- 1Department of Chemistry, University of Engineering and Technology, Lahore, Pakistan
- 2Institute of Polymer and Textile Engineering, University of the Punjab, Lahore, Pakistan
- 3Department of Chemistry, The University of Lahore, Lahore, Pakistan
- 4Electrical and Electronic Engineering Programme, Faculty of Engineering and Built Environment, Universiti Kebangsaan Malaysia, Bangi, Malaysia
Nanofibers mats of chitosan (CTS)/polyvinyl alcohol (PVA)/halloysite nanoclay and drug loaded CTS/PVA/halloysite nanoclay//3-glycidyoxypropyl trimethoxysilane were fabricated using the electrospinning method. Electrospun nanofiber samples were characterized by Fourier transform infrared spectroscopy (FTIR), scanning electron microscopy (SEM), and thermogravimetric analysis (TGA). FTIR confirmed the chemical and physical bonding among ingredients such as CTS, PVA, nanoclay and crosslinker in the nanofibers. SEM images showed the development of uniform nanofibers. The average nanofiber diameter was observed in the range of 50–200 nm. Antimicrobial activity was examined against E. coli and S. aurus bacteria. The results obtained indicated that all nanofiber samples showed significant antimicrobial activity against the Gram-positive as well as Gram-negative bacteria. TGA results indicated that the thermal stability of nanofibers increased with the addition of the crosslinker. The drug release was studied in phosphate buffer saline (PBS) solution (pH 7.4) at 37°C and was released from nanofibers in 2.5 h. Hence, these prepared nanofibers can be used in medication where the drug is required for a long duration.
1 Introduction
Nanotechnology has been utilized extensively with different compositions and biological properties in medicine for therapeutic drug delivery, tissue engineering applications, and the development of treatments for a variety of diseases and disorders. The high surface are a to volume ratio of nanomaterials can also be used in composites, chemical reactions, and energy storage applications (Suri et al., 2007; Shi et al., 2010; Safari and Zarnegar, 2014; Sutradhar and Amin, 2014). A fiber is an elongated, slender, and thread-like structure. The term “nanofiber” means that the fibers have a diameter in the nanometer range. These nanofibers are referred to as superfine, ultrafine, and submicron fibers (Adam et al., 2013). The electrospun nanofibers are very small in size, and they have many distinctive properties that allow them to be used in many small places. They also have a high surface area to volume ratio which significantly changes the physical, chemical, and morphological properties of the nanofiber mats (Mishra et al., 2019). The large surface area of nanofibers makes them appropriate for use in new technologies which need a smaller size and smaller environments to take place the chemical reactions. Chemical reactions speed up with increasing the surface area. Nanofibers can be produced by using several methods, such as self-assembly, phase separation technique, drawing method, electrospinning, and template synthesis. Electrospinning is a unique, easy, flexible, efficient, and cost-effective process for the fabrication of continuous nanofibers using a high potential electric field with a diameter range from 10 nm to several hundreds nanometers. Compared to other techniques, electrospinning is the most efficient method to produce nano or microscale nanofiber from a polymer solution using a high voltage electrical field that induces charges to the polymer solution. An electrically charged jet is expelled from the needle tip when the surface tension of the polymer solution is overcome by the electrostatic forces and nonwoven nanofibers mat are formed and collected on the collector (Yu et al., 2009; Sirin et al., 2013).
Biopolymers show good biocompatibility but poor mechanical properties and high production cost (Gull et al., 2019a). Natural polymers are highly sensitive to change in pH and temperature and lose their basic structure immediately. To overcome this drawback many strategies have been used (Rafique et al., 2016). Polymer blending is an attractive method that has been extensively used for providing new desirable characteristics (Gull et al., 2020a). Polymer blends are prepared by various methods including solution blending, which is very simple and rapid because it only requires simple equipment and does not involve any complicated process. This is mainly due to its simplicity, the availability of a wide range of synthetic and natural polymers for blending, and its effectiveness for practical utilization. The blending of polymers may result in the reduction of their basic cost, the improvement of their processing, and the maximization of their important properties. Polymer blends are physical mixtures of two or more structurally different homopolymers or copolymers and they interact through secondary forces with out covalent bonding (Mudigoudra et al., 2012).
