- 1Affiliated Stomatology Hospital of Guangzhou Medical University, Guangdong Engineering Research Center of Oral Restoration and Reconstruction, Guangzhou Key Laboratory of Basic and Applied Research of Oral Regenerative Medicine, Guangzhou, China
- 2School of Mechanical, Medical, and Process Engineering, Queensland University of Technology (QUT), Brisbane, QLD, Australia
- 3Centre for Biomedical Technologies, Queensland University of Technology (QUT), Brisbane, QLD, Australia
- 4The Australia-China Centre for Tissue Engineering and Regenerative Medicine (ACCTERM), Westmead, NSW, Australia
- 5NICM Health Research Institute, Western Sydney University, Westmead, NSW, Australia
It is presently difficult to achieve dental pulp vitality maintenance and regeneration in adult teeth. Dentin destruction results in the exposure of the dental pulp tissue to infectious oral environments, thereby triggering continuous severe pulp inflammation that impedes the self-regenerative capacity of the pulp. For these reasons, the regeneration of dentin bridges to block pulp tissue from the oral environment is an indispensable step. Nevertheless, this goal is difficult to achieve using present strategies, because the importance of immunoregulation in the pulp inflammatory microenvironment has been ignored. In our previous study, we found that the nanomaterial dihydrolipoic acid-functionalized gold nanoclusters (DHLA-AuNCs) efficiently regulated inflammatory responses in microglia (resident macrophages in the central nervous system), suggesting that DHLA-AuNCs may induce dentin bridge regeneration by regulating dental pulp macrophage responses. In the present study, we found that DHLA-AuNCs inhibited the M1 phenotype while promoting the M2 phenotype in macrophages in inflammatory conditions in vitro. This regulation of the inflammatory environment in dental pulp enhanced the differentiation of human dental pulp stromal cells (hDPC) toward odontoblasts, a beneficial effect on dentin regeneration. DHLA-AuNCs also had a direct role in the differentiation and mineralization of hDPC. These findings suggest that DHLA-AuNCs facilitate dentin regeneration through both efficient immunomodulation and direct induction of stromal cell differentiation/mineralization, providing a potential therapeutic nanomaterial for dentin bridge regeneration, effects that would be beneficial for dental pulp regeneration.
Introduction
Irreversible pulpitis is one of the most common oral diseases. The long-term harmful effects of dental caries, periodontal inflammation, and injury result in dental pulp infection, inflammation, and finally, dentin degeneration that eventually causes pulp necrosis and apical periodontitis (Colombo et al., 2014). Maintenance of vitality and regeneration in dental pulp are challenging issues for modern dentistry (Colombo et al., 2014; Xu et al., 2019); for these reasons, the present treatment of irreversible pulpitis is root canal treatment (RCT). However, this treatment requires the removal of the whole dental pulp tissue, which results in permanently devitalized teeth lacking vascularization and therefore, nutrition exchange; this eventually leads to a fragile tooth that often fractures, a common complication of RCT (Colombo et al., 2014). For these reasons, it is desirable to provide better options for dental therapy, especially for the treatment of irreversible pulpitis (Colombo et al., 2014). Recent advances in material science have demonstrated the potential of using materials for hard tissue regeneration (Wang et al., 2016; Bottino et al., 2017; Hu et al., 2017), suggesting that novel biomaterials can be developed for dental applications, especially for dentin regeneration (Colombo et al., 2014).
Located in the central part, dental pulp tissue constitutes the main structural component of teeth (Harris and Griffin, 1969; Lowder and Mueller, 1998; Colombo et al., 2014). The dental pulp consists of collagen-based extracellular matrixes and cell populations such as odontoblasts, mesenchymal progenitor cells (dental pulp stem cells and stromal cells), fibroblasts, immune cells, vascular cells, and neurons (Miura et al., 2003; Sloan and Smith, 2007; Sloan and Waddington, 2009; Balic et al., 2010; Casagrande et al., 2011; Iwasaki et al., 2011; Schmalz and Galler, 2011; Colombo et al., 2014). The pulp blood vessel network connects pulp tissue with the circulatory system, thereby providing nutrition and waste exchange for pulp cells such as odontoblasts, which are columnar-like cells lining the pulp chamber. Odontoblasts contain long cytoplasm tubule-like structures named dentinal tubules that run through the dentin (Harris and Griffin, 1969; Sigal et al., 1984; Colombo et al., 2014). Odontoblasts are responsible for the maintenance of dentin mineralization, which also induces the formation of sclerotic dentin in response to dentinal tubule infections (Tziafas et al., 2000; Harichane et al., 2011; Colombo et al., 2014). Nevertheless, more severe infections due to progressive carious lesions can trigger strong inflammatory responses of the pulp immune cells, eventually resulting in odontoblast death and dentin degeneration (Colombo et al., 2014). The degeneration of the dentin matrix then triggers the recruitment and activation of pulp progenitor/stromal cells that could potentially differentiate into odontoblasts for dentin regeneration (Miura et al., 2003; Sloan and Waddington, 2009; Waddington et al., 2009; Balic et al., 2010; Lin and Rosenberg, 2011). In addition to odontoblasts, pulp progenitor cells have shown multipotent differentiation capacities and can differentiate into osteoblasts, adipocytes, chondrocytes, and vascular cells (Waddington et al., 2009; d'Aquino et al., 2007; Iohara et al., 2008). This suggests that dental pulp regeneration is theoretically feasible (Colombo et al., 2014), evidenced by the initial regeneration of a dentin bridge (Slutzky-Goldberg et al., 2009; Kermanshahi et al., 2010; Başaran et al., 2012), an indispensable part that blocks and secures the pulp tissue from bacteria in the oral environment. In this manner, dentin regeneration may be considered a critical approach to dental pulp viability maintenance and regeneration.
Previous studies identified the critical role of immunomodulation in tissue regeneration (Chen et al., 2016), especially in the healing of hard tissue (such as bone). The biomaterial-induced regulation of macrophage inflammatory responses has been found to facilitate bone regeneration (Chen et al., 2016). The polarization of macrophages (among the major cell types in the innate immune system) plays a key role in dental pulp infection and inflammation (Izumi et al., 1995; Iwasaki et al., 2011; Colombo et al., 2014). In our recent study (Xiao et al., 2020), we found that dihydrolipoic acid-functionalized gold nanoclusters (DHLA-AuNCs) (Zhang et al., 2013; Li et al., 2014; Zhang and Wang, 2014; Shahsavari and Behroozi, 2016; Zhao et al., 2016; Zheng et al., 2017) efficiently regulated inflammatory responses. DHLA-AuNCs (referred to as AuNCs herein) directed the phenotype switch from M1 toward M2, creating an immune microenvironment beneficial for tissue regeneration. We speculated that AuNCs might exhibit regulatory effects on macrophages to facilitate dentin regeneration. Therefore, in the present study, we tested the regulatory role of AuNCs on macrophage inflammatory responses and investigated the effect of AuNCs on odontoblast differentiation and mineralization, thereby evaluating the potential effect of AuNCs on dentin regeneration.
Materials and Methods
Cell Culture
To study the effects of AuNCs on the macrophage inflammatory response, we used the murine macrophage cell line RAW 264.7 (RAW). RAW cells were grown in Dulbecco’s Modified Eagle’s Medium (DMEM; Gibco TM, Thermo Fisher Scientific, Waltham, MA, United States) containing 5% (v/v) fetal bovine serum (FBS, heat-inactivated at 60°C for more than 30 min, Lonza, Basel, Switzerland) and 1% (v/v) penicillin/streptomycin (P/S, Gibco TM, Thermo Fisher Scientific). The culture medium was changed every 2 days. After reaching 80% confluence, the cells were passaged by treating them with 0.25% trypsin (containing 1 mM EDTA) for 2 min.
Human dental pulp stromal cells (hDPC) were isolated from six healthy adult teeth harvested from six patients (18–30 years old) who were undergoing standard third molar extractions or premolar extractions for orthodontic purposes. All experimental procedures conformed to the Guiding Principles of the Ethics Committee of the Queensland University of Technology, Australia (ethics approval number: 1900000941). All patients provided informed written consent.
Dental pulp tissue was harvested under sterile conditions. Briefly, the extracted teeth were washed with phosphate-buffered saline (PBS) containing 1% P/S, then dental fissure burrs were used to cut the teeth open at the cementum–enamel junction to expose the pulp chamber (Cao et al., 2015). The pulp tissue was gently separated from the crown and root and then cut into small pieces. The pulp tissue fragments were resuspended in DMEM containing 10% FBS and 1% P/S, then transferred into a culture flask and cultured in incubators with 5% CO2 at 37°C. At 3 days, the non-attached cells/tissue were washed away using PBS, and the attached cells were cultured with DMEM containing 10% FBS and 1% P/S. The culture medium was changed every 2–3 days. The cells (80% confluence) were passaged by treating with 0.25% trypsin (containing 1 mM EDTA, Gibco TM, Thermo Fisher Scientific) for 2 min. The cells were used for experiments within five passages.
MTT Assay
MTT assays were performed to evaluate the effects of AuNCs on the proliferation of hDPC and RAW cells as previously described (Wu and Chang, 2012). Briefly, hDPC were seeded into 96-well plates (2,000 cells/well, 4 wells/group) and treated with AuNCs at graded concentrations: 0 (vehicle control), 0.5, 1, 5, 10, or 50 μg/ml, which were supplemented in DMEM with 10% FBS and 1% P/S. After 1, 2, 3, or 5 days, the culture medium was removed and the cells were washed with PBS. Similarly, the RAW cells were seeded into 96-well plates at 2,000 cells/well (4 wells/group) and treated with AuNCs at graded concentrations: 0 (vehicle control), 0.1, 1, 5, 10, or 30 μg/ml supplemented in DMEM with 10% FBS and 1% P/S for 24 h and then washed with PBS. Then, 100 µL fresh culture medium (DMEM with 10% FBS) containing MTT solution (0.5 mg/ml, Sigma-Aldrich Pty., Ltd., Sydney, Australia) was added to each well and the plates were incubated at 37°C for 4 h. After the medium removal, the deposits were dissolved in 100 µL dimethyl sulfoxide in each well to solubilize the formazan product, and the plates were read at 570 nm using a SpectraMax, Plus 384 plate reader (Molecular Devices, LLC, San Jose, CA, United States). All experiments were repeated three times.
