- 1Centro de Química Estrutural-CQE, DEQ, Instituto Superior Técnico, Universidade de Lisboa, Lisbon, Portugal
- 2ESTSetúbal, CDP2T, Instituto Politécnico de Setúbal, Setúbal, Portugal
Magnesium and magnesium alloys have attracted growing attention over the last decades as lightweight materials for a wide range of applications. In particular, WE series magnesium alloys have experienced growing interest over the last years due to their favourable mechanical properties at room and elevated temperatures. In addition, it has been reported that these rare earth-containing alloys possess superior corrosion resistance compared to other commonly used magnesium alloys, such as AZ series. This review aims at providing a concise overview of the research efforts made during recent years regarding the properties of WE series magnesium alloys (e.g., mechanical properties, corrosion behaviour), how these properties can be enhanced by controlling the microstructure of these materials, and the role of specific alloying elements that are used for the WE series. The widespread use of these materials has been limited, mainly due to their susceptibility to corrosion. Thus, in the present review, strong emphasis has been given to recent work studying the corrosion behaviour of the WE series alloys, and to protective strategies that can be employed to mitigate their degradation.
Introduction
Magnesium and Magnesium Alloys
Magnesium Alloys as Lightweight Solution
Growing environmental concerns have been leading to the need for reduction of greenhouse gas emissions into the atmosphere. This reduction in emissions has been heavily regulated for several industries. In particular, the automotive and aeronautic sectors are expected to meet specific targets over the next years (European Union, 2009; European Union, 2014). Thus, substantial research efforts regarding possible solutions for mitigation of greenhouse gas emissions, such as improved component design and performance, and fuel efficiency improvement, have been made in these industries. Weight reduction of components has been regarded as the most simple and cost-effective solution to improve fuel efficiency and reduce greenhouse gas emissions (Grote et al., 2014; Kiani et al., 2014; Esmaily et al., 2017). To meet this goal, the use of lightweight magnesium alloys represents an interesting approach for component weight reduction, since magnesium is the lightest structural metal. With densities ranging between 1.74 and 1.81 g/cm3, magnesium and magnesium alloys are lighter than the most commonly used materials in the automotive and aeronautic industries, such as aluminium (density of 2.7 g/cm3) and steel (density of 7.86 g/cm3) (Kulekci, 2008; Prabhu et al., 2017). Furthermore, magnesium alloys possess high strength-to-weight ratio, good castability, and good damping capacity, while being non-toxic and recyclable (Čížek et al., 2004; Kulekci, 2008).
The Challenges
Despite the advantages of magnesium alloys for overall structural weight reduction, the widespread use of these materials has been limited, mainly due to their high susceptibility to corrosion, and, to some extent, due to inferior mechanical properties compared to other structural metals. Therefore, magnesium alloys are currently used for non-structural applications. In the automotive industry, magnesium alloys are used, for instance, for manual transmission housings, steering wheels, and clutch and brake pedal support brackets, while the aeronautic industry makes use of magnesium alloys for aircraft door panels, gearboxes, and transmission casings, for example (Kulekci, 2008; James et al., 2011; Mirza et al., 2013; Czerwinski, 2014; Moosbrugger, 2017). Other applications for magnesium alloys include frames for eyeglasses, electronic devices, and luggage (Callister and Rethwisch, 2014). Furthermore, in recent years, magnesium alloys have been intensively studied as materials for biodegradable implants (Córdoba et al., 2016) due to their high biocompatibility. However, the high corrosion susceptibility of these materials is a concern for biomedical applications as well. Different strategies have been used to mitigate this issue and, as a consequence, magnesium alloys are gaining interest as bioresorbable materials (Zomorodian et al., 2013; Zomorodian et al., 2015).
Alloying Magnesium
Over the past years, many different magnesium alloy systems have been developed as an effort to improve the mechanical properties and corrosion resistance of these lightweight materials. To date, the coding system developed by the American Society for Testing and Materials (ASTM) has been the most widely used both in industry and in research (Moosbrugger, 2017; Mouritz, 2012). It is an alphanumeric system, in which each alloy designation is composed of four main parts, in the following order: two letters indicating the main alloying elements; two numbers indicating the percentage of each of the main alloying elements (rounded-off); one letter to distinguish different alloys that differ only in type and amount of minor alloying elements; and temper designation (Moosbrugger, 2017). The lettering code for alloying elements in magnesium alloys is shown in Table 1. For example, magnesium alloy WE43C-T5 has yttrium (W) and rare earths (E) as main alloying elements, in approximate amounts of 4 wt.% and 3 wt.%, respectively. The letter C indicates that it is the third composition of this alloy that became standard, and T5 designates the temper condition (Moosbrugger, 2017).
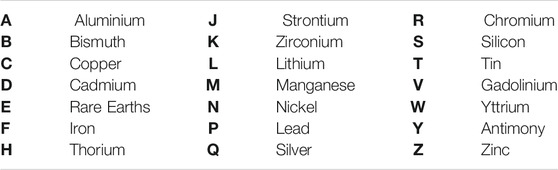
TABLE 1. Lettering code for main alloying elements in magnesium alloys. Data based on (Moosbrugger, 2017).
The most common magnesium alloys belong to the AZ series. These alloys typically consist of a α-Mg matrix and intermetallic β-Mg17Al12 phase, distributed along the α grain boundaries (Song et al., 1999). These intermetallic particles have a strengthening effect, and the presence of aluminium-containing β-phase increases the corrosion resistance of the magnesium alloy (Song and Atrens, 1999; Mouritz, 2012). The presence of zinc is believed to enhance the tolerance of magnesium alloys for impurity elements, such as nickel and iron (Song and Atrens, 1999; Kabirian and Mahmudi, 2009). However, the use of these types of alloys is limited to low-temperature applications due to deterioration of alloy properties at temperatures above 120°C, ascribed to the softening of the β-Mg17Al12 phase at high temperatures (Ghali and Revie, 2011; Mokhtarishirazabad et al., 2013). This issue can be overcome by the introduction of other alloying elements, such as calcium or rare earth elements (REEs), thereby creating thermally stable precipitates (Mokhtarishirazabad et al., 2013). Another problem associated with the AZ series is the fact that their toughness and ductility tend to be low (Mouritz, 2012). For this reason, magnesium alloys with a reduced aluminium content were developed, namely AM60 and AM50, which display improved toughness and ductility due to a reduction of Mg17Al12 intermetallic particles (Magnesium Alloy Fatigue Data, 1995).
Most magnesium alloys possess inferior mechanical properties than other commonly used structural metals such as aluminium, steel, and titanium (Callister and Rethwisch, 2014; Prabhu et al., 2017). Compared to these widely used materials, magnesium alloys generally possess lower strength, creep resistance, fatigue properties, and high-temperature capability (Toda-Caraballo et al., 2014; Manakari et al., 2017; Kujur et al., 2018). Furthermore, the flammability, and ignition behaviour of magnesium alloys can also constitute a downside to their use for many potential applications (Czerwinski, 2014).
The use of different alloying elements has been an effective way to improve the properties of magnesium alloys. For example, zinc is often used to improve the strength of magnesium-based materials, leading to grain refinement and precipitation strengthening (Gupta and Sharon, 2011; Hu et al., 2018; Juan et al., 2021). Creep resistance and thermal stability of magnesium alloys can be enhanced with the addition of calcium (Li et al., 2016; Kondori and Mahmudi, 2017; Incesu and Gungor, 2020). The use of calcium as alloying element leads to the formation of Al2Ca secondary phase, replacing the thermally unstable β-Mg17Al12 phase. Furthermore, calcium also improves the biocompatibility of the alloys (Esmaily et al., 2017). Besides alloying, the properties of magnesium alloys can also be improved with addition of nanoparticles. Metal oxide nanoparticles such as ZnO (Tun et al., 2012) or CeO2 (Kujur et al., 2018) have been shown to improve the strength of magnesium alloys, while SiC nanoparticles have been studied as reinforcements to improve the creep resistance (Ganguly and Mondal, 2018). These types of nanoparticles hinder dislocation motions, thereby reinforcing the alloy (Ganguly and Mondal, 2018; Kujur et al., 2018). The improvement of magnesium alloy properties with addition of rare earth elements has been described since the 1930s (Luo, 2004). For example, cerium can improve deformability at room temperature (Wang et al., 2019a), addition of neodymium has been shown to decrease the corrosion rate (Zhang et al., 2011; Arrabal et al., 2012), and yttrium has been found to improve the ultimate tensile strength and elongation of magnesium alloys (Li et al., 2007).
Corrosion of Magnesium and Magnesium Alloys
Corrosion can be defined as the degradation of a material and its properties due to interaction with its environment (Montemor, 2014). To this regard, magnesium is an extremely reactive material. It is anodic to almost all metals and will, therefore, corrode preferentially when present in any galvanic couple. Corrosion of magnesium in aqueous media involves metal dissolution and water reduction, via the following generally accepted reaction (Makar and Kruger, 1993):
During magnesium corrosion, formation of a magnesium hydroxide film on the surface of the metal occurs, accompanied by local alkalization (Makar and Kruger, 1993). The global corrosion reaction of magnesium (Eq. 1) can be divided into an anodic and a cathodic reaction (Feliu and Llorente, 2015):
Some authors consider that the magnesium oxidation reaction (Eq. 2) could be a two-step process, involving the formation and hydrolysis of monovalent magnesium ions (Makar and Kruger, 1993; Natta, 2001; Gomes et al., 2019). However, the increased reactivity of Mg+ would lead to a significantly short lifetime of this ion in solution, and recent experimental evidence, as well as computational analysis, point to direct conversion from Mg to Mg2+ as the most probable mechanism for magnesium dissolution (Cain et al., 2017; Esmaily et al., 2017; Yuwono et al., 2019). Nevertheless, the monovalent Mg theory is, to date, still a controversial and intensively discussed topic.
