- 1Key Laboratory of Design and Assembly of Functional Nanostructures, Fujian Provincial Key Laboratory of Nanomaterials, Fujian Institute of Research on the Structure of Matter, Chinese Academy of Sciences, Fuzhou, China
- 2CAS Haixi Industrial Technology Innovation Center, Ningbo, China
- 3University of Chinese Academy of Sciences, Beijing, China
Thermoplastic foam additive manufacturing is a brand-new industry that perfectly combines the advantages of polymer foaming with AM. The 3D printing industry currently suffers from limited available materials and monolithic part manufacturing, and 3D printed foam offers a new way of thinking to address these challenges. Designing multifunctional components with additive manufacturing gives designers great flexibility, while foaming reduces the weight of materials and costs. The combination of the two allows for the creation of lightweight structural and functional items with differentiated physical properties. This one-of-a-kind and innovative approach can be achieved in the printed section. 3D printing foam, on the other hand, is still in its infancy. This review examines the respective functions and applications of additive manufacturing and foaming, and then attempts to summarize four commonly used 3D printing methods at this stage:1) cellular scaffolds; 2) composite printing foam; 3) post-foaming of printed solid scaffolds; 4) in-situ foam 3D printing. Among these methods, in-situ foam 3D printing is the technique that properly merges the foaming and fused filament fabrication processes. Although in the early stages of research and not yet fully established, this foam 3D printing technique seems to be the trend to replace other foaming processes.
1 Introduction
In today’s increasingly energy-constrained world, polymer foam products are attracting widespread attention for their material-saving and low-cost qualities, as well as their good mechanical and thermal stability, low thermal conductivity and excellent dielectric properties (Di Maio and Kiran, 2018). The design concept of thermoplastic foaming perfectly fits the human pursuit of high-performance materials by improving the mechanical properties of materials while decreasing material density. Nevertheless, each high-performance thermoplastic foam has a relatively single function, and the addition of micro- and nano-nucleating agents is an effective technique to improve the foam structure and give it better mechanical properties. With the development of high-end fields such as building materials, automotive, aerospace and military industry, thermoplastic polymer foam materials and products are also facing new challenges (Ling et al., 2013; Costeux, 2014; Forest et al., 2015). The accuracy and performance of the foam produced by traditional foam molding technology can no longer meet the increasingly stringent requirements. The foam produced by the microcellular foam molding process has smaller pore sizes than the original defects or microscopic cracks in conventional foam. These micro-pores can blunt the cracks in the original material and improve the mechanical properties of the material by increasing the strength of the plastic.
Additive manufacturing is an emerging manufacturing technology that uses digital models (Shmueli et al., 2019; Narupai and Nelson, 2020) as a basis to create solid objects from materials in a layer-by-layer build-up (Nadgorny and Ameli, 2018). Because additive manufacturing does not require the process planning of subtractive manufacturing, it largely simplifies the process of producing complex parts. This has overturned the traditional manufacturing concepts and models, and has had a profound impact on the transformation of traditional manufacturing into modern manufacturing.
The current microcellular foaming methods generally include batch foaming method, rapid temperature rise method, rapid pressure reduction method, continuous extrusion foaming method, injection molding foaming method, etc. Here, we propose that additive manufacturing technology can also be used as a novel microcellular foam molding process. Combined with seemingly unrelated concepts of thermoplastic foaming and additive manufacturing, it enables the development of unique engineered materials with a variety of geometric shapes and specific physical properties. Figure 1 depicts the respective advantages of additive manufacturing and foaming. It is believed that 3D printing foaming technology will become an advanced technology with broad application prospects. This review will focus on these two topics (Attaran, 2017).
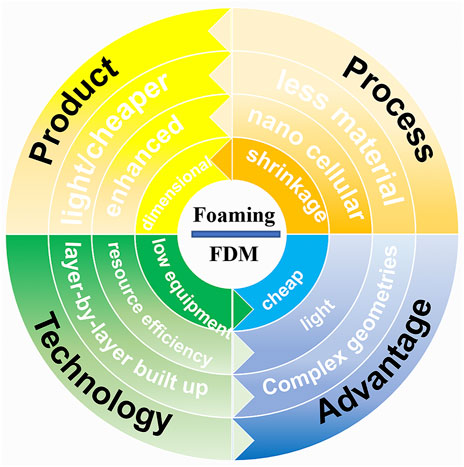
FIGURE 1. The huge advantages of the combination of additive manufacturing and foaming are expected to make it an epoch-making manufacturing process.
2 Additive manufacturing and foaming are two relatively independent technologies
2.1 Additive manufacturing
2.1.1 3D printing methods
Often referred to as 3D printing, additive manufacturing (AM) was initially created in the 1980s to address the extremely specific demands of model creation and rapid prototyping (RP). Since then, it has evolved into a versatile technological platform for rapid prototyping and computer-assisted design (CAD) (Hofmann, 2014). A variety of techniques that quickly and easily convert virtual solid model data into physical model are what constitute additive manufacturing (Rashid, 2019). Without the requirement for molds or machining, which are typical of traditional forming and subtractive manufacturing, it allows custom components to be made from metals (Yang et al., 2022), ceramics (Felzmann et al., 2012; Franchin et al., 2022) and polymers. Polymer-based additive manufacturing can be roughly classified into four categories, each of which has its own technology. Among them, polymers are undoubtedly the most frequently used additive manufacturing material category. Table 1 summarizes the advantages and disadvantages of AM classification methods for polymers. The first additive manufacturing technique is photopolymerization, in which a liquid photopolymer in a vat is selectively cured by photoactivated polymerization (Kaiser and Chalfin, 2017). Stereolithography (SLA) (Credi et al., 2016; Palaganas et al., 2017; Voet et al., 2018), digital light processing (DLP) (Ligon-Auer et al., 2016; Zhu et al., 2017, 2018), continuous liquid interface production (CLIP) (Tumbleston et al., 2015; Janusziewicz et al., 2016) and multiphoton polymerization (MPP) (Nikolić and Stevanović, 2006) etc., All fall into this category. In stereolithography, the light source may be either a laser or a projector in digital processing of the light (Borrello et al., 2018). The second technique is called powder bed fusion technology (PFT), where the polymer is delivered towards the print bed as a powder layer and then portions of the powder bed are thermally fused. Selective laser sintering (SLS) also belongs to this technology, as defined in the standard text of ASTM F2792-12 (Kaiser and Chalfin, 2017).The technique can be applied in cores or molds for manufacturing facilities made of various metal alloys as well as organic materials like sand. The powder bed used in the majority of SLS applications is made of thermoplastic polymers, especially different types of polyamide like PA11 and PA12 (Hofmann, 2014). The third technique is called material jetting, in which UV crosslinking is employed to selectively deposit viscous droplets of photopolymers (Udroiu et al., 2019). Material extrusion is the final significant additive manufacturing technique (Schirmeister et al., 2019). This technique is based on the original fused filament manufacturing (FFF) process and relies on a simple design using thermoplastic materials that appear to be essentially industry standard. (Li et al., 2020). The idea of melting a “typical” plastic product and extruding it via a narrow, heated nozzle seems to be quite straightforward as shown in the schematic of Figure 2 (Hofmann, 2014). This allows the extruded melt to deposit in the X-Y control motion of the anterior layer of the manufactured element (Rashid, 2019).
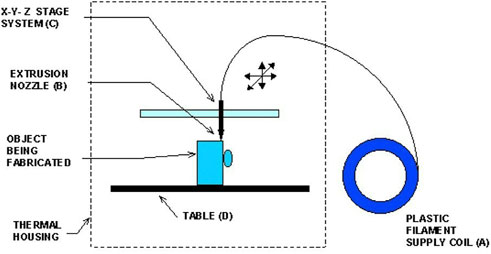
FIGURE 2. The popular FFF procedure uses an X-Y-Z-mobile extrusion head to apply thermoplastic lines. Copyright 2014, American Chemical Society.
Additive manufacturing technology plays an important role in meeting the needs of individual production, overcoming many of the long-standing limitations of traditional processes. However, the technology still has certain shortcomings. For example, models printed with ABS or PET have rough details and poor fineness (Fu et al., 2021). Multiple material substitution combinations are required to produce fine print details. In addition, the SLS powder sintering process requires additional curing of the printed object; otherwise, it tends to cause liquid absorption and staining of the porous surface Wang et al. (2020b). Due to the limitations of material properties, additive manufacturing is still unable to accurately replace real products and remains more at the stage of simulating products. On the other hand, additive manufacturing is not very efficient. For the 3D printers commonly used in the market, a three-dimensional plastic model the size of a puck requires 28 h (Stansbury and Idacavage, 2016) of continuous machine work. The development of lightweight thermoplastic polymer foams enables researchers to quickly print small-scale parts before expanding them. An innovative and creative manufacturing process is created by the successful combination of polymer foaming with additive manufacturing, and thermoplastic polymer foam can be produced by SLA (Wirth et al., 2020), FFF and direct ink writing (Chen et al. (2018a), 2019), etc. Of these combined foaming processes, the SLA process is available for fewer materials because it requires a higher cure rate. In addition, SLA relies on the combination of cured polymers with soluble components for post-processing to form honeycomb structures (Wirth et al., 2020). In contrast, the FFF process is well suited to thermoplastic foams due to its low system cost, wide material selection, and improved toughness. The FFF technologies will be highlighted in this review.
