08603
Fu, F., Shang, L., Chen, Z., Yu, Y., and Zhao, Y. (2018). Bioinspired Living Structural Color Hydrogels. Sci. Robot. 3, eaar8580. doi:10.1126/scirobotics.aar8580
Fusco, S., Sakar, M. S., Kennedy, S., Peters, C., Pane, S., Mooney, D., et al. (2014). “Self-folding mobile Microrobots for Biomedical Applications,” in 2014 IEEE International Conference on Robotics and Automation (ICRA) (IEEE), 3777–3782. doi:10.1109/ICRA.2014.6907406
Gao, G., Du, G., Sun, Y., and Fu, J. (2015). Self-Healable, Tough, and Ultrastretchable Nanocomposite Hydrogels Based on Reversible Polyacrylamide/Montmorillonite Adsorption. ACS Appl. Mater. Inter. 7, 5029–5037. doi:10.1021/acsami.5b00704
Gong, J. P., Katsuyama, Y., Kurokawa, T., and Osada, Y. (2003). Double-Network Hydrogels with Extremely High Mechanical Strength. Adv. Mater. 15, 1155–1158. doi:10.1002/adma.200304907
Goyal, R., Lakshmi Madhuri, P., Krishna Prasad, S., Hegde, G., and Vivekananda Shanmuganathan, P. (2019). Fast Responsive Soft Bio-Mimetic Robotic Actuators. Mater. Today Proc. 15, 300–308. doi:10.1016/j.matpr.2019.05.009
Hamidi, M., Azadi, A., and Rafiei, P. (2008). Hydrogel Nanoparticles in Drug Delivery. Adv. Drug Deliv. Rev. 60, 1638–1649. doi:10.1016/j.addr.2008.08.002
Han, D., Lu, Z., Chester, S. A., and Lee, H. (2018). Micro 3D Printing of a Temperature-Responsive Hydrogel Using Projection Micro-stereolithography. Sci. Rep. 8, 1963. doi:10.1038/s41598-018-20385-2
Haraguchi, K., Farnworth, R., Ohbayashi, A., and Takehisa, T. (2003). Compositional Effects on Mechanical Properties of Nanocomposite Hydrogels Composed of Poly(N,N-dimethylacrylamide) and Clay. Macromolecules 36, 5732–5741. doi:10.1021/ma034366i
Haraguchi, K., and Takehisa, T. (2002). Nanocomposite Hydrogels: A Unique Organic-Inorganic Network Structure with Extraordinary Mechanical, Optical, and Swelling/De-Swelling Properties. Adv. Mater. 14, 1120. doi:10.1002/1521-4095(20020816)14:16<1120::aid-adma1120>3.0.co;2-9
Hassan, C. M., and Peppas, N. A. (2000). “Structure and Applications of Poly(vinyl Alcohol) Hydrogels Produced by Conventional Crosslinking or by Freezing/Thawing Methods,” in Biopolymers PVA Hydrogels, Anionic Polymerisation Nanocomposites (Berlin, Heidelberg: Springer Berlin Heidelberg), 37–65. doi:10.1007/3-540-46414-X_2
He, Q., Wang, Z., Yan, Y., Zheng, J., and Cai, S. (2016). Polymer Nanofiber Reinforced Double Network Gel Composite: Strong, Tough and Transparent. Extreme Mech. Lett. 9, 165–170. doi:10.1016/j.eml.2016.06.004
Hoare, T., Santamaria, J., Goya, G. F., Irusta, S., Lin, D., Lau, S., et al. (2009). A Magnetically Triggered Composite Membrane for On-Demand Drug Delivery. Nano Lett. 9, 3651–3657. doi:10.1021/nl9018935
Hoffman, A. S. (2012). Hydrogels for Biomedical Applications. Adv. Drug Deliv. Rev. 64, 18–23. doi:10.1016/j.addr.2012.09.010
Holzapfel, G. A., and Gasser, T. C. (2001). A Viscoelastic Model for Fiber-Reinforced Composites at Finite Strains: Continuum Basis, Computational Aspects and Applications. Computer Methods Appl. Mech. Eng. 190, 4379–4403. doi:10.1016/S0045-7825(00)00323-6