The nature and composition of the surface film formed on magnesium and magnesium alloys during corrosion depends on the alloy composition and on its external environment. Research work regarding this topic has shown that this surface film can be composed of an inner MgO and outer Mg(OH)2 layer (Santamaria et al., 2007; Liu et al., 2009). In the specific case of magnesium-aluminium alloys, a three-component surface layer has been suggested, with an inner aluminium-enriched layer at the alloy surface, followed by a middle MgO layer and an outer layer composed of Mg(OH)2 (Zhang et al., 2015; Esmaily et al., 2016). Furthermore, it has been shown that atmospheric exposure of magnesium alloys can lead to a mixed surface film, composed of MgO, Mg(OH)2, and MgCO3 (Feliu et al., 2009; Feliu and Llorente, 2015). Alongside the surface film, the exact composition of the corrosion products that are formed is also dependent on the elements present in the magnesium alloy (Ghali and Revie, 2011).
Aluminium, neodymium, yttrium, cerium, and zinc have been shown to improve the corrosion behaviour of magnesium alloys (Zhang et al., 2009; Ghali and Revie, 2011; Zhang et al., 2011; Gusieva et al., 2015). The beneficial effects are, however, greatly dependent on the concentration of the alloying elements. While some detrimental effects on the corrosion rate have been observed with the addition of calcium, for example, magnesium alloy corrosion is heavily accelerated when iron, nickel, copper, and/or cobalt are present (Song and Atrens, 1999). These elements are generally known as impurity elements and their concentration in the alloy is maintained as low as possible (Song and Atrens, 1999). Manganese is often added to Mg-Al alloys as scavenger for impurity elements, decreasing the corrosion rate of the alloy due to the decrease of impurity contents (Song and Atrens, 1999; Gusieva et al., 2015).
One of the most efficient strategies against magnesium and magnesium alloy corrosion has been the application of protective coatings. These coatings act as a physical barrier between the underlying metal and the external environment (Montemor, 2014). Many different types of coatings have been developed and applied to various magnesium alloys. Examples include epoxy-based coatings, sol-gel coatings, and protective films formed via plasma electrolytic oxidation. In addition, the concept of self-healing coatings has been studied in more detail in recent years. These coatings contain corrosion inhibitors that are able to impart active protection to the substrate. Furthermore, these corrosion inhibitors can be stored in appropriate carriers, and release of the inhibitor (or inhibitors) is achieved in response to specific stimuli, such as mechanical stress, the presence of certain ions, or changes in local pH (Montemor, 2014). These types of coatings have been designated as “smart” coatings throughout the literature (Montemor, 2014).
WE Series Magnesium Alloys
As previously mentioned, WE series magnesium alloys possess yttrium and rare earths as major alloying elements (see Corrosion of Magnesium and Magnesium Alloys ). Usually, minor alloying elements for this series of alloys include neodymium, zinc, and zirconium.
Development of Mg-Y alloys has been an ongoing process for several decades (Polmear, 1994). Continuous demands for high performance lightweight alloys led to the development of the WE series magnesium alloys (Lyon et al., 1991). Several different alloys are part of this category, but the most common are WE43 and WE54. Other alloys in this class include WE32, WE33, and WE94. However, to date, these alloys have rarely been discussed in literature, and no significant studies can be found regarding these alloys. This is mainly related to the fact that WE43 and WE54 possess the most adequate cost-benefit relation of the alloys included in the WE series. In recent years, the increasing interest in WE43 and WE54 as lightweight solutions for different industry sectors has brought on and increasing number of publications regarding these two alloys, in detriment of other alloys included in the WE series. For this reason, the present review focuses primarily on WE43 and WE54. However, with continued research regarding WE series Mg alloys, new and improved alloy formulations are likely to be developed and implemented in industry.
Properties
Magnesium alloys with addition of yttrium and rare earth elements were designed to possess enhanced properties compared to other commonly used alloys, such as AZ31. Room temperature mechanical properties of WE alloys are superior when compared to other magnesium alloys (Szakács et al., 2014). Table 2 shows the comparison of some room temperature mechanical properties of WE43 and WE54 with the properties of the most used all-purpose magnesium alloy today, AZ91. A summary of additional alloy properties is shown in Table 3.

TABLE 2. Comparison of mechanical properties at room temperature of as-fabricated WE43, WE54, and AZ91.
WE43 and WE54 are able to retain their properties at high temperatures (Gupta and Sharon, 2011), which is a clear advantage to the use of magnesium alloys belonging to the AZ, QE, and ZE series (Szakács et al., 2014). Long-time exposure tests at temperatures up to 250 °C showed that WE43 retains its initial tensile properties, exhibiting a superior high-temperature performance than aluminium alloys (Lyon et al., 1991). Furthermore, WE series alloys have been shown to be more creep resistant than other magnesium alloys (Jahedi et al., 2018a), while also displaying superior ductility, strength, and corrosion resistance (Ghorbanpour et al., 2019a). In addition, these alloys are biodegradable (Oshibe et al., 2019), extending even further the advantages of their use for a wide range of applications.
Contrary to alloys belonging to the AM series, for example, WE series alloys are age hardenable (Pan et al., 2016). Typically, depending on the specified conditions, higher hardness is achieved with the lowest ageing temperature, while the highest ageing temperature leads to a decrease in peak ageing time (Kang et al., 2017). Throughout literature, the hardness testing conditions, the ageing temperature and ageing time ranges, as well as the initial state of the alloy before testing vary greatly. Nevertheless, the general consensus lies in the good age hardening response of WE series magnesium alloys below 300°C, as well as the fact that lower ageing temperatures generally lead to increased peak ageing hardness values (Mengucci et al., 2008; Kandalam et al., 2015; Huang, 2017; Kang et al., 2017; Kiełbus et al., 2018). In addition, it has been reported that double-step ageing treatments can bring additional benefits for WE alloy performance (Riontino et al., 2008). The age hardening characteristics of WE series magnesium alloys are closely related to the precipitates that are formed during these treatments (see Heat Treatments).
Comparing the age hardening behaviour of WE alloys with other magnesium alloys, WE43 and WE54 usually present enhanced age hardening response. AZ31, for example, exhibits a weak strengthening effect, displaying a nearly constant hardness over ageing time (Xu et al., 2018). Furthermore, artificial ageing processes have also a more positive effect on the mechanical properties of WE series magnesium alloys than on EV31 (Kiełbus et al., 2018).
Microstructure
The microstructures of both WE43 and WE54 have been reported to be rather similar. These alloys consist of the α-Mg matrix, usually with Y and Nd present in solid solution in the matrix, and several types of precipitates distributed along grain boundaries and in the grain interiors (Rzychoń and Kiełbus, 2007; Soltan et al., 2019; Kang et al., 2020). Figure 1 shows a typical microstructure of as-cast WE43. The most commonly identified precipitate phases in WE series magnesium alloys are based on the ternary Mg-Y-Nd system (Zumdick et al., 2019). Rectangular-shaped precipitates are found in these alloys mostly along grain boundaries (Xiang et al., 2018). These precipitates have been identified as Mg24Y5 (Soltan et al., 2019). In addition, fine particles composed of Mg41Nd5 phase and Zr-rich globular precipitates are also part of the microstructure of these alloys (Barylski et al., 2017; Soltan et al., 2019).
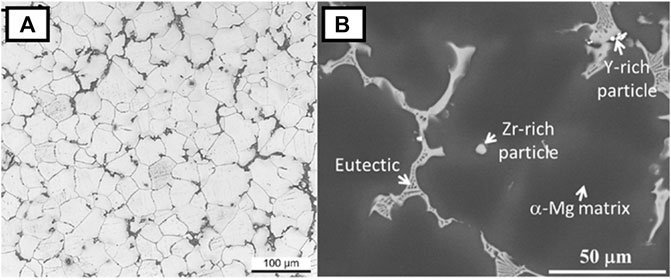
FIGURE 1. Microstructure of as-cast WE43. (A) Optical micrograph evidencing the α-Mg matrix of WE43; (B) Scanning Electron Microscopy image of WE43. Images reproduced from (Kang et al., 2020) under the terms of the Creative Commons CC-BY 4.0 License.
In order to further enhance alloy performance, WE series magnesium alloys are usually subjected to different heat treatments (see Heat Treatments). Heat treatment has an important influence on alloy microstructure and, therefore, on the alloy properties.
Heat Treatments
Tempers designate a series of different natural or artificial ageing procedures. The designation of each temper for magnesium alloys follows the system generally used for aluminium alloys. For WE series magnesium alloys, the most used tempers are the age-hardening processes (Mouritz, 2012) T5 and T6. T5 indicates that the alloy was cooled from fabrication temperature and then artificially aged, while T6 indicates solution-treatment, followed by artificial ageing (Mouritz, 2012). During T6 treatment, the alloy undergoes full recrystallization (Ghorbanpour et al., 2019a; Ghorbanpour et al., 2019b). The precipitates formed during this process tend to be localized at grain-boundaries (Ghorbanpour et al., 2019a). In contrast, the materials’ original grain structure is maintained during T5 treatment, and the formed precipitates are more evenly distributed (Ghorbanpour et al., 2019a).