Chitosan (CTS) is the most abundant natural polymer distributed widely in nature. The main source of the CTS and chitin are crabs shells, fungi, and shrimps shells. Its molecular weight (MW) varies in the ranges of 300–1,000 kDa depending on the chitin source (Mahdavi et al., 2013; Pokhrel et al., 2015). CTS has several properties which make it useful for many applications like drug delivery, wound healing, immunology, and hematology (Gull et al., 2020b). The MW and degree of deacetylation are the main factors that affect the properties of CTS. Reactions of CTS are more versatile because of the existence of -NH2 groups (Gull et al., 2019b). Nitrogen present in the CTS depends on the degree of deacetylation and mostly in the form of primary aliphatic amine which makes it more reactive (Dutta et al., 2004). It shows good antimicrobial activity and inhibits microbial growth. This antibacterial activity is due to the binding of cationic amino groups of CTS with the microorganisms’ anionic groups which inhibit the growth of microorganisms. In agriculture, CTS hinders bacterial growth and infection and stimulates the natural defenses and growth of plants (Ding et al., 2013).
Polyvinyl alcohol (PVA) is non-carcinogenic, biocompatible, biodegradable, nontoxic, and hydrophilic polymer. It has good film-forming properties, processing ability, and good chemical resistance (Fahmy et al., 2020; Fahmy et al., 2021). It is used in agriculture to deliver fertilizers, pesticides, herbicides, and fungicides, as well as to coat seeds. Such prepared seeds are ready for germination only under adequate temperature and humidity conditions (Reis et al., 2006; Haweel and Ammar, 2008; Ranjha and Khan, 2013; Gaaz et al., 2015; Swapna et al., 2015). 3-Glycidoxypropyl trimethoxysilane (3-GPTMS) is a colorless transparent liquid that is soluble in a variety of organic solvents, undergoes hydrolysis easily and condensation reaction to form polysiloxanes. It polymerizes easily in the presence of light, heat, and peroxides (Sapic et al., 2009).
Controlled drug delivery systems offer numerous advantages compared to conventional dosage forms including enhanced effectiveness, condensed toxicity, site-specificity to reduce the side effects of the drug, improved concentration maintenance, and controlled duration of drug release (Coelho et al., 2010; Baptista et al., 2013; Hu et al., 2014). Controlled targeted delivery of the drug is attained by utilizing the material, composed of either non-degradable or biodegradable polymer. The drug release is reliant on polymer nanofibers degradation. A broad range of polymer materials are used as drug delivery media or sources. The polymer medium for drug delivery is determined according to the requirements of the application. The type of polymer, solvent, and compatibility of the drug are important processing variables when trying to attain reproducible and stable drug delivery. Several drugs have been incorporated into electrospun nanofibers and transported to the desired target site in the body (Gholipour-Kanani and Bharami, 2010). The aim of this study was to prepare the novel electrospun nanofibers comprised of polymer blend which was crosslinked with silane crosslinker and used for controlled drug release system.
2 Materials and Methods
2.1 Materials
Chitosan (C8H13NO5)n was purchased from Biolog (GmbH) Trademark, Germany. Polyvinyl alcohol (C2H4O)n and formic acid were bought from Sigma-Aldrich. Potassium dihydrogen phosphate (KH2PO4), sodium dihydrogen phosphate (NaH2PO4), and sodium chloride (NaCl) were procured from Merck, Germany, and potassium chloride (KCl) was procured from Fisher chemicals. All chemicals were used without any further treatment.
2.2 Fabrication of Nanofibers
CTS (0.5 g) weighed on the electronic balance and dissolved in 1% formic acid solution with continuous stirring on a magnetic hot plate. PVA (3.5 g) was added to the pre-heated water at 90°C while creating a shear well and stirred until it completely dissolved. The PVA solution was added to the CTS solution and stirred for 1.5 h. Then, 100 µL 3-GPTMS was dissolved into methanol and added in the blended solution dropwise while creating a continuous shear well by stirring on a magnetic plate for 2 h, and then the blended solution was sonicated for a further 1 h. The blended solution was stirred again, and the solvent was evaporated till the solution becomes viscous for electrospinning. CPC-1 was a controlled nanofiber sample containing CTS and PVA without crosslinker. CPC-2 was a nanofiber sample containing 100 µL crosslinker (3-GPTMS). CPC-3 was a benzocaine-loaded sample.
