- 1Department of Chemistry, School of Science, University of Management and Technology, Lahore, Pakistan
- 2Department of Chemistry, School of Natural Sciences (SNS), National University of Science and Technology (NUST), Islamabad, Pakistan
- 3Chemistry Department, Faculty of Science, Jazan University, Jazan, Saudi Arabia
- 4Department of Chemistry, College of Science, Princess Nourah bint Abdul Rahman University, Riyadh, Saudi Arabia
- 5Department of Pharmaceutical Sciences, College of Pharmacy, AlMaarefa University, Riyadh, Saudi Arabia
- 6Department of Chemistry, Faculty of Applied Science, Umm Al-Qura University, Makkah, Saudi Arabia
- 7Department of Chemistry, College of Science, Taif University, Taif, Saudi Arabia
- 8Department of Biotechnology College of Science, Taif University, Taif, Saudi Arabia
- 9Research Center for Advanced Materials Science (RCAMS), King Khalid University, Abha, Saudi Arabia
- 10Department of Physics, Faculty of Science, King Khalid University, Abha, Saudi Arabia
In the modern era, problems like eutrophication caused by increased nutrients such as ammonia and phosphorous in freshwater bodies have become the cause of freshwater ecosystem deterioration. To save freshwater by reducing eutrophication, new cost-effective strategies and methods are urgently needed. In this study, titanium oxide nanoparticles dispersed on zeolite were chemically synthesized for the simultaneous removal of phosphate and ammonium ions from aqueous solutions. SEM and XRD analysis were used to characterize the synthesized TiO2/zeolite nanocomposites, which revealed that the synthesized material was more stable and dispersed than zeolite. The nanocomposites removed 38.8% NH4+ and 98.1% PO43− from an initial concentration of both ions of 20 mg 100 ml−1. The removal of both ions was investigated under various conditions including different concentrations of nanocomposites, initial concentration of the solution, temperature, time, and pH. The maximum adsorption of nanocomposites for PO43- was 38.63 mg g−1 at optimal conditions, and 3.75 mg g−1 for NH4+. Kinematics studies showed that both the ions were adsorbed by a pseudo-second-order model. Ion chemisorption occurred as a result of ligand exchange or electrostatic adsorption between ions and nanocomposites. Overall, it was determined that this strategy is a viable and efficient method for simultaneously removing both ions (anionic phosphate and cationic ammonium) from eutrophic waters.
Highlights
1) Loading of TiO2 nanoparticles on the surface of zeolites by functional group and electrostatic interactions.
2) Greater stability and disperse ability of TiO2/zeolite nanocomposite than natural zeolite.
3) The synthesized system Hybrid TiO2/zeolites showed efficient potential for removing ammonium and phosphate ions from water.
4) It is believed that 20°C is the ideal temperature for removing ammonium and phosphate ions from a mixed solution.
5) The chemisorption process follows a pseudo-second-order kinetic model.
Introduction
Eutrophication has emerged as a major global issue. It occurs when seaweeds form in water as a result of abnormal algae growth. The structure of the ecosystem changes as nutrient salts in the water increase. Human activities have caused a variety of changes in the biosphere, one of which is eutrophication (Howarth et al., 2002; Smith, 2003). When limiting growth ingredients, such as phosphate, are released into water bodies and accumulate there, it results in eutrophication, one of the major environmental challenges. The natural equilibrium of the aquatic ecosystem is impacted by eutrophication conditions, which are marked by an algal bloom followed by oxygen deficiency. Aquatic ecosystems are being harmed by eutrophication (Smith et al., 2006). The removal of phosphorous and nitrogen is critical to preserving the water and the ecosystem’s integrity (Schindler, 2006; Smith & Schlinder, 2009). Nutrient enrichment is caused by factors such as ecosystem stability and the presence of disease-causing agents (Smith & Schlinder, 2009). Phosphorus and nitrogen levels in Pakistan’s rivers, coastal waters, and lakes have increased due to human activities. As a result, eutrophication is on the rise, and toxic algal blooms are on the rise (Søndergaard & Jeppesen, 2007).
Composites are the outstanding materials of the modern world, having two components consisting of continuous and noncontinuous phases. Composites possess the best features due to the reinforcement (less than 100 nm in size) of the material of each component (Ates et al., 2017). Nanocomposites have vast applications in almost every field of life such as automotive, engineering, energy storage, plastic, adhesive, coating, rubber, food, wastewater treatment, electronics, optics, electro analysis, biomedical, etc. TiO2/zeolite nanocomposites synthesized in the present study have large applications but here the main concern is their use in wastewater treatment. The heterointerface between TiO2 nanoparticles and zeolite has proved beneficial particularly in wastewater treatment. TiO2/geopolymer composites are used for the removal of antibiotics from hospital wastewater (Sanguanpak et al., 2022). TiO2/zeolite composites are also used for the removal of atenolol from water by photocatalytic degradation or adsorption. These composites can be reused four times until their activity has been reduced (Stojanović et al., 2022). TiO2/zeolite composite sheet is equipped with an oxidation contractor to remove the organic contaminants from industrially treated water by adsorption (Nomura et al., 2022). TiO2 nanoparticles act as good adsorbers for organic contaminants and inorganic ions. Zeolite is also a natural adsorber and has many applications in wastewater treatment. A Hybrid of both these materials will prove to be more effective in wastewater treatment by adsorption of contaminants. Because dispersion of nanoparticles on zeolite increases the adsorption capacity of zeolite (Xu et al., 2020).