Stimulation of Macrophage Inflammatory Response
To mimic macrophage inflammatory responses in vitro, the RAW cells were treated with IFN-γ (100 ng/ml) and LPS (100 ng/ml) for 24 h, as previously described (Huang et al., 2017). To investigate the effect of AuNCs on macrophage inflammatory responses, the RAW cells were treated with graded doses of AuNCs during IFN-γ and LPS stimulation: 0 μg/ml (vehicle control), 0.5 μg/ml (low dose), and 5 μg/ml (high dose). Then, the culture medium was removed, the cells were washed twice with PBS, and then subjected to serum-free DMEM for another 12 h to harvest the conditioned medium (CM). The CM was centrifuged (1,000 × g, 10 min, 4°C) and then filtered using a 0.45-µm filter (Millipore Corporation, Billerica, MA, United States) to remove cell debris. The filtered CM was stored at –80°C for further experiments. The RAW cells were harvested for RNA isolation and protein analysis.
Mineralization of Human Dental Pulp Stromal Cells
To examine the effect of AuNCs on the mineralization of hDPC in vitro, the cells were treated with 10 mM β-glycerophosphate, 50 μM ascorbic acid, and 100 nM dexamethasone (in DMEM with 10% FBS and 1% P/S) with AuNCs at graded concentrations: 0 μg/ml (vehicle control), 0.5 μg/ml (low dose), and 5 μg/ml (high dose), to induce odontoblast-like differentiation as previously described (Athirasala et al., 2018). The cells were harvested on 3, 7, and 14 days of treatment for RNA isolation, protein analysis, and Alizarin red S staining.
To evaluate the effect of AuNC-treated macrophages on the osteogenic differentiation of hDPC in vitro, the harvested RAW cell-CM (as mentioned previously) was mixed with 2× osteogenic medium (DMEM, 20% FBS, 2% P/S, 20 mM β-glycerophosphate, 100 μM ascorbic acid, and 200 nM dexamethasone) at a ratio of 1:1 and the mixture was used to treat hDPC for osteogenic differentiation for 3, 7, or 14 days. The cells were subsequently harvested for RNA isolation, protein analysis, and Alizarin red S staining.
RNA Extraction, cDNA Synthesis, and Real-Time Quantitative-PCR
The Total RNA was extracted from the RAW cells (24 h of stimulation) or hDPC (3/7 days of differentiation) using TRIzol reagent (Ambion®, Thermo Fisher Scientific). We used 1 μg total RNA for cDNA synthesis using the SensiFAST™ cDNA Synthesis Kit (Bioline Reagents, Meridian Bioscience Inc., Cincinnati, OH, United States) following the manufacturer’s protocol. A real-time polymerase chain reaction (qRT-PCR) was performed to measure the mRNA levels of the following target genes: CD11c, CD86, iNOS, IL-6, MHC-Ⅱ, TNF, BMP2, BMP6, IL-10, Wnt3a, and Wnt5a (in RAW cells) and BSP, Runx2, OCN, OPN, DMP-1, and DSPP (in hDPC). Primer sequences are listed in Table 1 in the Supporting Information. Relative gene expression was normalized against Actb and Gapdh, calculated as previously described (Bookout and Mangelsdorf, 2003). All experiments followed MIQE guidelines (Bustin et al., 2009) and were repeated three times.
Protein Extraction and Western Blotting
The total protein was extracted from hDPC (3 or 7 days of differentiation) using a lysis buffer (20 mM HEPES (pH 7.4), 10% glycerol, 1% Triton X-100, and 2 mM EDTA) containing the cOmpleteTM protease inhibitor cocktail (Roche, Dee Why, NSW, Australia). The BCA Protein Assay Kit (Thermo Fisher Scientific) was used to determine the protein concentrations of the extracts following the manufacturer’s protocol. Western blotting was performed as previously described (Xiao et al., 2020). Briefly, each sample (20 µg of protein) was loaded onto SDS-PAGE gels (10–15%) and then separated and transferred onto nitrocellulose membranes (Merck Millipore, Billerica, MA, United States). After 1 h of blocking with the Odyssey Blocking Buffer (LI-COR Biosciences, Lincoln, NE, United States) at room temperature, the membranes were subjected to incubation with primary antibodies at 4°C overnight. The primary antibodies (all from rabbits) were as follows: ALP (1:1,000, Abcam, Cambridge, United Kingdom), osterix (1:1,000, Abcam), β-catenin (1:1,000, Cell Signaling Technology, Danvers, MA, United States), iNOS (1:250, Abcam), arginase (1:500, Cell Signaling Technology), and α-tubulin (1:2000, Abcam, loading control). After three washes with Tris-buffered saline (TBS) supplemented with 0.1% Tween 20 (TBST), the membranes were incubated with an anti-rabbit IgG IRDye 800-conjugated secondary antibody (1:10,000, Rockland Immunochemicals, Limerick, PA, United States) for 1 h at room temperature. After washing with TBS, the membranes were scanned using the Odyssey® Infrared Imaging System and Image Studio software (LI-COR Biosciences) according to the manufacturer’s instructions. The Odyssey Blocking Buffer was used for antibody dilution. All procedures were repeated three times.
Alizarin Red S Staining
After 14 days of osteogenic differentiation, the hDPC were fixed with 4% paraformaldehyde for 10 min at room temperature. After washing with ultra-pure water, the cells were then stained with 1% Alizarin red S solution (pH: 4.1–4.3, Sigma Aldrich Pty., Ltd.) for 20 min at room temperature and washed again with ultra-pure water. Cells without osteogenic differentiation were also stained with Alizarin red S and served as negative controls. The samples were observed and imaged with a stereoscopic microscope. Quantification of positive-stained areas was performed using the ImageJ software. All procedures were repeated three times.
Statistical Analysis
For comparison between multiple groups, data were subjected to a statistical analysis using the one-way ANOVA followed by the Student–Newman–Keul test at α = 0.05. p < 0.05 was considered to indicate statistically significant differences. The data were analyzed using Prism 7.0 (GraphPad software, San Diego, CA, United States).
Results
Effect of Gold Nanoclusters on Cell Proliferation
To examine the cytotoxic effects of AuNCs, the MTT assay was performed to test metabolic changes in RAW cells and hDPC treated with graded doses of AuNCs. As shown in Figure 1A, RAW cells showed no metabolic changes (as compared with the blank control) when treated with ≤5 μg/ml for 24 h. For hDPC (Figures 1B–F), AuNCs inhibited the cell metabolism from day 3 to day 5 in a dose-dependent manner, while doses lower than 0.5 μg/ml did not show significant differences as compared with the blank control group. We then chose a low dose (0.5 μg/ml) and a high dose (5 μg/ml) for subsequent experiments.
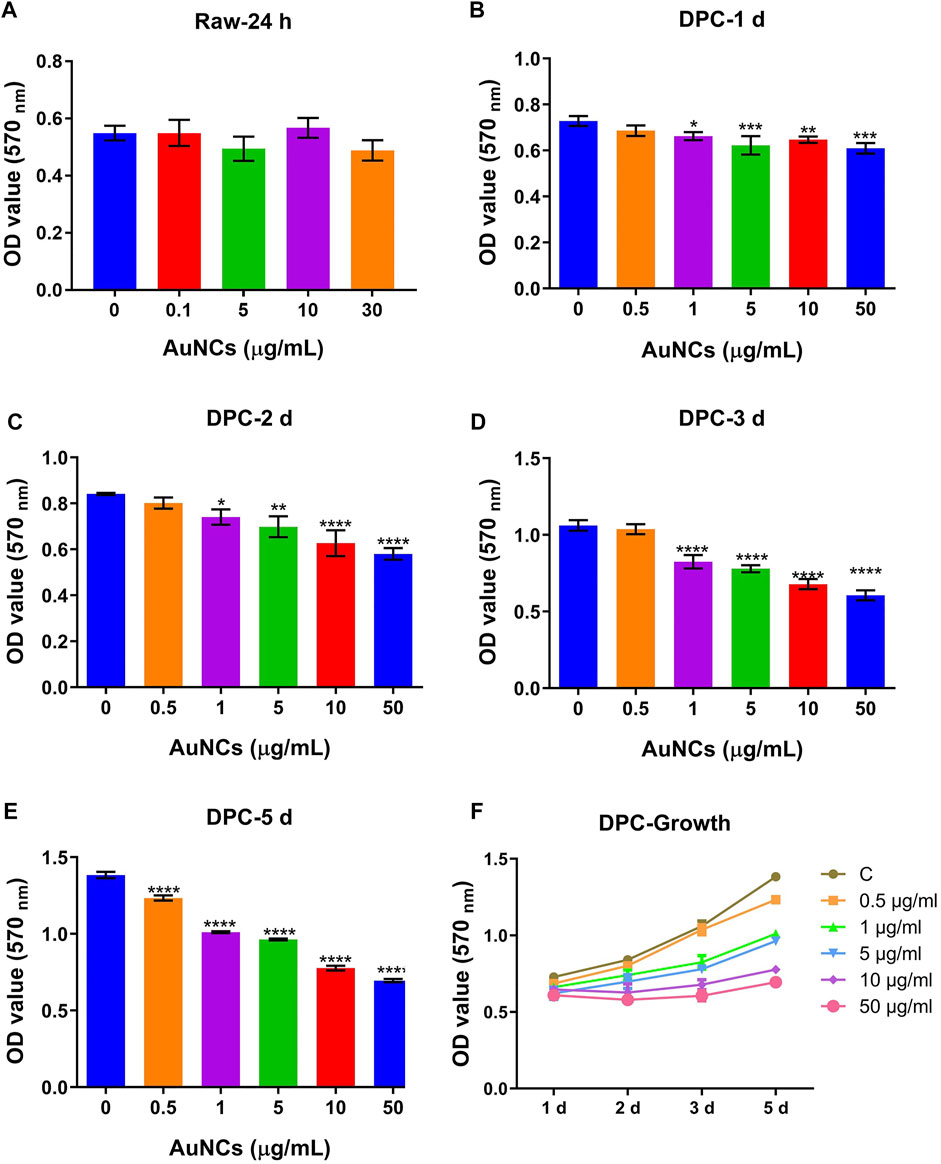
FIGURE 1. Cytotoxicity of AuNCs on macrophages (RAW cells) and hDPC examined using an MTT assay. (A) There was significant difference in the proliferation in RAW cells treated with graded AuNCs for up to 24 h. (B–E) MTT assay results in hDPC treated with graded AuNCs for up to 5 days. AuNCs at doses than 0.5 μg/ml significantly inhibited the proliferation in hDPC (as compared with AuNC-0 µg/ml-treated controls). (F) Growth curves of hDPC treated with graded doses of AuNCs. Data are presented as the mean ± SD (n = 3). *p < 0.05, **p < 0.01, ***p < 0.001, ****p < 0.0001 versus the control group (AuNC-0 µg/ml).