Hong, Y., Huber, A., Takanari, K., Amoroso, N. J., Hashizume, R., Badylak, S. F., et al. (2011). Mechanical Properties and In Vivo Behavior of a Biodegradable Synthetic Polymer Microfiber-Extracellular Matrix Hydrogel Biohybrid Scaffold. Biomaterials 32, 3387–3394. doi:10.1016/j.biomaterials.2011.01.025
Hong, Y., Song, H., Gong, Y., Mao, Z., Gao, C., and Shen, J. (2007). Covalently Crosslinked Chitosan Hydrogel: Properties of In Vitro Degradation and Chondrocyte Encapsulation. Acta Biomater. 3, 23–31. doi:10.1016/j.actbio.2006.06.007
Hou, K., Nie, Y., Tendo Mugaanire, I., Guo, Y., and Zhu, M. (2020). A Novel Leaf Inspired Hydrogel Film Based on Fiber Reinforcement as Rapid Steam Sensor. Chem. Eng. J. 382, 122948. doi:10.1016/j.cej.2019.122948
Hsu, S.-h., Chen, C.-W., Hung, K.-C., Tsai, Y.-C., and Li, S. (2016). Thermo-Responsive Polyurethane Hydrogels Based on Poly(ε-Caprolactone) Diol and Amphiphilic Polylactide-Poly(Ethylene Glycol) Block Copolymers. Polymers 8, 252. doi:10.3390/polym8070252
Huang, G., Li, F., Zhao, X., Ma, Y., Li, Y., Lin, M., et al. (2017). Functional and Biomimetic Materials for Engineering of the Three-Dimensional Cell Microenvironment. Chem. Rev. 117, 12764–12850. doi:10.1021/acs.chemrev.7b00094
Hutmacher, D. W. (2000). Scaffolds in Tissue Engineering Bone and Cartilage. Biomaterials 21, 2529–2543. doi:10.1016/S0142-9612(00)00121-6
Ilievski, F., Mazzeo, A. D., Shepherd, R. F., Chen, X., and Whitesides, G. M. (2011). Soft Robotics for Chemists. Angew. Chem. Int. Ed. 50, 1890–1895. doi:10.1002/anie.201006464
Ionov, L. (2010). Actively-moving Materials Based on Stimuli-Responsive Polymers. J. Mater. Chem. 20, 3382. doi:10.1039/b922718k
Ionov, L. (2013). Biomimetic Hydrogel-Based Actuating Systems. Adv. Funct. Mater. 23, 4555–4570. doi:10.1002/adfm.201203692
Ismail, Y. A., Martínez, J. G., Al Harrasi, A. S., Kim, S. J., and Otero, T. F. (2011). Sensing Characteristics of a Conducting Polymer/hydrogel Hybrid Microfiber Artificial Muscle. Sensors Actuators B: Chem. 160, 1180–1190. doi:10.1016/j.snb.2011.09.044
Iwaso, K., Takashima, Y., and Harada, A. (2016). Fast Response Dry-type Artificial Molecular Muscles with [c2]daisy Chains. Nat. Chem 8, 625–632. doi:10.1038/nchem.2513
Ji, D., and Kim, J. (2021). Recent Strategies for Strengthening and Stiffening Tough Hydrogels. Adv. Nanobio Res. 1, 2100026. doi:10.1002/anbr.202100026
Khademhosseini, A., and Langer, R. (2016). A Decade of Progress in Tissue Engineering. Nat. Protoc. 11, 1775–1781. doi:10.1038/nprot.2016.123
Kim, J., Hanna, J. A., Byun, M., Santangelo, C. D., and Hayward, R. C. (2012). Designing Responsive Buckled Surfaces by Halftone Gel Lithography. Science 335, 1201–1205. doi:10.1126/science.1215309
Kim, S. J., Kim, H. I., Park, S. J., Kim, I. Y., Lee, S. H., Lee, T. S., et al. (2005). Behavior in Electric fields of Smart Hydrogels with Potential Application as Bio-Inspired Actuators. Smart Mater. Struct. 14, 511–514. doi:10.1088/0964-1726/14/4/008