To remove phosphorous and nitrogen from the water, various methods such as adsorption, biological processes, precipitation, crystallization, and ion exchange have been reported (Huo et al., 2012). The best and most efficient way to remove ions is through adsorption (Zheng and Wang, 2010). Activated carbon, copper oxides, iron oxides, titanium oxides, clay minerals, and zeolites are examples of naturally occurring adsorbents that remove phosphorous and nitrogen (Bonetto et al., 2015; Fernández-Catalá et al., 2020). As a result, many researchers are working on developing green materials (Gao et al., 2008) that can be used to remove phosphorous and nitrogen from wastewater at the same time (Wang & Peng, 2010; Liu et al., 2013; Wan et al., 2017). Because they are biocompatible, cost-effective, and environmentally friendly, green synthesized titanium oxide nanoparticles can remove phosphorous and nitrogen. Nanoparticles made from plant extracts (Parsons et al., 2007) are widely used to remove phosphorus (Cao et al., 2016) and nitrogen from water (Devatha et al., 2016). Agglomeration in aqueous solution due to high surface area is a disadvantage of water treatment methods because it reduces dispersibility and reactivity. The dispersion of nanoparticles on natural supports such as zeolite solves this problem.
Zeolites are aluminosilicate minerals that are microporous and frequently utilized as catalyst and adsorbent materials in the industry. Numerous materials have been used to support TiO2, but natural zeolite has emerged as the most preferred of these (Liao et al., 2019). Zeolites are considered to be great supporting materials because of their characteristics like higher cation exchange capacity, microporous structure, and large surface area (Bowman, 2003; Alshameri et al., 2014). Zeolites and modified zeolites play a vital role in the removal of organic, cationic, and anionic pollutants and thus reducing environmental pollution (Wang and Peng, 2010, Ning et al., 2008). Zeolites are appealing adsorbents because they remove phosphorus and ammonia from wastewater effectively and simultaneously (Huang et al., 2014). Secondary chemical reagents should not be used to modify zeolites because this type of modification produces secondary pollution.
Phosphate and ammonium ion adsorption was adsorbed using a variety of materials. Natural zeolite removed 17.68 mg g−1 ammonium and 2.73 mg g−1 phosphate ions in the batch experiments (Wan et al., 2017). Based on these findings, titanium oxide nanoparticle-zeolite (TiO2/Zeolite) nanocomposites were created to determine the factors that influence phosphate and ammonium ion removal efficiency (Belhadj et al., 2022). In order to combat eutrophication in wastewater treatment processes, the systematic, in-depth strategy used in the current experiment with the produced composite material may be crucial (Italiya et al., 2022). This research focused on 1) characterization of TiO2/Zeolite nanocomposites for the recognition of changes in chemical and surface species before and after the calcination process; and 2) batch experiments for the evaluation of phosphate and ammonium ions’ adsorption by TiO2/Zeolite nanocomposites using thermodynamic and adsorption kinetic isotherms.
Experimental
Materials
Titanium Isopropoxide (C12H28O4Ti, 98% Analytical Grade), Urea (CH₄N₂O, 99% Analytical Grade), and Zeolite ((SiO2) x (Al2O3) y 86.33% Analytical Grade were bought from Sigma-Aldrich and used as such. In all the experiments, deionized (DI) water was used.
Preparation of TiO2/Zeolite nanocomposites
Pour 0.5 g Urea into 25 ml deionized water for the synthesis of TiO2/Zeolite Nanocomposites (Figure 1). After 5 min of stirring, 5.5 ml of Titanium Isopropoxide was poured dropwise into the solution. The obtained suspension was placed in a water bath at 50°C for 30 min after stirring it for 30 min. Meanwhile, took 50 ml of deionized water and dissolved 4 g of zeolite in it and the resulting suspension was placed in a 37°C water bath for 30 min. The first suspension was poured dropwise into the zeolite suspension, and the final mixture was stirred for 4 h. The final mixture was cooled at room temperature before being aged for 16 h. The product was washed three times with deionized water after filtration. The filtrate was dried for 2–4 h at 60–70°C. The finished product was placed in a vial for future use. Titanium oxide nanoparticles are charged, and because different functional groups are present on the surface of zeolites, nanoparticles can be loaded on the surface of zeolites by functional group and electrostatic interactions.
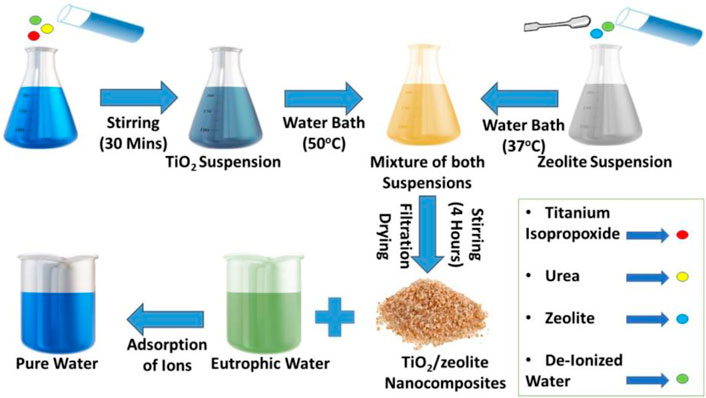
FIGURE 1. The mechanism for the synthesis of TiO2/zeolite nanocomposites and their role in the adsorption of ions.
Characterization
Scanning Electron Microscope was used to examine the particle size distribution and morphology of the synthesized titanium oxide/zeolite nanocomposites (FEI Nova Nano SEM 450, America). An X-Ray Powder Diffractometer (Bruker AXS D8 Advance) with a high-power radioactive source in the 10–80° range of 2θ was used to examine the crystalline structure of synthesized titanium oxide/zeolite nanocomposites.
