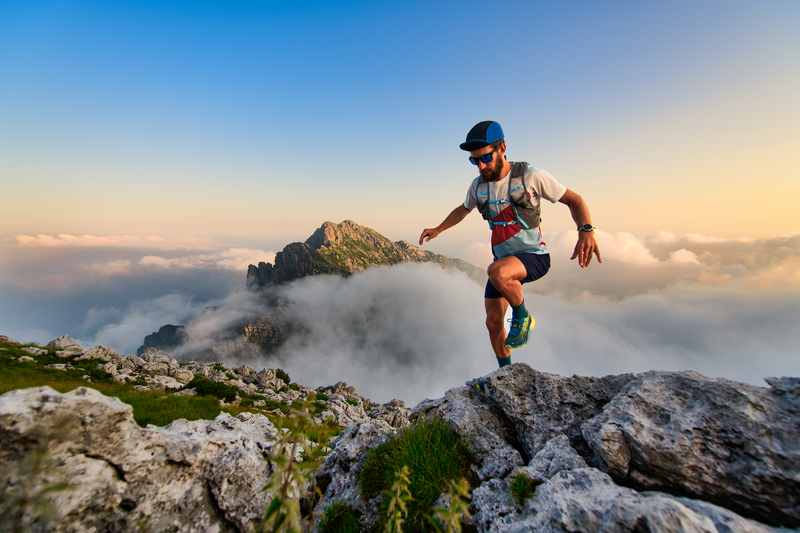
95% of researchers rate our articles as excellent or good
Learn more about the work of our research integrity team to safeguard the quality of each article we publish.
Find out more
ORIGINAL RESEARCH article
Front. Mater. , 09 December 2022
Sec. Polymeric and Composite Materials
Volume 9 - 2022 | https://doi.org/10.3389/fmats.2022.1097547
This article is part of the Research Topic Additive Manufacturing for Polymers View all 10 articles
The industrial ammonia synthesis reaction has the disadvantage of large energy consumption; thus, the electrochemical reduction method of ammonia synthesis characterized by its clean nature and environmental protectiveness has received extensive attention. Molybdenum nitride is a commonly used electrocatalyst for ammonia synthesis, and its Faraday efficiency is low, which may be due to many internal grain boundaries and few active sites. In this work, we grow microscale porous Mo2N single crystals and polycrystalline Mo2N from non-porous MoO3 single crystals. Porous molybdenum nitride materials facilitate charge transport in grain boundaries due to their single-crystal nature and enhance the catalytic properties of ammonia synthesis reactions. Compared with polycrystalline Mo2N, the porous Mo2N single crystal shows better performance, with a high NH4+ yield of 272.56 μg h−1 mg−1 and a Faradaic efficiency of 7.3%. In addition, the porous Mo2N single crystal exhibits superior long-term stability with little attenuation after 16 h electrolysis reaction. It provides a new method for the catalyst of ammonia synthesis.
Shortage of ammonia (NH3), which is used as the raw material for chemical fertilizer, chemical pharmaceuticals, and zero-carbon emission fuel, has become an urgent energy problem with the continuous growth of the population. Fixing N2 in the atmosphere to NH3 is an important method to realize nitrogen storage and the conversion of Earth’s nitrogen cycle (Service, 2014; Brown et al., 2016). Up to now, the Haber–Bosch method (N2 + 3H2 → 2NH3) has been used to synthesize NH3 in the industry, which works at high temperatures and pressure with high energy consumption (Van Der Ham et al., 2014). Most raw materials of hydrogen and energy consumption are obtained through the steam reformation of fossil fuels, while a large amount of CO2 is also produced (Kyriakou et al., 2020). Therefore, the more economical, environmental, and sustainable method of producing NH3 is in great demand.
Electrochemical reduction is a prospective method for artificial nitrogen fixation (Amar et al., 2011; Costentin et al., 2013; She et al., 2017; Guo et al., 2018). However, the N2 reduction reaction (NRR) requires breaking the inert molecular structure of N2, which requires sufficient energy to break the N≡N bond (∼941 kJ mol−1) (Han et al., 2021). Noble metal catalysts (Ru, Au, Ag, and Rh) have shown to have superior NRR activity, but the high cost and rareness limit their commercial applications (Aioub et al., 2017; Bao et al., 2017; Nazemi et al., 2018; Liu et al., 2019; Tao et al., 2019). The search for inexpensive, earth-abundant materials with high activity and excellent stability has recently attracted significant research interest and has become an important pursuit toward ammonia economy. In recent years, transition metal has attracted extensive attention as NRR catalysts because of their high activity, abundant reserves, and low price. It has been reported that molybdenum-based catalysts have been designed and applied to artificial nitrogen fixation (Banerjee et al., 2015; Sun et al., 2017; Wickramasinghe et al., 2017; Yang et al., 2017; Han et al., 2018; Li et al., 2019; Ou et al., 2019). Molybdenum nitride has attracted much attention due to its platinum-like properties. Specifically, Mo2N is a typical interstitial compound in which the metal atoms express a tight stacking pattern to stabilize the lattice structure, where the top element is mainly a metal atom on the surface. The nearest metal ions with short distances will provide the possibility to form metallic bonds to facilitate electron transport in the connected channels. MoN and Mo2N nanosheets also have an electrocatalytic activity of N2 reduction, with the FE of 1.15% and 4.5%, respectively (Ren et al., 2018; Zhang et al., 2018). Although molybdenum-based catalysts have excellent performance, they still suffer from weak FE efficiency. Thus, it is attractive to search for new Mo-based catalysts with enhanced FE performance.
