- 1Department of Pharmaceutical Technology, Brainware University, Kolkata, India
- 2Koringa College of Pharmacy, Kakinada, India
- 3Department of Pharmaceutical Technology, Jadavpur University, Kolkata, India
- 4Department of Pharmaceutics, Gupta College of Technological Sciences, Asansol, India
- 5Department of Chemistry, Indira Gandhi National Tribal University, Amarkantak, India
In this study, matrix-type transdermal patches of glibenclamide were developed using a combination of hydrophilic and hydrophobic polymers for investigating the efficacy of transdermal carriers. A cellulose derivative, HPMC E50, was used as a hydrophilic matrix-forming polymer, and Eudragit RS 100 was used as a hydrophobic polymer. The solvent casting technique was employed to develop a transdermal blend patch formulation using chloroform and methanol as the casting solvent. No drug–polymer interaction was observed by the FTIR study. The membrane permeation study exhibited a sustained release of glibenclamide up to 12 h within a range of 76.15 ± 2.80% to 101.01 ± 0.33% depending on the polymeric ratio. The increased concentration of Eudragit RS 100 in different formulations has gradually decreased the amount of drug penetration through the membrane. The kinetic analysis showed the release is best explained by zero-order kinetics, followed by Higuchi and first order. The release exponent suggested shifting of non-Fickian diffusion to the super case II transport drug release mechanism when Eudragit RS 100 concentration was increased. It is concluded that the developed formulations may be a better alternative to the conventional oral delivery of glibenclamide.
1 Introduction
Since the emergence of transdermal patches, it has been considered one of the prime delivery systems for therapeutics. Transdermal drug delivery systems (TDDS) are presently regarded as a traditional means of avoiding hepatic first-pass metabolism and delivering lipophilic drugs. The transdermal application also prevents enzymatic degradation and acid-mediated decomposition of drugs (Al Hanbali et al., 2019). This technique is also useful for transporting low-molecular-weight drugs over a prolonged time span (Mutalik and Udupa 2004; Davis et al., 2020). Other major advantages of transdermally administered systems are self-administration, patient compliance, controlled release of therapeutics, and instant cessation of drug uptake by removing the patch (Alkilani et al., 2015). Continuous penetration of drugs also facilitates lowering the dose minimizing the side effects caused due to higher plasma concentration of the drug. Despite having numerous advantages, there are few drawbacks to transdermal films, that is, delivering ionic and macromolecular drugs, skin irritability, and patients with decreased peripheral blood circulation (Murphy and Carmichael 2000). According to the current scenario, transdermal uptake of a drug depends on the molecular weight of the drug, partition coefficient, degree of ionization, and hydrophilic–lipophilic balance of polymers (Chandrashekar and Shobha Rani, 2008; Saoji et al., 2015). The highly selective barrier nature of skin limits the passage of drugs and acts as a dominant factor in transdermal absorption. The release of the medicament from a transdermal polymeric matrix can depend on the polymer ratio and permeation enhancer. According to the recent trend in transdermal delivery, drugs can also be incorporated into the adhesive layer of transdermal films (Davis et al., 2020). This approach has many advantages, including the lightweight, thin and flexible delivery system which increases patient compliance. It also minimizes the chance of drug leakage with respect to reservoir-type transdermal patches (Li et al., 2010).
Cellulose derivatives found unparalleled application as excipients in the field of pharmaceutical research and the manufacturing industry. Hydroxypropyl methylcellulose (HPMC) is one of the widely applied cellulose-based pharmaceutical excipients. This hydrophilic matrix forming polymer found extensive application in developing oral, mucosal, and transdermal delivery systems. The swellable nature and excellent safety profile, along with its enzymatic and pH-independent stability have made it an automatic choice as a hydrophilic matrix-forming agent (Mašková et al., 2020). In the molecular structure of HPMC, hydroxypropyl and methoxyl groups are attached to the linear chain of cellulose (Ford, 2014). Depending on the ratio and degree of substitution, variations in molecular weight are observed. The insignificant influence of pH and non-ionic behavior of the polymer ensures reproducible release of drug and nominal drug interaction (Nokhodchi et al., 2012; Mašková et al., 2020). The availability of various grades of HPMC has contributed to the need of designing individual drug delivery systems. This bioadhesive matrix forming agent is being widely applied in developing TDDS. A relatively less viscous grade, HPMC E50 (Hydroxypropyl methylcellulose E50- E type 2910; M.W.: 90000 Da), was selected as one of the matrices forming agents for the developed TDDS.