Batch experiments
Using the following experimental conditions, four batch experiments were performed to investigate the simultaneous adsorption of phosphate and ammonium ions by TiO2/Zeolite nanocomposites. The initial concentrations (20 mg 100 ml−1), pH (6.00), and temperature (30°C) were fixed in the first experiment. However, the concentration of nanocomposites was varied (0.4, 0.6, 0.7, 0.8, and 1 g 100 ml−1). The concentration of nanocomposites (0.7 g 100 ml−1) was fixed in the second experiment, as was the pH (6.00) and temperature (30°C). While, the initial concentrations of the ions (20, 40, 60, 80, and 100 mg 100 ml−1) were altered. The initial concentrations (20 mg 100 ml−1), nanocomposites concentration (0.7 g 100 ml−1), and pH (6.00) were all fixed in the third experiment. However, the temperature (20, 30, and 40°C) was changed. The starting concentrations of the ions (20 mg 100 ml−1), nanocomposites concentration (0.7 g 100 ml−1), and temperature (30°C) were all fixed in the fourth experiment. However, the pH (4.00, 6.00, 8.00, 10.00, and 12.00) was altered. Final solutions were stirred for 30 min in all four experiments to ensure that the maximum amount of phosphate and ammonium ions were adsorbed by TiO2/zeolite nanocomposites. The solutions were filtered after shaking for the desired amount of time to remove the adsorbents. A UV-Spectrophotometer (Metash UV-9000S) was utilized for the observation of the adsorption of ions from the solution before and after the addition of TiO2/zeolite nanocomposites, and all experimental conditions were analyzed to find the best conditions. The efficiency of ion removal from the solution was then found with the help of the following equation:
Here
Results and discussion
Characterization
A small spherical structure of TiO2 nanoparticles with an average size of 50 nm was revealed by SEM images of TiO2/zeolite nanocomposites (Figure 2A). It has been suggested that urea can reduce metal ions to produce nanoparticles. Nanoparticles with an amorphous appearance are evenly distributed above zeolites and dispersed in their pore spaces. The zeolite has the appearance of a hollow tube (Figure 2B). The sizes of the nanocomposites vary. Some particles are large, while others are small. The size mainly depends upon the dispersion of nanoparticles on zeolite. Some particles are large due to more dispersion of nanoparticles on zeolite and some particles are small due to less dispersion. Dispersion of the particles on zeolite varies with the synthetic conditions (Monpezat et al., 2019). The aggregation of high-energy surface particles causes large size. The resulting hybrid product had greater potential and advantages for removing ammonium and phosphate ions from water.
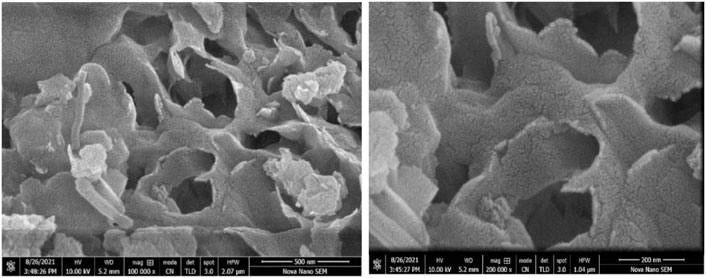
FIGURE 2. Scanning electron microscopy images of TiO2/Zeolite nanocomposites at 500 nm (A) and 200 nm (B).
The XRD pattern of TiO2/zeolite nanocomposites revealed a clear, sharp peak, the values were compared to the JCPDS values (Figure 3). JCPDS value confirmed a broad peak at 2θ = 8.04° as a zeolite characteristic peak (Ren et al., 2018). All of the peaks are rougher, indicating that the surface of the zeolite is covered by a stabilizer or capping agent. In this case, nano titanium acts as a stabilizer or capping agent, roughening the zeolite peaks. Specific peaks at 2θ of 13.16°, 35.6°, and 10–35° have appeared and are due to rutile, anatase, and brookite (TiO2 polymorphs) (Li et al., 2014). The XRD pattern also followed Bragg’s equation (Yuzhen & Shangmin, 1998), indicating that TiO2/zeolite nanocomposites were formed in all aspects.
Batch experiments
Both ammonium (NH4+) and phosphate (PO43−) ions were removed with extremely fast kinetics (Figure 4). The removal efficiency for NH4+ and PO43− ions increased to 27.2 and 89.1%, respectively, within the first 5 min. Within 30 min, however, efficiency increased to 38.8% for NH4+ ions and 94.6% for PO43− ions, respectively. Phosphate removal is more efficient and effective than ammonium ion removal. Furthermore, after 15 min, the removal of ammonium ions became constant, whereas the removal of phosphate took 25 min. The adsorption efficiency increased by increasing the time until the equilibrium is reached (Chen et al., 2011). More the time, more will be the chances of agitation of TiO2 nanoparticles with zeolite (Popuri et al., 2009). But at equilibrium, the surface of the composites is completely blocked by the ions resulting in constant adsorption efficiency. At this point, the adsorption of ions on the surface of the composites becomes equal to the desorption of ions (Dotto & Pinto, 2011). It has been seen that the removal of phosphate ions by these composites is faster. While removal of ammonium ions is also fast but not as efficient as phosphate ions because the removal efficiency also depends upon many factors other than time which is discussed further.
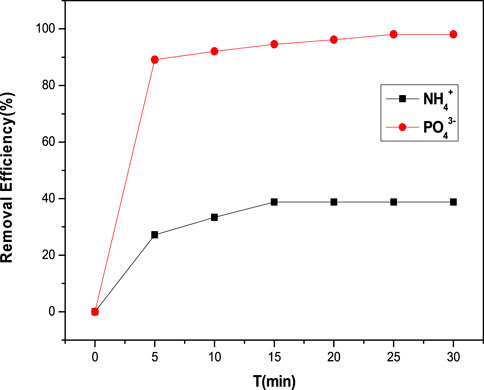
FIGURE 4. Adsorption of phosphate and ammonium ions Requirements: C (PO43- NH4+) = 10 mg 100 ml−1; 30°C; dosage 0.7 g 100 ml−1; 30 min.
In adsorption experiments, increasing the dose of nanocomposites increased the adsorption efficiency of ammonium ions while decreasing the dose of nanocomposites decreased it. However, the adsorption efficiency of phosphate ions was unaffected (Figure 5A). This nanocomposite has a high capacity for phosphate ions but a low capacity for ammonium ions. As a result, increasing the dose of nanocomposites creates more binding sites for ammonium ions. Adsorption efficiency of ammonium and phosphate ions increased from 2.4 to 52.4 percent and 95.9 to 100 percent. By increasing the dose of the nanocomposites, the available surface area increased. As a result, the interactive absorption sites for both ions were expanded, increasing overall efficiency. However, the static unit volume adsorption capacity and adsorbent dose were reduced. If we keep the contaminant concentration constant, increasing the adsorbent dose will increase the points of contact and thus the adsorbability of the nanocomposites (Chen et al., 2013). The adsorbent activity was reduced at the peak value of the adsorbent dose, resulting in fewer binding sites. As a result, the appropriate dosage of nanocomposites is 0.7 g 100 ml−1.