Kishi, R., Ichijo, H., and Hirasa, O. (1993). Thermo-Responsive Devices Using Poly(vinyl Methyl Ether) Hydrogels. J. Intell. Mater. Syst. Structures 4, 533–537. doi:10.1177/1045389X9300400413
Kotzar, G., Freas, M., Abel, P., Fleischman, A., Roy, S., Zorman, C., et al. (2002). Evaluation of MEMS Materials of Construction for Implantable Medical Devices. Biomaterials 23, 2737–2750. doi:10.1016/S0142-9612(02)00007-8
Kue, R., Sohrabi, A., Nagle, D., Frondoza, C., and Hungerford, D. (1999). Enhanced Proliferation and Osteocalcin Production by Human Osteoblast-like MG63 Cells on Silicon Nitride Ceramic Discs. Biomaterials 20, 1195–1201. doi:10.1016/S0142-9612(99)00007-1
Kwon, G. H., Choi, Y. Y., Park, J. Y., Woo, D. H., Lee, K. B., Kim, J. H., et al. (2010). Electrically-driven Hydrogel Actuators in Microfluidic Channels: Fabrication, Characterization, and Biological Application. Lab. Chip 10, 1604. doi:10.1039/b926443d
Kwon, G. H., Park, J. Y., Kim, J. Y., Frisk, M. L., Beebe, D. J., and Lee, S.-H. (2008). Biomimetic Soft Multifunctional Miniature Aquabots. Small 4, 2148–2153. doi:10.1002/smll.200800315
Langer, R. (1990). New Methods of Drug Delivery. Science 249, 1527–1533. doi:10.1126/science.2218494
Lee, K. Y., and Mooney, D. J. (2001). Hydrogels for Tissue Engineering. Chem. Rev. 101, 1869–1880. doi:10.1021/cr000108x
Leijten, J., Seo, J., Yue, K., Trujillo-de Santiago, G., Tamayol, A., Ruiz-Esparza, G. U., et al. (2017). Spatially and Temporally Controlled Hydrogels for Tissue Engineering. Mater. Sci. Eng. R: Rep. 119, 1–35. doi:10.1016/j.mser.2017.07.001
Li, J., Illeperuma, W. R. K., Suo, Z., and Vlassak, J. J. (2014). Hybrid Hydrogels with Extremely High Stiffness and Toughness. ACS Macro Lett. 3, 520–523. doi:10.1021/mz5002355
Liao, I.-C., Moutos, F. T., Estes, B. T., Zhao, X., and Guilak, F. (2013). Composite Three-Dimensional Woven Scaffolds with Interpenetrating Network Hydrogels to Create Functional Synthetic Articular Cartilage. Adv. Funct. Mater. 23, 5833–5839. doi:10.1002/adfm.201300483
Lichtenegger, H., Müller, M., Paris, O., Riekel, C., and Fratzl, P. (1999). Imaging of the Helical Arrangement of Cellulose Fibrils in wood by Synchrotron X-ray Microdiffraction. J. Appl. Cryst. 32, 1127–1133. doi:10.1107/S0021889899010961
Lin, H., Ma, S., Yu, B., Cai, M., Zheng, Z., Zhou, F., et al. (2019). Fabrication of Asymmetric Tubular Hydrogels through Polymerization-Assisted Welding for Thermal Flow Actuated Artificial Muscles. Chem. Mater. 31, 4469–4478. doi:10.1021/acs.chemmater.9b00965
Lin, S., Cao, C., Wang, Q., Gonzalez, M., Dolbow, J. E., and Zhao, X. (2014). Design of Stiff, Tough and Stretchy Hydrogel Composites via Nanoscale Hybrid Crosslinking and Macroscale Fiber Reinforcement. Soft Matter 10, 7519–7527. doi:10.1039/C4SM01039F
Liu, S., Gao, G., Xiao, Y., and Fu, J. (2016). Tough and Responsive Oppositely Charged Nanocomposite Hydrogels for Use as Bilayer Actuators Assembled through Interfacial Electrostatic Attraction. J. Mater. Chem. B 4, 3239–3246. doi:10.1039/C6TB00583G
Liu, Z., and Calvert, P. (2000). Multilayer Hydrogels as Muscle-like Actuators. Adv. Mater. 12, 288–291. doi:10.1002/(sici)1521-4095(200002)12:4<288::aid-adma288>3.0.co;2-1