3 Characterizations
3.1 Fourier Transformer Infrared Spectroscopy
Fourier Transformer Infrared Spectroscopy (FTIR) spectra of the nanofiber samples were analyzed by using Agilent technology. The FTIR of electrospun nanofibers were analyzed over the wavenumber range of 4,000–650 cm−1 at a resolution of 4 cm−1.
3.2 Thermogravimetric Analysis
Thermogravimetric characterization of electrospun nanofibers was carried out by using stare sw 12.10 model. The heating rate was kept at 10°C/min from room temperature to 600°C and nitrogen flow was maintained at (10 ml/min).
3.3 Scanning Electron Microscopy
The morphology and diameter of the electrospun nanofiber were analyzed using nano nova SEM 450. The SEM images results were obtained at different resolutions.
3.4 Antibacterial Activity
The antimicrobial activity of prepared nanofibers was determined using the disc diffusion method. A nutrient agar medium was prepared to grow the bacterial culture on a petri dish in the form of a slant. It was prepared by adding the respective amount of water and mixing it thoroughly. Then the agar containing vessels were heated in a water bath for 30–45 min to produce a transparent medium. After 45 min, the vessels were removed from the water bath and cooled. After maintaining the pH, the vessel was covered with aluminum foil and placed in an autoclave for sterilization at 15 psi and 121°C for 20 min. This semi-hot agar media was spread on petri dishes which were placed in an incubator at 37°C for 24 h. These petri dishes had clear agar medium, which means that there was no contamination. Nutrient broth medium was also prepared, and 10–15 ml autoclaved nutrient broth was poured into sterilized test tubes to be used for bacterial growth. A platinum loop was heated to red hot to sterilize it, then some strains were taken from pure and transferred to a test tube containing nutrient broth, covered with a sterilized cotton plug, and placed in an incubator for 24 h at 37°C. The prepared agar plates and bacteria-containing nutrient broth test tubes were kept in laminar flow. The petri dishes containing nutrient agar media were uncovered, and the nutrient broth was poured into each and spread across the whole petri dish. Samples were placed on the petri dish containing the bacterial culture. The petri dishes were placed in an incubator for 24 h at 37°C and then the zone of inhibition was measured with the help of a measuring scale in mm.
3.5 Drug Loading and Release Study
50 mg benzocaine was dissolved in ethanol and added to the CPC-2 blended solution. This solution was stirred for 1 h and then crosslinker was added dropwise and stirred for a further 2 h. The blended solution was sonicated for 1 h and then stirred and evaporated till the solution became viscous.
Electrospun nanofiber drug release study was carried out in phosphate buffer saline (PBS) solution. PBS solution was prepared by dissolving 8 g NaCl, 0.24 g KCL, 1.44 g NaH2PO4, and KH2PO4 in 800 ml distilled water. The pH of the solution was adjusted to 7.4 by the addition of a few drops of sodium chloride solution. Finally, distilled water was added up to mark.
CPC-2 loaded with a drug was placed in PBS solution separately at 37°C. After every 20 min, 5 ml solution was taken out from the beaker through a syringe and a fresh solution of PBS was added to keep the volume same. Samples were collected for 3 h from the PBS solution and the amount of released drug was determined using an ultraviolet-visible spectrophotometer at 294 nm for CPC-2. The amount of drug released from nanofibers was calculated with reference to a standard solution (100 ppm of benzocaine in PBS solution).