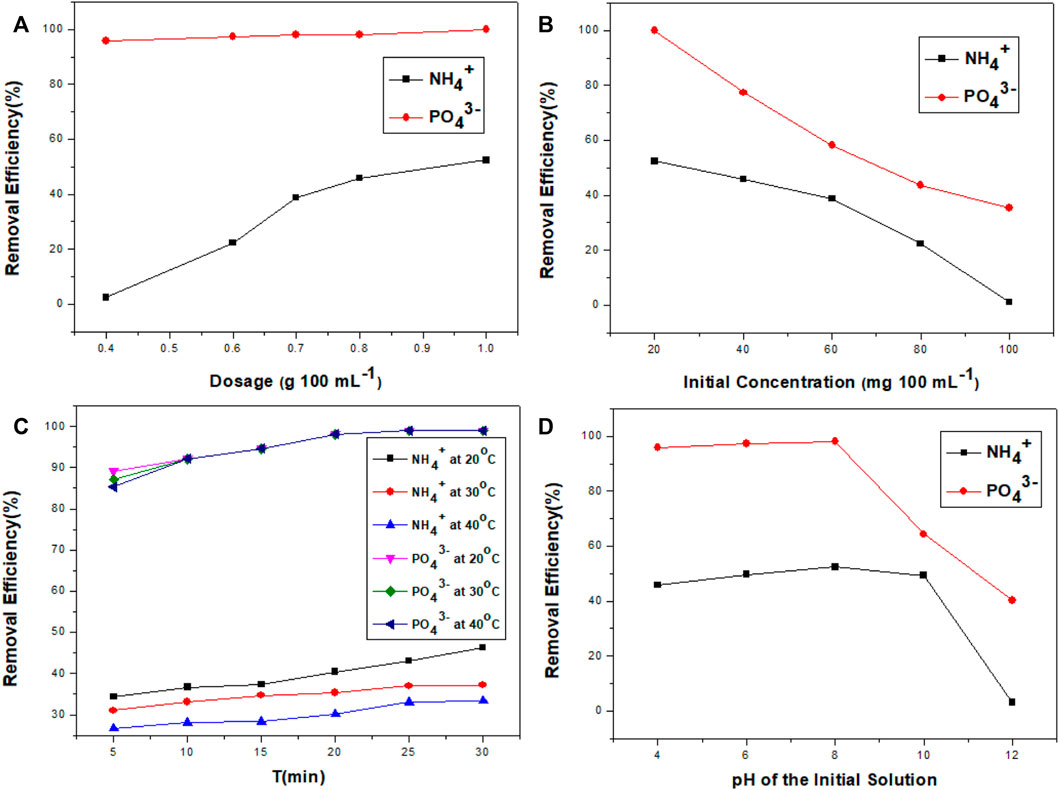
FIGURE 5. Influence of adsorbent dosage (A); starting concentration of contaminants (B); temperature (C); and the pH of the initial solution (D) on the adsorption efficiency of phosphate and ammonium ions by TiO2/zeolite nanocomposites.
When the concentrations of the ions were raised from 20 to 100 mg 100 ml−1, the removal efficiency of ammonium ions decreased from 52.4 to 1% and for phosphate ions from 100 to 35.4 percent (Figure 5B). Because of the limited adsorption sites on TiO2/zeolite nanocomposites, this decrease occurred. In this experiment, the adsorbent dose was held constant while the number of contaminants increased. As a result, competitive adsorption between contaminants occurred (Wan et al., 2017). As a result, adsorption efficiency is reduced. When the initial concentration is lower, more adsorption sites are available to remove contaminants from the nanocomposites’ surface. As a result, maximum adsorption took place. When the initial concentration is higher, however, the minimum adsorption sites are available to remove contaminants from the nanocomposites’ surface. As a result, there was minimal adsorption.
At the optimum temperature, the majority of experiments yield satisfactory results. The removal of ammonium and phosphate ions was studied at three temperatures for the determination of the optimal temperature for ion adsorption. At these three temperatures, the removal efficiencies of ammonium ions were 34.4, 31.0, and 26.7 percent in the first 5 min. However, after 30 min of reaction equilibrium, the ammonium ion removal efficiencies stabilized at 46.3, 37.2, and 33.4 percent, respectively. While phosphate ion removal was high, at 99 percent at all three temperatures, and remained stable (Figure 5C). Because ammonium ion adsorption was an exothermic process, the removal of ammonium ions decreased as the temperature increased. As a result, at higher temperatures, ammonium ion removal was not favored. However, temperature change had not affected the adsorption of phosphate ions. The optimal temperature for the removal of phosphate and ammonium ions from a mixed solution is thought to be 20°C.
At high pH levels, the adsorption efficiency of phosphate and ammonium ions is greatly influenced (Figure 5D). Phosphate adsorption was unaffected until the pH reached 8.00. However, if the pH value raised from 8.00 to 12.00, the phosphate removal decreased from 98.1 to 40.2 percent. The ammonium removal was 52.4 percent at pH 4.00. It stayed at this level until pH 10.00. The removal of ammonium ions dropped to 3.1 percent at pH 12.00, as shown below (Figure 5D). Because of the following reason, the removal efficiency varied with pH. The adsorption sites on the nanocomposites were in a more deprotonated state between pH 4.00 and 10.00 of an aqueous solution, producing a more negative surface charge due to the following mechanism:
Ammonium ions can interact more with maximum anion binding sites at pH 4.00 to 10.00. However, at lower pH, more H+ ions were produced, which competed with NH4+ for adsorption sites and reduced ammonium ion removal efficiency (Huang et al., 2014; Wan et al., 2017). At higher pH, more OH− ions were produced, which competed with PO43− for adsorption sites and reduced phosphate ion removal efficiency. An optimal pH of 6.00 is required for ion removal.