Löwenberg, C., Balk, M., Wischke, C., Behl, M., and Lendlein, A. (2017). Shape-Memory Hydrogels: Evolution of Structural Principles to Enable Shape Switching of Hydrophilic Polymer Networks. Acc. Chem. Res. 50, 723–732. doi:10.1021/acs.accounts.6b00584
Lv, C., Sun, X.-C., Xia, H., Yu, Y.-H., Wang, G., Cao, X.-W., et al. (2018). Humidity-responsive Actuation of Programmable Hydrogel Microstructures Based on 3D Printing. Sensors Actuators B: Chem. 259, 736–744. doi:10.1016/j.snb.2017.12.053
Ma, C., Li, T., Zhao, Q., Yang, X., Wu, J., Luo, Y., et al. (2014). Supramolecular Lego Assembly towards Three-Dimensional Multi-Responsive Hydrogels. Adv. Mater. 26, 5665–5669. doi:10.1002/adma.201402026
Ma, Y., Feng, X., Rogers, J. A., Huang, Y., and Zhang, Y. (2017). Design and Application of 'J-Shaped' Stress-Strain Behavior in Stretchable Electronics: a Review. Lab. Chip 17, 1689–1704. doi:10.1039/C7LC00289K
Malda, J., Visser, J., Melchels, F. P., Jüngst, T., Hennink, W. E., Dhert, W. J. A., et al. (2013). 25th Anniversary Article: Engineering Hydrogels for Biofabrication. Adv. Mater. 25, 5011–5028. doi:10.1002/adma.201302042
Mao, L., Hu, Y., Piao, Y., Chen, X., Xian, W., and Piao, D. (2005). Structure and Character of Artificial Muscle Model Constructed from Fibrous Hydrogel. Curr. Appl. Phys. 5, 426–428. doi:10.1016/j.cap.2004.11.003
McCracken, J. M., Rauzan, B. M., Kjellman, J. C. E., Su, H., Rogers, S. A., and Nuzzo, R. G. (2019). Ionic Hydrogels with Biomimetic 4D‐Printed Mechanical Gradients: Models for Soft‐Bodied Aquatic Organisms. Adv. Funct. Mater. 29, 1806723. doi:10.1002/adfm.201806723
Morales, D., Palleau, E., Dickey, M. D., and Velev, O. D. (2014). Electro-actuated Hydrogel Walkers with Dual Responsive Legs. Soft Matter 10, 1337–1348. doi:10.1039/C3SM51921J
Moschou, E. A., Peteu, S. F., Bachas, L. G., Madou, M. J., and Daunert, S. (2004). Artificial Muscle Material with Fast Electroactuation under Neutral pH Conditions. Chem. Mater. 16, 2499–2502. doi:10.1021/cm049921p
Naji, A., and Harmand, M.-F. (1991). Cytocompatibility of Two Coating Materials, Amorphous Alumina and Silicon Carbide, Using Human Differentiated Cell Cultures. Biomaterials 12, 690–694. doi:10.1016/0142-9612(91)90118-T
Nakahata, M., Takashima, Y., Hashidzume, A., and Harada, A. (2013). Redox-Generated Mechanical Motion of a Supramolecular Polymeric Actuator Based on Host-Guest Interactions. Angew. Chem. Int. Ed. 52, 5731–5735. doi:10.1002/anie.201300862
Nguyen, K. T., and West, J. L. (2002). Photopolymerizable Hydrogels for Tissue Engineering Applications. Biomaterials 23, 4307–4314. doi:10.1016/S0142-9612(02)00175-8
Ni, Y., Ji, R., Long, K., Bu, T., Chen, K., and Zhuang, S. (2017). A Review of 3D-Printed Sensors. Appl. Spectrosc. Rev. 52, 623–652. doi:10.1080/05704928.2017.1287082
Nikouei, N. S., Ghasemi, N., and Lavasanifar, A. (2016). Temperature/pH Responsive Hydrogels Based on Poly(ethylene Glycol) and Functionalized Poly(e-Caprolactone) Block Copolymers for Controlled Delivery of Macromolecules. Pharm. Res. 33, 358–366. doi:10.1007/s11095-015-1794-z