2.1.2 Introduction and application of fused filament manufacturing
Since Scott Crump created fused deposition modeling (FDM) and received a patent for it in 1989, the term “fused filament fabrication” (FFF) is widely used as a replacement (Schmitt et al., 2017). A fusion of thermoplastic material is extruded from the nozzle by heating and then the material is selectively printed on the platform according to the predetermined trajectory of the model slice (Zhang et al., 2020). The raw material, which is targeted at the inside of the hot-end, is bought on reels of filament with a 1.75 or 3 mm diameter (Eltorai et al., 2015). For amorphous or semi-crystalline polymers, the extruder is heated to a polymer-appropriate process temperature, which is either above the glass or melting temperature for semi-crystalline polymers (Nadiyapara and Pande, 2017). For a successful construction procedure, the CAD geometry parameters for a particular filament, build speed, processing temperature, and polymer melt rheology must all be carefully balanced (Pérez et al., 2018). The various elements that affect the performance of FFF printers are graphically represented in Figure 3. On the other hand, there are many challenges in implementing the FFF process to achieve thermoplastic foaming. Since it is printed in one piece, the structure used for support may also be embedded inside the model during the printing process and cannot be removed. At the same time, human manipulation can lead to warping, deformation, etc. Hwang et al. effectively solves the deformation problem due to the thermal expansion of the material by embedding metal particles into the polymer (Hwang et al., 2015). In addition to the control of deformation problems, Kokkinis et al. developed a magnetically assisted 3D printing platform in terms of workpiece anisotropy (Kokkinis et al., 2015). They achieved particle orientation control by adding magnetized aluminum platelets to a polymer matrix. Owing to the arrangement of anisotropic particles, the target performance of the workpiece in a specific direction was improved (Liu et al., 2022). Another problem with the FFF process is that the printed part contains a certain amount of porosity that can adversely affect the final mechanical properties (Kakumanu and Srinivas Sundarram, 2018). Even though the technology can achieve high precision in theory, it is still very difficult to manufacture in practice.
Because it can print objects made of various material types and then alter the print substance, FFF has the obvious benefit of allowing for more users control over device manufacturing for experimental applications (Weng et al., 2016). FFF is used as a tool for bioengineering tissue, with components ranging from bones or teeth to blood vessels or organ scaffolding. FFF is being used in a multitude of ways in the building industry (Zhang et al., 2021). In contrast to complicated geometries, FFF provides design freedom that aids in the optimization of design models that enable higher functional integration and lower material waste. Biomimetic actuators, such as worm-like and flower-like actuators, are examples of intricate magnetic actuators with complex topologies. FFF has been successfully applied to these actuators (Qi et al., 2020). In conclusion, additive manufacturing is becoming increasingly prevalent outside of specialized industries and is currently used in a variety of fields, including lightweight engineering, energy technology, pharmaceuticals and even food production. It performs significantly more than just its conventional role in rapid prototyping, rapid tooling, and concept modeling (Gross et al., 2014).
2.2 Foaming
2.2.1 Foaming and foaming mechanism in polymers
The concept of polymer foams was originally proposed in the hope of introducing a large number of voids into the polymer matrix that were smaller than the defects already existed in the polymer. Therefore, the rigidity of the product can be improved while the quality of the product is reduced, and other properties such as strength are not significantly affected. Polymer foam materials, as compared to non-foamed materials, have certain good qualities like high impact strength, extended fatigue life, high toughness (Zhai et al., 2022), strong thermal stability (Wicklein et al., 2015; Hu et al., 2019), low thermal conductivity (Ji et al., 2013) and dielectric constant (Wu et al., 2013), etc. Polymeric foams can be generated using a variety of techniques, including physical blowing agents (PBA) (Sauceau et al., 2011; Zhai et al., 2022) and chemical blowing agents (CBA) (Sadik et al., 2018). Thermally expandable microspheres (TEMs) (Diani and Gall, 2006; Peng et al., 2013; Zhang et al., 2019) have received increasing attention as a type of PBA. The polymer foam molding process generally includes three basic stages: the formation stage of the polymer/gas homogeneous system; the nucleation stage of the bubbles; and the growth and shaping stage of the cells, as shown in Figure 4. At first, the gas diffuses and disperses into the polymer under specific conditions, and after a certain time it reaches thermodynamic equilibrium to form a homogeneous system; changing the temperature or pressure causes the gas solubility in the homogeneous system to drop quickly, resulting in a supersaturated state. Numerous microscopic bubbles are generated in the system as it tends to revert to a low-energy steady state. The system’s pressure differential between the inner and outer the bubbles increases as the system’s gas solubility is further reduced, which causes the bubbles to grow and expand. Afterward, the system is cooled and shaped to take on the final cell shape and structure.
2.2.2 Polymer foam technology
In order to manufacture polymer foam materials, the foam molding process can be separated into batch autoclave foaming (Yeh et al., 2017), continuous extrusion foaming (Lee et al., 2008; Sauceau et al., 2011), and injection molding foaming (Ameli et al., 2014; Villamil Jiménez et al., 2020), depending on the degree of continuous operation. Table 2 lists the various foaming techniques in alphabetical order. Batch autoclave foaming is typically performed in a high-pressure reactor, which provides the sample being foamed with a specific high temperature and high pressure airtight environment, allowing gases like CO2 and N2 to gradually diffuse and dissolve into the polymer matrix. Cell nucleation develops and finally takes shape as the thermodynamic conditions change. Anson Wong (Wong et al., 2011) and colleagues examined the foaming behavior of semi-crystalline polymers and discovered that samples using the Wessling method, including HDPE, PP, and PET, showed varying degrees of crystallization. They discovered that the dissolution slope reduced as crystallinity increased. This indicates that the amorphous area of the polymer is the sole place where adsorption takes place. Due to the semi-continuous foaming process, the diffusion of the gas in the polymer matrix can take place at a much higher rate and efficiency (Yu et al., 2015). Kuma et al. (Kumar et al., 2000) proposed a method for producing solid-phase PET foamed sheets using a semi-continuous method in response to the drawbacks of discontinuous methods for producing polymeric micro-foam materials. After the gas had been saturated, the separator layer was removed with a heating device and then foamed. The last foaming technology is continuous foaming, which is currently the most popular polymer foaming technology (Rizvi et al., 2018; Li et al., 2021; Gunasekaran et al., 2022a). Supercritical CO2 can significantly reduce the time required for polymers to reach saturation, allowing for the production of microporous polymers in an industrial environment (Yeh et al., 2017; Hu et al., 2021). Therefore, it is imperative to provide a continuous preparation technology for micro-foamed materials based on established plastic processing techniques like extrusion (Nofar, 2016; Rizvi et al., 2018) and injection molding (Jahani et al., 2014, 2015; Contreras et al., 2020).
3 Approaches to generating cellular structures through additive manufacturing
When solids are converted into cellular materials, their single-valued qualities are enlarged (Ashby, 2006). Properties include stiffness, strength, thermal conductivity and diffusivity, electrical resistivity, (Wang et al. 2(2020a); Nofar et al., 2022) all of which have numerous potential applications in the areas of personal thermal management (Hu et al., 2020; Zhou and Hsieh, 2020), IR stealth (Ahn et al., 2019), superhydrophobic surfaces (Wu et al., 2020), and sensors (Hu et al., 2020). Performance is significantly influenced by density and honeycomb structure in addition to the polymer matrix. Compared to conventional production techniques, additive manufacturing offers unprecedented flexibility in achieving controlled structure, geometric characteristics, feature, and complexity (Kim and Oh, 2008). Conventional foams have a random distribution of porosity throughout, inspired by the topology of natural honeycomb structures. Structures found in bone and wood, for example, where the solids and voids are organized in accordance with the load-bearing requirements (Jiang et al., 2020). First, it should be noted that additive manufacturing utilizes two distinct forms of porosity. When building the structure layer by layer, there are still certain voids without polymer deposition even when the filler content is set to 100% (Nofar et al., 2022). However, porosity can also be used to create certain honeycomb structures. The porosity parameter generated in this manner is controversial and even considered as a drawback in studies. Specific pore types can carry out specialized tasks like sound and thermal insulation. Regular porous scaffolds, such as cellular structures Chen et al. (2018b), truss-based lattices (Rehme, 2010), octahedron and rhombicuboctahedron structures (Liu et al., 2017), and surface based lattices like gyroid or diamond structures (Maskery et al., 2018) are the main focus of additive manufacturing research. To date, the following methods have been documented for generating cellular structures by additive manufacturing (FFF printing technology): 1) cellular scaffolds; 2) composite printing foam; 3) post-foaming of printed solid scaffolds; 4) in-situ foam 3D printing. Of course, there are many challenges in implementing thermoplastic foams using the FFF process. Pre-foaming must use foamed filament as the raw material for additive manufacturing, limiting the range of honeycomb mesh structures that can be obtained and also risking loss of structure during the printing process (Kuang et al., 2017). Complex post-processing stages, high pressure-reducing device requirements, and limited expansion may be problems for post-foaming methods, which retouch the item after printing (Zhai et al., 2022). In-situ foaming, on the other hand, is the most recent approach that matches the foaming and fused filament fabrication processes flawlessly. However, obtaining an uniform and stable polymer or gas solution via the foaming agent during the material extrusion process is difficult. The approach may result in inhomogeneous cell nucleation, poor vesicle density, uneven cell structure, and other problems. Below, we detail the particular material molding process.