Adsorption kinetics, thermodynamics, and isothermal studies
For a better calculation of adsorption and kinetic isothermal models, different values of removal efficiencies of ammonium and phosphate ions at three temperatures were analyzed for 20–30 h (Figure 6).
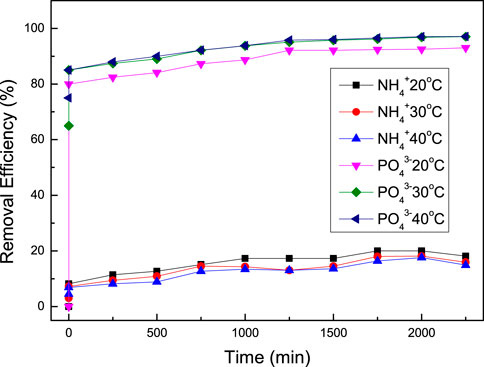
FIGURE 6. Variation in adsorption efficiency of PO43- and NH4+ with TiO2/zeolite nanocomposites at temperatures (20, 30 & 40°C). Conditions: concentration of ions (20 mg 100 ml−1), dose (1 g 100 ml−1) and pH (6.00).
Pseudo-first-order and second-order kinetic models were applied for the study of the adsorption mechanism.
Where qt and qe are adsorption capacities at the time (min) and equilibrium and k1 (min−1) and k2 (g mg−1 h−1) are the pseudo-first-order adsorption and pseudo-second-order adsorption rate constants, respectively. Table 1 shows the proper values for all parameters.
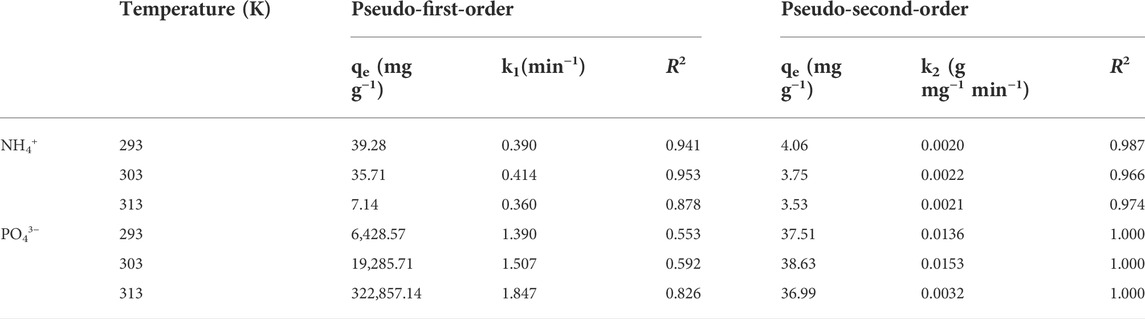
TABLE 1. Values of adsorption kinetics for co-adsorption of phosphate and ammonium ions on TiO2/zeolite nanocomposites at three temperatures.
The pseudo-first-order correlation coefficient (R2) values for NH4+ and PO43− are 0.941, 0.953, 0.878 and 0.553, 0.592, 0.862, respectively. While the pseudo-second-order values for NH4+ and PO43− are 0.987, 0.966, 0.974 and 1.000, 1.000, 1.000, respectively. As a result, it was determined that pseudo-second order is a more consistent kinetic model for hybrids’ removal behavior. Both the correlation coefficient (R2) values for NH4+ and PO43− ions at three temperatures are greater than 0.990, indicating that these ions are removed entirely by chemical adsorption. Values of correlation coefficient at lower temperature are greater than 0.990 suggested that the adsorption process was not first order reaction but followed the pseudo second order kinetic model (Wu et al., 2009). The adsorption process took place in many steps and the rate determining step involved the sharing or exchange of electrons suggesting that the adsorption process was mainly chemisorption. The adsorption of the ions followed an exothermic reaction and should be taken place at lower temperature for the maximum adsorption of the ions.
Thus, high values of correlation coefficient for ions are due to the pseudo second order kinetics and chemisorption exothermic process (Ho & McKay, 1999). As a result of the interaction between ions and nanocomposites, good removal efficiency of both NH4+ and PO43 ions was observed. This interaction occurred via ligand exchange or electron sharing between NH4+—PO43− ions and nanocomposites. The rate constants k2 of NH4+ ions for pseudo-second-order remained unaffected by increasing the temperature, but PO43- ions varied more efficiently. It was discovered that this method is faster, more practical, and simple for controlling pollution caused by these ions. The temperature had not affected the removal of NH4+ ions with nanocomposites; it demonstrated that NH4+ ion adsorption with nanocomposites remained stable at these three temperatures. While increasing the temperature reduced PO43- ion adsorption with nanocomposites, it revealed that PO43− ion adsorption with nanocomposites is an exothermic process. At a lower temperature, it would remain stable.
The adsorption data were also fitted into Freundlich and Langmuir equations (Table 2) to determine the precise adsorption capacity of the nanocomposites for these two water pollutants (Zhang et al., 2011). Eq. 6 depicts the Freundlich model equation:

TABLE 2. Isotherm co-adsorption values of phosphate and ammonium ions on TiO2/zeolite nanocomposites.
While qe is called equilibrium adsorption capacity (mg g−1), KF is known as the Freundlich equilibrium constant (L g−1), 1/n is constant for adsorption capacity, and Ce is NH4+ and PO43− equilibrium concentration (mg L−1).
While Eq. 7 is used to express the Langmuir isotherm model:
KL (L mg−1) is called the Langmuir adsorption constant and qm (mg g−1) is known as the maximum adsorption capacity.
Based on correlation coefficient (R2) values for the Freundlich & Langmuir models, ammonium and phosphate ions adsorb on nanocomposites via a monolayer adsorption process. Because ammonium and phosphate ions have a positive and a negative charge, NH4+ is less adsorbed on nanocomposites than PO43−. Adsorption is aided by negative charges. The 1/n values of ammonium and phosphate ions are both very low, indicating that the adsorption process was mainly chemical.