Oh, J. K., Drumright, R., Siegwart, D. J., and Matyjaszewski, K. (2008). The Development of Microgels/nanogels for Drug Delivery Applications. Prog. Polym. Sci. 33, 448–477. doi:10.1016/j.progpolymsci.2008.01.002
Osada, Y. (1991). Chemical Valves and Gel Actuators. Adv. Mater. 3, 107–108. doi:10.1002/adma.19910030209
Otake, M., Kagami, Y., Inaba, M., and Inoue, H. (2002). Motion Design of a Starfish-Shaped Gel Robot Made of Electro-Active Polymer Gel. Robotics Autonomous Syst. 40, 185–191. doi:10.1016/S0921-8890(02)00243-9
Park, S.-J., Gazzola, M., Park, K. S., Park, S., Di Santo, V., Blevins, E. L., et al. (2016). Phototactic Guidance of a Tissue-Engineered Soft-Robotic ray. Science 353, 158–162. doi:10.1126/science.aaf4292
Peppas, N. A., Hilt, J. Z., Khademhosseini, A., and Langer, R. (2006). Hydrogels in Biology and Medicine: From Molecular Principles to Bionanotechnology. Adv. Mater. 18, 1345–1360. doi:10.1002/adma.200501612
Pradas, M. M., and Calleja, R. D. (1990). Nonlinear Viscoelastic Behaviour of the Flexor Tendon of the Human Hand. J. Biomech. 23, 773–781. doi:10.1016/0021-9290(90)90024-W
Qiu, Y., and Park, K. (2001). Environment-sensitive Hydrogels for Drug Delivery. Adv. Drug Deliv. Rev. 53, 321–339. doi:10.1016/S0169-409X(01)00203-4
Qiu, Y., Zhang, E., Plamthottam, R., and Pei, Q. (2019). Dielectric Elastomer Artificial Muscle: Materials Innovations and Device Explorations. Acc. Chem. Res. 52, 316–325. doi:10.1021/acs.accounts.8b00516
Rafat, M., Li, F., Fagerholm, P., Lagali, N. S., Watsky, M. A., Munger, R., et al. (2008). PEG-stabilized Carbodiimide Crosslinked Collagen-Chitosan Hydrogels for Corneal Tissue Engineering. Biomaterials 29, 3960–3972. doi:10.1016/j.biomaterials.2008.06.017
Reyssat, E., and Mahadevan, L. (2009). Hygromorphs: from pine Cones to Biomimetic Bilayers. J. R. Soc. Interf. 6, 951–957. doi:10.1098/rsif.2009.0184
Roche, E. T., Horvath, M. A., Wamala, I., Alazmani, A., Song, S.-E., Whyte, W., et al. (2017). Soft Robotic Sleeve Supports Heart Function. Sci. Transl. Med. 9, eaaf3925. doi:10.1126/scitranslmed.aaf3925
Roeder, L., Bender, P., Tschöpe, A., Birringer, R., and Schmidt, A. M. (2012). Shear Modulus Determination in Model Hydrogels by Means of Elongated Magnetic Nanoprobes. J. Polym. Sci. B Polym. Phys. 50, 1772–1781. doi:10.1002/polb.23202
Rossiter, J., Winfield, J., and Ieropoulos, I. (2016). Here Today, Gone Tomorrow: Biodegradable Soft Robots. Here Today, Gone Tomorrow: Biodegradable Soft Robots. in, eds. Y. Bar-Cohen, and F. Vidal, 97981S. doi:10.1117/12.2220611
Sahoo, N. G., Jung, Y. C., Goo, N. S., and Cho, J. W. (2005). Conducting Shape Memory Polyurethane-Polypyrrole Composites for an Electroactive Actuator. Macromol. Mater. Eng. 290, 1049–1055. doi:10.1002/mame.200500211
Sano, K., Ishida, Y., and Aida, T. (2018). Synthesis of Anisotropic Hydrogels and Their Applications. Angew. Chem. Int. Ed. 57, 2532–2543. doi:10.1002/anie.201708196
Satarkar, N. S., Zhang, W., Eitel, R. E., and Hilt, J. Z. (2009). Magnetic Hydrogel Nanocomposites as Remote Controlled Microfluidic Valves. Lab. Chip 9, 1773. doi:10.1039/b822694f