In catalytic and electrocatalytic systems, uniform dispersion of active sites on the surface of porous structures ensures the high activity of porous materials (Zhang et al., 2021; Lu et al., 2022). Single crystals with almost no grain boundary reduce the resistance of charge transmission between grain boundaries. Therefore, porous single crystals with anisotropy and a long-range structural order and structural coherence combined with a large specific surface area provide unsaturated nitrogen coordination on the surface of metal nitride, and its internal structures exhibit regularity and periodicity in three-dimensional space. Due to the porous single-crystal structure, the electrocatalytic properties are also improved (Wu et al., 2019; Zhang et al., 2019; Lin et al., 2020, 2022; Xiao and Xie, 2022). Different from the conventionally used nano-catalyst materials such as nanosheets or nanorods, which are smooth in surface and poor in single crystallinity, porous single crystals have a large amount of nanopores and good crystallinity which increase the active site and improve the stability during the reaction, thus improving the catalytic performance of the catalyst.
In this paper, we prepare the micron-scale porous Mo2N single crystal (PSC Mo2N) and polycrystalline Mo2N (PPC Mo2N) cubes by the lattice reconstruction strategy and investigate their catalytic performance in the electrocatalytic reduction of N2 to NH3. PSC Mo2N can provide a large surface area for chemical adsorption, and the internal interconnected pores and channels are conducive to the diffusion of ions in the electrolyte. PSC Mo2N exhibits ultra-high yield of NH3 and FE, which greatly enhances the catalytic performance of NRR.
The MoO3-PEG precursor is obtained by the hydrothermal method. MoO3-PEG is kept at 300°C for 5 h in air and cooled down naturally to obtain MoO3. PSC Mo2N is obtained in a tube furnace at 650°C for 10 h at a pressure of 50–200 Torr with 100 sccm NH3 gas flow rates. Similarly, irregular porous polycrystalline molybdenum nitride (PPC Mo2N) is obtained by direct treatment of MoO3 single crystals in a tube furnace at atmospheric pressure with NH3 gas at 50–200 sccm for 10 h at 650°C. All reagents are used without any purification.
An X-ray diffractometer (XRD, Bruker D8-Advance) is used to characterize the phase composition of synthetic materials and determine the crystal face and crystal orientation. The surface morphologies of MoO3 and Mo2N are observed by field emission scanning electron microscopy (FE-SEM, SU8010) with 10 kV accelerating voltage. The Tecnai F30 microscope with 200 kV accelerating voltage is tested for transmission electron microscopy (TEM) and selected area electron diffraction (SAED). TEM (FEI Titan 3G2 60-300) is corrected by the spherical aberration method at 300 kV accelerating voltage, and the atomic information and crystal structure of the material are characterized. The Brunauer–Emmett–Teller (BET) tests are performed by an automatic specific surface and micropore analyzer (Tristar II 3020). X-ray photoelectron spectroscopy (XPS, Escalab 250Xi) tests the binding energy of the crystals. The product NH4+ is quantified by cation chromatography (IC 1826 Qingdao, Shunyuhengping).
The catalyst samples are mixed in ethanol and Nafion solution and sonicated for 30 min to prepare a homogeneous suspension of the catalyst. The prepared suspension is then dropped on a piece of carbon paper (CP) to obtain a 5 mg cm−2 sample, which is dried naturally at room temperature to acquire the working electrode.