Precipitation during ageing treatments of WE series magnesium alloys and the effect of these precipitates on alloy properties have been heavily studied topics in recent years (Nie and Muddle, 2000; Nie et al., 2001; Antion et al., 2003; Nie, 2012; Jiang et al., 2017). The exact structure and composition of the formed precipitates depend on the processing history of the material (Ghorbanpour et al., 2019b). The most commonly encountered precipitates in WE alloys are designated as β’’, β′, β1, and β (Ghorbanpour et al., 2019b). The β’’ phase is characterized by a Mg3Nd composition and contributes to an increase in alloy hardness during the initial stages of the ageing process (Liu et al., 2010; Ghorbanpour et al., 2019b). This β’’ phase is gradually transformed to β′ phase (Mg12NdY) at ageing temperatures between 200°C and 250°C (Jiang et al., 2017; Ghorbanpour et al., 2019b). Ageing of the alloy at 250°C leads to the appearance of the β1 phase, with a Mg3(Nd,Y) composition (Jiang et al., 2017; Ghorbanpour et al., 2019b). If the ageing process is being conducted at 250°C for a long time, precipitation of the equilibrium β phase (Mg14Nd2Y) occurs (Jiang et al., 2017; Ghorbanpour et al., 2019b). From these precipitation phases, β′ has been identified as the phase mostly responsible for the enhanced strength of WE series magnesium alloys after heat treatment, although it has been found that β’’ can also contribute to the precipitation strengthening effect (Nie and Muddle, 2000; Liu et al., 2013; Jahedi et al., 2018a). It has been demonstrated that the shape and orientation of β’ precipitates can have an important effect on dislocation motions by creating effective barriers to this movement (Nie and Muddle, 2000; Xu et al., 2018).
In general, WE series magnesium alloys exhibit superior mechanical properties after appropriate heat treatment, compared to their properties before these treatments (Yu et al., 2008). Comparing the effects of T5 and T6 treatments to WE43, it has been demonstrated that WE43-T6 usually exhibits limited ductility compared to WE43-T5 due to large grain-boundary precipitates that facilitate intergranular fracture (Jahedi et al., 2018a; Ghorbanpour et al., 2019c). Furthermore, fatigue strength of WE43-T5 tends to be improved in comparison to WE43-T6 due to finer grain size achieved after the T5 treatment (Adams et al., 2016; Wang et al., 2019b; Ghorbanpour et al., 2019c).
Processing Methodologies
To date, magnesium alloys are most commonly produced by casting methods (Luo, 2013), and the WE series is no exception. WE43 and WE54 are often processed by sand casting or permanent mould casting (Jiang et al., 2017; Westengen et al., 2006). In recent years, direct-chill casting has emerged as a favourable alternative to conventional casting methods. Direct-chill casting makes use of a higher cooling rate than other casting techniques (Jahedi et al., 2018b). A higher solidification rate of the cast material leads to high supersaturation and solid solubility of Nd and Y in the α-Mg matrix (Jiang et al., 2017). Therefore, an improvement in strength and hardness of the as-cast material can be achieved (Jiang et al., 2017). In general, however, magnesium alloys are not used in the as-cast condition, as further property improvement is usually needed for most applications (Jahedi et al., 2018b). In this sense, the cast material is subjected to mechanical and/or thermal processing (thermal treatments were discussed in Heat Treatments) (Jahedi et al., 2018b).
Mechanical deformation is employed for alloy grain refinement and microstructure homogenization (Jahedi et al., 2018b). Examples of common deformation techniques include hot-rolling (Yu et al., 2008), forging (Salandari-Rabori et al., 2018), and hydrostatic pressing (Pachla et al., 2012), all of which have been employed for grain refinement of WE43 (Lukyanova et al., 2017). Alloy deformation by rolling, for example, leads to crystallographic reorientation of the grains, which can lead to an increase in dislocation density in the alloy microstructure (Jahedi et al., 2018b). Dislocations represent preferential sites for precipitate formation (Ghorbanpour et al., 2019b). Thus, an increase in dislocation density can lead to increased density of precipitates within the microstructure of the processed alloy, with consequent improvement in alloy strength, elongation in tension, and fatigue resistance (Ghorbanpour et al., 2019b). This has been demonstrated for WE43 (Ghorbanpour et al., 2019b). If both mechanical deformation techniques and heat treatments are employed to the same alloy, the deformation techniques are used before the thermal treatments (Choudhuri et al., 2013).
Continuous improvements in alloy processing technology have led to the development of several new processing techniques to further enhance the properties of magnesium alloys in general, and WE series in particular (Minárik et al., 2018). Many of these techniques are based on severe plastic deformation (SPD) of the material (Minárik et al., 2018). From these, the most commonly studied in combination with WE series magnesium alloys are Equal Channel Angular Pressing (ECAP) and High Pressure Torsion (HPT) (Torkian et al., 2018).
During ECAP, the material (usually in rod or bar form) is forced through a bended channel (Pereira et al., 2017). At the channel bend, shear strain is generated in the material as it passes through (Shaat, 2018). The intensity of the shear deformation can be controlled by varying the internal channel angle and the outer curvature angle of the channel (Shaat, 2018). Furthermore, higher strains can be obtained by repeating the process several times (Wang et al., 2017). These multiple passes can be performed repetitively with no change to the processing conditions, or a rotation can be imposed on the material between different ECAP passes (Wang et al., 2017). Scalability of the ECAP process to industrial standards is currently being investigated (Frint et al., 2011; Lefstad et al., 2012; Frint et al., 2016; Shaat, 2018). ECAP processing leads to significant grain refinement of the alloy, generally resulting in improved strength. For example, Zhang et al. (Zhang et al., 2020) found that the ultimate tensile strength of WE43 increased from 255 MPa in the as-extruded condition, to 380 MPa after three-pass ECAP. This increase in the strength of the alloy was accompanied by a decrease in the ductility, as expected: from 20.9% in the as-extruded alloy to 8.8% after three-pass ECAP (Zhang et al., 2020). Furthermore, the authors found that multi-pass ECAP led to the generation of significant dislocations and residual stresses, resulting in significant work hardening of the WE43 alloy (Zhang et al., 2020).
For HPT processing, the material, in the form of a thin disk, is compressed under high pressure, and simultaneously subjected to torsional strain (Zhilyaev and Langdon, 2008). The material is deformed by shear due to surface frictional forces that arise during the process (Zhilyaev and Langdon, 2008). Similar to ECAP, HPT leads to an increase in the ultimate tensile strength and yield strength of the alloy (Figueiredo and Langdon, 2019). Liu et al. (Liu et al., 2017) found that extruded WE43 possessed an ultimate tensile strength of 244 MPa, with 8% elongation, while processing by HPT yielded an ultimate tensile strength of 256 MPa with 0.5% elongation. Once again, the strengthening effect conferred by SPD processing of the material led to a significant decrease in alloy ductility (Liu et al., 2017).
Both ECAP and HPT are used to achieve an ultrafine grain microstructure in the processed material (Minárik et al., 2018). It has been extensively demonstrated that using ECAP (Martynenko et al., 2018; Minárik et al., 2018; Torkian et al., 2018) or HPT (Lukyanova et al., 2016; Lukyanova et al., 2017) for WE43 processing can lead to improvements in hardness and overall mechanical properties of the alloy due to significant grain refinement.
Over the last 2 decades, additive manufacturing has been considered an attractive manufacturing process for a wide range of materials (Bourell et al., 2009). Additive manufacturing has been established as a cost-efficient method to manufacture complex shapes (Zumdick et al., 2019). In recent years, additive manufacturing technology has begun to be tested for WE series magnesium alloys. To date, the most investigated additive manufacturing technique for WE43 is selective laser melting (SLM). SLM is a powder bed fusion process: thin layers of metal powder are deposited onto the working area and focused laser radiation is responsible for melting the powder into the desired shape (Frazier, 2014; Zumdick et al., 2019). Repetitive powder deposition and melting leads to the creation of three-dimensional components (Frazier, 2014). It has been shown that it is possible to manufacture WE43 with a dense and homogeneous microstructure via SLM (Zumdick et al., 2019; Gangireddy et al., 2019). However, SLM requires secondary densification processes to achieve the desired material properties (Calvert, 2015; Gangireddy et al., 2019). For example, Zumdick et al. (2019) found no significant improvement in the ultimate tensile strength of as-extruded WE43 and the same alloy subjected to SLM. In addition, high operating temperatures during SLM can lead to loss of material performance due to increased grain growth (McClelland et al., 2019). Therefore, other additive manufacturing techniques, such as additive friction stir deposition, are currently being investigated as an alternative to SLM (Calvert, 2015).
In general, direct comparison of mechanical properties of WE series alloys obtained by different processing methodologies and/or subjected to different heat and ageing treatments is not feasible, since widely different processing parameters are used throughout literature, in addition to the varying original conditions of the studied alloys.
Alloying Elements
The rare earth content specified for each magnesium alloy from the WE series is a mixture of different rare earth elements that vary from alloy to alloy. Most commonly, the rare earth elements included in the alloys are Nd and other heavier REEs such as Gd (Pan et al., 2016).
Addition of rare earth elements to magnesium and magnesium alloys brings several improvements to the properties of these materials. Rare earths are able to improve the mechanical properties of magnesium-based materials at ambient and elevated temperatures due to the precipitation of stable RE-Mg intermetallic compounds (Maruyama et al., 2002; Pai et al., 2012). These intermetallics can act as obstacles to dislocations, therefore improving the creep resistance of the material (Witte et al., 2008; Pai et al., 2012). Furthermore, it has been shown that the presence of rare earth elements in magnesium alloys can have a positive effect on the corrosion resistance of these materials (Zhang et al., 2011). In general, rare earth elements have the ability to form intermetallic compounds with impurity elements, such as Fe for example, therefore providing a scavenger effect that mitigates the negative effect of the presence of impurity elements in magnesium alloys (Zhang et al., 2011). Furthermore, studies have shown that rare earth elements can be incorporated into the passive oxide/hydroxide surface film on magnesium alloys in aqueous media, stabilizing it and thereby increasing the protective performance of this film (Nordlien et al., 1997; Zhang et al., 2011). Despite the advantages of using rare earths as alloying elements, the addition of heavy REEs can lead to processing issues that often result in inhomogeneous composition and, therefore, performance-related problems in the final alloy (Ning et al., 2014; Pan et al., 2016). In addition, the cost of heavy rare earth elements can be very high, due to the fact that the global reserves of these elements are limited (Ning et al., 2014; Pan et al., 2016). Thus, several studies have been devoted to the modification of commercial WE series magnesium alloys, aiming for the reduction of heavy rare earth elements (Pan et al., 2016). Such modifications have mainly been focused on the substitution of heavy rare earth elements by other elements such as Y, Nd, and Zr (Pan et al., 2016).