4 Result and Discussion
4.1 Fourier Transforms Infrared Spectroscopy
FTIR analysis was performed to confirm the possible physical and chemical interactions among CTS, PVA, nanoclay, and crosslinker in the nanofibers. FTIR spectra of all nanofiber samples are shown in Figure 1. A broad band was observed in the range of 3,380–3,190 cm–l in all samples due to the stretching vibration of the–OH group of PVA and the –NH 2 of CTS indicating the intensity of the hydrogen bonding. This broad band is due to the merging of -OH and -NH2 frequencies (Chaudhary et al., 2013a; Chaudhary et al., 2015; Chaudhary et al., 2019). The peak at 2,975–2,880 cm−1 was observed due to the vibrational frequency of -CH of alkyl groups. The sharp band at 1765–1,685 cm−1 is ascribed to the carbonyl group of acetylated groups of CTS. The band observed in the range of 1,120–990 cm−1 indicated the C-O-C group of CTS and siloxane linkage (–Si–O–C and–Si–O–Si) in the crosslinked nanofiber (Wang et al., 2016).
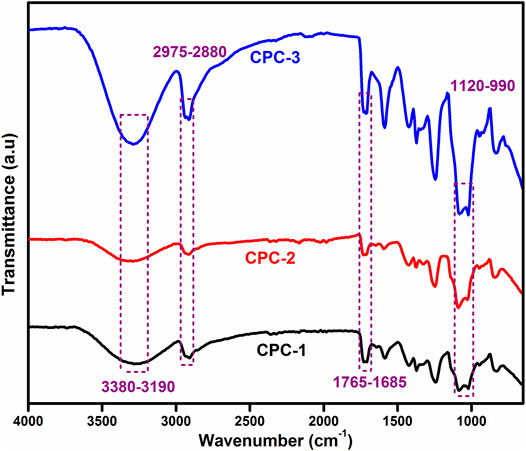
FIGURE 1. FTIR spectra of CTS and PVA blend CPC-1 and crosslinked blend solution CPC-2 and drug-loaded sample CPC-3.
4.2 Thermogravimetric Analysis
The thermal stability of nanofibers was studied by thermogravimetric analysis (TGA) and the results of the crosslinked nanofibers are shown in Figure 2, which shows the multistep degradation. The first thermal degradation step was observed from room temperature to 225°C, which is corresponded to the moisture evaporation of bound water and H-bonded water due to the highly absorption nature of CTS and PVA (Yeh et al., 2006; Chaudhary et al., 2013b; Society C.A.M., 2013). The next degradation step is due to the oxygen-containing pendent groups, depolymerization, oxidation, and the release of gases from organic molecules like CTS and PVA, and the next degradation was due to the pyrolysis of the main carbon chain. The widening of decomposition is due to the proper blending of PVA and CTS with the crosslinker which forms chemical and hydrogen bonds (Gull et al., 2019c). Table 1 shows the T50% and residue. T50% was 361°C for CPC-1 and 406°C for the crosslinked nanofiber which means that with crosslinking, the thermal stability of the nanofiber sample was increased and a high temperature was required to degrade the crosslinked sample.
4.3 Scanning Electron Microscopy
Figure 3 shows the SEM micrographs of uncrosslinked and crosslinked nanofibers and the drug-loaded sample. The surface morphology of the prepared nanofibers represents more crosslinking with an increase in the concentration of crosslinker (Potbhare et al., 2019). By crosslinking, and with all other parameters of the electrospinning kept constant, more uniform nanofibers were obtained and the average diameter of the nanofiber was in the range of 50–100 nm. CPC-1 showed a crosslinked structure which was due to the physical group of PVA and the crosslinking among all ingredients of nanofibers, but this crosslinking was more dense and compact in CPC-2 with more crosslinked mesh-like structure, due to the addition of crosslinker (Gull et al., 2020a).
4.4 Antimicrobial Analysis
The antimicrobial activity of the prepared nanofibers was determined using the disc diffusion method using Gram-negative bacteria, i.e., E. coli and Gram-positive bacteria i.e., S. aurus. The antibacterial activity of the samples was determined by measuring inhibition zones on a culture medium after 24 h of incubation. The resistance of bacterial growth was due to the cationic nature of CTS, which interacts with the anionic cell wall of bacteria. This opposite charge interaction caused the osmotic imbalance of cells and led to the cell lysis of bacteria. Another suggested mechanism is due to the interaction of CTS with bacterial DNA and penetrated bacterial nuclei, thus retarding the alteration of DNA into mRNA which eventually inhibited the growth of bacteria. Results have shown that the antimicrobial activity of the sample increased as compared to the controlled sample (Table 2).