Electrochemical data are obtained by testing with the three-electrode system (Zahner, Ennium) of the chemical workstation. Electrocatalytic NRR is a typical hydrogen reduction process. The study is performed in a closed H-shaped vessel with the middle section separated by a Nafion 117 membrane. The electrochemical workstation is operated at room temperature with Mo2N (Mo2N/CP) as the working electrode, carbon paper as the substrate, a graphite electrode as the counter electrode, and a saturated Ag/AgCl electrode as the reference electrode, as well as 0.1 M HCl solution as the electrolyte. With the help of N2 and H+, NH3 is obtained at the cathode. Two-compartment batteries can avoid NH3 oxidation in the anode. In particular, the resistance of the Nafion 117 membrane is negligible. The Nafion 117 membrane only transmits H+, which can limit the diffusion of NH3 to the anode. The potential is calibrated and converted into a reversible hydrogen electrode (RHE) using the following formulas (Ren et al., 2018).
where E (RHE) is the corrected potential (V) versus RHE, E (Ag/AgCl) is the experimental test potential with Ag/AgCl as the reference electrode, and 0.197 V is the potential at room temperature at the standard electrode for the Ag/AgCl electrode.
We simultaneously collected products under different potential conditions ranging from −0.3 to −0.8 V for 2 h. The concentration of NH4+ is tested by cation chromatography. The generation rate of NH4+ and the Faradaic efficiency (FE) of the current are calculated by the formula and plotted. Among them, the calculation of the NH3 generation rate and FE is as follows:
where [NH4+] is the result of measuring the NH4+ concentration, V is the volume of the electrolyte at the cathode, m is the loaded mass of the catalyst, t is the time of potential application, Q is the Coulomb volume through the electrode, and F is the Faraday constant.
Linear sweep voltammetry (LSV) is applied to estimate the catalytic performance and stability of OER at 10 mV s−1 in 0.1 M HCl. We measure a three-electrode cell system whose electrochemical impedance spectrum (EIS) is measured between 0.1 Hz and 2 MHz at 0.1 M hydrochloric acid and 5 mV AC potential. The current density curve is obtained from the variation of Mo2N of NRR with time at various potentials in 0.1 M HCl.
Figure 1A shows the XRD patterns of MoO3, PSC Mo2N, and PPC Mo2N. The XRD patterns of MoO3 and Mo2N single crystals conform to the JCPDS standard cards 75-0512 and 75-1150, respectively. The diffraction peaks are sharp and narrow, indicating that molybdenum nitride has excellent crystallinity. From a polycrystalline versus a single crystal perspective, molybdenum nitride single crystals tend to be [111] oriented. The inset in Figure 1A is ball-and-stick model of the crystal structure of Mo2N. Supplementary Figure S1 illustrates the growth mechanism for obtaining micron-scale metal nitride cubes from metal oxides. When the intrinsic oxygen atoms in the MoO3 lattice are heated in an ammonia atmosphere, the oxygen atoms gradually leave the intrinsic position in the lattice and slowly disappear. The nitrogen atom in ammonia gradually enters the position before the oxygen atom. Due to the different sizes of oxygen and nitrogen atoms, the lattice configuration also changes, and then, the molybdenum nitride skeleton is gradually formed. By changing the ammonia flow rate and pressure, the molybdenum nitride lattice is reconstructed to form a porous Mo2N cube.
FIGURE 1. (A) XRD patterns of MoO3 microcubes, Mo2N porous single crystals, and polycrystalline microcubes. The inset in (A) is the crystal structure of Mo2N. (B) Mo2N of XPS spectra. (C) EDX elemental mapping of Mo and N in Mo2N microcubes. SEM of (D) MoO3, (E) PSC Mo2N, and (F) PPC Mo2N. The inserted image of (E) is a partial amplification of the SEM image.
To explore the relationship between the structure and properties of porous single-crystal molybdenum nitride, we perform XPS tests. The test results are shown in Figure 1B; the results specifically show that the characteristic peaks at 229.8 and 232.3 eV are caused by Mo 3d5/2 and Mo 3d3/2 (Ozkan et al., 1997; Afanasiev, 2002; Liu et al., 2013). In addition, the combining energies at 228.9 and 235.9 eV are consistent with Moδ+ and Mo6+, respectively, which is ascribed to the surface oxidation of Mo2N (Yoon et al., 2012). The peak at 397.81 eV is the N 1 s of Mo2N, while the peak value of 394.77 eV corresponds to the shoulder of Mo3p3/2 with Mo2N, indicating the presence of Mo–N bonds (Ozkan et al., 1997; Afanasiev, 2002; Yoon et al., 2012; Liu et al., 2013). To further confirm the presence of chemical elements in Mo2N, energy dispersive X-ray (EDX) and its mapping are used for further identification (Figure 1C). The results indicate that only two chemical elements (Mo and N) can be detected in the final product Mo2N, and no other impurity phases are observed. Moreover, the chemical elements Mo and N are uniformly distributed in the sample, which confirms well the complete transformation process of the non-porous MoO3 single crystal.