Eudragit was introduced as a pharmaceutical excipient in 1954 and since then it has revolutionized the concept of drug targeting. Chemically, Eudragits are polymethacrylates which are non-ionic, anionic, and cationic polymers of methacrylic acid, methacrylic acid esters, and diethylaminoethyl methacrylates in changing ratios (Yoshida et al., 2013). Eudragits are generally considered non-irritant and non-toxic in nature. It is commonly applied as a film-forming polymer in site-specific gastrointestinal delivery of drugs. The solubility of the films can be altered depending on the need for dosage forms by changing the grades of the polymer. It has also found application in pH-dependent drug release, formulation of mucoadhesive films, colon specific drug targeting, and enteric coating for enhancing stability and oral bioavailability of therapeutics (Thakral et al., 2013). Over the years, as various grades of eudragit became available, it has found widespread application in the field of drug delivery. The application of Eudragit in TDDS was extensively reported by various researchers. The transparent and elastic film forming ability of the polymer has been attributed to the development of transdermal patches (Tran and Tran, 2019). The adhesive property of the polymer helps in bioadhesion. Wrinkle free pale yellowish transdermal matrix can be developed which undergoes erosion to release the entrapped drug. Eudragit RS100 (ethyl prop-2-enoate; methyl 2-methylprop-2-enoate; trimethyl-[2-(2-methylprop-2-enoyloxy) ethyl] azanium; chloride; M.W.: 407.9 Da) having low permeability and sustained release ability which was utilized to formulate matrix based transdermal patches (Jafri et al., 2019).
Glibenclamide is chemically known as 5-chloro-N-{4-[N-(cyclohexyl-carbamoyl) sulfamoyl] phenethyl}-2-methoxy benzamide (Maiti et al., 2014). The plasma half-life of glibenclamide is about 4–6 h which leads to repeated dosing for maintaining therapeutic concentration in vivo (Nayak et al., 2012). This second-generation sulfonylurea is used in the therapy of non-insulin-dependent diabetes mellitus (NIDDM). Glibenclamide can stimulate insulin secretion by closing the ATP-sensitive ion channels which elevate the intracellular concentration of potassium and calcium ions in beta cells (Davis and Granner, 1996). The increased concentration of calcium ions can enhance the secretion of insulin causing hypoglycemia. Various sulfonylureas, including glibenclamide, are associated with serious hypoglycemia, and gastric side effects including vomiting, heartburn, nausea, and anorexia (Reynolds, 1993). Glibenclamide and its metabolites are reported to show hypoglycemic effects on humans owing to the increased secretion of insulin (Rydberg et al., 1994). Furthermore, Glibenclamide is reported to worsen Indomethacin-induced gastric injury and infiltration of neutrophils into the gastrointestinal mucosa (Akar et al., 1999). Oral therapy of glibenclamide can also result in increased appetite. Due to the hypoglycemic effect of glibenclamide, the use of the drug is not recommended for patients suffering from renal impairment (Mutalik and Udupa 2004). Glibenclamide is highly lipophilic and weakly acidic in nature. The use of polymeric blends in the field of drug delivery is gaining interest amongst researchers (Ghasemiyeh and Mohammadi-Samani 2021; Raza et al., 2021). Considering the adverse effects of the drug, an alternative approach to deliver glibenclamide at a controlled rate could be an acceptable approach for achieving a therapeutic level with reduced adverse effects. In this study, we have designed a novel polymeric blended transdermal system for delivering glibenclamide. A combination of hydrophilic and hydrophobic polymers including HPMC E50 and Eudragit RS 100, respectively in varying concentrations was used in order to achieve sustained release of glibenclamide through the skin. The study also aimed to investigate the effect of polymeric concentrations on physicochemical properties of the patch and drug release patterns. Various in vitro evaluations were performed for the characterization and optimization of the formulations.
2 Materials and Methods
2.1 Materials
Glibenclamide was obtained from TCI Chemicals India Pvt., Ltd. HPMC E50 and Eudragit RS 100 were commercially procured from Nice Chemicals Ltd., Bangalore. PEG 400 and propylene glycol were purchased from SD Fine Chemicals Ltd. Methanol and chloroform were procured from Molychem Chemicals Ltd. All the chemicals used were of analytical grades.
2.2 Methods
2.2.1 Fourier Transform-Infrared Spectroscopy
The IR spectra of pure glibenclamide, HPMC E50, Eudragit RS 100, and a physical mixture of glibenclamide with polymers were obtained after scanning of KBr mixed pellets using Shimadzu FTIR spectrophotometer (IRPrestige- 21). The samples were scanned within the wave number range of 4000–400 cm−1 with a scanning speed of 2 mm/s.