4.5 In-vitro Release Study of Benzocaine
The release of benzocaine in CPC-2 was analyzed in PBS solution at 37°C in Figure 4. It was observed that the drug was released in 2.5 h which is according to the US Pharmacopeia standard. At neutral pH, CTS does not undergo protonation, which enables it to make physical interaction to develop a network which subsequently caused the controlled release of the drug from the nanofibers. From this result, it is concluded that such crosslinked nanofibers can be used for the controlled release of drugs in the biomedical field (Abbaspour et al., 2015).
4.5.1 Kinetic Study of Drug Release Analysis
To evaluate the transport mechanism of benzocaine from CPC-2, data were modeled by Ritger-Peppa’s model as shown in Eq. 1:
Mt is the amount of drug released at time t, M
5 Conclusion
In this study, electrospun nanofibers were prepared using a simple and cost-effective electrospinning technique. Nanofibers were prepared by using CTS, PVA, and halloysite nanoclay which is crosslinked with 3-GPTMS. Electrospun nanofibers were characterized by using FTIR, TGA, and SEM. FTIR analysis results confirmed the bonding between the polymer chains and siloxane linkage in nanofibers. SEM micrographs showed that the continuous and long nanofibers formed and with crosslinking more uniform nanofibers were obtained. The antimicrobial activity of the nanofiber was studied against E. coli and S. aureus and results indicated that all the samples showed significant antimicrobial behavior. TGA results indicated that the thermal strength of the nanofibers increased with crosslinking. A drug release study was conducted in PBS solution (pH 7.4) at 37°C using ultraviolet-visible spectroscopy and results showed that the drug was released completely in 2.5 h in a controlled manner. Hence, it is concluded that such drug-loaded nanofibers can be used in different clinical applications.
Data Availability Statement
The raw data supporting the conclusion of this article will be made available by the authors, without undue reservation.
Author Contributions
MN performed experimental work and physical analyses. SJ assisted in manuscript writing. NG contributed in writing, editing proof reading of manuscript. AI conceived and designed the project. AG supervised the project. MR and HA contributed in interpretation of results. AR performed antibacterial analysis. SK guided in data curation. RK guided in overall direction and planning of project.
Conflict of Interest
The authors declare that the research was conducted in the absence of any commercial or financial relationships that could be construed as a potential conflict of interest.
Publisher’s Note
All claims expressed in this article are solely those of the authors and do not necessarily represent those of their affiliated organizations, or those of the publisher, the editors and the reviewers. Any product that may be evaluated in this article, or claim that may be made by its manufacturer, is not guaranteed or endorsed by the publisher.
References
Abbaspour, M., Sharif Makhmalzadeh, B., Rezaee, B., Shoja, S., and Ahangari, Z. (2015). Evaluation of the Antimicrobial Effect of Chitosan/Polyvinyl Alcohol Electrospun Nanofibers Containing Mafenide Acetate. Jundishapur J. Microbiol. 8, e24239–6. doi:10.5812/jjm.24239
Adam, P., Sasikanth, K., Nama, S., Suresh, P., and Brahmaiah, B. (2013). Nanofiber - A New Trend in Nano Drug Delivery System. Int. J. Pharm. Res. Anal. 3, 47–55.