3.1 Cellular scaffolds
Cellular scaffolds designed by conventional manufacturing methods (Yang et al., 2001) have a single type of pore unit, and most of them can only make coarse adjustments to individual parameters such as porosity and pore size (Jia et al., 2020; Jiang et al., 2020). The internal microscopic honeycomb structure of the forming scaffold is random, and the pores are not completely connected, which cannot meet the actual needs. FFF printing technology applied to cellular scaffolds assembly can not only precisely control the spatial structure distribution of the scaffold, but also effectively control the functional gradient of the scaffold material, thus enabling the engineering of scaffold manufacturing. On the other hand, the construction of honeycomb structures by layer-by-layer printing is different from the traditional foaming process. In terms of mechanical qualities and accessible active surfaces, hierarchical cellular scaffolds perform better than their non-hierarchical counterparts. This is due to hierarchical cellular scaffolds are highly heterogeneous at mesoscale in geometry and porosity distribution, with coupling effect among cells (Wang et al., 2018; Yin et al., 2023).The FFF method may produce a variety of random and ordered geometries. The resulting scaffold topology can then be used to modify the unit cell units, as shown in Figure 5, to alter the overall quality of the completed project (Habib et al., 2016). In this instance, the specified geometry, unit cell components, and their structures can be used to modify the mechanical characteristics of this structured cellular structure (Pajunen et al., 2019). By employing additive manufacturing, two-dimensional (2D) and three-dimensional (3D) architectures of composite have been created to investigate the impact of unit cell connection and geometry on mechanical properties. Sirui Bi et al. synthesized brittle foams with regulated morphological properties and conducted an array of tests to gauge the compressive response and strength of 3D printed foams (Bi et al., 2020).
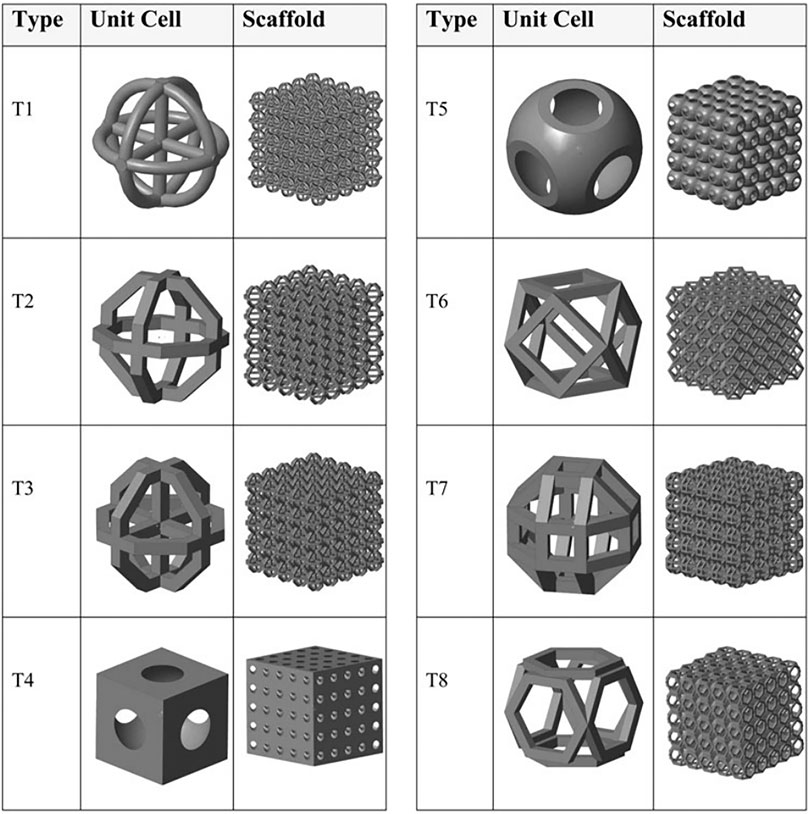
FIGURE 5. Models of all (eight) types of unit cells and scaffolds. Copyright 2016, Mary Ann Liebert. Inc.
Highly porous scaffold materials are required for tissue engineering (TE)with the aim of accommodating and directing proliferation, regeneration, and development of cells in three dimensions (Habib et al., 2016). Polymeric materials have shown strong competitiveness in cellular scaffolds, such as polyethylene glycol (PEG) (Liang et al., 2022), poly (L-lactic acid) (PLLA) (Kuang et al., 2017), polyglycolic acid (PGA) (Yang et al., 2001), polycaprolactone (PCL) and their copolymers (Cho et al., 2019). Porous scaffolds prepared from these synthetic polymers have biocompatible, high porosity and interconnected pores that allow for the accommodation of large numbers of cells in these pores (Melchels et al., 2010). FFF printing technology is used to create personalized TE scaffold materials and can even carry cells for in situ cell printing on tissue defect sites. Insoles for the treatment of diabetes also benefit from an optimized porous structure. The design with a variable gradient modulus enhances the contact stress between the foot and the insole to avoid ulceration. (Ma et al., 2019). However, a significant barrier to the therapeutic use of 3D printed cellular structures is the realization of tissue heterogeneity through the controlled distribution of various cell and biomaterial types at the micro- and macro-scales (Fang et al., 2022). All in all, it could be stated that the main objective of 3D printed cellular structures is novel functional integration, which can be precisely accomplished by regulating the important characteristics of unit cells (Nofar et al., 2022).
3.2 Composite printing foam
3D printing of polymer composites addresses these issues by combining the polymer matrix and nanomaterial reinforcements to generate a society with more valuable structural and functional features than either of the constituents could achieve on their own (Thomas et al., 2019).These reinforcements are usually denser and can be incorporated directly into the polymer matrix to form porous structures (Patil et al., 2019; Bharath et al., 2020; Bonthu et al., 2020).The development of polymer matrix composites, which have great mechanical performance and outstanding functionality, is made feasible by adding reinforcements to polymers in the form of fibers, particles or nanomaterials (Wang et al., 2017). Porosity and fiber orientation of composites have a considerable impact on the properties of final composite products (Patil et al., 2019). The short fiber (0.2–0.4 mm) reinforced acrylonitrile–butadiene–styrene composite made by FFF printing composite foam is depicted in Figure 6 (Tekinalp et al., 2014). Printed composite foams had relatively higher porosity than composites made by traditional compression molding (CE), but their tensile strength and modulus were comparable. The purpose of this research is to develop a compression molding-based manufacturing technique for glass micro-balloon/high density polyethylene (GMB/HDPE) syntactic foams (Jayavardhan et al., 2017) while examining their mechanical characteristics in order to establish correlations between structures and features. They claimed that the entrapped hollow spheres were retained in the produced filaments as well as in the 3D printed samples (Jayavardhan et al., 2017; Bonthu et al., 2020).As the Figure 7 shows, particle fracture increases with increasing GMB content due to increased particle-to-particle interaction during processing and a higher breakdown of GMB is seen in the syntactic foam developed at a higher screw speed. M. Doddamani et al. generated three distinct volume fractions of GMB particles with varying wall thicknesses 20%, 40%, and 60% (particle density variations) (Doddamani, 2019). When compared to HDPE matrix resin, storage modulus, loss modulus, and damping are seen to increase with particle wall thickness and volume fraction. When these composite foams are combined, they often allow for the tailoring of properties in two dimensions (i.e., wall thickness and volume fraction modification), providing versatility in creating materials for a wide variety of purposes.
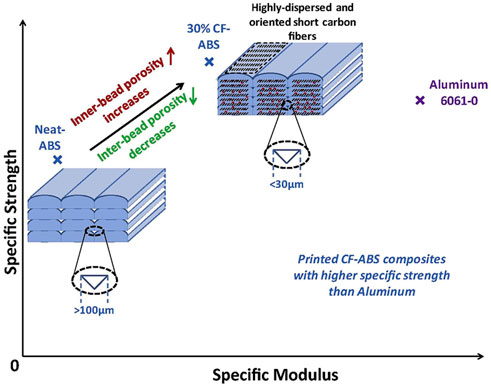
FIGURE 6. Schematic diagram of 3D-printed fiber-reinforced composite by Fused Filament Fabrication. Copyright 2014, Elsevier.
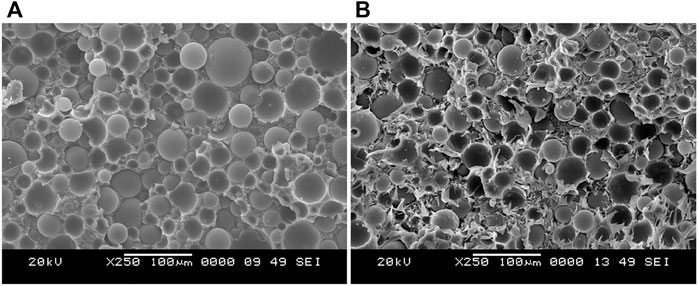
FIGURE 7. SEM image of H200-60 syntactic foam that has been formed and frozen and was taken at the same magnifications for (A) 10 and (B) 40 rpm screw rotation Copyright 2017, Elsevier.