2.2.2 Preparation of Glibenclamide-Loaded Transdermal Patch
The transdermal patches of glibenclamide were prepared by solvent casting technique by dissolving the polymers in chloroform and methanol (Cherukuri et al., 2017). HPMC E50 and Eudragit RS100 were dissolved in chloroform and methanol (1:1 ratio) as a casting solvent mixture. The required quantity of the drug was separately dispersed in casting solvent. The two phases were mixed and PEG 400 was incorporated as a plasticizer. Propylene glycol was added as a permeation enhancer which was mixed homogeneously. A specific amount of sodium benzoate was added and uniformly mixed. The polymeric patches were cast in petri plates and the solvent was allowed to evaporate. An inverted funnel was placed on a petri plate to slow down the solvent evaporation rate and for avoiding dust accumulation. The arrangement was kept undisturbed for 24 h and then the dried films were carefully removed from the petri plates. The formed patches were cut to a uniform size, glued to the backing membrane, and stored in a desiccator wrapped in the wax paper sheet. The composition of developed transdermal patches was shown in Table 1.
2.2.3 Thickness
The patches were selected randomly from each batch and subjected to thickness measurement by using slide calipers. The thicknesses of the developed films were measured in three different areas, and the mean thickness was calculated for individual formulation batches.
2.2.4 Drug Content
A specific patch area (1 cm2) was taken and dissolved in 100 ml of pH 5.6 phosphate buffer solution and stirred for 6 h. Then the solution was kept undisturbed for up to 24 h for complete solubilization of glibenclamide. After 24 h, the solution was filtered and the filtrate was scanned by using a UV-Visible spectrophotometer [(UV-2540, SHIMADZU)] at 228 nm. The concentration of dissolved glibenclamide was determined for all the formulations using the following equation:
2.2.5 Folding Endurance
Folding endurance for the developed transdermal patches of glibenclamide was determined by repeated folding of film at the same point until it breaks. It helps to determine the efficacy of plasticizers and also to measure the strength of the developed patches. The number of folding was noted up to which the specific strip of film resisted breaking (Singh and Bali, 2016). The experiment was carried out in triplicate to obtain mean folding endurance for the respective formulation.
2.2.6 % Moisture Content
Randomly selected transdermal patches of specific surface area were weighed individually and placed inside desiccators containing activated silica beads at room temperature (Madan et al., 2015). The initial weight (Wi) of the patches was recorded. The films were weighed at regular intervals until a constant final weight (Wd) was obtained. The percentage of moisture content was calculated by using the following equation:
2.2.7 Moisture Uptake Capacity
The percentage of moisture uptake for the developed glibenclamide patches was determined by placing them initially in a desiccator for 24 h in presence of silica gel beads (Madan et al., 2015). Then the patches were removed, and the weight of individual patches was noted as initial weight (W0). The patches were then kept in another desiccator containing saturated sodium chloride to maintain high humidity conditions. The films were removed every 7 days at the interval and weighed until obtaining constant weight (WF). The increase in weight due to moisture uptake was determined and the percentage of moisture uptake was calculated by using the following formula:
2.2.8 In Vitro Membrane Permeation Study
In vitro membrane permeation study was carried out by using a Franz diffusion cell through a dialysis membrane (LA390, approximate capacity—1.99 ml/cm, average diameter—15.9 mm, average flat width—25.27 mm). The dialysis membrane was soaked overnight prior to use in dissolution fluid (phosphate buffer of pH 5.6). The transdermal films were cut according to the diameter of the donor cell and the dialysis membrane was placed over it and tied to one end. The donor cell was placed in such a way that the dissolution medium just touches the surface of the membrane covering the transdermal patch of glibenclamide. The stirring speed and the medium temperature were maintained at 50 rpm and 37 ± 0.5°C, respectively. 1 ml of aliquots was withdrawn at regular time intervals up to 12 h with an equal volume of fresh buffer replacement each time. The collected aliquots were filtered and spectrophotometrically analyzed for glibenclamide content at 228 nm by using a UV-visible spectrophotometer (UV-2540, SHIMADZU) (Jana et al., 2010).
2.2.9 Kinetic Analysis of Drug Release
The release behavior of glibenclamide from the developed transdermal polymer matrix can be predicted by fitting the in vitro drug release data into various mathematical models. Different mathematical models followed for this purpose are zero order, first order, Korsmeyer–Peppas, and Higuchi models (Korsmeyer et al., 1983; Peppas and Sahlin, 1989). The correlation coefficient obtained from each model has been noted from which the highest value indicates the best-fit mathematical model for drug release.