Gold Nanoclusters Inhibited M1 Makers and Induced M2 Markers in Macrophages Upon Inflammatory Stimulation
To investigate the regulatory effect of AuNCs on macrophage inflammatory responses, the RAW cells were treated with graded doses of AuNCs during inflammatory stimulation and we measured M1/inflammatory markers and M2/anti-inflammatory and tissue regenerative markers. As shown in Figure 2A, AuNC treatment significantly reduced the mRNA levels of inflammatory markers (iNOS, CD86, CD11c, IL-6, MHC-Ⅱ, and TNF) (Xue et al., 2017; Xiao et al., 2020). In particular, the mRNA levels of iNOS, CD86, IL-6, and TNF were downregulated by AuNCs in a dose-dependent manner (Figure 2A), suggesting that the M1 phenotype was inhibited under inflammatory conditions. The mRNA levels of anti-inflammatory/tissue regenerative markers (BMP2, BMP6, IL-10, Wnt3a, and Wnt5a) (Zhang et al., 2017a; Li et al., 2018; Shapouri-Moghaddam et al., 2018; Cosin-Roger et al., 2019) were elevated by AuNCs in a dose-dependent manner (Figure 2B). Accordingly, AuNC treatment downregulated the protein levels of the M1 marker iNOS, whereas the protein levels of the M2 marker arginase were elevated following AuNC treatment (Figure 2C). These findings suggest that AuNCs effectively regulated macrophage inflammatory responses by introducing the phenotype switch from M1 toward M2.
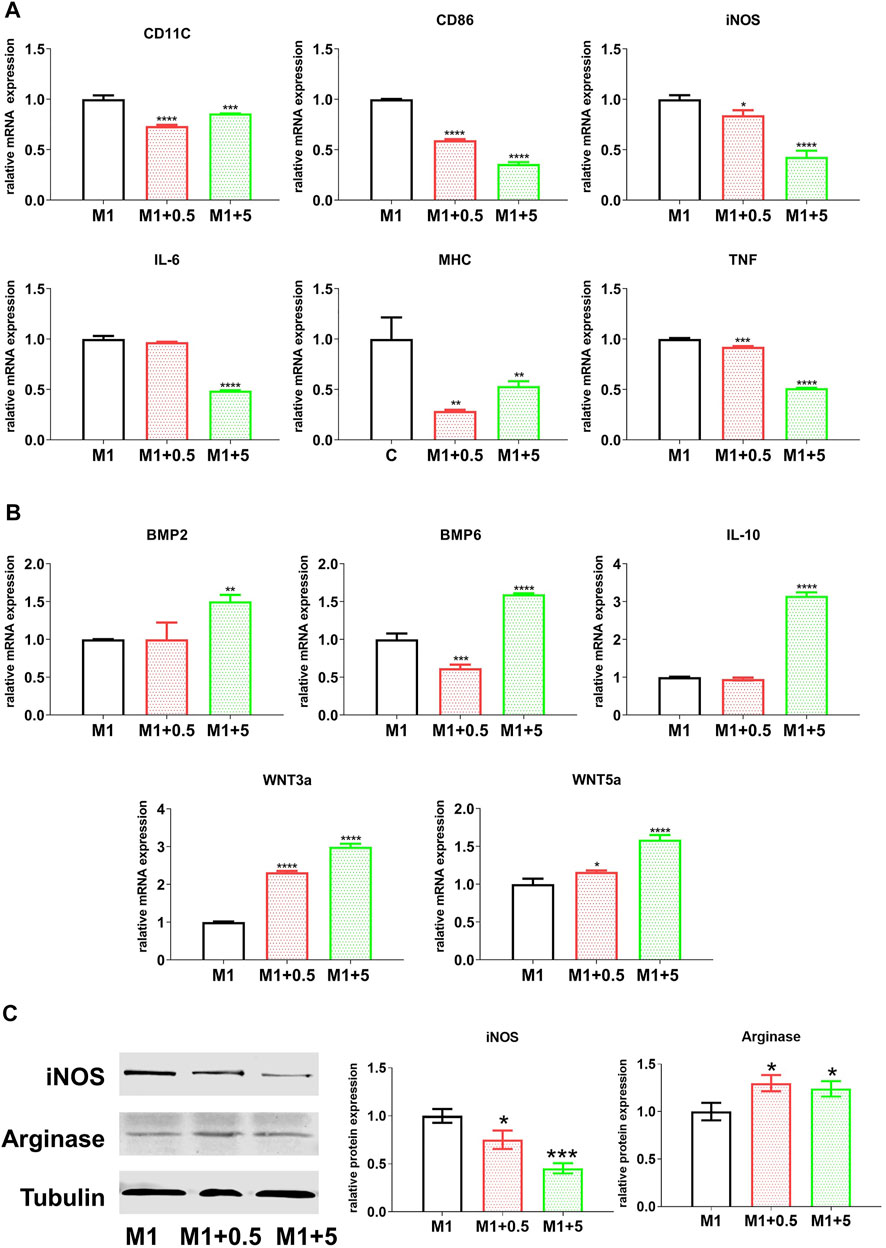
FIGURE 2. Effect of AuNCs on the pro-inflammatory response of RAW cells, treated with AuNCs (0, 0.5, and 5 μg/ml; AuNCs at 0 μg/ml served as the control group) during inflammatory stimulation (LPS + IFNγ) for 24 h. mRNA levels of AuNCs-0.5/5 μg/ml groups were normalized against the control group and are expressed as fold-changes. (A) mRNA levels of pro-inflammatory M1-like markers (CD11c, CD86, iNOS, IL-6, MHC-Ⅱ, and TNF) were significantly downregulated, whereas (B) the levels of anti-inflammatory M2-like markers (BMP2, BMP6, IL-10, Wnt3a, and Wnt5a) were significantly higher than those of the controls. (C) Western blotting results revealing that protein levels of iNOS (M1) and arginase were downregulated following AuNC application, while arginase expression levels (M2) were elevated in AuNC-treated cells. Data are presented as the mean ± SD (n = 3). *p < 0.05, **p < 0.01, ***p < 0.001 versus the M1 control group (AuNC-0 µg/ml), respectively. M1/M1 + 0.5/M1 + 5: RAW cells treated with AuNCs at 0, 0.5, and 5 μg/ml, upon LPS and IFNγ stimulation.
Gold Nanoclusters-Derived Macrophage Regulation Facilitated Odontoblast-like Differentiation of Human Dental Pulp Stromal Cells
To examine the effect of the M1–M2 switch on hDPC differentiation toward odontoblasts, the CM harvested from RAW cells (treated with graded AuNCs under inflammatory stimulation) was used to treat hDPC under conditions of stimulated differentiation. As shown in Figure 3, at 3 and 7 days of differentiation, the CM from AuNC-treated macrophages gave rise to elevated mRNA levels of the odontoblast differentiation markers BSP, OCN, and Runx2 in hDPC (Ching et al., 2016; Jani et al., 2018; Wang et al., 2018), which were increased consistently with the graded AuNC doses in the macrophage treatment, as compared with hDPC treated with M1–CM. In particular, the dentin generation markers DMP-1 and DSPP (Ching et al., 2016) were upregulated by the CM from AuNC-treated macrophages in a dose-dependent manner. On the other hand, at 3 days of differentiation, the mRNA levels of OPN were downregulated in hDPC (Figure 3A) treated with the CM from AuNC-treated macrophages and at 7 days of differentiation, the CM from AuNC-5 µg/ml-treated macrophages showed significantly greater OPN expression in hDPC (Figure 3B) than in hDPC treated with M1-CM. Similarly, AuNC-treated macrophage-derived CM upregulated the expression levels of odontoblast-differentiation markers (OCN and Runx2) and dentin generation markers (DMP-1 and DSPP) in hDPC (Figure 3). Consistent with the mRNA results, the CM from AuNC-treated macrophages gave rise to elevated protein levels of the odontoblast differentiation markers ALP and osterix (Kim et al., 2015), and upregulated β-catenin (key factors in the Wnt/β-catenin odontoblast differentiation pathway (Wang et al., 2018)) in hDPC at both 3 and 7 days of differentiation, as compared with hDPC treated with M1-CM (Figure 4). These findings suggest that the AuNC-directed M1-to-M2 macrophage phenotype switch facilitated hDPC’ odontoblast-like differentiation to favor dentin regeneration.
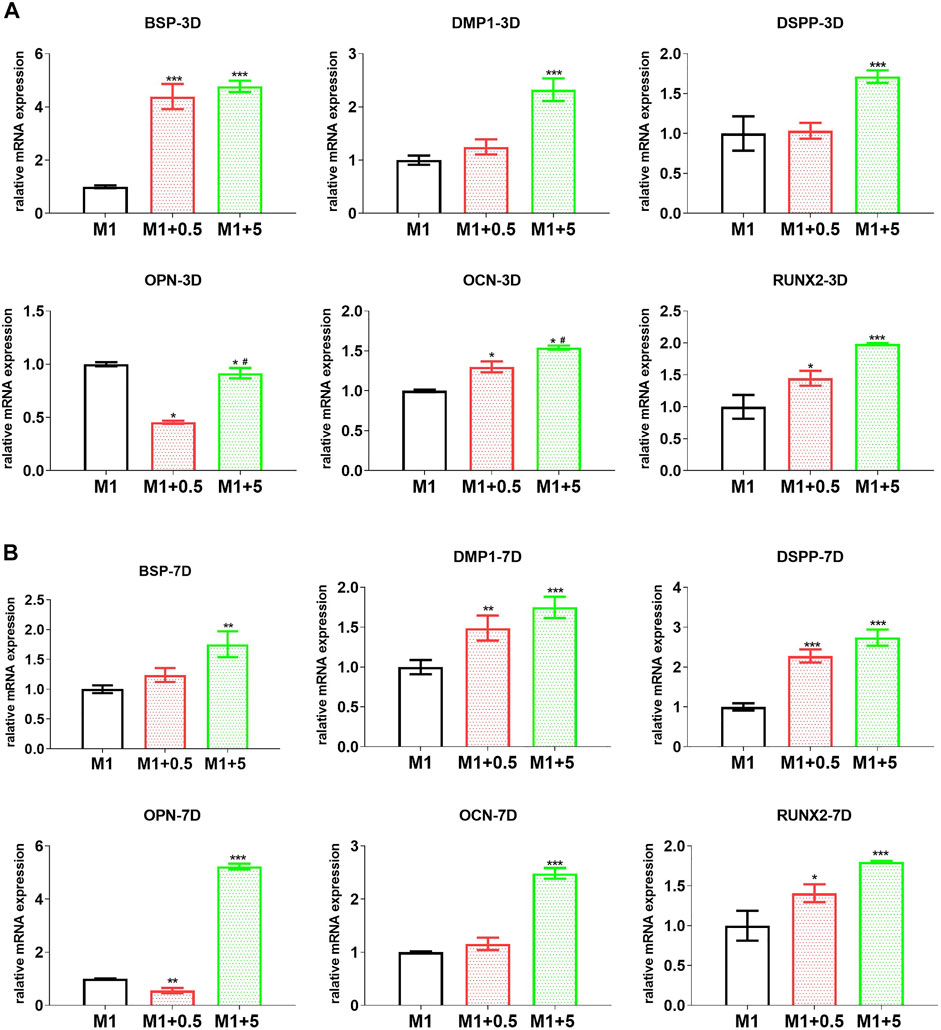
FIGURE 3. AuNC-treated RAW cell-originated CM improved the odontoblast differentiation of hDPC. The mRNA levels of odontoblat differentiation-related markers (BSP, OPN, OCN, and RUNX2) and especially dentin regeneration markers DMP-1 and DSPP were upregulated in hDPC treated with the CM from AuNC-treated RAW cells, at both 3 (A) and 7 (B) days of differentiation. Data are presented as the mean ± SD (n = 3). *p < 0.05, **p < 0.01, ***p < 0.001 versus the M1 control group. M1/M1 + 0.5/M1 + 5: hDPC treated with the CM from RAW cells (stimulated by LPS and IFNγ) treated with AuNCs at 0, 0.5, and 5 μg/ml.