3.3 Post-foaming of printed solid scaffolds
FFF printing technology in combination with gas foaming technology was used to construct a recently created solid scaffold with a precise microstructure of about 10 μm (Zhou et al., 2016). The sample is first 3D printed using FFF in this process, and then a porous structure is created in the sample using the gas foaming technique. By trimming and cutting stages in the post-processing of the foam, this method of printing cellular foam with post-foaming in conjunction with additive manufacturing plays an important role in decreasing the traditional supercritical gas foaming phase and minimizing material waste. Figure 8 shows the rapid CO2 foaming process of 3D printed thermoplastic polyurethane elastomer developed by our research group (Gunasekaran et al., 2022a). In this work, the foaming behavior of printed TPU elastomers with three different hardness values was examined. The resulting foam samples can be used for high-end applications, such as shoe bottoms, which conventional foaming cannot provide (Gunasekaran et al., 2022a). Additionally, compared to their un-foamed counterparts, microcellular TPU honeycombs showed greater elasticity and better elastic recovery (Hu et al., 2021). The foam produced through the post-foaming route also differs from prefabricated structures in terms of its properties. For example, the foaming technique produces a low mass density and high sensitivity sensor in addition to creating a conductive network on the surface and interface of the printing system. Figure 9 (Gunasekaran et al., 2022b) depicts the conductive TPU foam’s assembly procedure. Our research has shown that adding graphene sensors directly to the surface of TPU has a limited impact, and that foaming creates a microporous structure that helps embed graphene and create a conductive network.
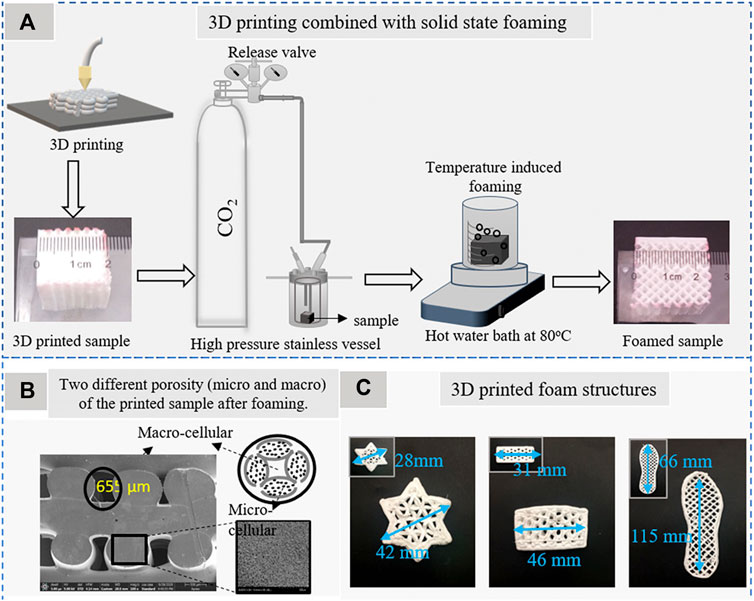
FIGURE 8. (A) Schematic presentation of the 3D printing combined CO2 solid-state foaming process. (B) Two different porosities of the printed sample. (C) 3D printed foamed sample with different structures. Copyright 2022, American Chemical Society.
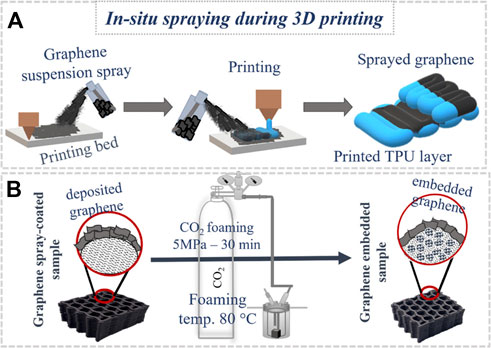
FIGURE 9. (A) Fabrication process of the in situ spray-coated 3D printed sensor. (B) Graphene embedding and microporous generation. Copyright 2022, American Chemical Society.
3.4 In-Situ foam 3D printing
In-situ foam 3D printing is the technique that properly merges the foaming and fused filament fabrication processes. Despite in the early stages of study and not yet fully established, this foam 3D printing technique appears to be the most successful way to produce foamed printed structures (Nofar et al., 2022). There are now two proven techniques for in-situ foam 3D printing, with the key distinction being whether a single-phase polymer or a gaseous solution is reached before cell nucleation and growth (Kalia et al., 2022). Unexpanded filaments containing blowing agents, either physical or chemical blowing agents, are prepared in the first technique. Thermally expandable microspheres (TEMs) can be used to 3D print in-situ foam without the need for extra gas impregnation (Cai et al., 2021). TEM has also been found to provide a more homogeneous porous structure in conventional foam operations like as extrusion and injection molding when compared to alternative foaming techniques (Peng et al., 2013; Kmetty and Litauszki, 2020). This method may be able to address the aforementioned difficulties without the need for any extra preprocessing, postprocessing, or specialized 3D printing equipment (Contreras et al., 2020). In a recent study, Andersson et al. (2021) looked into the mechanical strength, micromorphology of in situ foam 3D printed using PLA and TEM-ethylene vinyl acetate masterbatch. However, the reported foams had substantial differences in densities, brittleness, and rough surfaces, which can be related to the formulation of the material as well as the lack of homogeneous TEM dispersion (Andersson et al., 2021). They also showed nonuniform cellular architecture.
In addition, the second in-situ foaming method involves foaming the extruded filament by means of an external CO2 gas. The filament exits the nozzle during printing, and foam expands as a result of changes in the thermodynamics. As shown in Figure 10, Li et al. (2020) reported using polyetherimide and polylactic acid (PLA) filaments to manufacture hierarchical porous portions that were impregnated with CO2 gas. Zhang et al. (2022) offered a simple solution by combining 3D FFF printing with supercritical microcellular foaming to address the primary obstacle for tissue-engineering scaffolds with hierarchical topologies. As seen Figure 11 (Zhang et al., 2022), the flexibility of additive manufacturing design results in pores are larger than microns due to layer stacking, whereas microcellular structures result in pores smaller than microns due to foaming. In-situ foam 3D printing requires an additional stage of gas impregnation before printing and the gas-saturated filaments commence to release gas as soon as they are removed from the high-pressure chamber, which is one of its biggest advantages. As a result, the first barrier is generating a homogeneous polymer matrix from a physical blowing agent, and the second barrier is handling and printing filaments that have been impregnated with gas.
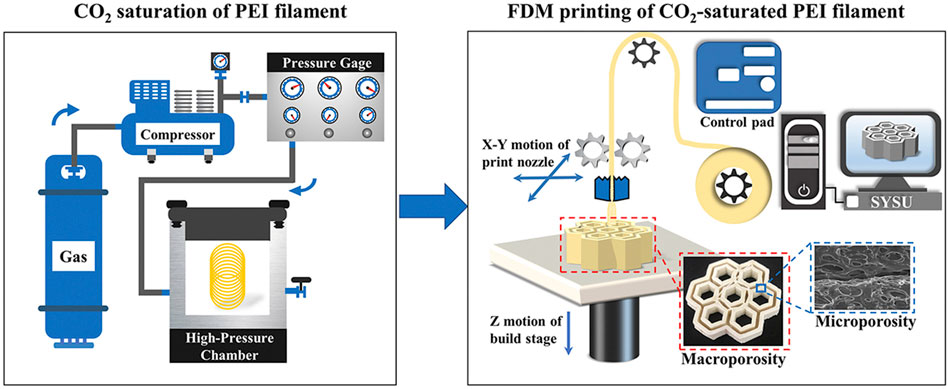
FIGURE 10. Diagram of the CO2-saturated PEI filament’s FFF printing process. Copyright 2020, Elsevier.
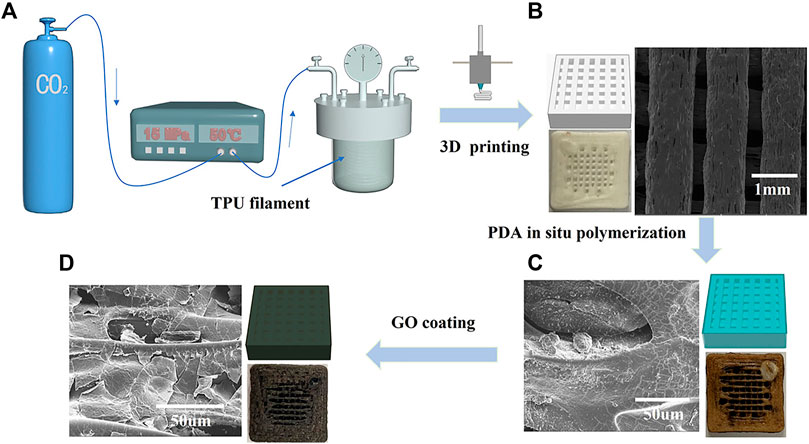
FIGURE 11. Schematic illustration of the fabrication for FFF printed TPU scaffold with microcellular foaming and surface coating with graphene oxide. Copyright 2022, Wiley-VCH.