Seidi, A., Ramalingam, M., Elloumi-Hannachi, I., Ostrovidov, S., and Khademhosseini, A. (2011). Gradient Biomaterials for Soft-To-Hard Interface Tissue Engineering. Acta Biomater. 7, 1441–1451. doi:10.1016/j.actbio.2011.01.011
Shankar, A., Safronov, A. P., Mikhnevich, E. A., and Beketov, I. V. (2017). Multidomain Iron Nanoparticles for the Preparation of Polyacrylamide Ferrogels. J. Magnetism Magn. Mater. 431, 134–137. doi:10.1016/j.jmmm.2016.08.075
Shepherd, R. F., Ilievski, F., Choi, W., Morin, S. A., Stokes, A. A., Mazzeo, A. D., et al. (2011). Multigait Soft Robot. Proc. Natl. Acad. Sci. 108, 20400–20403. doi:10.1073/pnas.1116564108
Shi, Q., Liu, H., Tang, D., Li, Y., Li, X., and Xu, F. (2019). Bioactuators Based on Stimulus-Responsive Hydrogels and Their Emerging Biomedical Applications. NPG Asia Mater. 11. doi:10.1038/s41427-019-0165-3
Shiga, T., Hirose, Y., Okada, A., and Kurauchi, T. (1993). Electrically Driven Polymer Gel Finger Working in the Air. J. Intell. Mater. Syst. Structures 4, 553–557. doi:10.1177/1045389X9300400417
Shikinami, Y., Kotani, Y., Cunningham, B. W., Abumi, K., and Kaneda, K. (2004). A Biomimetic Artificial Disc with Improved Mechanical Properties Compared to Biological Intervertebral Discs. Adv. Funct. Mater. 14, 1039–1046. doi:10.1002/adfm.200305038
Sidorenko, A., Krupenkin, T., Taylor, A., Fratzl, P., and Aizenberg, J. (2007). Reversible Switching of Hydrogel-Actuated Nanostructures into Complex Micropatterns. Science 315, 487–490. doi:10.1126/science.1135516
Slaughter, B. V., Khurshid, S. S., Fisher, O. Z., Khademhosseini, A., and Peppas, N. A. (2009). Hydrogels in Regenerative Medicine. Adv. Mater. 21, 3307–3329. doi:10.1002/adma.200802106
Stanton, M. M., Samitier, J., and Sánchez, S. (2015). Bioprinting of 3D Hydrogels. Lab. Chip 15, 3111–3115. doi:10.1039/C5LC90069G
Studart, A. R. (2015). Biologically Inspired Dynamic Material Systems. Angew. Chem. Int. Ed. 54, 3400–3416. doi:10.1002/anie.201410139
Suntornnond, R., Tan, E., An, J., and Chua, C. (2016). A Mathematical Model on the Resolution of Extrusion Bioprinting for the Development of New Bioinks. Materials 9, 756. doi:10.3390/ma9090756
Sydney Gladman, A., Matsumoto, E. A., Nuzzo, R. G., Mahadevan, L., and Lewis, J. A. (2016). Biomimetic 4D Printing. Nat. Mater 15, 413–418. doi:10.1038/nmat4544
Takashima, Y., Hatanaka, S., Otsubo, M., Nakahata, M., Kakuta, T., Hashidzume, A., et al. (2012). Expansion-contraction of Photoresponsive Artificial Muscle Regulated by Host-Guest Interactions. Nat. Commun. 3, 1270. doi:10.1038/ncomms2280
Tan, H., Chu, C. R., Payne, K. A., and Marra, K. G. (2009). Injectable In Situ Forming Biodegradable Chitosan-Hyaluronic Acid Based Hydrogels for Cartilage Tissue Engineering. Biomaterials 30, 2499–2506. doi:10.1016/j.biomaterials.2008.12.080
Tang, J., Yin, Q., Qiao, Y., and Wang, T. (2019). Shape Morphing of Hydrogels in Alternating Magnetic Field. ACS Appl. Mater. Inter. 11, 21194–21200. doi:10.1021/acsami.9b05742