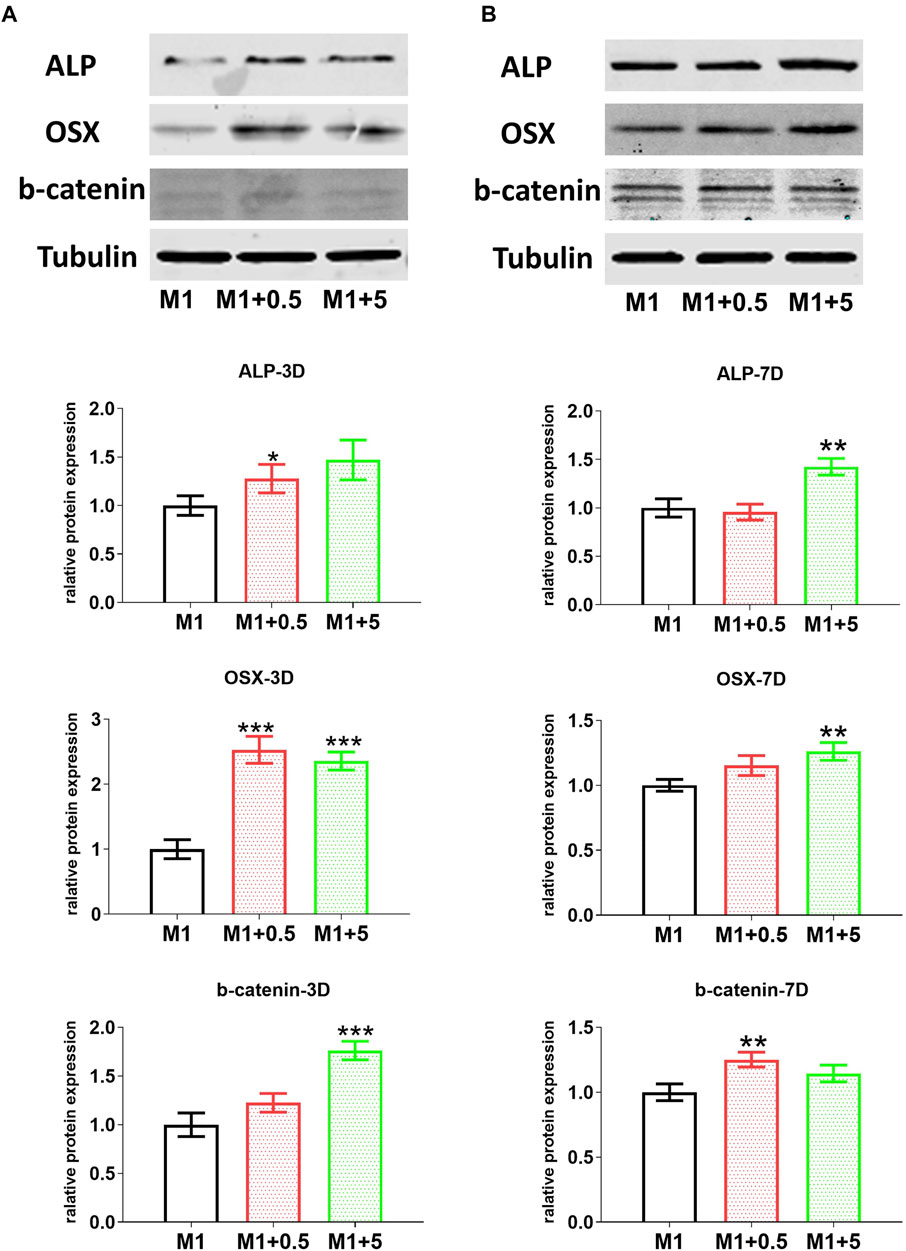
FIGURE 4. AuNC-treated RAW cell-originated CM improved the odontoblast differentiation of hDPC. Western blotting results showed that protein levels of odontoblast differentiation-related markers ALP, osterix (OSX) and especially the marker β-catenin (b-catenin) for the key signaling pathway in differentiation were upregulated in hDPC treated with the CM from AuNC-treated RAW cells, at both 3 (A) and 7 (B) days of differentiation. Data are presented as the mean ± SD (n = 3). *p < 0.05, **p < 0.01, ***p < 0.001 versus the M1 control group. M1/M1 + 0.5/M1 + 5: hDPC treated with the CM from RAW cells (stimulated by LPS and IFNγ) treated with AuNCs at 0, 0.5, and 5 μg/ml.
Gold Nanoclusters Treatment-Induced Odontoblast-like Differentiation and Mineralization of Human Dental Pulp Stromal Cells
In vitro experiments were performed to examine the direct effect of AuNCs on the odontoblast differentiation of hDPC. As shown in Figure 5A, the AuNC treatment gave rise to significantly higher mRNA levels of odontoblast differentiation markers (BSP OPN, OCN, and Runx2 (Ching et al., 2016; Jani et al., 2018; Wang et al., 2018)) than in the control group. In particular, AuNCs upregulated the expression levels of dentin generation markers DMP-1 and DSPP in a dose-dependent manner. Accordingly, the protein levels of odontoblast differentiation markers ALP and osterix were elevated following the AuNC treatment (Figure 6). β-catenin expression was also significantly higher in AuNC-treated hDPC than in the controls (Figure 6). Furthermore, at 14 days of differentiation, AuNCs significantly enhanced the mineralization of hDPC in a dose-dependent manner, suggesting that AuNC treatment facilitated dentin regeneration (Figure 7).
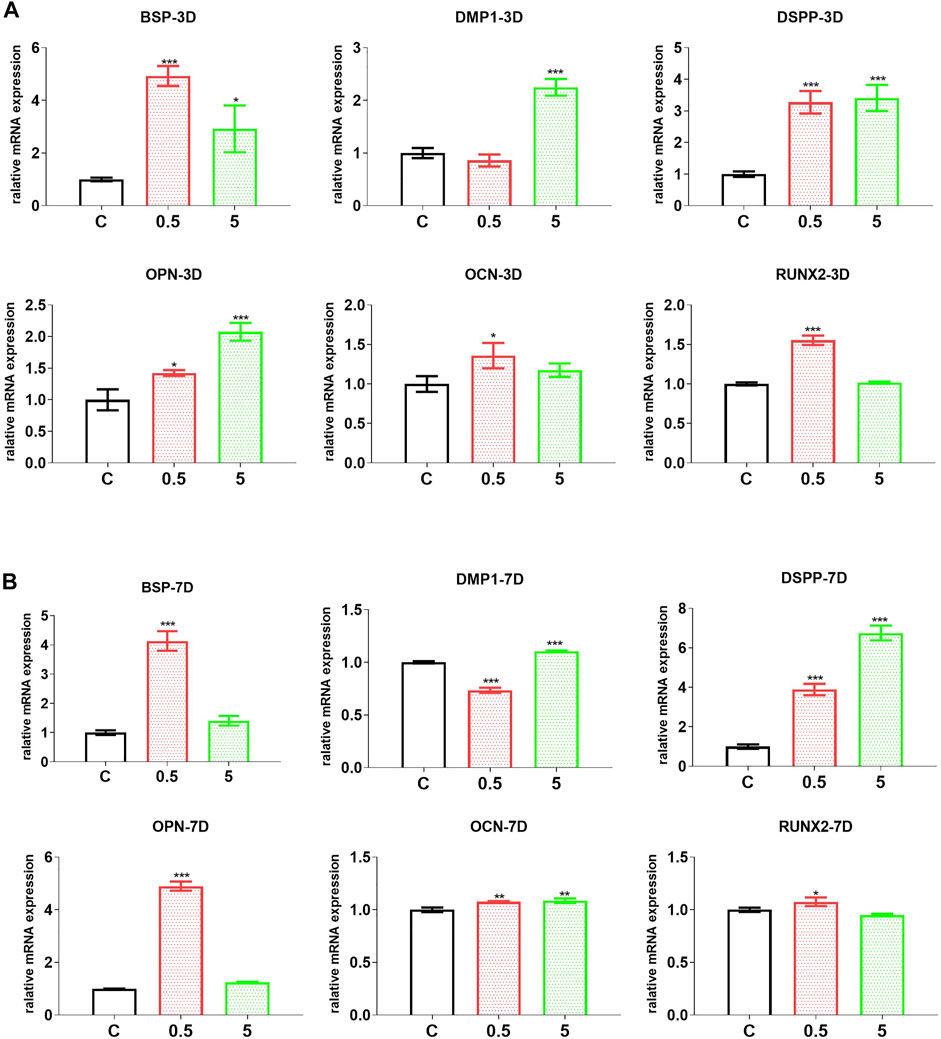
FIGURE 5. AuNC-treatment directly improved the odontoblast differentiation of hDPC. The mRNA levels of odontoblast differentiation-related markers (BSP, OPN, OCN, and RUNX2) and especially dentin regeneration markers DMP-1 and DSPP were upregulated in hDPC treated with AuNCs at both 3 (A) and 7 (B) days of differentiation. Data are presented as the mean ± SD (n = 3). *p < 0.05, **p < 0.01, ***p < 0.001 versus the control group (treated with AuNC-0 µg/ml). C/0.5/5: hDPC treated with AuNCs at 0, 0.5, and 5 μg/ml.