We observe the changes in the microscopic morphology of the crystals during the growth and preparation processes by SEM, and Figure 1D shows the MoO3 cubes of about 3–10 μm obtained after calcination of the precursor MoO3-PEG. Figures 1E,F display the microcube scanning diagram of PSC Mo2N and PPC Mo2N formed by ammoniation of MoO3, which have the same morphology before and after calcination from the microscopic scale. In addition, Figure 1E reveals the magnified morphology, and it can be seen that the pore channels are uniformly connected roughly around 50 nm, while the polycrystalline surface is in a sintered state with some cracks. Therefore, we speculate that the specific surface area of single crystals should be larger than that of polycrystals. We use a lattice reconstruction strategy to grow porous molybdenum nitride from non-porous molybdenum nitride, whose BET results (Supplementary Figure S2) indicate that the BET-specific surface areas of PSC Mo2N and PPC Mo2N are 14.347 and 7.676 m2 g−1, respectively. The larger specific surface area has more active sites, which is consistent with our subsequent test results (PSC Mo2N > PPC Mo2N).
For the purpose of obtaining further information about the microstructure and crystal orientation of the synthesized material, TEM and HRTEM are used for characterization and analysis. Figure 2A demonstrates that the precursor MoO3 has good single crystallinity, where SAED (Figure 2B) corresponds to the (002) plane of MoO3. HRTEM (Figure 2C) indicates a crystal surface spacing of 0.198 nm that corresponds to the (002) face of MoO3, which is in agreement with both results. Mo2N retains the morphology and structure of the precursor, irrespective of it being a single crystal (Figures 1E, 2D) or polycrystalline (Figures 1F, 2G). Figure 2F taken with HRTEM from a single Mo2N cube (Figure 2I) reveals highly resolved lattice stripes between the crystal planes with a crystalline spacing of 0.238 nm associated with the (111) plane of Mo2N, which is consistent with SAED (Figure 2E). This further establishes that the sample has a defined crystal orientation. However, in Figures 2H,I, the SAED is tending to a ring-like pattern, and there are no distinct and regular lattice stripes, which suggests that the sample is polycrystalline.
FIGURE 2. TEM image, SAED pattern, and HRTEM image of the material. (A–C) MoO3 single-crystal microcube, (D–F) PSC Mo2N microcube, and (G–I) PPC Mo2N.
NRR is a prototypical reduction process by hydrogenation. As shown in Figure 3A, N2 bubbles to the cathode surface, and H+ can be transferred through the electrolyte of 0.1 M HCl to react with the cathode Mo2N to form NH3. At first, we test the electrochemical performance of the sample and the catalytic activity of N2. The LSV curves for the reduction reaction (NRR) are obtained by saturating with N2 and Ar in 0.1 M HCl electrolyte (Figure 3B), and it is clear that the current density of N2 increases slightly compared to Ar in the saturated electrolyte, which indicates the potential NRR activity of PSC Mo2N. Apart from that, the AC impedance method is used to verify the electron transfer resistance of molybdenum nitride by fitting the EIS data on the sample to an equivalent circuit. The Nyquist curve (Figure 3C) shows that PSC Mo2N has a smaller resistance than PPC Mo2N and therefore has a higher current for the same voltage conditions applied. This indicates that the porous single crystal is conducive to carrier migration, which has better catalytic properties. All these results clearly indicate that the porous Mo2N single crystals are more effective as electrocatalysts than polycrystals.
FIGURE 3. (A) Schematic diagram of electrocatalytic NRR. (B) LSV curve of PSC Mo2N electrocatalytic NRR in a saturated electrolyte of N2 (red line) and Ar (black line). (C) Nyquist plots of CP, PSC Mo2N, and PPC Mo2N catalysts in 0.1 M HCl solution. The yields of NH4+ and FEs at different potentials of (D) PSC Mo2N and (E) PPC Mo2N. (F) Yields of NH4+ in different catalysts under the same environmental conditions at −0.6 V vs. RHE.
In the next step, we collect the products at different voltages at the same time and analyze their contents by cation chromatography. The results are shown in Figures 3D,E, which exhibit the NH4+ yields and FEs of PSC Mo2N and PPC Mo2N at different voltages. Among them, the catalytic effect of the catalysts is best at −0.6 V, when the NH4+ production rate of PPC Mo2N is 77.78 μg h−1 mg−1 and the FE reached 0.6%, while the NH4+ production rate of PSC Mo2N reached 272.56 μg h−1 mg−1 and the FE reached 7.3%. Obviously, PSC Mo2N implies extremely high catalytic activity compared to PPC Mo2N. The NH4+ yield and FE content increase with the increase in negative voltage; however, the yield of NH4+ and FE decreases significantly when it passes −0.6 V vs. RHE. It is possible that the hydrogen evolution reaction (HER) competes fiercely at the Mo2N electrode to preferentially occupy the active site with a large amount of free H and limit the effective trapping of N2, hindering the forward progress of the NRR reaction. Compared with other water-based catalysts, PSC Mo2N has higher ammonia yield and FE. The specific performance of other catalysts is shown in Supplementary Table S1.