The solid solubility of yttrium in magnesium is relatively high and yttrium enhances the strength of magnesium alloys, mainly due to solid-solution hardening (Gupta and Sharon, 2011; Ghorbanpour et al., 2019a). At 500°C, the solid solubility of Y in the α-Mg phase is ∼4 at. % (Zhao et al., 2011). This solubility decreases significantly with decreasing temperature (at 450°C, solid solubility of Y decreases to 2.7 at. %), which enables effective solution and ageing strengthening effects in yttrium-containing magnesium alloys (Zhao et al., 2011; Jiang et al., 2017). It has been reported that the use of yttrium as alloying element improves the hardness and creep resistance of magnesium alloys via precipitation of intermetallic phases that impede dislocation movements (Aghion et al., 2008; Su et al., 2013). Furthermore, the increase of Y in Mg-Y-RE alloys leads to continuous increase of the yield strength of these alloys (Su et al., 2013; Luo et al., 2019). Further increase of yttrium content can, however, lead to coarse grain boundary precipitates which can lead to a decrease of the tensile strength and elongation of the alloy due to a reduction in the cohesive strength of the grain boundaries (Su et al., 2013). The precipitation of metastable Y-containing phases also contributes to the increase in the heat resistance of magnesium (Anyanwu et al., 2001). These precipitate phases remain stable at temperatures >200°C (Anyanwu et al., 2001). However, it has been reported that yttrium can be oxidized at the melting stage during alloy processing (Luo et al., 2019). If very high amounts of yttrium are used, Y2O3 inclusions may be formed during the casting process (Luo et al., 2019). These inclusions have a detrimental effect on the mechanical properties of the alloy (Luo et al., 2019). To overcome this issue, gadolinium can be used as a partial substitute for yttrium in magnesium alloys, due to the fact that Gd has a low tendency to form oxide inclusions during alloy processing (Luo et al., 2019). In addition, it has been shown that, due to the similar atomic radius of yttrium and gadolinium, these two rare earth elements are easily interchangeable in magnesium alloy formulations (Luo et al., 2019). Furthermore, Gd has a strengthening effect similar to the one reported for yttrium (Hort et al., 2010; Jiang et al., 2017; Luo et al., 2019). In fact, several studies have shown that an increased amount of Gd (up to 10 wt.%) can lead to a more significant improvement in the mechanical properties of WE series magnesium alloys than yttrium (Hort et al., 2010; Szakács et al., 2014; Pan et al., 2016; You et al., 2017). However, the use of high weight fractions of Gd leads to an increase in alloy density, with the added disadvantage of higher cost (Li et al., 2019). Therefore, Gd content in commercial WE series magnesium alloys is usually kept low.
Similarly to yttrium and gadolinium, neodymium, as alloying element, also has a positive effect on the mechanical properties of magnesium alloys (Jin et al., 2015). This element has been used as a substitute of heavy rare earth elements (Ning et al., 2014). Neodymium displays a decreased solid solubility in Mg compared to Y (0.63 at. % at 550°C), and forms stable precipitates with magnesium (Yan et al., 2008; Le et al., 2010; Gupta and Sharon, 2011). The Mg12Nd phase is thermally stable and able to pin dislocation movement, thereby improving the high temperature creep resistance of magnesium alloys (Yan et al., 2008; Gupta and Sharon, 2011). Furthermore, the presence of Nd in aluminium-containing magnesium alloys can decrease the microgalvanic corrosion rate in these alloys via formation of Al3Nd phase (Zhang et al., 2011).
In addition to the benefits discussed above, yttrium, gadolinium, and neodymium increase the ignition temperature and flammability resistance of magnesium alloys (Tan et al., 2019). This effect has been attributed to the ability of these elements to form a rare earth-containing oxide layer on the magnesium alloy surface, preventing the access of oxygen, moisture and aggressive species to the alloy (Tan et al., 2019).
Apart from rare earth elements, zirconium and zinc are common alloying elements for WE series magnesium alloys. Zirconium is the most efficient grain refiner for magnesium alloys to date, leading to significant improvement in structural uniformity of the alloys, and to consequent improvement in alloy properties (StJohn et al., 2005; Ali et al., 2015). Zinc is usually present in WE series magnesium alloys in low quantities (<0.02 wt.%). The main effect of the use of zinc is the improvement of alloy ductility due to formation of Zn-Zr secondary phases (Jahedi et al., 2018a; Ghorbanpour et al., 2019a). In addition, it has been shown that addition of zinc to Mg-Y binary alloys leads to improved creep strength of the alloy due to a higher dislocation density in the presence of zinc (Maruyama et al., 2002; Suzuki et al., 2004). Extensive research work has been performed on the modification of commercially available WE series magnesium alloys with an increased amount of Zn. It was found that WE43-T6 modified with 0.2 wt.% of zinc leads to a tensile strength of 345 MPa, representing an improvement of 38% (Pan et al., 2016). Furthermore, it has been suggested that the use of a higher Zn-content in WE43 can lead to a decrease in the solubility of rare earth elements, resulting in a higher volume fraction of precipitation phases that are able to reduce the mobility of dislocations (Kang et al., 2014). Zinc can also improve the stability of the passive layer formed on the surface of magnesium alloys in aqueous media (Nordlien et al., 1997).
Corrosion of WE Series Magnesium Alloys
Corrosion Mechanism
The corrosion mechanism of WE series magnesium alloys has been extensively studied over the years. In general, the corrosion resistance of metal alloys is tested during immersion of the alloy under study in an appropriate electrolyte. In the case of WE series magnesium alloys, these studies have been carried out in a wide range of different electrolytes. The most commonly used media for the study of aqueous corrosion of WE magnesium alloys are NaCl, Na2SO4, and electrolytes that simulate physiological conditions, such as SBF (Simulated Body Fluid).
Similarly to the general corrosion processes observed for pure magnesium and for many other magnesium alloys (as discussed in section 1.3), WE series magnesium alloys also form protective surface layers, mainly based on Mg(OH)2 and MgO. However, the exact composition of these surface films is highly dependent on the conditions in which they are formed, i.e. on the composition of the electrolyte to which the alloys are exposed, and on the original alloy composition. Nonetheless, the surface films formed in Na2SO4, NaCl, or SBF seem to be qualitatively similar. While some authors report the formation of a uniform surface layer, mainly composed of Mg(OH)2 and with low amounts of rare earth elements (Arrabal et al., 2008), others suggest a bi-layered surface film: Mg(OH)2 outer layer, which has been reported to be porous, and an inner layer constituted mainly by MgO, Y2O3, and Y(OH)3 (Ardelean et al., 2013; Chu and Marquis, 2015). Ca and P are usually present in the surface film when the alloy is exposed to SBF (Dvorský et al., 2019). WE43 has also been tested in alkaline media containing Cl− ions, and this alloy was reported to form a passive layer composed of MgO, Mg(OH)2, and RE2O3 phases (where REEs can be Y, Nd, or Gd) (Ninlachart et al., 2017). (Chu and Marquis, 2015)
In general, it has been found that the surface films formed on WE series magnesium alloys are thinner than for pure magnesium (Zucchi et al., 2006a; Leleu et al., 2018). This has been attributed to the fact that a steady-state condition between surface film thickening and propagation of corroded area may be achieved for WE magnesium alloys, while expansion of the corroded area prevails for pure magnesium (Zucchi et al., 2006a). While thinner, the surface films formed on WE series magnesium alloys have been reported to be more stable and more protective due to the presence of rare earth elements in their composition (Kalb et al., 2012; Dvorský et al., 2019; Leleu et al., 2019; Soltan et al., 2019).
Comparing the corrosion behaviour of WE series magnesium alloys with pure magnesium or with other magnesium alloys, there seems to be no definitive consensus as to which magnesium-material has the superior corrosion resistance. WE43 has been reported to be less susceptible to the action of aggressive Cl− ions than pure magnesium (Leleu et al., 2018; Knapek et al., 2018), while also displaying much less severe localized damage in SBF than ZK60 (Jamesh et al., 2015). In comparison to the commonly used AZ91, it has been shown that WE43 possesses a slower hydrogen evolution reaction (Eq. 3) in Na2SO4 (Ardelean et al., 2013), and an overall higher corrosion resistance in NaCl (Arrabal et al., 2008). However, a higher corrosion rate of WE43 in Na2SO4 compared to AZ31 and AZ91 has also been reported (Leleu et al., 2019). The corrosion rate of WE43 has been shown to be lower than other rare earth containing magnesium alloys, such as EV31 and ZE41 (Soltan et al., 2019). WE54 was found to be slightly more resistant to in vitro degradation than pure magnesium, but the corrosion performance of WE54 was reported to be significantly poorer than for AZ91 for the same testing conditions (Walter and Kannan, 2011). Other authors reported that the corrosion rate of WE43 and WE54 during salt fog tests was only slightly higher than for AZ91 and for some aluminium casting alloys (e.g. A201) (Cho et al., 2008). These seemingly contradictory results can be related to the use of different combinations of parameters such as electrolyte type, electrolyte concentration, processing methodology of the studied alloys, and heat treatments to which the alloys were subjected prior to corrosion testing. As is the case for the previously stated mechanical properties of WE series magnesium alloys, the microstructure of these materials has a major influence in their corrosion behaviour.