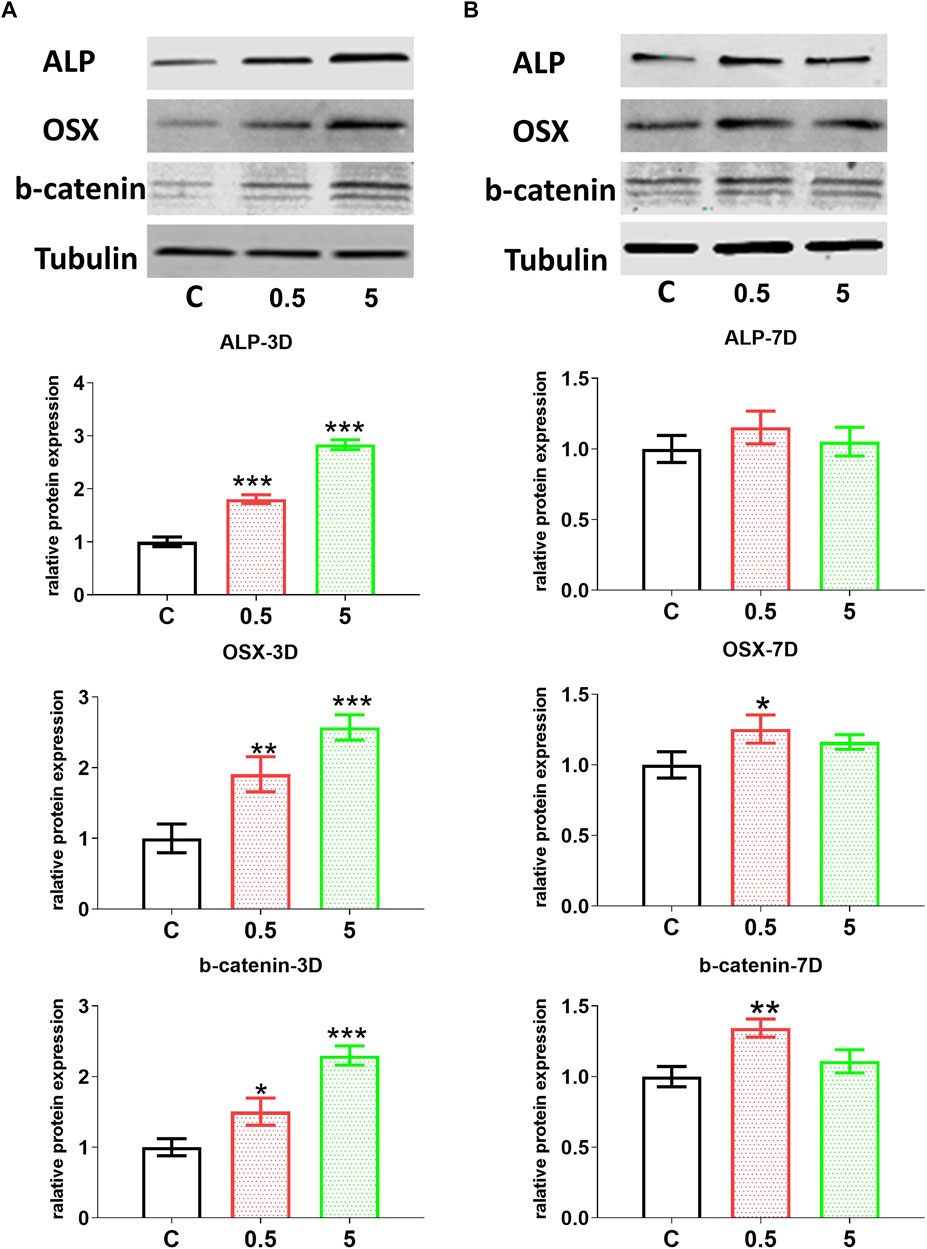
FIGURE 6. AuNC-treatment directly improved the odontoblast differentiation of hDPC. Western blotting results showed that the protein levels of odontoblast differentiation-related markers ALP, osterix/OSX, and especially the marker (β-catenin/b-catenin) for the key signaling pathway in differentiation were upregulated in hDPC treated with the CM from AuNC-treated RAW cells, at both 3 (A) and 7 (B) days of differentiation. Data are presented as the mean ± SD (n = 3). *p < 0.05, **p < 0.01, ***p < 0.001 versus the control group (treated with AuNC-0 µg/ml). C/0.5/5: hDPC treated with AuNCs at 0, 0.5, and 5 μg/ml, respectively.
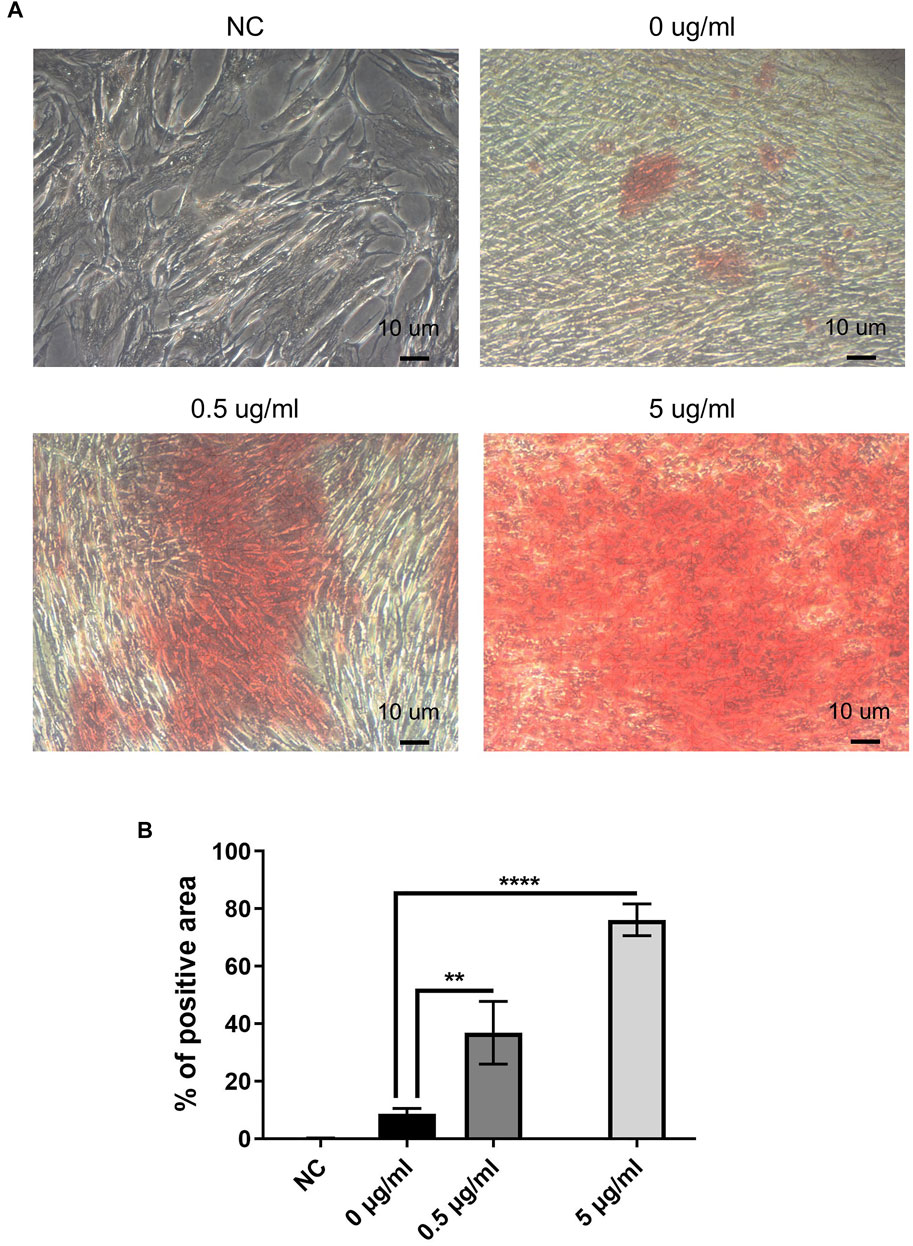
FIGURE 7. AuNC treatment directly facilitated hDPC’ mineralization. (A) Alizarin red S staining results (original magnification: 200×) showed that the mineralization of hDPC (red-staining area) was significantly greater in cells with AuNC treatment than in the AuNC-0 µg/ml control. AuNC-5 μg/ml showed the strongest positive staining. In cells without osteogenic differentiation (negative control, NC), no positive stain was observed. (B) Quantification of Alizarin red S staining was performed (percentage of positive area) using the ImageJ software. **p < 0.01, ****p < 0.0001 versus the AuNC-0 µg/ml group.
Discussion
Macrophages are found in several phenotypic subsets. The non-activated subset is the M0 phenotype, which is polarized on stimulation to generate a spectrum of macrophage phenotypes; the two ends of this spectrum are termed the classically activated pro-inflammatory M1 phenotype [by microbe-derived lipopolysaccharide (LPS) and cytokine interferon γ, (IFNγ)], and the alternatively activated anti-inflammatory M2 phenotype [by interleukin 4 (IL-4) and IL-13] (Mills et al., 2000; Mantovani et al., 2004; Murray et al., 2014; Horwood, 2015). On infection, pulp macrophages are activated by bacteria-derived LPS, which then induce M1 polarization. M1 macrophages induce an inflammatory microenvironment and then trigger the activation of adaptive immune cells to produce pro-inflammatory cytokines (e.g., tumor necrosis factor-α/TNFα, interleukin-1β/IL-1β), and especially matrix metalloproteinases to exacerbate tissue degeneration (Shapouri‐Moghaddam et al., 2018; Yang et al., 2018; Aristorena et al., 2019). By contrast, cytokines from M2 macrophages not only downregulate inflammation (e.g., IL-10) (Shapouri‐Moghaddam et al., 2018), but also induce dentin regeneration (e.g., BMP-2) (Zhang et al., 2017a; Pajarinen et al., 2019). Biomaterials inducing the M1-to-M2 phenotype switch have been found to promote bone regeneration (Chen et al., 2014; Wu et al., 2014; Chen et al., 2015; Chen et al., 2016). In a rat molar pulp tissue regeneration model, macrophages showed phenotype conversion from M1 at the early inflammatory stage to M2 at the late regenerative stage (Gu et al., 2019), suggesting that modulating the M1-to-M2 phenotype switch could be a strategy to induce dentin regeneration.