Baptista, A. C., Ferreira, I. F., Ferreira, I., and Borges, J. P. (2013). Electrospun Fibers in Composite Materials for Medical Applications. J. Compos. Biodegradable Polym. 1, 56–65. doi:10.12974/2311-8717.2013.01.01.7
Chaudhary, R. G., Ali, P., Gandhare, N. V., Tanna, J. A., and Juneja, H. D. (2019). Thermal Decomposition Kinetics of Some Transition Metal Coordination Polymers of Fumaroyl Bis (Paramethoxyphenylcarbamide) Using DTG/DTA Techniques. Arabian J. Chem. 12, 1070–1082. doi:10.1016/j.arabjc.2016.03.008
Chaudhary, R. G., Juneja, H. D., and Gandhare, N. V. (2013). Evaluation of Kinetic Parameters from TG/DTG Data of Chelate Polymer Compounds of Isophthaoylbis(paramethoxyphenylcarbamide). J. Chin. Adv. Mater. Soc. 1, 305–316. doi:10.1080/22243682.2013.871210
Chaudhary, R. G., Juneja, H. D., and Gharpure, M. P. (2013). Thermal Degradation Behaviour of Some Metal Chelate Polymer Compounds with Bis(bidentate) Ligand by TG/DTG/DTA. J. Therm. Anal. Calorimjournal Therm. Anal. Calorim. 112, 637–647. doi:10.1007/s10973-012-2616-8
Chaudhary, R. G., Juneja, H. D., Pagadala, R., Gandhare, N. V., and Gharpure, M. P. (2015). Synthesis, Characterisation and thermal Degradation Behaviour of Some Coordination Polymers by Using TG-DTG and DTA Techniques. J. Saudi Chem. Soc. 19, 442–453. doi:10.1016/j.jscs.2014.06.002
Coelho, J. F., Ferreira, P. C., Alves, P., Cordeiro, R., Fonseca, A. C., Góis, J. R., et al. (2010). Drug Delivery Systems: Advanced Technologies Potentially Applicable in Personalized Treatments. EPMA J. 1, 164–209. doi:10.1007/s13167-010-0001-x
Ding, F., Deng, H., Du, Y., Shi, X., and Wang, Q. (2013). Emerging Chitin and Chitosan Nanofibrous Materials for Biomedical Applications. Nanoscale 6, 1–47. doi:10.1039/C4NR02814G
Dutta, P. K., Dutta, J., and Tripathi, V. S. (2004). Chitin and Chitosan: Chemistry, Properties and Applications. J. Scientific Ind. Res. 63, 20–31.
Fahmy, A., Abu-Saied, M., Helaly, H., El-Dessoki, F., and Mohamed, T. A. (2021). Novel PVA/Methoxytrimethylsilane Elastic Composite Membranes: Preparation, Characterization and DFT Computation. J. Mol. Struct. 1235, 130173. doi:10.1016/j.molstruc.2021.130173
Fahmy, A., Mohamed, T. A., Abu-Saied, M., Helaly, H., and El-Dossoki, F. (2020). Structure/property Relationship of Polyvinyl Alcohol/dimethoxydimethylsilane Composite Membrane: Experimental and Theoretical Studies. Spectrochim Acta A. Mol. Biomol. Spectrosc. 228, 117810. doi:10.1016/j.saa.2019.117810
Gaaz, T., Sulong, A., Akhtar, M., Kadhum, A., Mohamad, A., and Al-Amiery, A. (2015). Properties and Applications of Polyvinyl Alcohol, Halloysite Nanotubes and Their Nanocomposites. Molecules 20, 22833–22847. doi:10.3390/molecules201219884
Gholipour-Kanani, A., and Bharami, H. (2010). Scaffold and Biomedical Application:Review on Electrospun Nanofibers Scaffold and Biomedical Applications. Trends Biomater. Artif. organs 24, 93–115.