Despite the different conclusions drawn by different researchers, the general consensus regarding the corrosion mechanism of WE series magnesium alloys lies in the fact that corrosion of these types of alloys involves the Zr-rich precipitates present in the microstructure, due to microgalvanic coupling effect (Coy et al., 2010; Ardelean et al., 2013). Zr-rich particles can be found both in WE43 and in WE54, and it has been demonstrated that these precipitates possess a Volta potential difference with respect to the magnesium matrix superior to +170 mV (Coy et al., 2010). This difference is high enough to cause significant galvanic coupling between the precipitates and the magnesium matrix, favouring the dissolution of the latter (Coy et al., 2010). This high Volta potential difference has been attributed to the presence of Fe in these precipitates (Coy et al., 2010). In this sense, the intermetallic particles act as local cathodes that promote the dissolution of the neighbouring magnesium matrix, with eventual propagation of the corrosion process (Leleu et al., 2019; Soltan et al., 2019). This phenomenon has been consistently reported for WE series magnesium alloys (Coy et al., 2010; Kalb et al., 2012; Ninlachart et al., 2017; Leleu et al., 2019; Soltan et al., 2019). In contrast, it has been reported that yttrium-containing intermetallic phases have little to no effect on the corrosion process of WE magnesium alloys. The Volta potential difference of these precipitates in relation to the magnesium matrix has been demonstrated to be negligible compared to the Zr-rich intermetallic phases, even though they demonstrate cathodic behaviour relative to the magnesium matrix (Coy et al., 2010; Kalb et al., 2012; Soltan et al., 2019; Kharitonov et al., 2021).
In general, micro-galvanic corrosion in WE series magnesium alloys may be reduced through grain refinement and a more continuous distribution of secondary phases (Pereira et al., 2021). A high density of REE-containing secondary phases in WE series Mg alloys has been shown to attenuate the corrosion process of these alloys since these secondary phases may act as corrosion barriers (Xie et al., 2021). However, an increased density of intermetallics also increases the density of corrosion-prone grain boundaries (Eivani et al., 2021). The overall corrosion behaviour of WE series Mg alloys is, in general terms, governed by two major factors: a) the microgalvanic coupling effect between the intermetallic phases/particles and the magnesium matrix has a detrimental effect on the corrosion performance of these materials; and b) the inclusion of REEs in the alloy matrix and the incorporation of these elements in the surface film during alloy corrosion can be beneficial (Leleu et al., 2019). Figure 2 illustrates the possible mechanism of corrosion for WE series magnesium alloys, involving the formation of a Mg(OH)2 layer on the surface of the alloy and localized microgalvanic corrosion at preferential sites. However, it should be stated that, for binary Mg-Y alloys, it has been demonstrated that an increase in the Y content, which leads to an increase in the volume fraction of Mg24Y5 phase, may be responsible for inferior corrosion resistance due to the increase in cathodic sites (Sudholz et al., 2011). Yttrium, when present in solid solution, delays the dissolution of the magnesium matrix (Sudholz et al., 2011). It is worth to note that high purity magnesium is often reported as a material possessing higher corrosion resistance compared to magnesium alloys due to the absence of intermetallic phases that can induce microgalvanic coupling (Dvorský et al., 2019).
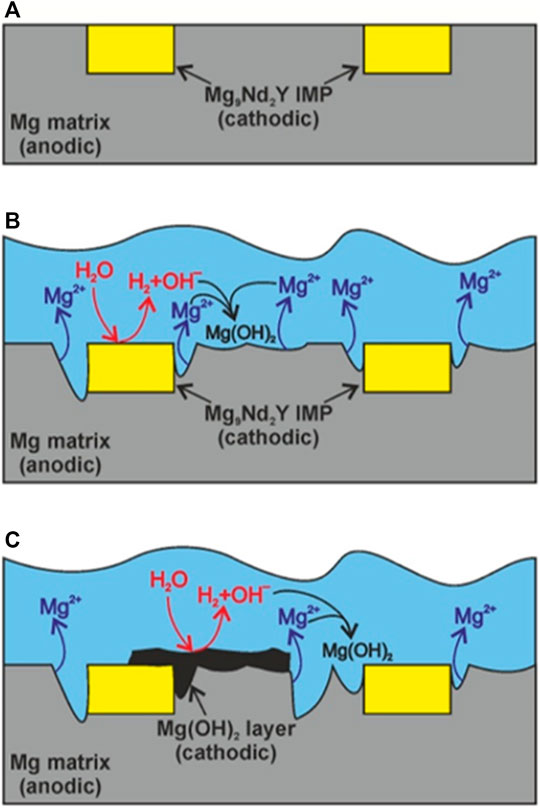
FIGURE 2. Possible mechanism of corrosion for WE series magnesium alloys (A), involving the formation of a Mg(OH)2 layer on the surface of the alloy (B), with localized microgalvanic corrosion at preferential sites (C). Figure reproduced from (Kharitonov et al., 2021) under the terms of the Creative Commons CC-BY 4.0 License.
As previously mentioned, the corrosion behaviour of WE series magnesium alloys is dependent on the specific microstructure of the alloy. Thus, the processing conditions can play a major role in the resistance of these alloys to degradation. Comparing as-cast and aged WE54 specimens, it was reported that corrosion propagation was most uniform for the as-cast condition (Rzychoń et al., 2007). This was related to the fact that an increase in ageing time leads to an increase in the volume fraction of intermetallic phases and, therefore, of microgalvanic cells (Rzychoń et al., 2007). Similar findings were reported for both WE54 and WE43 (Smola et al., 2012; Yang et al., 2020). However, if a fine dispersion of precipitates can be achieved through ageing treatments, propagation of alloy dissolution can be delayed (Chu and Marquis, 2015).
The overall corrosion behaviour of WE series magnesium alloys seems to be governed by two major factors: on the one hand, the microgalvanic coupling effect encountered between the intermetallic phases and the magnesium matrix has a detrimental effect on the corrosion performance of these materials; on the other hand, the incorporation of rare earth elements in the surface film during alloy corrosion can be beneficial (Leleu et al., 2019).
Even though WE series magnesium alloys may possess a somewhat enhanced corrosion resistance when compared to other magnesium alloys, the anticorrosive performance of these materials has to be further improved for most applications. Several different protection strategies against corrosion of WE magnesium alloys are reported in Corrosion Protection Strategies .
Corrosion Protection Strategies
The application of protective coatings has been the most efficient way to protect magnesium alloys from corrosion. These coatings are designed to be effective barriers that protect the underlying metallic substrate from the aggressive environment. For years, chromate coatings were the dominant protection method for several different metals and alloys. These coatings offer a highly inhibitive effect of the corrosion processes, while displaying improved coating strength and hardness (Eppensteiner and Jenkins, 1999; Gray and Luan, 2002). However, hexavalent chromium is linked to serious health hazards and, thus, its use has been heavily regulated (European Union, 2006; Annangi et al., 2016; Junaid et al., 2016). In this sense, extensive research has been carried out regarding the search for safer coating formulations. For magnesium alloys, the most used coating alternatives to chromate are anodized coatings (Pinto et al., 2010), Plasma Electrolytic Oxidation (PEO) coatings (Liu et al., 2016a), rare-earth conversion coatings (Montemor et al., 2007), and organic coatings (Calado et al., 2017; Calado et al., 2018). The development of protective coatings for magnesium alloys for their use in the automotive and aerospace industries, for example, is aimed at the maximum possible long-term protection of the alloy. In contrast, coatings developed for biological applications are often aimed at slowing down substrate dissolution, to allow for the use of magnesium alloys as bioresorbable temporary implants.
Given the newly-gained interest in WE series magnesium alloys for industrial applications, the vast majority of literature concerning protective strategies for WE series magnesium alloys to date is based on protective solutions which have proven efficiency for other magnesium alloy series. Coatings applied by electrolytic processes (e.g., PEO) seem to display minimal dependency on the specific composition of the alloy and/or on the type and distribution of its secondary phases. However, the microstructure and composition of each alloy is expected to play a more major role in the application of organic and hybrid organic-inorganic coatings, mainly due to different interactions between the coating and the alloy surface, influencing coating adhesion. Moreover, corrosion inhibitors may display protective mechanisms which are dependent on the interaction with specific alloying elements and/or secondary phases of the Mg alloy under study. However, presently, literature on passive corrosion protection strategies and active, self-healing coatings for WE series magnesium alloys is too scarce to evaluate these specific challenges effectively and objectively. For this reason, the protective strategies cited in this review give an overview of protective mechanisms with proven efficiency for WE43 and WE54 alloys. These examples are given below.
Anodization is an electrolytic process through which the surface of the metal is modified by formation of a stable oxide layer through the application of anodic voltage or current (Blawert et al., 2006; Gupta and Sharon, 2011). WE43 was successfully anodized in an alkaline silicate-based electrolyte (Xia et al., 2004). It was shown that the anodized coating increased the protection of the alloy during testing in 0.86 M NaCl, as no pitting of the anodized surface was detected after 140 h of immersion in the corroding medium (Xia et al., 2004). This effect was attributed to formation of MgO, and subsequent hydration of MgO to Mg(OH)2, which was found to actively block the pores of the anodized film (Xia et al., 2004). Anodized WE43 was also tested in in vivo implants (Oshibe et al., 2019). The phosphorous-based anodized coating was able to delay the degradation of the implants while inhibiting the formation of hydrogen gas (Oshibe et al., 2019). This anodized coating also displayed good biocompatibility in bone tissue during long-term follow ups (Oshibe et al., 2019). If anodization is performed at very high voltages, the process is mostly known as Plasma Electrolytic Oxidation (PEO) (Bender et al., 2013). This process has effectively been applied to WE43. For example, subjecting WE43 to PEO in a silicate/phosphate-based electrolyte led to increased polarization resistance and decreased corrosion rate in comparison to the untreated alloy in 3.5 wt.% NaCl (Tekin et al., 2013). Using a commercial electrolyte formulation, it was demonstrated that implants made of WE43 subjected to PEO displayed a delayed gas release at the implant surface, while also enabling the alloy to retain its strength during an in vivo implantation period of 12 weeks (Imwinkelried et al., 2013). Since the coatings formed via anodization or PEO are usually porous, a top-layer can be applied to seal the pores and impart additional protection. This top-layer can, for example, be a polymeric coating.