In the present study, first, the cytotoxicity of AuNCs on RAW cells (macrophages) and hDPC was analyzed. Previous studies demonstrated that AuNCs have good biocompatibility and show negligible toxicity to cells in a culture (Shang et al., 2011; Shang et al., 2012; Shang et al., 2013). In our previous study (Xiao et al., 2020), AuNCs at doses ≤5 μg/ml did not inhibit the growth of BV2 microglia (residential macrophages in the central nervous system). In the present study, AuNCs at doses lower than 5 μg/ml did not significantly inhibit cell metabolism in the RAW cells (Figure 1A), suggesting no impact on the macrophage proliferation with AuNCs at doses ≤5 μg/ml. By contrast, hDPC were more sensitive to AuNCs, which reduced cell growth (metabolism) at doses higher than 0.5 μg/ml (Figures 1B–E), suggesting that primary cultures might be more sensitive than the cell lines. On the other hand, according to the cell growth curve (Figure 1F), there was no decrease in cell amount from days 1–5 with the treatment of AuNCs.
In our previous study (Kim et al., 2015), AuNCs reduced inflammatory responses of microglial cells, a form of regulation that was favorable for neuronal cell differentiation. Accordingly, AuNC treatment efficiently regulated the macrophage inflammatory response by introducing the phenotype conversion from M1 to M2, which reduced the expression of inflammatory markers (CD11c, CD86, iNOS, IL-6, MHC-Ⅱ, and TNF) in a dose-dependent manner, while inducing the expression of anti-inflammatory markers (BMP2, BMP6, IL-10, Wnt3a, and Wnt5a). Previous studies demonstrated the critical role of immunomodulation in material-derived hard tissue regeneration, such that the M1-to-M2 phenotype switch facilitated bone healing (Guihard et al., 2012; Loi et al., 2016; Xiao et al., 2019), while the continuous inflammatory response of M1 macrophages resulted in failed bone regeneration, culminating in the generation of fibrous tissue (Chen et al., 2016).
Factors such as TNF-α and iNOS not only exacerbate inflammatory responses to cause pain and tissue damage in the pulp (Ringe et al., 2007; Colombo et al., 2014), but they also impair osteogenic differentiation, thereby impeding the hard tissue regeneration (Chen et al., 2016). By contrast, M2-derived IL-10 exerts anti-inflammatory effects to protect cells from inflammatory damage (Ekström et al., 2013). Moreover, the tissue-regenerative factors such as BMPs and Wnt ligands demonstrated osteoinductive effects (Nakashima, 2005; Zhao et al., 2018; Ali et al., 2019). These findings suggest that the AuNC-directed M1-to-M2 phenotype switch should be beneficial for dentin regeneration.
To determine the effect of AuNC-derived macrophage regulation on dentin regeneration, the CM from AuNC-treated macrophages was applied during the hDPC’ odontoblast-like differentiation as previously described (Huang et al., 2017). As expected from the macrophage phenotype conversion, the CM from AuNC-treated macrophages significantly induced the expression of odontoblast differentiation-related markers in hDPC; in particular, the expression levels of the two markers reflecting dentin generation were upregulated in a dose-dependent manner (Figures 3, 4). Consistent with the induced expression of Wnt ligands in AuNC-treated macrophages (Figure 2B), elevated β-catenin protein levels were observed in hDPC stimulated with the CM from AuNC-treated macrophages (Figure 4), suggesting that AuNC-directed regulation induced the Wnt-ligand production from macrophages, which then subsequently activated the Wnt/β-catenin signaling pathway to facilitate odontoblast-like differentiation of the hDPC. Taken together, these findings suggest that AuNCs induce immunomodulation to create a microenvironment favorable for odontoblast-like differentiation, thereby benefiting dentin tissue regeneration.
We also examined the direct effects of AuNCs on odontoblast-like differentiation and mineralization. AuNCs induced the mRNA and protein levels of odontoblast differentiation-associated markers in a dose-dependent manner (Figures 5, 6). Similarly, β-catenin levels were elevated following AuNC treatment (Figure 6), suggesting that AuNCs facilitated odontoblast-like differentiation through the Wnt/β-catenin signaling pathway. AuNCs also induced the expression of dentin regeneration-related markers (Figure 5), which improved the in vitro mineralization of hDPC (Figure 7) in a dose-dependent manner. These results suggest that AuNCs potentially facilitate dentin tissue regeneration.
Because of the ultra-small size (less than 3 nm), AuNCs can be efficiently uptaken by both immune cells and tissue progenitor cells, as indicated in our previous study (Xiao et al., 2020). It has been found that AuNCs accumulated intracellularly in microglia (macrophages in the central nervous system), which partially co-localized with autophagosomes in the microglia and induced autophagy while reducing apoptosis in microglia under inflammatory stimulation, an effect which may contribute to the M1-to-M2 phenotype switch by facilitating the clearance of reactive oxygen species (ROS) (Xiao et al., 2020). It is, therefore, speculated that when applying AuNCs in the inflammatory macrophage-DPC interplay, the endocytosis of AuNCs can induce the M1-to-M2 conversion by activating autophagy, a mechanism which should be further explored in our future study. This then changes the microenvironment to induce DPC differentiation. On the other hand, the AuNC-endocytosis in DPC can improve the odontoblast-like differentiation and mineralization. This, together with the favorable immune microenvironment revised by AuNCs, significantly facilitates dentin regeneration. A future in vivo study is required to observe the distribution of AuNCs in the dental pulp tissue and the translational potential of AuNCs in dentin regeneration.
The most important feature in the maintenance of dental pulp vitality and regeneration is the induction of the generation of a dentin bridge that can prevent infection in the regenerating pulp tissue and also prevent pulp cells from micro-leakage and reinfection that would ultimately result in regeneration failure (Slutzky-Goldberg et al., 2009; Kermanshahi et al., 2010; Başaran et al., 2012). It is therefore unclear why dental pulp progenitor cells, which have shown efficient odontoblast differentiation and mineralization potential in vitro (Waddington et al., 2009; d'Aquino et al., 2007; Iohara et al., 2008), are unable to form functional dentin bridges in vivo in pulpitis, even with pulp sterilization, thus making RCT the only available clinical treatment of irreversible pulpitis in adult teeth. Because infection triggers macrophage inflammatory responses and induces M1 polarization, this phenotype would result in the failure in regeneration of hard tissue such as bone (Chen et al., 2016); we therefore wondered whether continuous inflammation could explain the dentin regeneration failure, and whether material-derived immunomodulation of macrophage responses would induce the M1-to-M2 phenotype switch to serve as a potential therapeutic approach for dental pulp regeneration. We found that AuNCs, a type of nanomaterial with demonstrated immunoregulatory effects (Xiao et al., 2020), effectively induced the conversion from M1 to M2 macrophages in inflammatory conditions, transforming the local immune microenvironment to one favorable to dentin tissue regeneration, as demonstrated by the induced odontoblast-like differentiation of hDPC.
Presently, dentin-regenerative material development focuses on stem cell transplantation with artificial scaffolds or adding growth factors such as platelet-derived growth factor (PDGF) to enhance osteoblast differentiation and dentin regeneration (Colombo et al., 2014; Wang et al., 2016; Bottino et al., 2017; Zhang et al., 2017b; Athirasala et al., 2018; Xu et al., 2019). The present study suggests that material-derived immunomodulation to induce the M1-to-M2 phenotype switch could be a potential strategy for the material design of dentin regeneration. We found that AuNCs directly enhanced the differentiation and mineralization of hDPC, further suggesting the potential of AuNC application in dentin regeneration. This study, therefore, provides a potential approach to induce dentin regeneration to favor dental pulp vitality maintenance and regeneration, and this would improve the clinical treatment against diseases such as irreversible pulpitis. Of note, because we only tested the effects of AuNCs in vitro, further in vivo and translational studies are required to verify the future of AuNC application in dentistry.
Taken together, our results suggest that AuNCs could be a potential therapeutic tool for dentin regeneration. AuNCs efficiently facilitate the resolution of inflammation by stimulating a macrophage phenotype switch from M1 to M2. This switch would generate an immune microenvironment suitable for odontoblast differentiation and mineralization, thereby potentially facilitating dentin bridge formation. The present study also suggests that immunomodulation could be a potential strategy to enhance dentin regeneration in the design of materials in the future.
Data Availability Statement
The raw data supporting the conclusion of this article will be made available by the authors, without undue reservation.
Ethics Statement
The studies involving human participants were reviewed and approved by the Human ethics committee, Queensland University of Technology. The patients provided their written informed consent to participate in this study.
Author Contributions
LY and LX: involved in experimental design, lab work, data analysis, and draft writing. TL and WG: assisted in lab work and data analysis. YX: involved in experimental design and manuscript review.
Funding
This work was supported by the NHMRC Idea Grant (APP2000647) of Australia, Young Researcher Grant (19-066) from the Osteology Foundation, Switzerland, QUT Centre for Biomedical Technologies ECR/MCR grant scheme 2021, the National Natural Science Foundation of China (31971262), Scientific research project of Guangdong Bureau of Traditional Chinese Medicine (20202115).
Conflict of Interest
The authors declare that the research was conducted in the absence of any commercial or financial relationships that could be construed as a potential conflict of interest.
Publisher’s Note
All claims expressed in this article are solely those of the authors and do not necessarily represent those of their affiliated organizations, or those of the publisher, the editors and the reviewers. Any product that may be evaluated in this article, or claim that may be made by its manufacturer, is not guaranteed or endorsed by the publisher.