3 Results and Discussion
3.1 FTIR Study
The FTIR of glibenclamide, HPMC E50, Eudragit RS 100, and physical mixture of drug and polymers was shown in Figure 1. The FTIR spectrum of pure glibenclamide (Figure 1A) shows a peak for symmetric S=O stretching at 1144.80 cm−1 which was observed at the same frequency in the spectrum of physical mixture. Pure glibenclamide shows asymmetric S=O stretching for the–SO2NH group at 1343.48 cm−1 which was found in the physical mixture at exactly the same frequency. The characteristic peak for N–H stretching for–C=O–NH–was observed at 3372.68 cm−1. A similar peak for N–H stretching was observed in the physical mixture of (Figure 1D) at 3374.61 cm−1. The peak for N–H deformation and–C–O stretching vibration of–C–O–NH–was observed at 1720.58 cm−1 which persisted in the physical mixture without significant shift at 1707.08 cm−1. The peak for asymmetric S–O stretching was found at 1384.95 cm−1 which was observed at 1383.98 cm−1 in the physical mixture (Bakshi et al., 2015). The characteristic peaks appearing in the FTIR spectrum of glibenclamide have also appeared in the spectrum of physical mixtures of the drug without any significant shifting of peaks, indicating the absence of any chemical interaction during and after preparation.
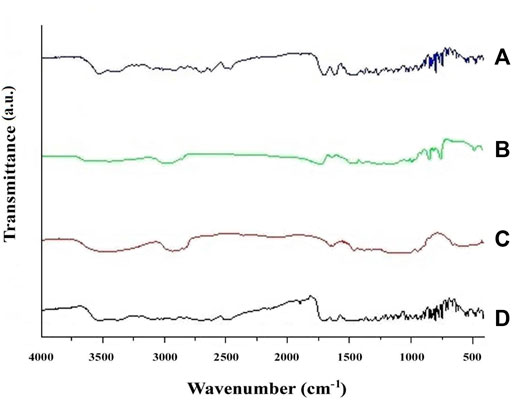
FIGURE 1. FTIR spectrum of (A) pure glibenclamide; (B) HPMC E50; (C) Eudragit RS 100; and (D) a physical mixture of glibenclamide with polymers.
3.2 Thickness of the Film
The thickness of glibenclamide-loaded transdermal films was measured with the help of a digital slide caliper at different points and the average thickness was calculated. Almost uniform thickness was observed for the developed patches of glibenclamide as shown in Table 2. The result indicated that there was not much variation in the thickness between the formulation and it was found to be within the range of 0.846 ± 0.020 mm–0.913 ± 0.020 mm without considerable variation similar to other study reports (Ofokansi et al., 2015).
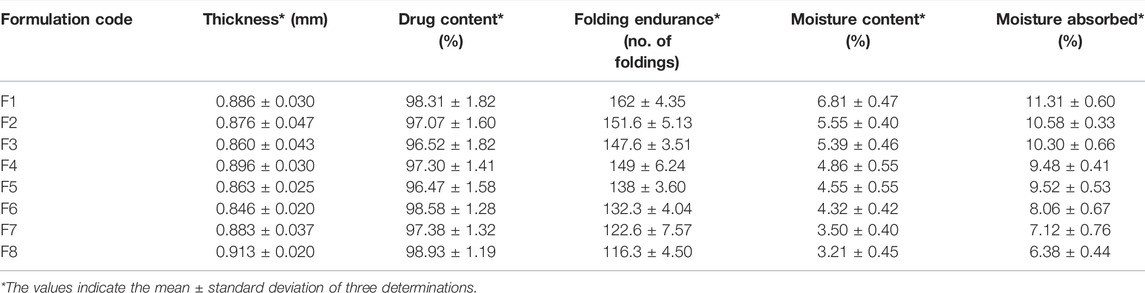
TABLE 2. Thickness, drug content, folding endurance, moisture content, and moisture absorbed for the developed patches.
3.3 Drug Content
The percentage of drug content was determined with respect to the incorporated amount of glibenclamide into the specific area (1 cm2) of the transdermal formulation. The drug content for all the formulations was observed between 96.47 ± 1.58% and 98.93 ± 1.19% (Table 2).
3.4 Folding Endurance
The folding endurance for the developed transdermal films was measured by repeated folding on either side of it. The matrix-based films were reported to have folding endurance within the range of 116.3 ± 4.50–162 ± 4.35 (Table 2). The obtained folding endurance values indicated good elasticity and strength of the designed transdermal patches. The values were roughly found to decrease with increasing Eudragit RS 100 concentration in the transdermal matrix.