In addition to coatings applied by electrolytic processes, organic coatings have also been successfully applied for WE series magnesium alloys. For example, long-chain silane compounds have been shown to form a crystalline-like protective structure on the surface of WE43, thereby aiding in the corrosion protection of this alloy (Zucchi et al., 2006b). In recent years, significant attention has been given to the development of anticorrosive coatings for biomedical applications of WE43. For example, efficient protection of WE43 has been achieved with bi-layered coatings consisting of hydroxyapatite/poly-L-lactic acid (Diez et al., 2016), or chitosan/bioactive glass (Witecka et al., 2021). To further improve the protective ability of coatings, corrosion inhibitors can be used. These additives have the ability to impart active protection in case the applied coating is damaged during its service-life. 8-hydroxyquinoline (Argade et al., 2019) and sodium dioctylphosphate (Chirkunov and Zheludkevich, 2018) have been found to be efficient inhibitors for WE series magnesium alloys due to their ability to form stable species with magnesium cations during substrate corrosion, thereby forming protective surface films. However, to date, very few inhibitors have been identified as effective for the protection of WE series magnesium alloys, and the protection conferred by these corrosion inhibitors has mostly been studied in non-coated systems (Argade et al., 2019; Shi et al., 2020; Yang et al., 2015). Recently, Calado et al. (Calado et al., 2021) reported a newly-developed cerium organophosphate corrosion inhibitor for smart corrosion protection of WE43. The authors showed that addition of cerium tri(bis(2-ethylhexyl)phosphate) (Ce(DEHP)3) to a thin epoxy-silane coating significantly improved coating barrier properties in comparison to the unmodified coating (Calado et al., 2021). In addition, Ce(DEHP)3 displayed a cyclic protective behaviour, as illustrated in Figure 3 (Calado et al., 2021). The corrosion protection conferred by Ce(DEHP)3 was linked to its pH-dependent activation: local alkalization due to magnesium corrosion (see Eq. 3) leads to hydrolysis of Ce(DEHP)3, resulting in the formation of cerium and organophosphate ions (Calado et al., 2021). The interaction between OH− and cerium ions, and between OH− and ions released from the substrate (i.e. Y3+, Nd3+, and Mg2+) leads to the formation of Ce(OH)3, Y(OH)3, Nd(OH)3, and Mg(OH)2 (Calado et al., 2021). While Mg(OH)2 is relatively soluble, the rare earth-containing hydroxides are extremely stable species, providing efficient local corrosion inhibition at cathodic sites (Calado et al., 2021). In addition, organophosphate ions can interact with Mg2+ ions released during magnesium dissolution, thereby forming stable magnesium-organosphosphate species, which confer corrosion inhibition at anodic sites (Calado et al., 2021). Figure 4 shows the corrosion protection mechanism proposed by the authors. Overall, Ce(DEHP)3 was shown to be one of the first truly efficient corrosion inhibitors for WE series magnesium alloys, conferring long-term, pH-sensitive, and self-healing protection. This inhibitor was also shown to be effective for AZ series magnesium alloys (Calado et al., 2020), and for mild steel (Morozov et al., 2019).
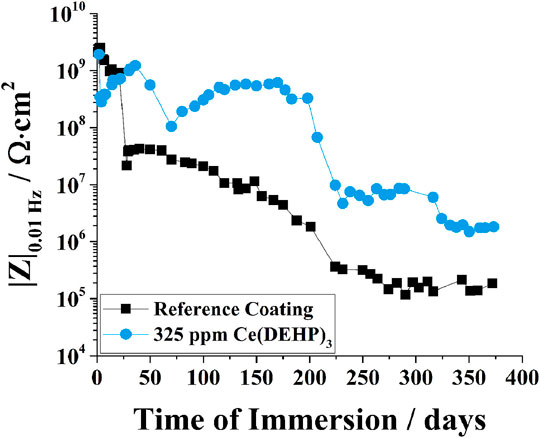
FIGURE 3. Evolution of low frequency impedance modulus (0.01 Hz) of WE43 coated with a hybrid epoxy-silane coating (reference coating), and with a hybrid epoxy-silane coating containing 325 ppm Ce(DEHP)3, during immersion in 0.5 M NaCl. Image reproduced from (Calado et al., 2021) with permission.
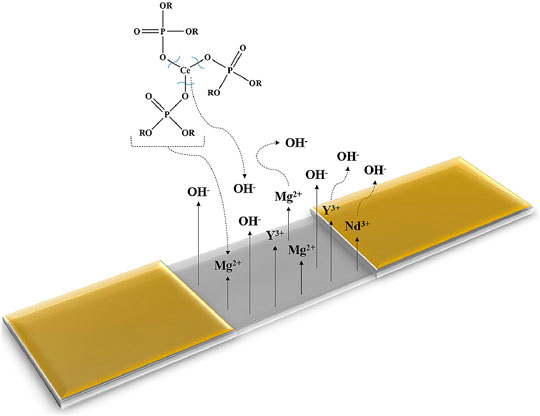
FIGURE 4. Mechanism of corrosion protection conferred by Ce(DEHP)3 to WE43 magnesium alloy. Figure reproduced from (Calado et al., 2021) with permission.
Apart from the most common coating methods that were cited above, WE series magnesium alloys have also been subjected to other alternative coating methods. Improved corrosion resistance of WE43 has been achieved, for example, by a) application of biofunctional chitosan-bioactive glass coatings via electrophoretic deposition (Heise et al., 2017; Höhlinger et al., 2017); b) surface modification using Laser Surface Melting (LSM) technique (Guo et al., 2005; Liu et al., 2016b); c) ion-implantation, e.g. hafnium (Jin et al., 2016) or Ti-O (Zhao et al., 2013); d) application of amorphous SiC film via Plasma Enhanced Chemical Vapour Deposition (Li et al., 2012); and e) application of TaN film using reactive magnetron sputtering (Jin et al., 2018).
The development of protective coatings for WE series magnesium alloys is still a growing research topic, which is in no way as explored as the development of protective solutions for other magnesium alloys, such as AZ series.
Applications
The superior properties of WE series magnesium alloys has led to an increasing usage of these types of alloys in different industries, in addition to a growing research interest in several fields. The good high temperature behaviour of WE magnesium alloys, allied to the low density of these materials, has been of particular interest for the automotive and aerospace industries.
In the automotive sector, WE alloys are widely used in high-performance cars as engine block components, e.g., pistons and cylinders (Kierzek and Adamiec, 2011; Malayoğlu and Tekin, 2015). Furthermore, given their superior creep resistance and high temperature stability, in contrast to other magnesium alloys, WE series alloys are expected to be suitable for powertrain applications (Blawert et al., 2004).
WE43 and WE54 are both used in helicopters (Gupta and Sharon, 2011). For example, WE43 is used in the gearboxes of the Eurocopter EC120, Eurocopter NH90, and Sikorsky S92 (Mouritz, 2012). Due to their stability at high temperatures, WE series magnesium alloys are used in jet engines, particularly in auxiliary power units, accessory drives, constant speed drives, and engine gearboxes (Malayoğlu and Tekin, 2015; Luxfer MEL Technologies and Engines, 2019). The Lockheed Martin F-16 and F-35 are two examples of fighter aircraft that make use of WE alloys as engine components (Luxfer MEL Technologies and Engines, 2019).
In the past, the use of magnesium alloys for applications in commercial aircraft interiors has been restricted due to concerns about the flammability of these materials in general. However, extensive tests conducted by the FAA (U. S. Federal Aviation Administration) (Marker, 2013) have shown that WE43 meets the necessary performance and safety requirements imposed for materials to be used in aircraft interiors (Luxfer MEL Technologies and Engines, 2019). In fact, the favourable outcome of the FAA flammability tests conducted on WE43 was a major driving force in the decision to lift the long-standing ban of the use of magnesium alloys in aircraft interiors in 2015 (Society of Automotive Engineers, 2015; Luxfer MEL Technologies and Aircraft Interiors, 2019). Thus, WE43 became particularly interesting for application in cabin seat frames.
The favourable combination of properties inherent to the WE series magnesium alloys is driving further research related to these alloys, regarding their properties and potential future applications. The high damping capacity and overall good ballistic performance of WE43 and WE54 have led to several studies regarding these alloys as armour materials, such as for lightweight armoured ground vehicle applications (Cho et al., 2008) and for protective helmet shells (Mathaudhu et al., 2014).
The biodegradability of magnesium alloys in general has led to increased interest in these materials for biomedical applications (Chen et al., 2018). Furthermore, the elastic modulus of magnesium alloys (41–45 GPa) is similar to the elastic modulus of natural bone (3–20 GPa) (Chen et al., 2014). Therefore, magnesium alloys can prevent clinical issues related to biomedical incompatibility (Chen et al., 2014). In fact, the elastic modulus of common stent-material 316L stainless steel (193 GPa) is much higher than that of WE43 (44 GPa), while displaying similar yield strength (Chen et al., 2014). Given this positive biocompatibility, and the adequate corrosion resistance of WE series magnesium alloys, intensive research on the potential use of these materials for the healthcare industry has been carried out in recent years. For instance, clinical trials have been performed with WE43-based bioresorbable screws and cardiovascular stents, positively demonstrating the applicability of this alloy in the medical field (Erbel et al., 2007; Plaass et al., 2016; Chen et al., 2018). However, despite of the promising results, these alloys have to be further studied to evaluate the potentially harmful effect of certain alloying elements on human health (Chen et al., 2019).