References
Ali, M., Okamoto, M., Komichi, S., Watanabe, M., Huang, H., Takahashi, Y., et al. (2019). Lithium-containing Surface Pre-reacted Glass Fillers Enhance hDPSC Functions and Induce Reparative Dentin Formation in a Rat Pulp Capping Model through Activation of Wnt/β-Catenin Signaling. Acta Biomater. 96, 594–604. doi:10.1016/j.actbio.2019.06.016
Aristorena, M., Gallardo-Vara, E., Vicen, M., de Las Casas-Engel, M., Ojeda-Fernandez, L., Nieto, C., et al. (2019). MMP-12, Secreted by Pro-inflammatory Macrophages, Targets Endoglin in Human Macrophages and Endothelial Cells. Ijms 20 (12), 3107. doi:10.3390/ijms20123107
Athirasala, A., Tahayeri, A., Thrivikraman, G., França, C. M., Monteiro, N., Tran, V., et al. (2018). A Dentin-Derived Hydrogel Bioink for 3D Bioprinting of Cell Laden Scaffolds for Regenerative Dentistry. Biofabrication 10 (2), 024101. doi:10.1088/1758-5090/aa9b4e
Balic, A., Aguila, H. L., Caimano, M. J., Francone, V. P., and Mina, M. (2010). Characterization of Stem and Progenitor Cells in the Dental Pulp of Erupted and Unerupted Murine Molars. Bone 46 (6), 1639–1651. doi:10.1016/j.bone.2010.02.019
Başaran, E. G., Ayna, E., and Halifeoğlu, M. (2012). Microleakage of Endodontically Treated Teeth Restored with 3 Different Adhesive Systems and 4 Different Fiber-Reinforced Posts. J. Prosthet Dent 107 (4), 239–251. doi:10.1016/s0022-3913(12)60069-9
Bookout, A. L., and Mangelsdorf, D. J. (2003). Quantitative Real-Time PCR Protocol for Analysis of Nuclear Receptor Signaling Pathways. Nucl. Receptor Signaling 1, nrs01012. doi:10.1621/nrs.01012
Bottino, M. C., Pankajakshan, D., and Nör, J. E. (2017). Advanced Scaffolds for Dental Pulp and Periodontal Regeneration. Dental Clin. North America 61 (4), 689–711. doi:10.1016/j.cden.2017.06.009
Bustin, S. A., Benes, V., Garson, J. A., Hellemans, J., Huggett, J., Kubista, M., et al. (2009). The MIQE Guidelines: Minimum Information for Publication of Quantitative Real-Time PCR Experiments. Clin. Chem. 55 (4), 611–622. doi:10.1373/clinchem.2008.112797
Cao, Y., Liu, Z., Xie, Y., Hu, J., Wang, H., Fan, Z., et al. (2015). Adenovirus-mediated Transfer of Hepatocyte Growth Factor Gene to Human Dental Pulp Stem Cells under Good Manufacturing Practice Improves Their Potential for Periodontal Regeneration in Swine. Stem Cel Res Ther 6, 249. doi:10.1186/s13287-015-0244-5
Casagrande, L., Cordeiro, M. M., Nör, S. A., and Nör, J. E. (2011). Dental Pulp Stem Cells in Regenerative Dentistry. Odontology 99 (1), 1–7. doi:10.1007/s10266-010-0154-z
Chen, Z., Klein, T., Murray, R. Z., Crawford, R., Chang, J., Wu, C., et al. (2016). Osteoimmunomodulation for the Development of Advanced Bone Biomaterials. Mater. Today 19 (6), 304–321. doi:10.1016/j.mattod.2015.11.004
Chen, Z., Yi, D., Zheng, X., Chang, J., Wu, C., and Xiao, Y. (2014). Nutrient Element-Based Bioceramic Coatings on Titanium alloy Stimulating Osteogenesis by Inducing Beneficial Osteoimmmunomodulation. J. Mater. Chem. B 2 (36), 6030–6043. doi:10.1039/c4tb00837e
Chen, Z., Yuen, J., Crawford, R., Chang, J., Wu, C., and Xiao, Y. (2015). The Effect of Osteoimmunomodulation on the Osteogenic Effects of Cobalt Incorporated β-tricalcium Phosphate. Biomaterials 61, 126–138. doi:10.1016/j.biomaterials.2015.04.044
Ching, H., Luddin, N., Rahman, I., and Ponnuraj, K. (2016). Expression of Odontogenic and Osteogenic Markers in DPSCs and SHED: A Review. Cscr 12 (1), 71–79. doi:10.2174/1574888x11666160815095733
Colombo, J. S., Moore, A. N., Hartgerink, J. D., and D’Souza, R. N. (2014). Scaffolds to Control Inflammation and Facilitate Dental Pulp Regeneration. J. endodontics 40 (4 Suppl. l), S6–S12. doi:10.1016/j.joen.2014.01.019
Cosin-Roger, J., Ortiz-Masià, M. D., and Barrachina, M. D. (2019). Macrophages as an Emerging Source of Wnt Ligands: Relevance in Mucosal Integrity. Front. Immunol. 10, 2297. doi:10.3389/fimmu.2019.02297
d'Aquino, R., Graziano, A., Sampaolesi, M., Laino, G., Pirozzi, G., De Rosa, A., et al. (2007). Human Postnatal Dental Pulp Cells Co-differentiate into Osteoblasts and Endotheliocytes: a Pivotal Synergy Leading to Adult Bone Tissue Formation. Cell Death Differ 14 (6), 1162–1171. doi:10.1038/sj.cdd.4402121
Ekström, K., Omar, O., Granéli, C., Wang, X., Vazirisani, F., and Thomsen, P. (2013). Monocyte Exosomes Stimulate the Osteogenic Gene Expression of Mesenchymal Stem Cells. PloS one 8 (9), e75227. doi:10.1371/journal.pone.0075227
Gu, B., Kaneko, T., Zaw, S. Y. M., Sone, P. P., Murano, H., Sueyama, Y., et al. (2019). Macrophage Populations Show an M1-To-M2 Transition in an Experimental Model of Coronal Pulp Tissue Engineering with Mesenchymal Stem Cells. Int. Endod. J. 52 (4), 504–514. doi:10.1111/iej.13033
Guihard, P., Danger, Y., Brounais, B., David, E., Brion, R., Delecrin, J., et al. (2012). Induction of Osteogenesis in Mesenchymal Stem Cells by Activated Monocytes/macrophages Depends on Oncostatin M Signaling. Stem cells (Dayton, Ohio) 30 (4), 762–772. doi:10.1002/stem.1040
Harichane, Y., Hirata, A., Dimitrova-Nakov, S., Granja, I., Goldberg, A., Kellermann, O., et al. (2011). Pulpal Progenitors and Dentin Repair. Adv. Dent Res. 23 (3), 307–312. doi:10.1177/0022034511405322
Harris, R., and Griffin, C. J. (1969). The fine Structure of the Mature Odontoblasts and Cell Rich Zone of the Human Dental Pulp. Aust. dental J. 14 (3), 168–177. doi:10.1111/j.1834-7819.1969.tb03348.x
Horwood, N. J. (2015). Macrophage Polarization and Bone Formation: A Review. Clin. Rev. Allergy Immunol., 51, 1–8. doi:10.1007/s12016-015-8519-2
Hu, L., Gao, Z., Xu, J., Zhu, Z., Fan, Z., Zhang, C., et al. (2017). Decellularized Swine Dental Pulp as a Bioscaffold for Pulp Regeneration. Biomed. Research International 2017, 1–9. doi:10.1155/2017/9342714
Huang, R., Wang, X., Zhou, Y., and Xiao, Y. (2017). RANKL-induced M1 Macrophages Are Involved in Bone Formation. Bone Res. 5, 17019. doi:10.1038/boneres.2017.19
Iohara, K., Zheng, L., Wake, H., Ito, M., Nabekura, J., Wakita, H., et al. (2008). A Novel Stem Cell Source for Vasculogenesis in Ischemia: Subfraction of Side Population Cells from Dental Pulp. Stem cells (Dayton, Ohio) 26 (9), 2408–2418. doi:10.1634/stemcells.2008-0393
Iwasaki, Y., Otsuka, H., Yanagisawa, N., Hisamitsu, H., Manabe, A., Nonaka, N., et al. (2011). In Situ proliferation and Differentiation of Macrophages in Dental Pulp. Cell Tissue Res 346 (1), 99–109. doi:10.1007/s00441-011-1231-5
Izumi, T., Kobayashi, I., Okamura, K., and Sakai, H. (1995). Immunohistochemical Study on the Immunocompetent Cells of the Pulp in Human Non-carious and Carious Teeth. Arch. Oral Biol. 40 (7), 609–614. doi:10.1016/0003-9969(95)00024-j
Jani, P., Liu, C., Zhang, H., Younes, K., Benson, M. D., and Qin, C. (2018). The Role of Bone Morphogenetic Proteins 2 and 4 in Mouse Dentinogenesis. Arch. Oral Biol. 90, 33–39. doi:10.1016/j.archoralbio.2018.02.004
Kermanshahi, S., Santerre, J. P., Cvitkovitch, D. G., and Finer, Y. (2010). Biodegradation of Resin-Dentin Interfaces Increases Bacterial Microleakage. J. Dent Res. 89 (9), 996–1001. doi:10.1177/0022034510372885
Kim, T. H., Bae, C. H., Lee, J. C., Kim, J. E., Yang, X., de Crombrugghe, B., et al. (2015). Osterix Regulates Tooth Root Formation in a Site-specific Manner. J. Dent Res. 94 (3), 430–438. doi:10.1177/0022034514565647
Li, J., Zhu, J.-J., and Xu, K. (2014). Fluorescent Metal Nanoclusters: from Synthesis to Applications. Trac Trends Anal. Chem. 58, 90–98. doi:10.1016/j.trac.2014.02.011
Li, X., Huang, Q., Liu, L., Zhu, W., Elkhooly, T. A., Liu, Y., et al. (2018). Reduced Inflammatory Response by Incorporating Magnesium into Porous TiO2 Coating on Titanium Substrate. Colloids Surf. B: Biointerfaces 171, 276–284. doi:10.1016/j.colsurfb.2018.07.032
Lin, L. M., and Rosenberg, P. A. (2011). Repair and Regeneration in Endodontics. Int. Endod. J. 44 (10), 889–906. doi:10.1111/j.1365-2591.2011.01915.x
Loi, F., Córdova, L. A., Zhang, R., Pajarinen, J., Lin, T.-h., Goodman, S. B., et al. (2016). The Effects of Immunomodulation by Macrophage Subsets on Osteogenesis In Vitro. Stem Cel Res Ther 7, 15. doi:10.1186/s13287-016-0276-5
Lowder, M. Q., and Mueller, P. O. E. (1998). Dental Embryology, Anatomy, Development, and Aging. Vet. Clin. North America: Equine Pract. 14 (2), 227–245. doi:10.1016/s0749-0739(17)30195-5
Mantovani, A., Sica, A., Sozzani, S., Allavena, P., Vecchi, A., and Locati, M. (2004). The Chemokine System in Diverse Forms of Macrophage Activation and Polarization. Trends Immunology 25 (12), 677–686. doi:10.1016/j.it.2004.09.015