3.5 % Moisture Content
The percentage of moisture content was determined in presence of activated silica beads. The % moisture content for all the transdermal films was determined within the range of 3.21 ± 0.45 to 6.81 ± 0.47 (Table 2). The obtained data clearly showed a gradual decrease in moisture content as the HPMC E50 concentration decreased with an increase in Eudragit RS 100 in the formulations. The minimum moisture content was observed for F8 as it contained a relatively lower concentration of HPMC E50 and a higher concentration of Eudragit RS 100 out of all the developed formulations. Similar results were observed in other matrix-type transdermal patches containing HPMC and Eudragit (Peddapalli et al., 2018).
3.6 Moisture Uptake Capacity
The moisture uptake ability was performed in a desiccator maintaining 75% relative humidity. A maximum of 11.31 ± 0.60 was observed for F1 whereas the least moisture content was found at 6.38 ± 0.44 for F8 (Table 2). The gradual decrease in % moisture uptake was observed due to the gradual lowering of hydrophilic HPMC E50. Minimum moisture uptake ability helps to reduce bulkiness, and also minimizes the chance of microbial contamination. Similar results were reported by Eudragit and HPMC-based transdermal films (Vijaya and Ruckmani 2011).
3.7 In Vitro Membrane Permeation of Glibenclamide
The release of glibenclamide from the developed transdermal films was performed in Franz diffusion cells through a dialysis membrane using pH 5.6 phosphate buffer as receptor medium. Figure 2 demonstrates the percentage of drug permeated through the dialysis membrane over 12 h of time. The membrane permeation of F1, showed complete permeation of glibenclamide after 10 h, whereas F2 exhibited complete release of drug after 11 h. For the remaining batches of formulation, the permeation study was continued for 12 h and the percentage of the permeated drug was found to vary between 76.15 ± 2.80% and 101.01 ± 0.33%. The data obtained clearly suggest the influence of polymer ratio in % of the permeated drug. An increase in Eudragit RS 100 concentration gradually reduces the water penetration into the developed patches, as a result, the release of glibenclamide decreases through the dialysis membrane. The simultaneous decrease in HPMC E50 concentration may also be responsible for retarding the release of glibenclamide. Being a hydrophilic polymer, HPMC E50 can easily uptake water and swelling occurs which facilitates a relatively rapid release of the drug. It has been observed that when Eudragit RS 100 concentration is gradually increased, leading to a reduction in water uptake, and the percentage of drug release is significantly reduced. A similar observation was reported by other HPMC E50 and Eudragit-based transdermal films (Yamsani et al., 2017).
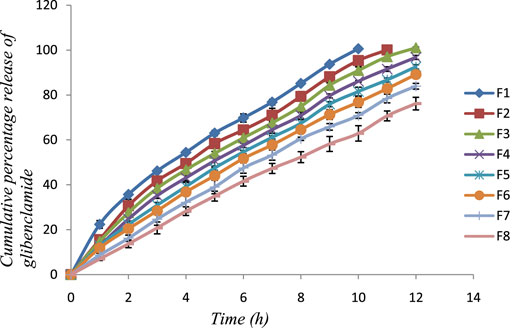
FIGURE 2. In vitro membrane permeation profile of glibenclamide from the developed patches (F1-F8) in phosphate buffer of pH 5.6.
3.8 Kinetic Analysis of Glibenclamide Release
The kinetic modeling of all the developed transdermal matrix films was performed. The data obtained from membrane permeation of glibenclamide incorporated patches were kinetically analyzed by zero order, first order, Higuchi, and Korsemeyer–Peppas models for explaining the release of the drug (Table 3). The release is best explained by zero order kinetic as (r2 > 0.977) for all the developed formulations except F1 followed by Higuchi and first order kinetics. The drug release kinetic data were analyzed by Korsemeyer–Peppas equation and the release exponent n from the developed formulations is found to vary from 0.64 to 0.96 indicating that the release mechanism is following non-Fickian diffusion and shifted gradually towards Super case II transport mechanism when Eudragit concentration is increased gradually. A similar observation was noticed in other Eudragit-based transdermal formulations (Chandak and Prasad Verma, 2010; Jana et al., 2014).
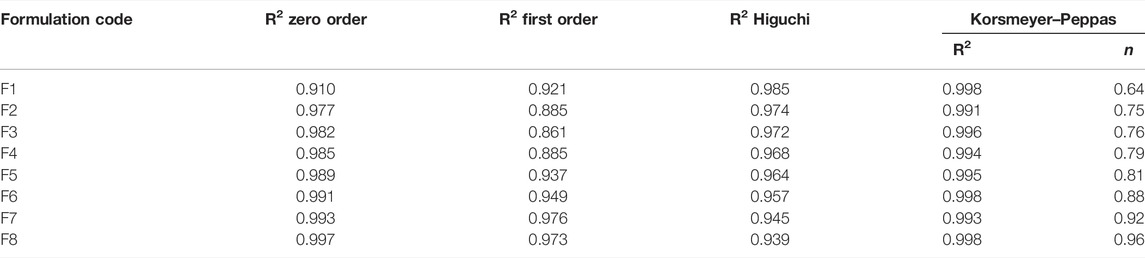
TABLE 3. Kinetic analysis of in vitro membrane permeation of glibenclamide-loaded transdermal patch.