Tobita, H., and Hamielec, A. E. (1992). Control of Network Structure in Free-Radical Crosslinking Copolymerization. Polymer 33, 3647–3657. doi:10.1016/0032-3861(92)90651-C
Tokarev, I., and Minko, S. (2009). Stimuli-responsive Hydrogel Thin Films. Soft Matter 5, 511–524. doi:10.1039/B813827C
Tolley, M. T., Shepherd, R. F., Mosadegh, B., Galloway, K. C., Wehner, M., Karpelson, M., et al. (2014). A Resilient, Untethered Soft Robot. Soft Robotics 1, 213–223. doi:10.1089/soro.2014.0008
Uhrich, K. E., Cannizzaro, S. M., Langer, R. S., and Shakesheff, K. M. (1999). Polymeric Systems for Controlled Drug Release. Chem. Rev. 99, 3181–3198. doi:10.1021/cr940351u
van Rees, W. M., Vouga, E., and Mahadevan, L. (2017). Growth Patterns for Shape-Shifting Elastic Bilayers. Proc. Natl. Acad. Sci. USA 114, 11597–11602. doi:10.1073/pnas.1709025114
Wagermaier, W., S. Gupta, H. H., Gourrier, A., Burghammer, M., Roschger, P., and Fratzl, P. (2006). Spiral Twisting of Fiber Orientation inside Bone Lamellae. Biointerphases 1, 1–5. doi:10.1116/1.2178386
Wang, E., Desai, M. S., and Lee, S.-W. (2013). Light-Controlled Graphene-Elastin Composite Hydrogel Actuators. Nano Lett. 13, 2826–2830. doi:10.1021/nl401088b
Webber, M. J., Appel, E. A., Meijer, E. W., and Langer, R. (2016). Supramolecular Biomaterials. Nat. Mater 15, 13–26. doi:10.1038/nmat4474
Wehner, M., Truby, R. L., Fitzgerald, D. J., Mosadegh, B., Whitesides, G. M., Lewis, J. A., et al. (2016). An Integrated Design and Fabrication Strategy for Entirely Soft, Autonomous Robots. Nature 536, 451–455. doi:10.1038/nature19100
Wei, M., Gao, Y., Li, X., and Serpe, M. J. (2017). Stimuli-responsive Polymers and Their Applications. Polym. Chem. 8, 127–143. doi:10.1039/C6PY01585A
Wu, Z. L., Moshe, M., Greener, J., Therien-Aubin, H., Nie, Z., Sharon, E., et al. (2013). Three-dimensional Shape Transformations of Hydrogel Sheets Induced by Small-Scale Modulation of Internal Stresses. Nat. Commun. 4, 1586. doi:10.1038/ncomms2549
Xiao, S., Yang, Y., Zhong, M., Chen, H., Zhang, Y., Yang, J., et al. (2017). Salt-Responsive Bilayer Hydrogels with Pseudo-double-network Structure Actuated by Polyelectrolyte and Antipolyelectrolyte Effects. ACS Appl. Mater. Inter. 9, 20843–20851. doi:10.1021/acsami.7b04417
Xue, B., Qin, M., Wang, T., Wu, J., Luo, D., Jiang, Q., et al. (2016). Electrically Controllable Actuators Based on Supramolecular Peptide Hydrogels. Adv. Funct. Mater. 26, 9053–9062. doi:10.1002/adfm.201603947
Yang, C., Liu, Z., Chen, C., Shi, K., Zhang, L., Ju, X.-J., et al. (2017). Reduced Graphene Oxide-Containing Smart Hydrogels with Excellent Electro-Response and Mechanical Properties for Soft Actuators. ACS Appl. Mater. Inter. 9, 15758–15767. doi:10.1021/acsami.7b01710
Yang, Q., Yang, W., Duan, J., and Yang, P. (2018). A Series of Conducting Gel Electrolytes for Quasi-Solid-State Quantum Dot-Sensitized Solar Cells with Boosted Electron Transfer Processes. J. Energ. Chem. 27, 335–341. doi:10.1016/j.jechem.2017.12.010