Kd (Thermodynamic distribution coefficient), ΔG° (kJ mol−1 Gibbs free energy), ΔH° (kJ mol−1 Enthalpy change) and ΔS° (J mol−1 K−1 Entropy change) were used for the calculation of thermodynamic values by using Eqs 8, 9:
When a graph was plotted between ln Kc and 1/T, ΔS°, Δ,H°, and ΔG° parameters were found from the intercept and slope of the graph (Table 3).

TABLE 3. Thermodynamic values for co-adsorption of phosphate and ammonium ions on TiO2/zeolite nanocomposites.
The ΔH° and ΔS° values for ammonium ion adsorption confirmed that the process of adsorption of these ions was exothermic. ΔG° negative values for ammonium ion adsorption confirmed that this was a highly favorable and spontaneous process. Phosphate ion adsorption ΔH°, ΔS°, and ΔG° values confirmed that the process was exothermic and spontaneous. ΔG° values decreased as temperature increased, confirming that higher temperatures were unfavorable for the removal process. The above calculations and conclusions confirmed that the removal of NH4+ by TiO2/zeolite nanocomposites was a physical adsorption process because the surface of the nanocomposites has a negative charge in water that electrostatically attracts NH4+ ions. While phosphate adsorption was caused by the addition of nanocomposites to water, Ti-OH bonds form as a result of hydrolysis at the titanium surface. TiPO42− is formed when this bond exchanges with phosphate.
Evaluation of adsorption measurements
TiO2/zeolite nanocomposites absorbed fewer ammonium ions (3.75 mg g−1) than natural zeolite (17.68 mg g−1), modified zeolite combined with struvite crystallization (17.55 mg g−1), and iron nanoparticles (9.70 mg g−1), but more than eucalyptus magnetic nanocomposites (3.47 mg g−1). Phosphate adsorption by TiO2/zeolite nanocomposites (38.63 mg g−1) was greater than that of natural zeolite (2.73 mg g−1) and modified zeolite combined with struvite crystallization (8.25 mg g−1), but it was slightly lower or nearly equal to that of eucalyptus magnetic nanoparticles dispersed on zeolite (38.91 mg g−1) (Huang et al., 2014; Wang et al., 2014; Wan et al., 2017; Xu et al., 2020). The prepared TiO2/zeolite nanocomposites have selective adsorption for NH4+ and PO43−, with PO43−adsorption being greater than NH4+ adsorption.
Conclusion
TiO2/Zeolite nanocomposites were chemically synthesised in this study, where titanium nanoparticles were successfully dispersed on zeolite. The role of zeolite as an inorganic carrier improved titanium nanoparticle dispersion. Titanium nanoparticles were also protected from oxidation and agglomeration by zeolite. Furthermore, it enhanced the number of adsorption sites, which improved the simultaneous adsorption of NH4+ and PO43−. The removal process depends upon factors like time, adsorbent concentration, pH, and temperature. Removal efficiency increased by increasing the agitation time but became constant after some time. The removal process is primarily determined by the adsorbent concentration because more concentration increased the adsorption sites but adsorption decreased as the reaction pH increased (pH > 8.00). PO43− and NH4+ removal efficiencies were 98.1 percent and 38.8 percent, respectively. The adsorption process was exothermic, and TiO2/Zeolite nanocomposites were more effective at removing both ions at low temperatures (20°C). The process of adsorption followed pseudo-second-order kinetics and was primarily chemical in nature.
Data availability statement
The original contributions presented in the study are included in the article/Supplementary Material, further inquiries can be directed to the corresponding authors.
Author contributions
All authors listed have made a substantial, direct, and intellectual contribution to the work and approved it for publication.
Funding
The authors would like to thank the Deanship of Scientific Research at Umm Al-Qura University for supporting this work by Grant Code: (22UQU4320141DSR18). This work was supported by King Khalid University through a grant (KKU/RCAMS/22) under the Research Center for Advanced Materials Science (RCAMS) at King Khalid University, Saudi Arabia. This research was funded by Princess Nourah bint Abdulrahman University Researchers Supporting Project number (PNURSP2022R134), Princess Nourah bint Abdulrahman University, Riyadh, Saudi Arabia.
Acknowledgments
The authors would like to thank the Deanship of Scientific Research at Umm Al-Qura University for supporting this work by Grant Code: (22UQU4320141DSR18). Email: cmFwYXNoYUB1cXUuZWR1LnNh. This work was supported by King Khalid University through a grant (KKU/RCAMS/22) under the Research Center for Advanced Materials Science (RCAMS) at King Khalid University, Saudi Arabia. This research was funded by Princess Nourah bint Abdulrahman University Researchers Supporting Project number (PNURSP2022R134), Princess Nourah bint Abdulrahman University, Riyadh, Saudi Arabia.
Conflict of interest
The authors declare that the research was conducted in the absence of any commercial or financial relationships that could be construed as a potential conflict of interest.
Publisher’s note
All claims expressed in this article are solely those of the authors and do not necessarily represent those of their affiliated organizations, or those of the publisher, the editors and the reviewers. Any product that may be evaluated in this article, or claim that may be made by its manufacturer, is not guaranteed or endorsed by the publisher.
References
Alshameri, A., Yan, C., and Lei, X. (2014). Enhancement of phosphate removal from water by TiO2/Yemeni natural zeolite: Preparation, characterization and thermodynamic. Microporous Mesoporous Mat. 196, 145–157. doi:10.1016/j.micromeso.2014.05.008
Ates, B., Koytepe, S., Balcioglu, S., Ulu, A., and Gurses, C. (2017). “Biomedical applications of hybrid polymer composite materials,” in Hybrid Polymer composite material (Sawston, Cambridge: Woodhead Publishing), 343–408.