Future Trends
In general terms, magnesium and magnesium alloys are attractive lightweight materials for many different industries, such as the automotive and aerospace sectors. These materials are also envisaged as appropriate for biodegradable implants. In particular, the good high temperature properties and general mechanical behaviour of WE alloys make this class of magnesium-based materials especially interesting for a wide range of applications. However, as all materials, these alloys have some drawbacks associated to their use for certain applications, such as a high cost of rare earth elements and general susceptibility to corrosion. In the near future, research regarding WE series magnesium alloys will continue to focus on further enhancement of the properties of these materials and mitigation (or even elimination) of some shortcomings of these alloys. Fine-tuning alloy composition and microstructure through alloying and/or through the use of new processing methodologies will play an important role for further property improvement. To take full advantage of the favourable properties of WE series magnesium alloys, the corrosion susceptibility of these materials needs to be addressed. Advances in the development of efficient corrosion protection strategies tailored for these types of alloys are currently being made. Undoubtedly, development and application of multifunctional and self-healing coatings that are able to provide long-term protection, and that are able to prevent corrosion propagation if the coating is damaged during its lifetime, will play a major role in further improving the performance of WE magnesium alloys for an even wider range of applications. Thus, in addition to a growing interest in these materials for several industries, mainly the automotive and aerospace sectors, emergence of an increasing number of new applications is expected to lead to a continuous increase in the global demand for WE series magnesium alloys over the next years.
Concluding Remarks
WE series alloys possess superior room temperature mechanical properties when compared to other magnesium alloys. In addition, these alloys have the ability to retain their properties at high temperatures, which constitutes a clear advantage in comparison to other classes of magnesium alloys, such as AZ and ZE.
Several different thermal and solution treatments are available for WE series magnesium alloys. Properties such as strength and ductility can be precisely controlled by the use of different combinations and sequences of ageing temperature and duration. For this purpose, continued research efforts have been carried out to obtain a fundamental understanding of the precipitation sequence that occurs during ageing treatments, and of the possible changes in alloy microstructure. In addition, the role of specific alloying elements and their content in common WE series magnesium alloys has been heavily studied over the years. Direct modification of the content of specific alloying elements, or minor addition of other elements, can also be a way of controlling alloy properties.
New processing methodologies have been emerging as a means to improve the properties of magnesium alloys in general. For WE series magnesium alloys, most experimental processing techniques are based on severe plastic deformation of the alloy. These techniques have been used to obtain ultrafine grain microstructure in the processed material. Significant grain refinement has been obtained for WE alloys using ECAP and HPT, which in turn leads to improvement in hardness and overall mechanical properties of these alloys. The scalability of these processes to industrial scale is currently under study.
Despite their various advantages, WE series magnesium alloys are susceptible to corrosion. For most applications, efficient protection strategies are needed to mitigate the degradation of these materials. Anodization and PEO have been the most used techniques for the protection of WE alloys, but a wide range of coating types has been successfully tested for this class of magnesium alloys. The development of WE-specific anticorrosive coatings is a topic that has recently been receiving growing research interest, with very fast developments.
While WE43 and WE54 are already being used in the automotive and aerospace industries, there has been an increasing interest in these alloys as lightweight biodegradable materials. Continued improvement, property tailoring for specific applications, and development of protective anticorrosive strategies for WE series magnesium alloys, will allow to take full advantage of these materials, and enable their widespread use for a wide range of applications.
Author Contributions
LC wrote the manuscript. All authors contributed to the choice of the review topic, to the general outline of the manuscript, and to revision and approval of the manuscript to be submitted.
Funding
Funding was provided by Fundação para a Ciência e a Tecnologia (FCT) via PhD grant SFRH/BD/127341/2016, and via funding of CQE (project UIDB/00100/2020)
Conflict of Interest
The authors declare that the research was conducted in the absence of any commercial or financial relationships that could be construed as a potential conflict of interest.
Publisher’s Note
All claims expressed in this article are solely those of the authors and do not necessarily represent those of their affiliated organizations, or those of the publisher, the editors and the reviewers. Any product that may be evaluated in this article, or claim that may be made by its manufacturer, is not guaranteed or endorsed by the publisher.
Acknowledgments
The authors would like to acknowledge Fundação para a Ciência e a Tecnologia (FCT) for PhD grant SFRH/BD/127341/2016, CQE (funded by FCT) for project UIDB/00100/2020, and Professor Rogério Colaço for the suggestions made throughout the planning and writing of this manuscript.
References
Adams, J. F., Allison, J. E., and Jones, J. W. (2016). The Effects of Heat Treatment on Very High Cycle Fatigue Behavior in Hot-Rolled WE43 Magnesium. Int. J. Fatigue 93, 372–386. doi:10.1016/j.ijfatigue.2016.05.033
Aghion, E., Gueta, Y., Moscovitch, N., and Bronfin, B. (2008). Effect of Yttrium Additions on the Properties of Grain-Refined Mg-3% Nd alloy. J. Mater. Sci. 43, 4870–4875. doi:10.1007/s10853-008-2708-9
Ali, Y., Qiu, D., Jiang, B., Pan, F., and Zhang, M.-X. (2015). Current Research Progress in Grain Refinement of Cast Magnesium Alloys: A Review Article. J. Alloys Compd. 619, 639–651. doi:10.1016/j.jallcom.2014.09.061
Annangi, B., Bonassi, S., Marcos, R., and Hernández, A. (2016). Biomonitoring of Humans Exposed to Arsenic, Chromium, Nickel, Vanadium, and Complex Mixtures of Metals by Using the Micronucleus Test in Lymphocytes. Mutat. Research/Reviews Mutat. Res. 770, 140–161. doi:10.1016/j.mrrev.2016.03.003
Antion, C., Donnadieu, P., Perrard, F., Deschamps, A., Tassin, C., and Pisch, A. (2003). Hardening Precipitation in a Mg-4Y-3RE alloy. Acta Materialia 51, 5335–5348. doi:10.1016/S1359-6454(03)00391-4
Anyanwu, I. A., Kamado, S., and Kojima, Y. (2001). Aging Characteristics and High Temperature Tensile Properties of Mg-Gd-Y-Zr Alloys. Mater. Trans. 42, 1206–1211. doi:10.2320/matertrans.42.1206
Ardelean, H., Seyeux, A., Zanna, S., Prima, F., Frateur, I., and Marcus, P. (2013). Corrosion Processes of Mg-Y-Nd-Zr Alloys in Na2SO4 Electrolyte. Corrosion Sci. 73, 196–207. doi:10.1016/j.corsci.2013.03.036
Argade, G. R., Sanders, S., Mohandass, G., Alsaleh, A., D’Souza, F., Golden, T. D., et al. (2019). Corrosion Inhibition Study of Mg-Nd-Y High Strength Magnesium Alloy Using Organic Inhibitor. J. Materi Eng. Perform. 28, 852–862. doi:10.1007/s11665-018-3849-x
Arrabal, R., Matykina, E., Viejo, F., Skeldon, P., and Thompson, G. E. (2008). Corrosion Resistance of WE43 and AZ91D Magnesium Alloys with Phosphate PEO Coatings. Corrosion Sci. 50, 1744–1752. doi:10.1016/j.corsci.2008.03.002
Arrabal, R., Pardo, A., Merino, M. C., Mohedano, M., Casajús, P., Paucar, K., et al. (2012). Effect of Nd on the Corrosion Behaviour of AM50 and AZ91D Magnesium Alloys in 3.5 wt.% NaCl Solution. Corrosion Sci. 55, 301–312. doi:10.1016/j.corsci.2011.10.033
Barylski, A., Kupka, M., Aniołek, K., and Rak, J. (2017). The Effect of Precipitation Hardening on the Structure and Mechanical and Tribological Properties of Magnesium alloy WE54. Vacuum 139, 77–86. doi:10.1016/j.vacuum.2017.02.015
Bender, S., Göllner, J., Heyn, A., Blawert, C., and Srinivasan, P. B. (2013). “Corrosion and Surface Finishing of Magnesium and its Alloys,” in Fundamentals of Magnesium Alloy Metallurgy. Editors M. O. Pekguleryuz, K. U. Kainer, and A. A. Kaya (Cambridge, UK: Woodhead Publishing Limited). doi:10.1533/9780857097293.232
Blawert, C., Dietzel, W., Ghali, E., and Song, G. (2006). Anodizing Treatments for Magnesium Alloys and Their Effect on Corrosion Resistance in Various Environments. Adv. Eng. Mater. 8, 511–533. doi:10.1002/adem.200500257
Blawert, C., Hort, N., and Kainer, K. U. (2004). Automotive Applications of Magnesium and its Alloys. Trans. Indian Inst. Met. 57, 397–408.
Bourell D. L., Leu M. C., and Rosen D. W. (Editors) (2009). “Roadmap for Additive Manufacturing - Identifying the Future of Freeform Processing,” Roadmap for Additive Manufacturing (RAM) Workshop (Alexandria, VA, USA: The University of Texas).