Yin, S.-N., Wang, C.-F., Liu, S.-S., and Chen, S. (2013). Facile Fabrication of Tunable Colloidal Photonic crystal Hydrogel Supraballs toward a Colorimetric Humidity Sensor. J. Mater. Chem. C 1, 4685. doi:10.1039/c3tc30659c
Yoda, R. (1998). Elastomers for Biomedical Applications. J. Biomater. Sci. Polym. Edition 9, 561–626. doi:10.1163/156856298X00046
Yu, C., Duan, Z., Yuan, P., Li, Y., Su, Y., Zhang, X., et al. (2013). Electronically Programmable, Reversible Shape Change in Two- and Three-Dimensional Hydrogel Structures. Adv. Mater. 25, 1541–1546. doi:10.1002/adma.201204180
Yuk, H., Lin, S., Ma, C., Takaffoli, M., Fang, N. X., and Zhao, X. (2017). Hydraulic Hydrogel Actuators and Robots Optically and Sonically Camouflaged in Water. Nat. Commun. 8, 14230. doi:10.1038/ncomms14230
Zhalmuratova, D., and Chung, H.-J. (2020). Reinforced Gels and Elastomers for Biomedical and Soft Robotics Applications. ACS Appl. Polym. Mater. 2, 1073–1091. doi:10.1021/acsapm.9b01078
Zhang, X., Pint, C. L., Lee, M. H., Schubert, B. E., Jamshidi, A., Takei, K., et al. (2011). Optically- and Thermally-Responsive Programmable Materials Based on Carbon Nanotube-Hydrogel Polymer Composites. Nano Lett. 11, 3239–3244. doi:10.1021/nl201503e
Zhang, Y., Liao, J., Wang, T., Sun, W., and Tong, Z. (2018). Polyampholyte Hydrogels with pH Modulated Shape Memory and Spontaneous Actuation. Adv. Funct. Mater. 28, 1707245. doi:10.1002/adfm.201707245
Zhang, Y., and Wang, Q. (2012). Magnetic-Plasmonic Dual Modulated FePt-Au Ternary Heterostructured Nanorods as a Promising Nano-Bioprobe. Adv. Mater. 24, 2485–2490. doi:10.1002/adma.201103991
Zhao, X. (2017). Designing Toughness and Strength for Soft Materials. Proc. Natl. Acad. Sci. USA 114, 8138–8140. doi:10.1073/pnas.1710942114
Zolfagharian, A., Kouzani, A. Z., Khoo, S. Y., Gibson, I., and Kaynak, A. (2016). “3D Printed Hydrogel Soft Actuators,” in 2016 IEEE Region 10 Conference (TENCON) (IEEE), 2272–2277. doi:10.1109/TENCON.2016.7848433
Keywords: biomimetic, robotic actuators, reinforced composite, stimulus-responsive, shape morphing
Citation: Goyal R and Mitra S (2022) A Brief Overview of Bioinspired Robust Hydrogel Based Shape Morphing Functional Structure for Biomedical Soft Robotics. Front. Mater. 9:837923. doi: 10.3389/fmats.2022.837923
Received: 17 December 2021; Accepted: 21 February 2022;
Published: 12 April 2022.
Edited by:
Anuj Kumar, Yeungnam University, South KoreaReviewed by:
Dejian Li, Fudan University Pudong Medical Center, ChinaDiana Ciolacu, Institute of Macromolecular Chemistry “Petru Poni”, Romania
Atul Dev, University of California, Davis, United States
Copyright © 2022 Goyal and Mitra. This is an open-access article distributed under the terms of the Creative Commons Attribution License (CC BY). The use, distribution or reproduction in other forums is permitted, provided the original author(s) and the copyright owner(s) are credited and that the original publication in this journal is cited, in accordance with accepted academic practice. No use, distribution or reproduction is permitted which does not comply with these terms.
*Correspondence: Santanu Mitra, c2FudGFudS5taXRyYUBzbnUuZWR1Lmlu