Belhadj, H., Moulefera, I., Sabantina, L., and Benyoucef, A. (2022). Effects of incorporating titanium dioxide with titanium carbide on hybrid materials reinforced with polyaniline: Synthesis, characterization, electrochemical and supercapacitive properties. Fibers 10 (5), 46. doi:10.3390/fib10050046
Bonetto, L. R., Ferrarini, F., De Marco, C., Crespo, J. S., Guégan, R., and Giovanela, M. (2015). Removal of methyl violet 2B dye from aqueous solution using a magnetic composite as an adsorbent. J. Water Process Eng. 6, 11–20. doi:10.1016/j.jwpe.2015.02.006
Bowman, R. S. (2003). Applications of surfactant-modified zeolites to environmental remediation. Microporous Mesoporous Mat. 61 (1-3), 43–56. doi:10.1016/s1387-1811(03)00354-8
Cao, D., Jin, X., Gan, L., Wang, T., and Chen, Z. (2016). Removal of phosphate using iron oxide nanoparticles synthesized by eucalyptus leaf extract in the presence of CTAB surfactant. Chemosphere 159, 23–31. doi:10.1016/j.chemosphere.2016.05.080
Chen, Y. G., Ye, W. M., Yang, X. M., Deng, F. Y., and He, Y. (2011). Effect of contact time, pH, and ionic strength on Cd (II) adsorption from aqueous solution onto bentonite from Gaomiaozi, China. Environ. Earth Sci. 64 (2), 329–336. doi:10.1007/s12665-010-0850-6
Chen, Z., Wang, T., Jin, X., Chen, Z., Megharaj, M., and Naidu, R. (2013). Multifunctional kaolinite-supported nanoscale zero-valent iron used for the adsorption and degradation of crystal violet in aqueous solution. J. Colloid Interface Sci. 398, 59–66. doi:10.1016/j.jcis.2013.02.020
Devatha, C. P., Thalla, A. K., and Katte, S. Y. (2016). Green synthesis of iron nanoparticles using different leaf extracts for treatment of domestic wastewater. J. Clean. Prod. 139, 1425–1435. doi:10.1016/j.jclepro.2016.09.019
Dotto, G. L., and Pinto, L. D. A. (2011). Adsorption of food dyes onto chitosan: Optimization process and kinetic. Carbohydr. Polym. 84 (1), 231–238. doi:10.1016/j.carbpol.2010.11.028
Fernández-Catalá, J., Sanchez-Rubio, M., Navlani-García, M., Berenguer-Murcia, Á., and Cazorla-Amorós, D. (2020). Synthesis of TiO2/nanozeolite composites for highly efficient photocatalytic oxidation of propene in the gas phase. ACS omega 5 (48), 31323–31331. doi:10.1021/acsomega.0c04793
Gao, S., Shi, Y., Zhang, S., Jiang, K., Yang, S., Li, Z., et al. (2008). Biopolymer-assisted green synthesis of iron oxide nanoparticles and their magnetic properties. J. Phys. Chem. C 112 (28), 10398–10401. doi:10.1021/jp802500a
Ho, Y. S., and McKay, G. (1999). Pseudo-second order model for sorption processes. Process Biochem. 34 (5), 451–465. doi:10.1016/s0032-9592(98)00112-5
Howarth, R. W., Sharpley, A., and Walker, D. (2002). Sources of nutrient pollution to coastal waters in the United States: Implications for achieving coastal water quality goals. Estuaries 25 (4), 656–676. doi:10.1007/bf02804898
Huang, H., Xiao, D., Pang, R., Han, C., and Ding, L. (2014). Simultaneous removal of nutrients from simulated swine wastewater by adsorption of modified zeolite combined with struvite crystallization. Chem. Eng. J. 256, 431–438. doi:10.1016/j.cej.2014.07.023
Huo, H., Lin, H., Dong, Y., Cheng, H., Wang, H., and Cao, L. (2012). Ammonia-nitrogen and phosphates sorption from simulated reclaimed waters by modified clinoptilolite. J. Hazard. Mat. 229, 292–297. doi:10.1016/j.jhazmat.2012.06.001
Italiya, G., Ahmed, M. H., and Subramanian, S. (2022). Titanium oxide bonded Zeolite and Bentonite composites for adsorptive removal of phosphate. Environ. Nanotechnol. Monit. Manag. 17, 100649. doi:10.1016/j.enmm.2022.100649
Li, W., Liang, R., Hu, A., Huang, Z., and Zhou, Y. N. (2014). Generation of oxygen vacancies in visible light activated one-dimensional iodine TiO2 photocatalysts. RSC Adv. 4 (70), 36959–36966. doi:10.1039/c4ra04768k
Liao, G., He, W., and He, Y. (2019). Investigation of microstructure and photocatalytic performance of a modified zeolite supported nanocrystal TiO2 composite. Catalysts 9 (6), 502. doi:10.3390/catal9060502
Liu, H., Chen, T., Zou, X., Xie, Q., Qing, C., Chen, D., et al. (2013). Removal of phosphorus using NZVI derived from reducing natural goethite. Chem. Eng. J. 234, 80–87. doi:10.1016/j.cej.2013.08.061
Monpezat, A., Topin, S., Thomas, V., Pagis, C., Aouine, M., Burel, L., et al. (2019). Migration and growth of silver nanoparticles in Zeolite socony mobil 5 (ZSM-5) observed by environmental electron microscopy: Implications for heterogeneous catalysis. ACS Appl. Nano Mat. 2 (10), 6452–6461. doi:10.1021/acsanm.9b01407
Nomura, Y., Koga, K., Ohnishi, K., Fukahori, S., and Fujiwara, T. (2022). Inactivation of plant pathogenic bacterium Ralstonia solanacearum in drainage solution from hydroponic system by a rotating advanced oxidation contactor equipped with TiO2/zeolite composite sheets. J. Water Process Eng. 48, 102936. doi:10.1016/j.jwpe.2022.102936
Parsons, J. G., Peralta-Videa, J. R., and Gardea-Torresdey, J. L. (2007). Use of plants in biotechnology: Synthesis of metal nanoparticles by inactivated plant tissues, plant extracts, and living plants. J. Environ. Dev. 5, 463–485. doi:10.1016/S1474-8177(07)05021-8