4 Conclusion
The present investigation has demonstrated a transdermal approach to delivering glibenclamide through polymeric transdermal patches. The matrix-type transdermal patches were developed by following the solvent casting technique dissolving a hydrophilic and a hydrophobic polymer. HPMC E50 was selected as a hydrophilic matrix forming polymer to combine with a hydrophobic Eudragit RS 100. The FTIR study ensured no significant interaction between the drug and polymers. The thickness of the patches was almost uniform without significant variation. The % moisture content and moisture uptake capacity were found to depend on the polymeric ratio of transdermal films. The decrease in hydrophilic polymer concentration exhibited reduced moisture content and reduced moisture uptake ability. The membrane permeation study demonstrated a sustained release of glibenclamide over 12 h depending on polymeric composition. The decrease in hydrophilic polymer (HPMC E50) concentration reduces the % water uptake and retards the diffusion of drug molecules through the transdermal matrix. Among all the formulations, the least percentage of glibenclamide permeated was 76.15 ± 2.80% from F8 after 12 h. Finally, it is concluded that the developed transdermal patches for sustained delivery of glibenclamide can be a useful alternative in terms of avoiding the adverse effects associated with oral delivery of the drug.
Data Availability Statement
The original contributions presented in the study are included in the article/Supplementary Material; further inquiries can be directed to the corresponding authors.
Author Contributions
All authors listed have made a substantial, direct, and intellectual contribution to the work and approved it for publication.
Conflict of Interest
The authors declare that the research was conducted in the absence of any commercial or financial relationships that could be construed as a potential conflict of interest.
Publisher’s Note
All claims expressed in this article are solely those of the authors and do not necessarily represent those of their affiliated organizations, or those of the publisher, the editors, and the reviewers. Any product that may be evaluated in this article, or claim that may be made by its manufacturer, is not guaranteed or endorsed by the publisher.
References
Akar, F., Uydeş-Doğan, B. S., Buharalioğlu, C. K., Abban, G., Heinemann, A., Holzer, P., et al. (1999). Protective Effect of Cromakalim and Diazoxide, and Proulcerogenic Effect of Glibenclamide on Indomethacin-Induced Gastric Injury. Eur. J. Pharmacol. 374, 461–470. doi:10.1016/s0014-2999(99)00277-0
Al Hanbali, O. A., Khan, H. M. S., Sarfraz, M., Arafat, M., Ijaz, S., and Hameed, A. (2019). Transdermal Patches: Design and Current Approaches to Painless Drug Delivery. Acta Pharm. 69, 197–215. doi:10.2478/acph-2019-0016
Alkilani, A., McCrudden, M. T., and Donnelly, R. (2015). Transdermal Drug Delivery: Innovative Pharmaceutical Developments Based on Disruption of the Barrier Properties of the Stratum Corneum. Pharmaceutics 7, 438–470. doi:10.3390/pharmaceutics7040438
Bakshi, P., Sadhukhan, S., and Maiti, S. (2015). Design of Modified Xanthan Mini-Matrices for Monitoring Oral Discharge of Highly Soluble Soluplus -glibenclamide Dispersion. Mater. Sci. Eng. C 54, 169–175. doi:10.1016/j.msec.2015.05.014
Chandak, A. R., and Prasad Verma, P. R. (2010). Eudragit-based Transdermal Delivery System of Pentazocine: Physico-Chemical, In Vitro and In Vivo Evaluations. Pharm. Dev. Technol. 15, 296–304. doi:10.3109/10837450903188501
Chandrashekar, N. S., and Shobha Rani, R. H. (2008). Physicochemical and Pharmacokinetic Parameters in Drug Selection and Loading for Transdermal Drug Delivery. Indian J. Pharm. Sci. 70, 94–96. doi:10.4103/0250-474x.40340
Cherukuri, S., Batchu, U. R., Mandava, K., Cherukuri, V., and Ganapuram, K. R. (2017). Formulation and Evaluation of Transdermal Drug Delivery of Topiramate. Int. J. Pharm. Investig. 7, 10–17. doi:10.4103/jphi.JPHI_35_16
Davis, D. A., Martins, P. P., Zamloot, M. S., Kucera, S. A., Williams, R. O., Smyth, H. D. C., et al. (2020). Complex Drug Delivery Systems: Controlling Transdermal Permeation Rates with Multiple Active Pharmaceutical Ingredients. AAPS PharmSciTech 21, 165. doi:10.1208/s12249-020-01682-4
Davis, S. N., and Granner, D. K. (1996). “Insulin, Oral Hypoglycemic Agents, and the Pharmacology of the Endocrine Pancreas,” in Goodman and Gilman’s the Pharmacological Basis of Therapeutics. Editor A. G. Gilman (New York: McGraw-Hill), 1487–1518.