The effect of other impurities in the experiments is excluded by conducting control experiments on the precursor MoO3 and carbon paper on the results. The results demonstrate that the NRR rate of Mo2N in Figure 3F is much higher than that of the MoO3 single crystal (26.21 μg h−1 mg−1), which implies that N plays an important role in the NRR and excludes the influence of surface oxidation on this reaction. At the same time, the bare CP shows weak NH4+ production, which has been subtracted from the starting data to reduce the experimental error. PSC Mo2N and PPC Mo2N have similar morphology and dimensions, but they have different specific surface areas, pore size, and lattice arrangement, leading to different ammonia yields of both. This suggests porous single crystals with large specific surface area and lattice continuity can reduce the energy loss of electron transfer between crystals, which results in higher catalytic activity. Therefore, PSC Mo2N exhibits excellent catalytic performance, which is consistent with the experimental results.
Durability is an important credential for assessing NRR response. Figures 4A,B indicate that the relative current density of PSC Mo2N and PPC Mo2N catalysts reduced to ammonia at different potentials respectively is stable, which shows the excellent durability of catalysts. Moreover, as the cathodic potential increases, the current density slightly decreases and the HER process is enhanced, inhibiting the NRR reaction, which is also consistent with the decrease of NH4+ production and FE. To further evaluate the stability of this catalytic process, we test the samples for 10 cycles at −0.6 V (Figures 4C,D), and the NH4+ production rate and FEs have no significant changes, indicating that they have good stability for NRR. In addition, the effect of a different N2 flow rate on the product is also tested in this electrocatalytic process. As shown in Figures 4E,F, the approximately constant yields of NH4+ and FEs demonstrate that the N2 diffusion rate has no obvious effect on the reaction, which ruled out the effect of the inlet concentration on this reaction. We perform some controlled experiments to verify the reliability of Mo2N/CP for the electrochemical reduction of N2 to NH3. N2 is led into the electrochemical reaction cell under open-circuit conditions, and saturated-Ar and saturated-N2 gas flows are introduced separately for 2 h at −0.6 V vs. RHE, respectively. In summary, the influence of the external environment on the reaction during the experiment is excluded, indicating that the obtained NH3 is derived from Mo2N/CP.
FIGURE 4. Current density curves of (A) PSC Mo2N and (B) PPC Mo2N of NRR with time at different voltages in 0.1 M HCl. The yields of NH4+ and FEs, 10 of (C) PSC Mo2N and (D) PPC Mo2N at −0.6 V vs. RHE potential during the cycle test. The yields of NH4+ and FEs of (E) PSC Mo2N and (F) PPC Mo2N catalysts at different N2 flow rates.
We test the long-term electrolysis process, as shown in Figure 5A, and the current density electrochemical NRR process hardly changes significantly within 16 h, indicating the excellent performance of the Mo2N catalyst. We perform a series of characterizations on the tested PSC Mo2N such as XRD (Figure 5B), XPS (Figure 5C), SEM (Figures 5D,E), EDX (Supplementary Figure S4), and Mapping (Figure 5F). The test results clearly show that the phase composition, valence state, and elemental distribution of the catalyst itself have not changed significantly compared with those before the reaction, indicating that no other impurity phases are generated in the catalyst during the reaction. Further from the microscopic scale, PSC Mo2N is able to maintain its initial composition and original cubic morphology after electrolysis, which also proves that the catalytic process is reproducibly cyclable and stable.
FIGURE 5. (A) Timing ampere curve of PSC Mo2N and PPC Mo2N electrolysis for 16 h at a potential of −0.6 V vs. RHE. (B) XRD of PSC Mo2N and PPC Mo2N after a long-term reaction. (C) Mo2N of the XPS energy spectrum after a long-term reaction. (D) SEM images of different magnifications after the reaction. (E) Magnified view of SEM of PSC Mo2N. (F) EDX elemental mapping of Mo and N in Mo2N microcubes.