To avoid the issues of low melt strength, poor foam expansion ratio, low cell density, and uneven shape of big cells caused by the use of gaseous or chemical blowing agents, special printer systems or pretreatment processes need to be developed. (Damanpack et al., 2021). As shown in Figure 12, PLA was employed as the feedstock material along with a polyethylene carrier, two weight percent triethyl citrate (TEC) plasticizer, and zero to five weight percent acrylonitrile-based TEM (Kalia et al., 2022). An improved extrusion procedure was used to create the unexpanded filaments, and then a commercially available printer was used to 3D print foam in place (Kalia et al., 2022). The degree of foam densities and TEM content was connected with the microstructure, cellular morphology, density, thermal and mechanical properties of the printed foams.
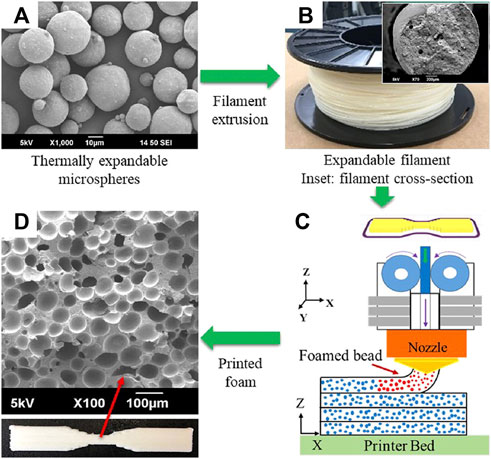
FIGURE 12. Overview of the in situ foam 3D-printing process: (A) TEM particles as blowing agents, (B) extruded expandable filament with an inset SEM micrograph showing its cross-sectional view, (C) part design and schematic of the in situ foam printing process, and (D) example of the printed tensile sample with a SEM micrograph showing the microcellular morphology in the Y-Z plane. Copyright 2022, American Chemical Society.
4 Summary and trends in 3D printing foams
Overall, the combination of additive printing with polymer foam opens up new possibilities for the production of lightweight goods with complex structures and hierarchical features. Depending on the shape of the created foamed part, we can also classify the first two processes as pre-foaming, the third as post-foaming, and the final as in-situ foaming. Pre-foaming must use foamed filament as the raw material for additive manufacturing, limiting the range of honeycomb mesh structures that can be obtained and also risking loss of structure during the printing process. Complex post-processing stages, high pressure-reducing device requirements, and limited expansion may be problems for post-foaming methods, which retouch the parts after printing. On the other hand, in-situ foaming is the latest approach that matches perfectly with foaming and FFF process. However, obtaining a homogeneous and stable polymer/gas solution via the foaming agent during the material extrusion process is difficult. The approach may result in inhomogeneous cell nucleation, poor vesicle density, uneven cell structure, and other problems.
The problems that arise in the use of gas or chemical blowing agents can be avoided by developing special printer systems or pretreatment processes. However, the current 3D printing foaming still has many shortcomings and defects, and the future direction of development may focus on the development of filament-free method by free-form technology. Establishing parallel strategies for thermoplastic printing and foaming to overcome the limitations of thermoplastic material selection, uncertainties caused by inhomogeneous dissolution of foaming agents. This method eliminates the manufacturing process of filaments and injects the foaming agent directly into the extruder barrel, thus achieving the integration of extrusion foaming and foaming injection molding.
Author contributions
LW: Funding acquisition, conceptualization, reviewing, project administration. BS: Methodology, literature search and sorting, writing.
Funding
This work is supported by Ningbo public welfare science and technology planning project (Grant No: 2022S099), the STS Project of Fujian-CAS (Grant Nos: 2021T3039, 2021T3047, 2021T3018, 2022T3009, 2022T3019, 2022T3045, 2022T3064), the regional development project of Fujian (Grant No: 2022H4020),the STS Project of Putian-CAS (Grant No: 2020HJST009) and the Major Project of Science and Technology in Fuzhou (Grant No: 2021-ZD-281), the project of Fujian Market Supervision Administration (Grant No: FJMS2021023).
Conflict of interest
The authors declare that the research was conducted in the absence of any commercial or financial relationships that could be construed as a potential conflict of interest.
Publisher’s note
All claims expressed in this article are solely those of the authors and do not necessarily represent those of their affiliated organizations, or those of the publisher, the editors and the reviewers. Any product that may be evaluated in this article, or claim that may be made by its manufacturer, is not guaranteed or endorsed by the publisher.
References
Ahn, J., Lim, T., Yeo, C. S., Hong, T., Jeong, S. M., Park, S. Y., et al. (2019). Infrared invisibility cloak based on polyurethane-tin oxide composite microtubes. ACS Appl. Mat. Interfaces 11, 14296–14304. doi:10.1021/acsami.8b22535
Ameli, A., Jahani, D., Nofar, M., Jung, P. U., and Park, C. B. (2014). Development of high void fraction polylactide composite foams using injection molding: Mechanical and thermal insulation properties. Compos. Sci. Technol. 90, 88–95. doi:10.1016/j.compscitech.2013.10.019
Andersson, H., Örtegren, J., Zhang, R., Grauers, M., and Olin, H. (2021). Variable low-density polylactic acid and microsphere composite material for additive manufacturing. Addit. Manuf. 40, 101925. doi:10.1016/j.addma.2021.101925
Ashby, M. F. (2006). The properties of foams and lattices. Phil. Trans. R. Soc. A 364, 15–30. doi:10.1098/rsta.2005.1678
Attaran, M. (2017). The rise of 3-D printing: The advantages of additive manufacturing over traditional manufacturing. Bus. Horiz. 60, 677–688. doi:10.1016/j.bushor.2017.05.011
Bharath, H. S., Sawardekar, A., Waddar, S., Jeyaraj, P., and Doddamani, M. (2020). Mechanical behavior of 3D printed syntactic foam composites. Compos. Struct. 254, 112832. doi:10.1016/j.compstruct.2020.112832
Bi, S., Chen, E., and Gaitanaros, S. (2020). Additive manufacturing and characterization of brittle foams. Mech. Mat. 145, 103368. doi:10.1016/j.mechmat.2020.103368
Bonthu, D., Bharath, H. S., Gururaja, S., Prabhakar, P., and Doddamani, M. (2020). 3D printing of syntactic foam cored sandwich composite. Compos. Part C. Open Access 3, 100068. doi:10.1016/j.jcomc.2020.100068
Borrello, J., Nasser, P., Iatridis, J. C., and Costa, K. D. (2018). 3D printing a mechanically-tunable acrylate resin on a commercial DLP-SLA printer. Addit. Manuf. 23, 374–380. doi:10.1016/j.addma.2018.08.019
Cai, J. H., Huang, M. L., Chen, X. D., and Wang, M. (2021). Thermo-expandable microspheres strengthened polydimethylsiloxane foam with unique softening behavior and high-efficient energy absorption. Appl. Surf. Sci. 540, 148364. doi:10.1016/j.apsusc.2020.148364
Chen, Q., Cao, P. F., and Advincula, R. C. (2018a). Mechanically robust, ultraelastic hierarchical foam with tunable properties via 3D printing. Adv. Funct. Mat. 28, 1800631–1800639. doi:10.1002/adfm.201800631
Chen, Q., Zhao, J., Ren, J., Rong, L., Cao, P. F., and Advincula, R. C. (2019). 3D printed multifunctional, hyperelastic silicone rubber foam. Adv. Funct. Mat. 29, 1900469–9. doi:10.1002/adfm.201900469
Chen, Y., Li, T., Jia, Z., Scarpa, F., Yao, C. W., and Wang, L. (2018b). 3D printed hierarchical honeycombs with shape integrity under large compressive deformations. Mat. Des. 137, 226–234. doi:10.1016/j.matdes.2017.10.028
Cho, Y. S., Choi, S., Lee, S. H., Kim, K. K., and Cho, Y. S. (2019). Assessments of polycaprolactone/hydroxyapatite composite scaffold with enhanced biomimetic mineralization by exposure to hydroxyapatite via a 3D-printing system and alkaline erosion. Eur. Polym. J. 113, 340–348. doi:10.1016/j.eurpolymj.2019.02.006
Contreras, V., Maturana, F. J., Poveda, J., Núñez, K. C., Merino, J. C., and Pastor, J. M. (2020). Optimization of injection parameters to obtain selected properties on foamed PP with hollow glass microspheres and thermally expandable microspheres using Taguchi method. J. Cell. Plast. 54, 313–327. doi:10.1177/0021955X20943097