Ford, J. L. (2014). “Design and Evaluation of Hydroxypropyl Methylcellulose Matrix Tablets for Oral Controlled Release: A Historical Perspective,” in Hydrophilic Matrix Tablets for Oral Controlled Release. Editors P. Timmins, S. R. Pygall, and C. D. Melia (New York: Springer-Verlag), 17–51. doi:10.1007/978-1-4939-1519-4_2
Ghasemiyeh, P., and Mohammadi-Samani, S. (2021). Polymers Blending as Release Modulating Tool in Drug Delivery. Front. Mat. 8, 752813. doi:10.3389/fmats.2021.752813
Jafri, I., Shoaib, M. H., Yousuf, R. I., and Ali, F. R. (2019). Effect of Permeation Enhancers on In Vitro Release and Transdermal Delivery of Lamotrigine from EudragitRS100 Polymer Matrix-type Drug in Adhesive Patches. Prog. Biomater. 8, 91–100. doi:10.1007/s40204-019-0114-9
Jana, S., Lakshman, D., Sen, K. K., and Basu, S. K. (2010). Development and Evaluation of Epichlorohydrin Cross-Linked Mucoadhesive Patches of Tamarind Seed Polysaccharide for Buccal Application. Int. J. Pharm. Sci. Drug Res. 2, 193–198.
Jana, S., Manna, S., Nayak, A. K., Sen, K. K., and Basu, S. K. (2014). Carbopol Gel Containing Chitosan-Egg Albumin Nanoparticles for Transdermal Aceclofenac Delivery. Colloids Surfaces B Biointerfaces 114, 36–44. doi:10.1016/j.colsurfb.2013.09.045
Korsmeyer, R. W., Gurny, R., Doelker, E., Buri, P., and Peppas, N. A. (1983). Mechanisms of Solute Release from Porous Hydrophilic Polymers. Int. J. Pharm. 15, 25–35. doi:10.1016/0378-5173(83)90064-9
Li, L., Fang, L., Xu, X., Liu, Y., Sun, Y., and He, Z. (2010). Formulation and Biopharmaceutical Evaluation of a Transdermal Patch Containing Letrozole. Biopharm. Drug Dispos. 31, 138–149. doi:10.1002/bdd.698
Madan, J. R., Argade, N. S., and Dua, K. (2015). Formulation and Evaluation of Transdermal Patches of Donepezil. Recent Pat. Drug Deliv. Formul. 9, 95–103. doi:10.2174/1872211308666141028213615
Maiti, S., Mukherjee, S., and Datta, R. (2014). Core-shell Nano-Biomaterials for Controlled Oral Delivery and Pharmacodynamic Activity of Glibenclamide. Int. J. Biol. Macromol. 70, 20–25. doi:10.1016/j.ijbiomac.2014.06.031
Mašková, E., Kubová, K., Raimi-Abraham, B. T., Vllasaliu, D., Vohlídalová, E., Turánek, J., et al. (2020). Hypromellose - A Traditional Pharmaceutical Excipient with Modern Applications in Oral and Oromucosal Drug Delivery. J. Control. Rel. 324, 695–727. doi:10.1016/j.jconrel.2020.05.045
Murphy, M., and Carmichael, A. J. (2000). Transdermal Drug Delivery Systems and Skin Sensitivity Reactions. Incidence and Management. Am. J. Clin. Dermatology 1, 361–368. doi:10.2165/00128071-200001060-00004
Mutalik, S., and Udupa, N. (2004). Glibenclamide Transdermal Patches: Physicochemical, Pharmacodynamic, and Pharmacokinetic Evaluations. J. Pharm. Sci. 93, 1577–1594. doi:10.1002/jps.20058
Nayak, A. K., Das, B., and Maji, R. (2012). Calcium Alginate/gum Arabic Beads Containing Glibenclamide: Development and In Vitro Characterization. Int. J. Biol. Macromol. 51 (5), 1070–1078. doi:10.1016/j.ijbiomac.2012.08.021
Nokhodchi, A., Raja, S., Patel, P., and Asare-Addo, K. (2012). The Role of Oral Controlled Release Matrix Tablets in Drug Delivery Systems. BioImpacts 2, 175–187. doi:10.5681/bi.2012.027
Ofokansi, K. C., Kenechukwu, F. C., and Ogwu, N. N. (2015). Design of Novel Miconazole Nitrate Transdermal Films Based on Eudragit RS100 and HPMC Hybrids: Preparation, Physical Characterization, In Vitro and Ex Vivo Studies. Drug Deliv. 22 (8), 1078–1085. doi:10.3109/10717544.2013.875604