Mills, C. D., Kincaid, K., Alt, J. M., Heilman, M. J., and Hill, A. M. (2000). M-1/M-2 Macrophages and the Th1/Th2 Paradigm. J. Immunol. 164 (12), 6166–6173. doi:10.4049/jimmunol.164.12.6166
Miura, M., Gronthos, S., Zhao, M., Lu, B., Fisher, L. W., Robey, P. G., et al. (2003). SHED: Stem Cells from Human Exfoliated Deciduous Teeth. Proc. Natl. Acad. Sci. U.S.A. 100 (10), 5807–5812. doi:10.1073/pnas.0937635100
Murray, P. J., Allen, J. E., Biswas, S. K., Fisher, E. A., Gilroy, D. W., Goerdt, S., et al. (2014). Macrophage Activation and Polarization: Nomenclature and Experimental Guidelines. Immunity 41 (1), 14–20. doi:10.1016/j.immuni.2014.06.008
Nakashima, M. (2005). Bone Morphogenetic Proteins in Dentin Regeneration for Potential Use in Endodontic Therapy. Cytokine Growth Factor. Rev. 16 (3), 369–376. doi:10.1016/j.cytogfr.2005.02.011
Pajarinen, J., Lin, T., Gibon, E., Kohno, Y., Maruyama, M., Nathan, K., et al. (2019). Mesenchymal Stem Cell-Macrophage Crosstalk and Bone Healing. Biomaterials 196, 80–89. doi:10.1016/j.biomaterials.2017.12.025
Ringe, J., Strassburg, S., Neumann, K., Endres, M., Notter, M., Burmester, G.-R., et al. (2007). Towards In Situ Tissue Repair: Human Mesenchymal Stem Cells Express Chemokine Receptors CXCR1, CXCR2 and CCR2, and Migrate upon Stimulation with CXCL8 but Not CCL2. J. Cel. Biochem. 101 (1), 135–146. doi:10.1002/jcb.21172
Schmalz, G., and Galler, K. M. (2011). Tissue Injury and Pulp Regeneration. J. Dent Res. 90 (7), 828–829. doi:10.1177/0022034511405331
Shahsavari, S., and Behroozi, F. (2016). Gold Nanoclusters: Nanomedicine Potentials and Applications. J. Nanomed Res. 3 (5), 00069. doi:10.15406/jnmr.2016.03.00069
Shang, L., Azadfar, N., Stockmar, F., Send, W., Trouillet, V., Bruns, M., et al. (2011). One-Pot Synthesis of Near-Infrared Fluorescent Gold Clusters for Cellular Fluorescence Lifetime Imaging. Small 7 (18), 2614–2620. doi:10.1002/smll.201100746
Shang, L., Stockmar, F., Azadfar, N., and Nienhaus, G. U. (2013). Intracellular Thermometry by Using Fluorescent Gold Nanoclusters. Angew. Chem. Int. Ed. 52 (42), 11154–11157. doi:10.1002/anie.201306366
Shang, L., Yang, L., Stockmar, F., Popescu, R., Trouillet, V., Bruns, M., et al. (2012). Microwave-assisted Rapid Synthesis of Luminescent Gold Nanoclusters for Sensing Hg2+ in Living Cells Using Fluorescence Imaging. Nanoscale 4 (14), 4155–4160. doi:10.1039/c2nr30219e
Shapouri‐Moghaddam, A., Mohammadian, S., Vazini, H., Taghadosi, M., Esmaeili, S. A., Mardani, F., et al. (2018). Macrophage Plasticity, Polarization, and Function in Health and Disease. J. Cel Physiol 233 (9), 6425–6440. doi:10.1002/jcp.26429
Sigal, M. J., Aubin, J. E., Ten Cate, A. R., and Pitaru, S. (1984). The Odontoblast Process Extends to the Dentinoenamel junction: an Immunocytochemical Study of Rat Dentine. J. Histochem. Cytochem. 32 (8), 872–877. doi:10.1177/32.8.6379038
Sloan, A. J., and Waddington, R. J. (2009). Dental Pulp Stem Cells: what, where, How? Int. J. paediatric dentistry 19 (1), 61–70. doi:10.1111/j.1365-263X.2008.00964.x
Sloan, A., and Smith, A. (2007). Stem Cells and the Dental Pulp: Potential Roles in Dentine Regeneration and Repair. Oral Dis. 13 (2), 151–157. doi:10.1111/j.1601-0825.2006.01346.x
Slutzky-Goldberg, I., Slutzky, H., Gorfil, C., and Smidt, A. (2009). Restoration of Endodontically Treated Teeth Review and Treatment Recommendations. Int. J. dentistry 2009, 1–9. doi:10.1155/2009/150251
Tziafas, D., Smith, A. J., and Lesot, H. (2000). Designing New Treatment Strategies in Vital Pulp Therapy. J. dentistry 28 (2), 77–92. doi:10.1016/s0300-5712(99)00047-0
Waddington, R. J., Youde, S. J., Lee, C. P., and Sloan, A. J. (2009). Isolation of Distinct Progenitor Stem Cell Populations from Dental Pulp. Cells Tissues Organsorgans 189 (1-4), 268–274. doi:10.1159/000151447
Wang, C., Song, Y., Gu, Z., Lian, M., Huang, D., Lu, X., et al. (2018). Wedelolactone Enhances Odontoblast Differentiation by Promoting Wnt/β-Catenin Signaling Pathway and Suppressing NF-Κb Signaling Pathway. Cell reprogramming 20 (4), 236–244. doi:10.1089/cell.2018.0004
Wang, W., Dang, M., Zhang, Z., Hu, J., Eyster, T. W., Ni, L., et al. (2016). Dentin Regeneration by Stem Cells of Apical Papilla on Injectable Nanofibrous Microspheres and Stimulated by Controlled BMP-2 Release. Acta Biomater. 36, 63–72. doi:10.1016/j.actbio.2016.03.015
Wu, C., and Chang, J. (2012). Mesoporous Bioactive Glasses: Structure Characteristics, Drug/growth Factor Delivery and Bone Regeneration Application. Interf. Focus. 2 (3), 292–306. doi:10.1098/rsfs.2011.0121
Wu, C., Chen, Z., Yi, D., Chang, J., and Xiao, Y. (2014). Multidirectional Effects of Sr-, Mg-, and Si-Containing Bioceramic Coatings with High Bonding Strength on Inflammation, Osteoclastogenesis, and Osteogenesis. ACS Appl. Mater. Inter. 6 (6), 4264–4276. doi:10.1021/am4060035
Xiao, L., Wei, F., Zhou, Y., Anderson, G. J., Frazer, D. M., Lim, Y. C., et al. (2020). Dihydrolipoic Acid-Gold Nanoclusters Regulate Microglial Polarization and Have the Potential to Alter Neurogenesis. Nano Lett. 20 (1), 478–495. doi:10.1021/acs.nanolett.9b04216
Xiao, L., Zhou, Y., Friis, T., Beagley, K., and Xiao, Y. (2019). S1P-S1PR1 Signaling: the "Sphinx" in Osteoimmunology. Front. Immunol. 10, 10. doi:10.3389/fimmu.2019.01409
Xu, F., Qiao, L., Zhao, Y., Chen, W., Hong, S., Pan, J., et al. (2019). The Potential Application of Concentrated Growth Factor in Pulp Regeneration: an In Vitro and In Vivo Study. Stem Cel Res Ther 10 (1), 134. doi:10.1186/s13287-019-1247-4
Xue, N., Zhou, Q., Ji, M., Jin, J., Lai, F., Chen, J., et al. (2017). Chlorogenic Acid Inhibits Glioblastoma Growth through Repolarizating Macrophage from M2 to M1 Phenotype. Sci. Rep. 7, 39011. doi:10.1038/srep39011
Yang, J., Zhu, Y., Duan, D., Wang, P., Xin, Y., Bai, L., et al. (2018). Enhanced Activity of Macrophage M1/M2 Phenotypes in Periodontitis. Arch. Oral Biol. 96, 234–242. doi:10.1016/j.archoralbio.2017.03.006
Zhang, C., Zhou, Z., Qian, Q., Gao, G., Li, C., Feng, L., et al. (2013). Glutathione-capped Fluorescent Gold Nanoclusters for Dual-Modal Fluorescence/X-ray Computed Tomography Imaging. J. Mater. Chem. B 1 (38), 5045–5053. doi:10.1039/c3tb20784f
Zhang, L., and Wang, E. (2014). Metal Nanoclusters: New Fluorescent Probes for Sensors and Bioimaging. Nano Today 9 (1), 132–157. doi:10.1016/j.nantod.2014.02.010
Zhang, M., Jiang, F., Zhang, X., Wang, S., Jin, Y., Zhang, W., et al. (2017). The Effects of Platelet-Derived Growth Factor-BB on Human Dental Pulp Stem Cells Mediated Dentin-Pulp Complex Regeneration. Stem Cell translational Med. 6 (12), 2126–2134. doi:10.1002/sctm.17-0033
Zhang, Y., Böse, T., Unger, R. E., Jansen, J. A., Kirkpatrick, C. J., and van den Beucken, J. J. J. P. (2017). Macrophage Type Modulates Osteogenic Differentiation of Adipose Tissue MSCs. Cel Tissue Res 369 (2), 273–286. doi:10.1007/s00441-017-2598-8
Zhao, Y., Detering, L., Sultan, D., Cooper, M. L., You, M., Cho, S., et al. (2016). Gold Nanoclusters Doped with 64Cu for CXCR4 Positron Emission Tomography Imaging of Breast Cancer and Metastasis. ACS nano 10 (6), 5959–5970. doi:10.1021/acsnano.6b01326
Zhao, Y., Yuan, X., Liu, B., Tulu, U. S., and Helms, J. A. (2018). Wnt-Responsive Odontoblasts Secrete New Dentin after Superficial Tooth Injury. J. Dent Res. 97 (9), 1047–1054. doi:10.1177/0022034518763151
Keywords: dental pulp, regeneration, immunomodulation, macrophage, nanomaterials, inflammation, cell differentiation
Citation: Yang L, Xiao L, Liu T, Gao W and Xiao Y (2022) Gold Nanoclusters Potentially Facilitate Dentin Regeneration by Functioning Immunomodulation. Front. Mater. 9:612052. doi: 10.3389/fmats.2022.612052
Received: 30 September 2020; Accepted: 25 March 2022;
Published: 28 April 2022.
Edited by:
Elizabeth R. Balmayor, Maastricht University, NetherlandsReviewed by:
Waruna Dissanayaka, The University of Hong Kong, Hong Kong SAR, ChinaDebanjan Sarkar, University at Buffalo, United States
Copyright © 2022 Yang, Xiao, Liu, Gao and Xiao. This is an open-access article distributed under the terms of the Creative Commons Attribution License (CC BY). The use, distribution or reproduction in other forums is permitted, provided the original author(s) and the copyright owner(s) are credited and that the original publication in this journal is cited, in accordance with accepted academic practice. No use, distribution or reproduction is permitted which does not comply with these terms.
*Correspondence: Yin Xiao, eWluLnhpYW9AcXV0LmVkdS5hdQ==
†These authors have contributed equally to this work