The aforementioned results and previous literature reports confirm that the possible NRR mechanism of Mo2N can be described by the Mars–van Krevelen (MvK) process (Supplementary Figure S5) (Abghoui et al., 2016; Yang et al., 2017; Ren et al., 2018; Jin et al., 2019). First, H+ is adsorbed on the N atoms on the surface of Mo2N. Second, N vacancies appear on the catalyst face due to the departure of NH3 molecules. Then, N2 molecules occupy the vacancies, attracting H+ to approach. Finally, NH3 is released from the catalyst surface, and the Mo2N catalyst returned to its original state. A steady stream of hydrogen ions in the electrolyte promotes the cyclic reaction. In the process of reaction, the porous structure increases the reaction area of the N atom and the active site of the reaction. Single-crystal materials are characterized by lattice continuity, so the migration process of N atoms is not blocked by grain boundaries. Compared with polycrystalline materials, it reduces the reaction kinetic energy of N atom migration, promotes the charge transfer of N atoms at the grain boundary, and accelerates the reaction rate. These results indicate that porous single-crystal molybdenum nitride has fine catalytic activity for the NRR reaction.
In summary, we prepare PSC Mo2N and PPC Mo2N. Among them, PSC Mo2N reduces the charge transfer rate between grain boundaries and improves the rate and stability of the catalytic reaction due to its single-crystal nature. Compared with PPC Mo2N, PSC Mo2N exhibits excellent catalytic performance at −0.6 V vs. RHE, resulting in FE and yield of NH4+ up to 7.3% and 272.56 μg h−1 mg−1. In addition, the reaction has superior recyclability and electrochemical durability, which maintains approximately constant yields of NH3 and FE during 10 cycles and hardly weakens after 16 h long-term electrolysis. This work explores a novel avenue for the reasonable design and advancement of the molybdenum-based porous single crystal as the electrocatalysts of the artificial nitrogen fixation reaction.
The original contributions presented in the study are included in the article/Supplementary Material; further inquiries can be directed to the corresponding author.
All authors listed have made a substantial, direct, and intellectual contribution to the work and approved it for publication. Investigation, data curation, software, formal analysis, visualization, writing—original draft: XL, Funding acquisition, project operation, writing review and editing: KX.
This work was supported by the National Key Research and Development Program of China (2021YFA1501500), the Natural Science Foundation of China (91845202), and the Strategic Priority Research Program of Chinese Academy of Sciences (XDB2000000).
Author KX was employed by the Advanced Energy Science and Technology Guangdong Laboratory.
The remaining author declares that the research was conducted in the absence of any commercial or financial relationships that could be construed as a potential conflict of interest.
All claims expressed in this article are solely those of the authors and do not necessarily represent those of their affiliated organizations, or those of the publisher, the editors, and the reviewers. Any product that may be evaluated in this article, or claim that may be made by its manufacturer, is not guaranteed or endorsed by the publisher.
The Supplementary Material for this article can be found online at the following link: https://www.frontiersin.org/articles/10.3389/fmats.2022.1097547/full#supplementary-material
Abghoui, Y., Garden, A. L., Howalt, J. G., Vegge, T., and Skúlason, E. (2016). Electroreduction of N2 to ammonia at ambient conditions on mononitrides of Zr, Nb, Cr, and V: A dft guide for experiments. ACS Catal. 6, 635–646. doi:10.1021/acscatal.5b01918
Afanasiev, P. (2002). New single source route to the molybdenum nitride Mo2N. Inorg. Chem. 41, 5317–5319. doi:10.1021/ic025564d
Aioub, M., Panikkanvalappil, S. R., and El-Sayed, M. A. (2017). Platinum-Coated gold nanorods: Efficient reactive oxygen scavengers that prevent oxidative damage toward healthy, untreated cells during plasmonic photothermal therapy. ACS Nano 11, 579–586. doi:10.1021/acsnano.6b06651
Amar, I. A., Lan, R., Petit, C. T. G., and Tao, S. (2011). Solid-state electrochemical synthesis of ammonia: A review. J. Solid State Electrochem. 15, 1845–1860. doi:10.1007/s10008-011-1376-x