Credi, C., Fiorese, A., Tironi, M., Bernasconi, R., Magagnin, L., Levi, M., et al. (2016). 3D printing of cantilever-type microstructures by stereolithography of ferromagnetic photopolymers. ACS Appl. Mat. Interfaces 8, 26332–26342. doi:10.1021/acsami.6b08880
Damanpack, A. R., Sousa, A., and Bodaghi, M. (2021). Porous plas with controllable density by fdm 3d printing and chemical foaming agent. Micromachines 12, 866–868. doi:10.3390/mi12080866
Di Maio, E., and Kiran, E. (2018). Foaming of polymers with supercritical fluids and perspectives on the current knowledge gaps and challenges. J. Supercrit. Fluids 134, 157–166. doi:10.1016/j.supflu.2017.11.013
Diani, J., and Gall, K. (2006). Finite strain 3D thermoviscoelastic constitutive model. Society 46, 1–10. doi:10.1002/pen.20497
Doddamani, M. (2019). Influence of microballoon wall thickness on dynamic mechanical analysis of closed cell foams. Mat. Res. Express 6, 125348. doi:10.1088/2053-1591/ab62f4
Eltorai, A. E. M., Nguyen, E., and Daniels, A. H. (2015). Three-dimensional printing in orthopedic surgery. Orthopedics 38, 684–687. doi:10.3928/01477447-20151016-05
Fang, Y., Guo, Y., Liu, T., Xu, R., Mao, S., Mo, X., et al. (2022). Advances in 3D bioprinting. Chin. J. Mech. Eng. Addit. Manuf. Front. 1, 100011. doi:10.1016/j.cjmeam.2022.100011
Felzmann, B. R., Gruber, S., Mitteramskogler, G., Tesavibul, P., Boccaccini, A. R., Liska, R., et al. (2012). Lithography-based additive manufacturing of cellular ceramic structures. Adv. Eng. Mat. 14, 1052–1058. doi:10.1002/adem.201200010
Forest, C., Chaumont, P., Cassagnau, P., Swoboda, B., and Sonntag, P. (2015). Polymer nano-foams for insulating applications prepared from CO2 foaming. Prog. Polym. Sci. 41, 122–145. doi:10.1016/j.progpolymsci.2014.07.001
Franchin, G., Elsayed, H., Botti, R., Huang, K., Schmidt, J., Giometti, G., et al. (2022). Additive manufacturing of ceramics from liquid feedstocks. Chin. J. Mech. Eng. Addit. Manuf. Front. 1, 100012. doi:10.1016/j.cjmeam.2022.100012
Fu, Y., Downey, A., Yuan, L., Pratt, A., and Balogun, Y. (2021). In situ monitoring for fused filament fabrication process: A review. Addit. Manuf. 38, 101749. doi:10.1016/j.addma.2020.101749
Gross, B. C., Erkal, J. L., Lockwood, S. Y., Chen, C., and Spence, D. M. (2014). Evaluation of 3D printing and its potential impact on biotechnology and the chemical sciences. Anal. Chem. 86, 3240–3253. doi:10.1021/ac403397r
Gunasekaran, H. B., Ponnan, S., Thirunavukkarasu, N., Laroui, A., Wu, L., and Wang, J. (2022a). Rapid carbon dioxide foaming of 3D printed thermoplastic polyurethane elastomers. ACS Appl. Polym. Mat. 4, 1497–1511. doi:10.1021/acsapm.1c01846
Gunasekaran, H. B., Ponnan, S., Zheng, Y., Laroui, A., Wang, H., Wu, L., et al. (2022b). Facile fabrication of highly sensitive thermoplastic polyurethane sensors with surface- and interface-impregnated 3D conductive networks. ACS Appl. Mat. Interfaces 14, 22615–22625. doi:10.1021/acsami.2c03351
Habib, F. N., Nikzad, M., Masood, S. H., and Saifullah, A. B. M. (2016). Design and development of scaffolds for tissue engineering using three-dimensional printing for bio-based applications. 3D Print. Addit. Manuf. 3, 119–127. doi:10.1089/3dp.2015.0014
Hofmann, M. (2014). 3D printing gets a boost and opportunities with polymer materials. ACS Macro Lett. 3, 382–386. doi:10.1021/mz4006556
Hu, B., Li, M., Jiang, J., and Zhai, W. (2021). Development of microcellular thermoplastic polyurethane honeycombs with tailored elasticity and energy absorption via CO2 foaming. Int. J. Mech. Sci. 197, 106324. doi:10.1016/j.ijmecsci.2021.106324
Hu, F., Wu, S., and Sun, Y. (2019). Hollow-structured materials for thermal insulation. Adv. Mat. 31, 1801001–1801017. doi:10.1002/adma.201801001
Hu, X., Tian, M., Xu, T., Sun, X., Sun, B., Sun, C., et al. (2020). Multiscale disordered porous fibers for self-sensing and self-cooling integrated smart sportswear. ACS Nano 14, 559–567. doi:10.1021/acsnano.9b06899
Hwang, S., Reyes, E. I., Moon, K. sik, Rumpf, R. C., and Kim, N. S. (2015). Thermo-mechanical characterization of metal/polymer composite filaments and printing parameter study for fused deposition modeling in the 3D printing process. J. Electron. Mat. 44, 771–777. doi:10.1007/s11664-014-3425-6
Jahani, D., Ameli, A., Jung, P. U., Barzegari, M. R., Park, C. B., and Naguib, H. (2014). Open-cell cavity-integrated injection-molded acoustic polypropylene foams. Mat. Des. 53, 20–28. doi:10.1016/j.matdes.2013.06.063
Jahani, D., Ameli, A., Saniei, M., Ding, W., Park, C. B., and Naguib, H. E. (2015). Characterization of the structure, acoustic property, thermal conductivity, and mechanical property of highly expanded open-cell polycarbonate foams. Macromol. Mat. Eng. 300, 48–56. doi:10.1002/mame.201400125
Janusziewicz, R., Tumbleston, J. R., Quintanilla, A. L., Mecham, S. J., and DeSimone, J. M. (2016). Layerless fabrication with continuous liquid interface production. Proc. Natl. Acad. Sci. U. S. A. 113, 11703–11708. doi:10.1073/pnas.1605271113
Jayavardhan, M. L., Kumar, B. R. B., Doddamani, M., Singh, A. K., Zeltmann, S. E., and Gupta, N. (2017). Development of glass microballoon/HDPE syntactic foams by compression molding. Compos. Part B Eng. 130, 119–131. doi:10.1016/j.compositesb.2017.07.037
Ji, J., Zhang, L. L., Ji, H., Li, Y., Zhao, X., Bai, X., et al. (2013). Nanoporous Ni(OH)2 thin film on 3D ultrathin-graphite foam for asymmetric supercapacitor. ACS Nano 7, 6237–6243. doi:10.1021/nn4021955
Jia, H., Lei, H., Wang, P., Meng, J., Li, C., Zhou, H., et al. (2020). An experimental and numerical investigation ofcompressive response ofdesigned Schwarz Primitive triply periodic minimal surface with non-uniform shell thickness. Extreme Mech. Lett. 37, 100671. doi:10.1016/j.eml.2020.100671
Jiang, H., Ziegler, H., Zhang, Z., Meng, H., Chronopoulos, D., and Chen, Y. (2020). Mechanical properties of 3D printed architected polymer foams under large deformation. Mat. Des. 194, 108946. doi:10.1016/j.matdes.2020.108946
Kaiser, D. L., and Chalfin, K. (2017). Standards from ASTM international technical committee E56 on nanotechnology. Metrol. Stand. Nanotechnol. 229, 269–278. doi:10.1002/9783527800308.ch15
Kakumanu, V., and Srinivas Sundarram, S. (2018). Dual pore network polymer foams for biomedical applications via combined solid state foaming and additive manufacturing. Mat. Lett. 213, 366–369. doi:10.1016/j.matlet.2017.11.027
Kalia, K., Francoeur, B., Amirkhizi, A., and Ameli, A. (2022). In situ foam 3D printing of microcellular structures using material extrusion additive manufacturing. ACS Appl. Mat. Interfaces 14, 22454–22465. doi:10.1021/acsami.2c03014
Kim, G. D., and Oh, Y. T. (2008). A benchmark study on rapid prototyping processes and machines: Quantitative comparisons of mechanical properties, accuracy, roughness, speed, and material cost. Proc. Institution Mech. Eng. Part B J. Eng. Manuf. 222, 201–215. doi:10.1243/09544054JEM724
Kmetty, Á., and Litauszki, K. (2020). Development of poly (lactide acid) foams with thermally expandable microspheres. Polym. (Basel) 12, 463. doi:10.3390/polym12020463
Kokkinis, D., Schaffner, M., and Studart, A. R. (2015). Multimaterial magnetically assisted 3D printing of composite materials. Nat. Commun. 6, 8643. doi:10.1038/ncomms9643
Kuang, T., Chen, F., Chang, L., Zhao, Y., Fu, D., Gong, X., et al. (2017). Facile preparation of open-cellular porous poly (L-lactic acid) scaffold by supercritical carbon dioxide foaming for potential tissue engineering applications. Chem. Eng. J. 307, 1017–1025. doi:10.1016/j.cej.2016.09.023
Kumar, V., Juntunen, R. P., and Barlow, C. (2000). Impact strength of high relative density solid state carbon dioxide blown crystallizable poly(ethylene terephthalate) microcellular foams. Cell. Polym. 19, 25–37.