Popuri, S. R., Vijaya, Y., Boddu, V. M., and Abburi, K. (2009). Adsorptive removal of copper and nickel ions from water using chitosan coated PVC beads. Bioresour. Technol. 100 (1), 194–199. doi:10.1016/j.biortech.2008.05.041
Ren, X., Xiao, L., Qu, R., Liu, S., Ye, D., Song, H., et al. (2018). Synthesis and characterization of a single-phase zeolite A using coal fly ash. RSC Adv. 8 (73), 42200–42209. doi:10.1039/c8ra09215j
Sanguanpak, S., Shongkittikul, W., Saengam, C., Chiemchaisri, W., and Chiemchaisri, C. (2022). TiO2-immobilized porous geopolymer composite membrane for removal of antibiotics in hospital wastewater. Chemosphere 307, 135760. doi:10.1016/j.chemosphere.2022.135760
Schindler, D. W. (2006). Recent advances in the understanding and management of eutrophication. Limnol. Oceanogr. 51, 356–363. doi:10.4319/lo.2006.51.1_part_2.0356
Smith, V. H. (2003). Eutrophication of freshwater and coastal marine ecosystems a global problem. Environ. Sci. Pollut. Res. 10 (2), 126–139. doi:10.1065/espr2002.12.142
Smith, V. H., Joye, S. B., and Howarth, R. W. (2006). Eutrophication of freshwater and marine ecosystems. Limnol. Oceanogr. 51, 351–355. doi:10.419/lo.2006.51.1_part_2.0351
Smith, V. H., and Schindler, D. W. (2009). Eutrophication science: Where do we go from here? Trends Ecol. Evol. 24 (4), 201–207. doi:10.1016/j.tree.2008.11.009
Søndergaard, M., and Jeppesen, E. (2007). Anthropogenic impacts on lake and stream ecosystems, and approaches to restoration. J. Appl. Ecol. 44, 1089–1094. doi:10.1111/j.1365-2664.2007.01426.x
Stojanović, S., Vranješ, M., Šaponjić, Z., Rac, V., Rakić, V., Ignjatović, L., et al. (2022). Photocatalytic performance of TiO2/zeolites under simulated solar light for removal of atenolol from aqueous solution. Int. J. Environ. Sci. Tech., 1–16.
Wan, C., Ding, S., Zhang, C., Tan, X., Zou, W., Liu, X., et al. (2017). Simultaneous recovery of nitrogen and phosphorus from sludge fermentation liquid by zeolite adsorption: Mechanism and application. Sep. Purif. Technol. 180, 1–12. doi:10.1016/j.seppur.2017.02.031
Wang, S., and Peng, Y. (2010). Natural zeolites as effective adsorbents in water and wastewater treatment. Chem. Eng. J. 156 (1), 11–24. doi:10.1016/j.cej.2009.10.029
Wang, T., Lin, J., Chen, Z., Megharaj, M., and Naidu, R. (2014). Green synthesized iron nanoparticles by green tea and eucalyptus leaves extracts used for removal of nitrate in aqueous solution. J. Clean. Prod. 83, 413–419. doi:10.1016/j.jclepro.2014.07.006
Weng, X., Huang, L., Chen, Z., Megharaj, M., and Naidu, R. (2013). Synthesis of iron-based nanoparticles by green tea extract and their degradation of malachite. Ind. Crops Prod. 51, 342–347. doi:10.1016/j.indcrop.2013.09.024
Wu, F. C., Tseng, R. L., Huang, S. C., and Juang, R. S. (2009). Characteristics of pseudo-second-order kinetic model for liquid-phase adsorption: A mini-review. Chem. Eng. J. 151 (1-3), 1–9. doi:10.1016/j.cej.2009.02.024
Xu, Q., Li, W., Ma, L., Cao, D., Owens, G., and Chen, Z. (2020). Simultaneous removal of ammonia and phosphate using green synthesized iron oxide nanoparticles dispersed onto zeolite. Sci. Total Environ. 703, 135002. doi:10.1016/j.scitotenv.2019.135002
Yuzhen, Y., and Shangmin, D. (1998). The application of Bragg’s equation in the study of polymer structure. Eng. Phy. 8 (3), 34–35.
Zhang, X., Lin, S., Chen, Z., Megharaj, M., and Naidu, R. (2011). Kaolinite-supported nanoscale zero-valent iron for removal of Pb2+ from aqueous solution: Reactivity, characterization, and mechanism. Water Res. 45 (11), 3481–3488. doi:10.1016/j.watres.2011.04.010
Keywords: phosphate, ammonium, eutrophication, titanium oxide nanocomposites, zeolite
Citation: Ashfaq MH, Shahid S, Javed M, Iqbal S, Hakami O, Aljazzar SO, Fatima U, Elkaeed EB, Pashameah RA, Alzahrani E, Farouk A-E and Somaily HH (2022) Controlled growth of TiO2/Zeolite nanocomposites for simultaneous removal of ammonium and phosphate ions to prevent eutrophication. Front. Mater. 9:1007485. doi: 10.3389/fmats.2022.1007485
Received: 30 July 2022; Accepted: 25 August 2022;
Published: 28 September 2022.
Edited by:
Muhammad Sufyan Javed, Lanzhou University, ChinaReviewed by:
Imtiaz Khan, The University of Manchester, United KingdomMuhammad Yaqub, Kumoh National Institute of Technology, South Korea
Abdelghani Benyoucef, University of Mascara, Algeria
Copyright © 2022 Ashfaq, Shahid, Javed, Iqbal, Hakami, Aljazzar, Fatima, Elkaeed, Pashameah, Alzahrani, Farouk and Somaily. This is an open-access article distributed under the terms of the Creative Commons Attribution License (CC BY). The use, distribution or reproduction in other forums is permitted, provided the original author(s) and the copyright owner(s) are credited and that the original publication in this journal is cited, in accordance with accepted academic practice. No use, distribution or reproduction is permitted which does not comply with these terms.
*Correspondence: Mohsin Javed, bW9oc2luLmphdmVkQHVtdC5lZHUucGs=; Shahid Iqbal, c2hhaGlkZ2NzMTBAeWFob28uY29t