Banerjee, A., Yuhas, B. D., Margulies, E. A., Zhang, Y., Shim, Y., Wasielewski, M. R., et al. (2015). Photochemical nitrogen conversion to ammonia in ambient conditions with femos-chalcogels. J. Am. Chem. Soc. 137, 2030–2034. doi:10.1021/ja512491v
Bao, D., Zhang, Q., Meng, F. L., Zhong, H. X., Shi, M. M., Zhang, Y., et al. (2017). Electrochemical reduction of N2 under ambient conditions for artificial N2Fixation and renewable energy storage using N2/NH3 cycle. Adv. Mat. 29, 1604799. doi:10.1002/adma.201604799
Brown, K. A., Harris, D. F., Wilker, M. B., Rasmussen, A., Khadka, N., Hamby, H., et al. (2016). Light-driven dinitrogen reduction catalyzed by a CdS:nitrogenase MoFe protein biohybrid. Science 352, 448–450. doi:10.1126/science.aaf2091
Costentin, C., Robert, M., and Savéant, J. M. (2013). Catalysis of the electrochemical reduction of carbon dioxide. Chem. Soc. Rev. 42, 2423–2436. doi:10.1039/c2cs35360a
Guo, C., Ran, J., Vasileff, A., and Qiao, S. Z. (2018). Rational design of electrocatalysts and photo(electro)catalysts for nitrogen reduction to ammonia (NH3) under ambient conditions. Energy Environ. Sci. 11, 45–56. doi:10.1039/c7ee02220d
Han, D., Liu, X., Cai, J., Xie, Y., Niu, S., Wu, Y., et al. (2021). Superior surface electron energy level endows WP2 nanowire arrays with N2 fixation functions. J. Energy Chem. 59, 55–62. doi:10.1016/j.jechem.2020.11.006
Han, J., Ji, X., Ren, X., Cui, G., Li, L., Xie, F., et al. (2018). MoO3 nanosheets for efficient electrocatalytic N2 fixation to NH3. J. Mat. Chem. A 6, 12974–12977. doi:10.1039/c8ta03974g
Jin, H., Li, L., Liu, X., Tang, C., Xu, W., Chen, S., et al. (2019). Nitrogen vacancies on 2D layered W2N3: A stable and efficient active site for nitrogen reduction reaction. Adv. Mat. 31, 1902709. doi:10.1002/adma.201902709
Kyriakou, V., Garagounis, I., Vourros, A., Vasileiou, E., and Stoukides, M. (2020). An electrochemical haber-bosch process. Joule 4, 142–158. doi:10.1016/j.joule.2019.10.006
Li, X., Ren, X., Liu, X., Zhao, J., Sun, X., Zhang, Y., et al. (2019). A MoS2 nanosheet-reduced graphene oxide hybrid: An efficient electrocatalyst for electrocatalytic N2 reduction to NH3 under ambient conditions. J. Mat. Chem. A 7, 2524–2528. doi:10.1039/c8ta10433f
Lin, G., Li, H., and Xie, K. (2022). Identifying and engineering active sites at the surface of porous single-crystalline oxide monoliths to enhance catalytic activity and stability. CCS Chem. 4, 1441–1451. doi:10.31635/ccschem.021.202000740
Lin, G., Li, H., and Xie, K. (2020). Twisted surfaces in porous single crystals to deliver enhanced catalytic activity and stability. Angew. Chem. Int. Ed. 59, 16440–16444. doi:10.1002/anie.202006299
Liu, G., Cui, Z., Han, M., Zhang, S., Zhao, C., Chen, C., et al. (2019). Ambient electrosynthesis of ammonia on a core–shell-structured Au@CeO2 catalyst: Contribution of oxygen vacancies in CeO2. Chem. Eur. J. 25, 5904–5911. doi:10.1002/chem.201806377
Liu, J., Tang, S., Lu, Y., Cai, G., Liang, S., Wang, W., et al. (2013). Synthesis of Mo2N nanolayer coated MoO2 hollow nanostructures as high-performance anode materials for lithium-ion batteries. Energy Environ. Sci. 6, 2691–2697. doi:10.1039/c3ee41006d
Lu, Z., Zhou, G., Li, B., Xu, Y., Wang, P., Yan, H., et al. (2022). Heterotopic reaction strategy for enhancing selective reduction and synergistic oxidation ability through trapping Cr (VI) into specific reaction site: A stable and self-cleaning ion imprinted CdS/htnw photocatalytic membrane. Appl. Catal. B Environ. 301, 120787. doi:10.1016/j.apcatb.2021.120787
Nazemi, M., Panikkanvalappil, S. R., and El-Sayed, M. A. (2018). Enhancing the rate of electrochemical nitrogen reduction reaction for ammonia synthesis under ambient conditions using hollow gold nanocages. Nano Energy 49, 316–323. doi:10.1016/j.nanoen.2018.04.039
Ou, P., Zhou, X., Meng, F., Chen, C., Chen, Y., and Song, J. (2019). Single molybdenum center supported on N-doped black phosphorus as an efficient electrocatalyst for nitrogen fixation. Nanoscale 11, 13600–13611. doi:10.1039/c9nr02586c
Ozkan, U. S., Zhang, L., and Clark, P. A. (1997). Performance and postreaction characterization of γ-Mo2N catalysts in simultaneous hydrodesulfurization and hydrodenitrogenation reactions. J. Catal. 172, 294–306. doi:10.1006/jcat.1997.1873