Gull, N., Khan, S. M., Butt, M. T. Z., Zia, S., Khalid, S., Islam, A., et al. (2019). Hybrid Cross‐linked Hydrogels as a Technology Platform for In Vitro Release of Cephradine. Polym. Adv. Technol. 30, 2414–2424. doi:10.1002/pat.4688
Gull, N., Khan, S. M., Butt, O. M., Islam, A., Shah, A., Jabeen, S., et al. (2020). Inflammation Targeted Chitosan-Based Hydrogel for Controlled Release of Diclofenac Sodium. Int. J. Biol. Macromolecules 162, 175–187. doi:10.1016/j.ijbiomac.2020.06.133
Gull, N., Khan, S. M., Islam, A., and Butt, M. T. Z. (2019). “Hydrogels Used for Biomedical Applications,” in Bio Monomers for Green Polymeric Composites Materials. Editors O. B. P.M. Visakh, and G Menon (Hoboken: Wiley-blackwell), 175–199. doi:10.1002/9781119301714.ch9
Gull, N., Khan, S. M., Khalid, S., Zia, S., Islam, A., Sabir, A., et al. (2020). Designing of Biocompatible and Biodegradable Chitosan Based Crosslinked Hydrogel for In Vitro Release of Encapsulated Povidone-Iodine: A Clinical Translation. Int. J. Biol. Macromolecules 164, 4370–4380. doi:10.1016/j.ijbiomac.2020.09.031
Gull, N., Khan, S. M., Zahid Butt, M. T., Khalid, S., Shafiq, M., Islam, A., et al. (2019). In Vitro study of Chitosan-Based Multi-Responsive Hydrogels as Drug Release Vehicles: A Preclinical Study. RSC Adv. 9, 31078–31091. doi:10.1039/c9ra05025f
Haweel, C. K., and Ammar, S. H. (2008). Preparation of Polyvinyl Alcohol from Local Raw Material. Iraqi J. Chem. Pet. Eng. 9, 15–21.
Hu, J., Zeng, F., Wei, J., Chen, Y., and Chen, Y. (2014). Novel Controlled Drug Delivery System for Multiple Drugs Based on Electrospun Nanofibers Containing Nanomicelles. J. Biomater. Sci. Polym. Edition 25, 257–268. doi:10.1080/09205063.2013.852367
Huang, Y., Yu, H., and Xiao, C. (2007). pH-Sensitive Cationic Guar Gum/poly (Acrylic Acid) Polyelectrolyte Hydrogels: Swelling and In Vitro Drug Release. Carbohydr. Polym. 69, 774–783. doi:10.1016/j.carbpol.2007.02.016
Mahdavi, H., Mirzadeh, H., Zohuriaan, J., and Talebnezhad, F. (2013). Poly (Vinyl Alcohol)/chitosan/clay Nano-Composite Films. J. Am. Sci. 9, 203–214.
Mishra, R. K., Mishra, P., Verma, K., Mondal, A., Chaudhary, R. G., Abolhasani, M. M., et al. (2019). Electrospinning Production of Nanofibrous Membranes. Environ. Chem. Lett. 17, 767–800. doi:10.1007/s10311-018-00838-w
Mudigoudra, B. S., Masti, S. P., and Chougale, R. B. (2012). Thermal Behavior of Poly (Vinyl Alcohol)/Poly (Vinyl pyrrolidone)/Chitosan Ternary Polymer Blend Films. Res. J. Recent Sci. 1, 83–86.
Pokhrel, S., Yadav, P. N., and Adhikari, R. (2015). Applications of Chitin and Chitosan in Industry and MedicalScience: A Review. Nepal J. Sci. Tech. 16, 99–104. doi:10.3126/njst.v16i1.14363
Potbhare, A. K., Chaudhary, R. G., Chouke, P. B., Yerpude, S., Mondal, A., Sonkusare, V. N., et al. (2019). Phytosynthesis of Nearly Monodisperse CuO Nanospheres Using Phyllanthus Reticulatus/Conyza Bonariensis and its Antioxidant/antibacterial Assays. Mater. Sci. Eng. C 99, 783–793. doi:10.1016/j.msec.2019.02.010
Rafique, A., Mahmood Zia, K., Zuber, M., Tabasum, S., and Rehman, S. (2016). Chitosan Functionalized Poly(vinyl Alcohol) for Prospects Biomedical and Industrial Applications: A Review. Int. J. Biol. Macromolecules 87, 141–154. doi:10.1016/j.ijbiomac.2016.02.035
Ranjha, N. M., and Khan, S. (2013). Chitosan/Poly (Vinyl Alcohol) Based Hydrogels for Biomedical Applications: A Review. J. Pharm. Altern. Med. 2, 30–41.