Peddapalli, H., Ganta, R. P., and Boggula, N. (2018). Formulation and Evaluation of Transdermal Patches for Antianxiety Drug. Asian J. Pharm. 12, 127–136. doi:10.22377/ajp.v12i02.2325
Peppas, N. A., and Sahlin, J. J. (1989). A Simple Equation for the Description of Solute Release. III. Coupling of Diffusion and Relaxation. Int. J. Pharm. 57, 169–172. doi:10.1016/0378-5173(89)90306-2
Raza, M. A., Jeong, J.-O., and Park, S. H. (2021). State-of-the-Art Irradiation Technology for Polymeric Hydrogel Fabrication and Application in Drug Release System. Front. Mat. 8, 769436. doi:10.3389/fmats.2021.769436
Reynolds, J. E. F. (1993). Martindale—The Extra Pharmacopoeia. 30th ed. London: The Pharmaceutical Press.
Rydberg, T., Jönsson, A., Røder, M., and Melander, A. (1994). Hypoglycemic Activity of Glyburide (Glibenclamide) Metabolites in Humans. Diabetes Care 17, 1026–1030. doi:10.2337/diacare.17.9.1026
Saoji, S. D., Atram, S. C., Dhore, P. W., Deole, P. S., Raut, N. A., and Dave, V. S. (2015). Influence of the Component Excipients on the Quality and Functionality of a Transdermal Film Formulation. AAPS PharmSciTech 16, 1344–1356. doi:10.1208/s12249-015-0322-0
Singh, A., and Bali, A. (2016). Formulation and Characterization of Transdermal Patches for Controlled Delivery of Duloxetine Hydrochloride. J. Anal. Sci. Technol. 7, 25. doi:10.1186/s40543-016-0105-6
Thakral, S., Thakral, N. K., and Majumdar, D. K. (2013). Eudragit: a Technology Evaluation. Expert Opin. Drug Deliv. 10, 131–149. doi:10.1517/17425247.2013.736962
Tran, T. T. D., and Tran, P. H. L. (2019). Controlled Release Film Forming Systems in Drug Delivery: The Potential for Efficient Drug Delivery. Pharmaceutics 11, 290. doi:10.3390/pharmaceutics11060290
Vijaya, R., and Ruckmani, K. (2011). In Vitro and In Vivo Characterization of the Transdermal Delivery of Sertraline Hydrochloride Films. Daru 19, 424–432.
Yamsani, V. V., Mudulaghar, M. K., Afreen, S., Wajid, S., Ravula, S. K., and Babelghaith, S. D. (2017). Formulation Design and In Vitro Ex Vivo Evaluation of Transdermal Patches of Cinnarizine. Pak. J. Pharm. Sci. 30, 2075–2083.
Keywords: transdermal patch, films, glibenclamide, HPMC E50, Eudragit RS 100
Citation: Manna S, Dhanalakshmi D, Bhowmik M, Jana S and Jana S (2022) Cellulose Derivative-Based Bioadhesive Blend Patch for Transdermal Drug Delivery. Front. Mater. 9:835507. doi: 10.3389/fmats.2022.835507
Received: 14 December 2021; Accepted: 16 May 2022;
Published: 22 June 2022.
Edited by:
Nafisa Gull, University of the Punjab, PakistanReviewed by:
Fatima Ali, University of Karachi, PakistanMuhammad Asim Raza, University of Science and Technology, South Korea
Copyright © 2022 Manna, Dhanalakshmi, Bhowmik, Jana and Jana. This is an open-access article distributed under the terms of the Creative Commons Attribution License (CC BY). The use, distribution or reproduction in other forums is permitted, provided the original author(s) and the copyright owner(s) are credited and that the original publication in this journal is cited, in accordance with accepted academic practice. No use, distribution or reproduction is permitted which does not comply with these terms.
*Correspondence: Sreejan Manna, bWFubmEuc3JlZWphbkBnbWFpbC5jb20=; Subrata Jana, amFuYS5zLm9jQGdtYWlsLmNvbQ==