Lee, S. T., Kareko, L., and Jun, J. (2008). Study of thermoplastic PLA foam extrusion. J. Cell. Plast. 44, 293–305. doi:10.1177/0021955X08088859
Li, B., Zhao, G., Wang, G., Zhang, L., Gong, J., and Shi, Z. (2021). Biodegradable PLA/PBS open-cell foam fabricated by supercritical CO2 foaming for selective oil-adsorption. Sep. Purif. Technol. 257, 117949. doi:10.1016/j.seppur.2020.117949
Li, M., Jiang, J., Hu, B., and Zhai, W. (2020). Fused deposition modeling of hierarchical porous polyetherimide assisted by an in-situ CO2 foaming technology. Compos. Sci. Technol. 200, 108454. doi:10.1016/j.compscitech.2020.108454
Liang, H., Fu, G., Liu, J., Tang, Y., Wang, Y., Chen, S., et al. (2022). A 3D-printed Sn-doped calcium phosphate scaffold for bone tissue engineering. Front. Mat. 9, 1–8. doi:10.3389/fmats.2022.1016820
Ligon-Auer, S. C., Schwentenwein, M., Gorsche, C., Stampfl, J., and Liska, R. (2016). Toughening of photo-curable polymer networks: A review. Polym. Chem. 7, 257–286. doi:10.1039/c5py01631b
Ling, J., Zhai, W., Feng, W., Shen, B., Zhang, J., and Zheng, W. G. (2013). Facile preparation of lightweight microcellular polyetherimide/graphene composite foams for electromagnetic interference shielding. ACS Appl. Mat. Interfaces 5, 2677–2684. doi:10.1021/am303289m
Liu, L., Kamm, P., García-Moreno, F., Banhart, J., and Pasini, D. (2017). Elastic and failure response of imperfect three-dimensional metallic lattices: The role of geometric defects induced by selective laser melting Lu. J. Mech. Phys. Solids 107, 160–184. doi:10.1016/j.jmps.2017.07.003
Liu, W. C., Chou, V. H. Y., Behera, R. P., and Le Ferrand, H. (2022). Magnetically assisted drop-on-demand 3D printing of microstructured multimaterial composites. Nat. Commun. 13, 5015. doi:10.1038/s41467-022-32792-1
Ma, Z., Lin, J., Xu, X., Ma, Z., Tang, L., Sun, C., et al. (2019). Design and 3D printing of adjustable modulus porous structures for customized diabetic foot insoles. Int. J. Lightweight Mater. Manuf. 2, 57–63. doi:10.1016/j.ijlmm.2018.10.003
Maskery, I., Aremu, A. O., Parry, L., Wildman, R. D., Tuck, C. J., and Ashcroft, I. A. (2018). Effective design and simulation of surface-based lattice structures featuring volume fraction and cell type grading. Mat. Des. 155, 220–232. doi:10.1016/j.matdes.2018.05.058
Melchels, F. P. W., Bertoldi, K., Gabbrielli, R., Velders, A. H., Feijen, J., and Grijpma, D. W. (2010). Mathematically defined tissue engineering scaffold architectures prepared by stereolithography. Biomaterials 31, 6909–6916. doi:10.1016/j.biomaterials.2010.05.068
Nadgorny, M., and Ameli, A. (2018). Functional polymers and nanocomposites for 3D printing of smart structures and devices. ACS Appl. Mat. Interfaces 10, 17489–17507. doi:10.1021/acsami.8b01786
Nadiyapara, H. H., and Pande, S. (2017). A review of variable slicing in fused deposition modeling. J. Inst. Eng. India. Ser. C 98, 387–393. doi:10.1007/s40032-016-0272-7
Narupai, B., and Nelson, A. (2020). 100th anniversary of macromolecular science viewpoint: Macromolecular materials for additive manufacturing. ACS Macro Lett. 9, 627–638. doi:10.1021/acsmacrolett.0c00200
Nikolić, S., and Stevanović, G. (2006). Neurocysticercosis--pathogenesis and clinical aspects. Srp. Arh. Celok. Lek. 134, 246–250. doi:10.1117/1.JBO.17.10
Nofar, M. (2016). Effects of nano-/micro-sized additives and the corresponding induced crystallinity on the extrusion foaming behavior of PLA using supercritical CO2. Mat. Des. 101, 24–34. doi:10.1016/j.matdes.2016.03.147
Nofar, M., Utz, J., Geis, N., Altstädt, V., and Ruckdäschel, H. (2022). Foam 3D printing of thermoplastics: A symbiosis of additive manufacturing and foaming technology. Adv. Sci. (Weinh). 9, 2105701–2105718. doi:10.1002/advs.202105701
Pajunen, K., Johanns, P., Pal, R. K., Rimoli, J. J., and Daraio, C. (2019). Design and impact response of 3D-printable tensegrity-inspired structures. Mat. Des. 182, 107966. doi:10.1016/j.matdes.2019.107966
Palaganas, N. B., Mangadlao, J. D., De Leon, A. C. C., Palaganas, J. O., Pangilinan, K. D., Lee, Y. J., et al. (2017). 3D printing of photocurable cellulose nanocrystal composite for fabrication of complex architectures via stereolithography. ACS Appl. Mat. Interfaces 9, 34314–34324. doi:10.1021/acsami.7b09223
Patil, B., Kumar, B. R. B., Bontha, S., Krishna, V., Powar, S., Kumar, V. H., et al. (2019). Eco-friendly lightweight fi lament synthesis and mechanical characterization of additively manufactured closed cell foams. Compos. Sci. Technol. 183, 107816. doi:10.1016/j.compscitech.2019.107816
Peng, J., Srithep, Y., Wang, J., Yu, E., Turng, L. S., and Peng, X. F. (2013). Comparisons of microcellular polylactic acid parts injection molded with supercritical nitrogen and expandable thermoplastic microspheres: Surface roughness, tensile properties, and morphology. J. Cell. Plast. 49, 33–45. doi:10.1177/0021955X12450208
Pérez, M., Medina-Sánchez, G., García-Collado, A., Gupta, M., and Carou, D. (2018). Surface quality enhancement of fused deposition modeling (FDM) printed samples based on the selection of critical printing parameters. Mater. (Basel) 11, 1382. doi:10.3390/ma11081382
Qi, S., Guo, H., Fu, J., Xie, Y., Zhu, M., and Yu, M. (2020). 3D printed shape-programmable magneto-active soft matter for biomimetic applications. Compos. Sci. Technol. 188, 107973. doi:10.1016/j.compscitech.2019.107973
Rashid, A. (2019). Additive manufacturing technologies. Editor S. Chatti, and T. Tolio (Springer, Berlin, Heidelberg: CIRP Encyclopedia of Production Engineering). doi:10.1007/978-3-642-35950-7_16866-1
Rehme, O. (2010). Cellular design for laser freeform fabrication. Available at: https://cuvillier.de/uploads/preview/public_file/1820/9783869552736.pdf.
Rizvi, A., Tabatabaei, A., Vahedi, P., Mahmood, S. H., and Park, C. B. (2018). Non-crosslinked thermoplastic reticulated polymer foams from crystallization-induced structural heterogeneities. Polymer 135, 185–192. doi:10.1016/j.polymer.2017.12.006
Sadik, T., Pillon, C., Carrot, C., and Reglero Ruiz, J. A. (2018). Dsc studies on the decomposition of chemical blowing agents based on citric acid and sodium bicarbonate. Thermochim. Acta 659, 74–81. doi:10.1016/j.tca.2017.11.007
Sauceau, M., Fages, J., Common, A., Nikitine, C., and Rodier, E. (2011). New challenges in polymer foaming: A review of extrusion processes assisted by supercritical carbon dioxide. Prog. Polym. Sci. 36, 749–766. doi:10.1016/j.progpolymsci.2010.12.004
Schirmeister, C. G., Hees, T., Licht, E. H., and Mülhaupt, R. (2019). 3D printing of high density polyethylene by fused filament fabrication. Addit. Manuf. 28, 152–159. doi:10.1016/j.addma.2019.05.003
Schmitt, B. M., Zirbes, C. F., Bonin, C., Lohmann, D., Lencina, D. C., and Da Costa Sabino Netto, A. (2017). A comparative study of cartesian and delta 3d printers on producing PLA parts. Mat. Res. 20, 883–886. doi:10.1590/1980-5373-mr-2016-1039
Shmueli, Y., Jiang, J., Zhou, Y., Xue, Y., Chang, C. C., Yuan, G., et al. (2019). Simultaneous in situ X-ray scattering and infrared imaging of polymer extrusion in additive manufacturing. ACS Appl. Polym. Mat. 1, 1559–1567. doi:10.1021/acsapm.9b00328
Stansbury, J. W., and Idacavage, M. J. (2016). 3D printing with polymers: Challenges among expanding options and opportunities. Dent. Mat. 32, 54–64. doi:10.1016/j.dental.2015.09.018
Tekinalp, H. L., Kunc, V., Velez-garcia, G. M., Duty, C. E., Love, L. J., Naskar, A. K., et al. (2014). Highly oriented carbon fiber – polymer composites via additive manufacturing. Compos. Sci. Technol. 105, 144–150. doi:10.1016/j.compscitech.2014.10.009
Thomas, S., Joseph, K., and S, M. (2019). Related titles in-situ synthesis of polymer nanocomposites characterization techniques for polymer nanocomposites handbook of biopolymer-based materials modeling and prediction of polymer nanocomposite properties multilayer thin films handbook of biodegra.