Reis, E. F. D., Campos, F. S., Lage, A. P., Leite, R. C., Heneine, L. G., Vasconcelos, W. L., et al. (2006). Synthesis and Characterization of Poly (Vinyl Alcohol) Hydrogels and Hybrids for rMPB70 Protein Adsorption. Mat. Res. 9, 185–191. doi:10.1590/s1516-14392006000200014
Safari, J., and Zarnegar, Z. (2014). Advanced Drug Delivery Systems: Nanotechnology of Health Design A Review. J. Saudi Chem. Soc. 18, 85–99. doi:10.1016/j.jscs.2012.12.009
Sapic, I. M., Bistricić, L., Volovsek, V., Dananic, V., and Furic, K. (2009). DFT Study of Molecular Structure and Vibrations of 3-glycidoxypropyltrimethoxysilane. Spectrochimica Acta A: Mol. Biomol. Spectrosc. 72, 833–840. doi:10.1016/j.saa.2008.11.032
Shi, J., Votruba, A. R., Farokhzad, O. C., and Langer, R. (2010). Nanotechnology in Drug Delivery and Tissue Engineering: From Discovery to Applications. Nano Lett. 10, 3223–3230. doi:10.1021/nl102184c
Sirin, S., Cetiner, S., and Sarac, A. S. (2013). Polymer Nanofibers via Electrospinning: Factors Affecting Nanofiber Quality. J. Eng. Sci. 16, 1–12.
Society C.A.M. (2013). Journal of the Chinese Advanced Materials Society. Milton Park: Taylor & Francis Group.
Suri, S. S., Fenniri, H., and Singh, B. (2007). Nanotechnology-based Drug Delivery Systems. J. Occup. Med. Toxicol. 2, 1–16. doi:10.1186/1745-6673-2-16
Sutradhar, K. B., and Amin, M. L. (2014). Nanotechnology in Cancer Drug Delivery and Selective Targeting. ISRN Nanotechnology 2014, 1–12. doi:10.1155/2014/939378
Swapna, V. P., Selvin Thomas, P., Suresh, K. I., Saranya, V., Rahana, M. P., and Stephen, R. (2015). Thermal Properties of Polyvinyl Alcohol (PVA)/Halloysite Nanotubes Reinforced Nanocomposites. Int. J. Plast. Tech. 19, 124–136. doi:10.1007/s12588-015-9106-3
Wang, D., Liu, W., Feng, Q., Dong, C., Liu, Q., Duana, L., et al. (2016). Effect of Inorganic/organic Ratio and Chemical Coupling on the Performance of Porous Silica/chitosan Hybrid Scaffolds. Mater. Sci. Eng. C 70, 1–7. doi:10.1016/j.msec2016.04.010
Yeh, J.-T., Chen, C.-L., Huang, K. S., Nien, Y. H., Chen, J. L., and Huang, P. Z. (2006). Synthesis, Characterization, and Application of PVP/chitosan Blended Polymers. J. Appl. Polym. Sci. 101, 885–891. doi:10.1002/app.23517
Keywords: chitoan, controlled release, crosslinked, nanofiber, silane
Citation: Naz M, Jabeen S, Gull N, Ghaffar A, Islam A, Rizwan M, Abdullah H, Rasool A, Khan S and Khan R (2022) Novel Silane Crosslinked Chitosan Based Electrospun Nanofiber for Controlled Release of Benzocaine. Front. Mater. 9:826251. doi: 10.3389/fmats.2022.826251
Received: 30 November 2021; Accepted: 18 January 2022;
Published: 07 April 2022.
Edited by:
Wenbo Wang, Inner Mongolia University, ChinaReviewed by:
Alaa Fahmy, Al-Azhar University, EgyptRatiram Gomaji Chaudhary, Seth Kesarimal Porwal College, India
Copyright © 2022 Naz, Jabeen, Gull, Ghaffar, Islam, Rizwan, Abdullah, Rasool, Khan and Khan. This is an open-access article distributed under the terms of the Creative Commons Attribution License (CC BY). The use, distribution or reproduction in other forums is permitted, provided the original author(s) and the copyright owner(s) are credited and that the original publication in this journal is cited, in accordance with accepted academic practice. No use, distribution or reproduction is permitted which does not comply with these terms.
*Correspondence: Atif Islam, ZHJhdGlmaXNsYW1AZ21haWwuY29t