Ren, X., Cui, G., Chen, L., Xie, F., Wei, Q., Tian, Z., et al. (2018). Electrochemical N2 fixation to NH3 under ambient conditions: Mo2N nanorod as a highly efficient and selective catalyst. Chem. Commun. 54, 8474–8477. doi:10.1039/c8cc03627f
Service, R. F. (2014). New recipe produces ammonia from air, water, and sunlight. Science 80345, 610. doi:10.1126/science.345.6197.610
She, Z. W., Kibsgaard, J., Dickens, C. F., Chorkendorff, I., Nørskov, J. K., and Jaramillo, T. F. (2017). Combining theory and experiment in electrocatalysis: Insights into materials design. Science 80, eaad4998. doi:10.1126/science.aad4998
Sun, S., Li, X., Wang, W., Zhang, L., and Sun, X. (2017). Photocatalytic robust solar energy reduction of dinitrogen to ammonia on ultrathin MoS2. Appl. Catal. B Environ. 200, 323–329. doi:10.1016/j.apcatb.2016.07.025
Tao, H., Choi, C., Ding, L. X., Jiang, Z., Han, Z., Jia, M., et al. (2019). Nitrogen fixation by Ru single-atom electrocatalytic reduction. Chem 5, 204–214. doi:10.1016/j.chempr.2018.10.007
Van Der Ham, C. J. M., Koper, M. T. M., and Hetterscheid, D. G. H. (2014). Challenges in reduction of dinitrogen by proton and electron transfer. Chem. Soc. Rev. 43, 5183–5191. doi:10.1039/c4cs00085d
Wickramasinghe, L. A., Ogawa, T., Schrock, R. R., and Müller, P. (2017). Reduction of dinitrogen to ammonia catalyzed by molybdenum diamido complexes. J. Am. Chem. Soc. 139, 9132–9135. doi:10.1021/jacs.7b04800
Wu, D., Wang, Y., Ma, N., Cao, K., Zhang, W., Chen, J., et al. (2019). Single-crystal-like ZnO mesoporous spheres derived from metal organic framework delivering high electron mobility for enhanced energy conversion and storage performances. Electrochim. Acta 305, 474–483. doi:10.1016/j.electacta.2019.03.077
Xiao, Y., and Xie, K. (2022). Active exsolved metal–oxide interfaces in porous single-crystalline ceria monoliths for efficient and durable CH4/CO2 reforming. Angew. Chem. Int. Ed. Engl. 61, e202113079. doi:10.1002/anie.202113079
Yang, D., Chen, T., and Wang, Z. (2017). Electrochemical reduction of aqueous nitrogen (N2) at a low overpotential on (110)-oriented Mo nanofilm. J. Mat. Chem. A 5, 18967–18971. doi:10.1039/c7ta06139k
Yoon, S., Jung, K. N., Jin, C. S., and Shin, K. H. (2012). Synthesis of nitrided MoO2 and its application as anode materials for lithium-ion batteries. J. Alloys Compd. 536, 179–183. doi:10.1016/j.jallcom.2012.04.116
Zhang, F., Xi, S., Lin, G., Hu, X., Lou, X. W., David), , and Xie, K. (2019). Metallic porous iron nitride and tantalum nitride single crystals with enhanced electrocatalysis performance. Adv. Mat. 31, 1806552. doi:10.1002/adma.201806552
Zhang, L., Ji, X., Ren, X., Luo, Y., Shi, X., Asiri, A. M., et al. (2018). Efficient electrochemical N2 reduction to NH3 on Mon nanosheets Array under ambient conditions. ACS Sustain. Chem. Eng. 6, 9550–9554. doi:10.1021/acssuschemeng.8b01438
Keywords: porous single crystal, molybdenum nitride, electrochemical reactions, ammonia, hydrothermal method
Citation: Li X and Xie K (2022) Porous single-crystalline molybdenum nitride enhances electroreduction of nitrogen to ammonia. Front. Mater. 9:1097547. doi: 10.3389/fmats.2022.1097547
Received: 14 November 2022; Accepted: 24 November 2022;
Published: 09 December 2022.
Edited by:
Jingjun Wu, Zhejiang University, ChinaReviewed by:
Xiaozhen Zhang, Jingdezhen Ceramic Institute, ChinaCopyright © 2022 Li and Xie. This is an open-access article distributed under the terms of the Creative Commons Attribution License (CC BY). The use, distribution or reproduction in other forums is permitted, provided the original author(s) and the copyright owner(s) are credited and that the original publication in this journal is cited, in accordance with accepted academic practice. No use, distribution or reproduction is permitted which does not comply with these terms.
*Correspondence: Kui Xie, a3hpZUBmamlyc20uYWMuY24=
Disclaimer: All claims expressed in this article are solely those of the authors and do not necessarily represent those of their affiliated organizations, or those of the publisher, the editors and the reviewers. Any product that may be evaluated in this article or claim that may be made by its manufacturer is not guaranteed or endorsed by the publisher.
Research integrity at Frontiers
Learn more about the work of our research integrity team to safeguard the quality of each article we publish.