Tumbleston, J. R., Shirvanyants, D., Ermoshkin, N., Janusziewicz, R., Johnson, A. R., Kelly, D., et al. (2015). Continuous liquid interface production of 3D objects. Sci. 347, 1349–1352. doi:10.1126/science.aaa2397
Udroiu, R., Braga, I. C., and Nedelcu, A. (2019). Evaluating the quality surface performance of additive manufacturing systems: Methodology and a material jetting case study. Mater. (Basel) 12, 995. doi:10.3390/ma12060995
Villamil Jiménez, J. A., Le Moigne, N., Bénézet, J. C., Sauceau, M., Sescousse, R., and Fages, J. (2020). Foaming of PLA composites by supercritical fluid-assisted processes: A review. Molecules 25, 3408. doi:10.3390/molecules25153408
Voet, V. S. D., Strating, T., Schnelting, G. H. M., Dijkstra, P., Tietema, M., Xu, J., et al. (2018). Biobased acrylate photocurable resin formulation for stereolithography 3D printing. ACS Omega 3, 1403–1408. doi:10.1021/acsomega.7b01648
Wang, J., Mubarak, S., Dhamodharan, D., Divakaran, N., Wu, L., and Zhang, X. (2020a). Fabrication of thermoplastic functionally gradient composite parts with anisotropic thermal conductive properties based on multicomponent fused deposition modeling 3D printing. Compos. Commun. 19, 142–146. doi:10.1016/j.coco.2020.03.012
Wang, S., Zheng, Z., Zhu, C., Ding, Y., and Yu, J. (2018). Crushing and densification of rapid prototyping polylactide foam: Meso-structural effect and a statistical constitutive model. Mech. Mat. 127, 65–76. doi:10.1016/j.mechmat.2018.09.003
Wang, X., Jiang, M., Zhou, Z., Gou, J., and Hui, D. (2017). 3D printing of polymer matrix composites : A review and prospective. Compos. Part B Eng. 110, 442–458. doi:10.1016/j.compositesb.2016.11.034
Wang, Y., Tan, Q., Pu, F., Boone, D., and Zhang, M. (2020b). A review of the application of additive manufacturing in prosthetic and orthotic clinics from a biomechanical perspective. Engineering 6, 1258–1266. doi:10.1016/j.eng.2020.07.019
Weng, Z., Wang, J., Senthil, T., and Wu, L. (2016). Mechanical and thermal properties of ABS/montmorillonite nanocomposites for fused deposition modeling 3D printing. Mat. Des. 102, 276–283. doi:10.1016/j.matdes.2016.04.045
Wicklein, B., Kocjan, A., Salazar-Alvarez, G., Carosio, F., Camino, G., Antonietti, M., et al. (2015). Thermally insulating and fire-retardant lightweight anisotropic foams based on nanocellulose and graphene oxide. Nat. Nanotechnol. 10, 277–283. doi:10.1038/nnano.2014.248
Wirth, D. M., Jaquez, A., Gandarilla, S., Hochberg, J. D., Church, D. C., and Pokorski, J. K. (2020). Highly expandable foam for lithographic 3D printing. ACS Appl. Mat. Interfaces 12, 19033–19043. doi:10.1021/acsami.0c02683
Wong, A., Chu, R. K. M., Leung, S. N., Park, C. B., and Zong, J. H. (2011). A batch foaming visualization system with extensional stress-inducing ability. Chem. Eng. Sci. 66, 55–63. doi:10.1016/j.ces.2010.09.038
Wu, C., Huang, X., Wu, X., Qian, R., and Jiang, P. (2013). Mechanically flexible and multifunctional polymer-based graphene foams for elastic conductors and oil-water separators. Adv. Mat. 25, 5658–5662. doi:10.1002/adma.201302406
Wu, J., Hu, R., Zeng, S., Xi, W., Huang, S., Deng, J., et al. (2020). Flexible and robust biomaterial microstructured colored textiles for personal thermoregulation. ACS Appl. Mat. Interfaces 12, 19015–19022. doi:10.1021/acsami.0c02300
Yang, J., Gu, D., Lin, K., Zhang, Y., Guo, M., Yuan, L., et al. (2022). Laser additive manufacturing of bio-inspired metallic structures. Chin. J. Mech. Eng. Addit. Manuf. Front. 1, 100013. doi:10.1016/j.cjmeam.2022.100013
Yang, S., Leong, K. F., Du, Z., and Chua, C. K. (2001). The design of scaffolds for use in tissue engineering. Part I. Traditional factors. Tissue Eng. 7, 679–689. doi:10.1089/107632701753337645
Yeh, S. K., Liu, Y. C., Chu, C. C., Chang, K. C., and Wang, S. F. (2017). Mechanical properties of microcellular and nanocellular thermoplastic polyurethane nanocomposite foams created using supercritical carbon dioxide. Ind. Eng. Chem. Res. 56, 8499–8507. doi:10.1021/acs.iecr.7b00942
Yin, H., Zhang, W., Zhu, L., Meng, F., Liu, J., and Wen, G. (2023). Review on lattice structures for energy absorption properties. Compos. Struct. 304, 116397. doi:10.1016/j.compstruct.2022.116397
Yu, P., Mi, H. Y., Huang, A., Geng, L. H., Chen, B. Y., Kuang, T. R., et al. (2015). Effect of poly(butylenes succinate) on poly(lactic acid) foaming behavior: Formation of open cell structure. Ind. Eng. Chem. Res. 54, 6199–6207. doi:10.1021/acs.iecr.5b00477
Zhai, W., Jiang, J., and Park, C. B. (2022). A review on physical foaming of thermoplastic and vulcanized elastomers. Polym. Rev. Phila. Pa. 62, 95–141. doi:10.1080/15583724.2021.1897996
Zhang, R. Z., Chen, J., Huang, M. W., Zhang, J., Luo, G. Q., Wang, B. Z., et al. (2019). Synthesis and compressive response of microcellular foams fabricated from thermally expandable microspheres. Chin. J. Polym. Sci. 37, 279–288. doi:10.1007/s10118-019-2187-2
Zhang, S., Shi, X., Miao, Z., Zhang, H., Zhao, X., Wang, K., et al. (2022). 3D-Printed polyurethane tissue-engineering scaffold with hierarchical microcellular foam structure and antibacterial properties. Adv. Eng. Mat. 24, 2101134–2101210. doi:10.1002/adem.202101134
Zhang, X., Fan, W., and Liu, T. (2020). Fused deposition modeling 3D printing of polyamide-based composites and its applications. Compos. Commun. 21, 100413. doi:10.1016/j.coco.2020.100413
Zhang, X., Wang, J., and Liu, T. (2021). 3D printing of polycaprolactone-based composites with diversely tunable mechanical gradients via multi-material fused deposition modeling. Compos. Commun. 23, 100600. doi:10.1016/j.coco.2020.100600
Zhou, C., Yang, K., Wang, K., Pei, X., Dong, Z., Hong, Y., et al. (2016). Combination of fused deposition modeling and gas foaming technique to fabricated hierarchical macro/microporous polymer scaffolds. Mat. Des. 109, 415–424. doi:10.1016/j.matdes.2016.07.094
Zhou, J., and Hsieh, Y. L. (2020). Nanocellulose aerogel-based porous coaxial fibers for thermal insulation. Nano Energy 68, 104305. doi:10.1016/j.nanoen.2019.104305
Zhu, W., Qu, X., Zhu, J., Ma, X., Patel, S., Liu, J., et al. (2017). Direct 3D bioprinting of prevascularized tissue constructs with complex microarchitecture. Biomaterials 124, 106–115. doi:10.1016/j.biomaterials.2017.01.042
Keywords: 3D printing, thermoplastics, foaming, reviews, microcellular foams
Citation: Sun B and Wu L (2022) Research progress of 3D printing combined with thermoplastic foaming. Front. Mater. 9:1083931. doi: 10.3389/fmats.2022.1083931
Received: 29 October 2022; Accepted: 14 November 2022;
Published: 28 November 2022.
Edited by:
Jingjun Wu, Zhejiang University, ChinaReviewed by:
Xu Zhang, Jiangnan University, ChinaMingfeng Chen, Fujian Normal University, China
Nidhin Divakaran, Central Institute of Plastics Engineering and Technology, India
Copyright © 2022 Sun and Wu. This is an open-access article distributed under the terms of the Creative Commons Attribution License (CC BY). The use, distribution or reproduction in other forums is permitted, provided the original author(s) and the copyright owner(s) are credited and that the original publication in this journal is cited, in accordance with accepted academic practice. No use, distribution or reproduction is permitted which does not comply with these terms.
*Correspondence: Lixin Wu, bHh3dUBmamlyc20uYWMuY24=