Cain, T. W., Gonzalez-Afanador, I., Birbilis, N., and Scully, J. R. (2017). The Role of Surface Films and Dissolution Products on the Negative Difference Effect for Magnesium: Comparison of Cl−versus Cl−Free Solutions. J. Electrochem. Soc. 164, C300–C311. doi:10.1149/2.1371706jes
Calado, L. M., and Montemor, M. F. (2017). “Handbook of Sol-Gel Science and Technology,” in Handbook of Sol-Gel Science and Technology. Editors L. Klein, M. Aparicio, and A. Jitianu (Berlin, Germany: Springer International Publishing). doi:10.1007/978-3-319-19454-7
Calado, L. M., Taryba, M. G., Carmezim, M. J., and Montemor, M. F. (2018). Self-healing Ceria-Modified Coating for Corrosion protection of AZ31 Magnesium alloy. Corrosion Sci. 142, 12–21. doi:10.1016/j.corsci.2018.06.013
Calado, L. M., Taryba, M. G., Morozov, Y., Carmezim, M. J., and Montemor, M. F. (2021). Cerium Phosphate-Based Inhibitor for Smart Corrosion protection of WE43 Magnesium alloy. Electrochimica Acta 365, 137368. doi:10.1016/j.electacta.2020.137368
Calado, L. M., Taryba, M. G., Morozov, Y., Carmezim, M. J., and Montemor, M. F. (2020). Novel Smart and Self-Healing Cerium Phosphate-Based Corrosion Inhibitor for AZ31 Magnesium alloy. Corrosion Sci. 170, 108648. doi:10.1016/j.corsci.2020.108648
Callister, W. D., and Rethwisch, D. G. (2014). Materials Science and Engineering - an Introduction. 9th ed. Hoboken, NJ, USA: John Wiley & Sons.
Calvert, J. R. (2015). Microstructure and Mechanical Properties of WE43 Alloy Produced via Additive Friction Stir Technology. Blacksburg, VA, USA: Virginia Polytechnic Institute and State University.
Chen, J., Tan, L., Yu, X., Etim, I. P., Ibrahim, M., and Yang, K. (2018). Mechanical Properties of Magnesium Alloys for Medical Application: A Review. J. Mech. Behav. Biomed. Mater. 87, 68–79. doi:10.1016/j.jmbbm.2018.07.022
Chen, Y., Dou, J., Yu, H., and Chen, C. (2019). Degradable Magnesium-Based Alloys for Biomedical Applications: The Role of Critical Alloying Elements. J. Biomater. Appl. 33, 1348–1372. doi:10.1177/0885328219834656
Chen, Y., Xu, Z., Smith, C., and Sankar, J. (2014). Recent Advances on the Development of Magnesium Alloys for Biodegradable Implants. Acta Biomater. 10, 4561–4573. doi:10.1016/j.actbio.2014.07.005
Chirkunov, A. A., and Zheludkevich, M. L. (2018). Corrosion Inhibition of Elektron WE43 Magnesium alloy in NaCl Solution. Int. J. Corros. Scale Inhib. 7, 376–389. doi:10.17675/2305-6894-2018-7-3-8
Cho, K., Sano, T., Doherty, K., Yen, C., Gazonas, G., Montgomery, J., et al. (2008). “Magnesium Technology and Manufacturing for Ultra Lightweight Armored Ground Vehicles,” in Army Science Conference, Orlando, FL, USA.
Choudhuri, D., Meher, S., Nag, S., Dendge, N., Hwang, J. Y., and Banerjee, R. (2013). Evolution of a Honeycomb Network of Precipitates in a Hot-Rolled Commercial Mg-Y-Nd-Zr alloy. Philos. Mag. Lett. 93, 395–404. doi:10.1080/09500839.2013.791751
Chu, P.-W., and Marquis, E. A. (2015). Linking the Microstructure of a Heat-Treated WE43 Mg alloy with its Corrosion Behavior. Corrosion Sci. 101, 94–104. doi:10.1016/j.corsci.2015.09.005
Čížek, L., Greger, M., Pawlica, L., Dobrzański, L. A., and Tański, T. (2004). Study of Selected Properties of Magnesium alloy AZ91 after Heat Treatment and Forming. J. Mater. Process. Technol. 157-158, 466–471. doi:10.1016/j.jmatprotec.2004.07.149
Córdoba, L. C., Montemor, M. F., and Coradin, T. (2016). Silane/TiO2 Coating to Control the Corrosion Rate of Magnesium Alloys in Simulated Body Fluid. Corrosion Sci. 104, 152–161. doi:10.1016/j.corsci.2015.12.006
Coy, A. E., Viejo, F., Skeldon, P., and Thompson, G. E. (2010). Susceptibility of Rare-Earth-Magnesium Alloys to Micro-galvanic Corrosion. Corrosion Sci. 52, 3896–3906. doi:10.1016/j.corsci.2010.08.006
Czerwinski, F. (2014). Controlling the Ignition and Flammability of Magnesium for Aerospace Applications. Corrosion Sci. 86, 1–16. doi:10.1016/j.corsci.2014.04.047
Diez, M., Kang, M.-H., Kim, S.-M., Kim, H.-E., and Song, J. (2016). Hydroxyapatite (HA)/poly-l-lactic Acid (PLLA) Dual Coating on Magnesium alloy under Deformation for Biomedical Applications. J. Mater. Sci. Mater. Med. 27, 34. doi:10.1007/s10856-015-5643-8
Dvorský, D., Kubásek, J., Voňavková, I., and Vojtěch, D. (2019). Structure, Mechanical and Corrosion Properties of Extruded Mg-Nd-Zn, Mg-Y-Zn and Mg-Y-Nd Alloys. Mater. Sci. Technol. 35, 520–529. doi:10.1080/02670836.2019.1570680
Eivani, A. R., Mehdizade, M., Chabok, S., and Zhou, J. (2021). Applying Multi-Pass Friction Stir Processing to Refine the Microstructure and Enhance the Strength, Ductility and Corrosion Resistance of WE43 Magnesium alloy. J. Mater. Res. Technol. 12, 1946–1957. doi:10.1016/j.jmrt.2021.03.021
Eppensteiner, F. W., and Jenkins, M. R. (1999). Chromate Conversion Coatings. Metal Finishing 97, 497–509. doi:10.1016/S0026-0576(00)83109-8
Erbel, R., Di Mario, C., Bartunek, J., Bonnier, J., De Bruyne, B., Eberli, F. R., et al. (2007). Temporary Scaffolding of Coronary Arteries with Bioabsorbable Magnesium Stents: a Prospective, Non-randomised Multicentre Trial. The Lancet 369, 1869–1875. doi:10.1016/S0140-6736(07)60853-8
Esmaily, M., Blücher, D. B., Svensson, J. E., Halvarsson, M., and Johansson, L. G. (2016). New Insights into the Corrosion of Magnesium Alloys - the Role of Aluminum. Scripta Materialia 115, 91–95. doi:10.1016/j.scriptamat.2016.01.008
Esmaily, M., Svensson, J. E., Fajardo, S., Birbilis, N., Frankel, G. S., Virtanen, S., et al. (2017). Fundamentals and Advances in Magnesium alloy Corrosion. Prog. Mater. Sci. 89, 92–193. doi:10.1016/j.pmatsci.2017.04.011
European Union (2009). Directive 2008/101/EC of the European Parliament and of the Council of 19 November 2008 Amending Directive 2003/87/EC So as to Include Aviation Activities in the Scheme for Greenhouse Gas Emission Allowance Trading within the Community. Luxembourg: Official Journal of the European Union, 3–21.
European Union (2006). Regulation (EC) No 1907/2006 of the European Parliament and of the Council of 18 December 2006 Concerning the Registration, Evaluation, Authorisation and Restriction of Chemicals (REACH), Establishing a European Chemicals Agency. Official J. Eur. Union 49, 1–849.
European Union (2014). Regulation (EU) No 333/2014 of the European Parliament and of the Council of 11 March 2014 Amending Regulation (EC) No 443/2009 to Define the Modalities for Reaching the 2020 Target to Reduce CO2 Emissions from New Passenger Cars. Luxembourg: Official Journal of the European Union, 15–21.
Feliu, S., and Llorente, I. (2015). Corrosion Product Layers on Magnesium Alloys AZ31 and AZ61: Surface Chemistry and Protective Ability. Appl. Surf. Sci. 347, 736–746. doi:10.1016/j.apsusc.2015.04.189
Feliu, S., Pardo, a., Merino, M. C., Coy, a. E., Viejo, F., and Arrabal, R. (2009). Correlation between the Surface Chemistry and the Atmospheric Corrosion of AZ31, AZ80 and AZ91D Magnesium Alloys. Appl. Surf. Sci. 255, 4102–4108. doi:10.1016/j.apsusc.2008.10.095
Figueiredo, R. B., and Langdon, T. G. (2019). Processing Magnesium and its Alloys by High-Pressure Torsion: An Overview. Adv. Eng. Mater. 21, 1801039. doi:10.1002/adem.201801039
Frazier, W. E. (2014). Metal Additive Manufacturing: A Review. J. Materi Eng. Perform. 23, 1917–1928. doi:10.1007/s11665-014-0958-z
Frint, P., Hockauf, M., Dietrich, D., Halle, T., Wagner, M. F.-X., and Lampke, T. (2011). Influence of Strain Gradients on the Grain Refinement during Industrial Scale ECAP. Mat.-wiss. U. Werkstofftech. 42, 680–685. doi:10.1002/mawe.201100839
Frint, S., Hockauf, M., Frint, P., and Wagner, M. F.-X. (2016). Scaling up Segal's Principle of Equal-Channel Angular Pressing. Mater. Des. 97, 502–511. doi:10.1016/j.matdes.2016.02.067
Gangireddy, S., Gwalani, B., Liu, K., Faierson, E. J., and Mishra, R. S. (2019). Microstructure and Mechanical Behavior of an Additive Manufactured (AM) WE43-Mg alloy. Additive Manufacturing 26, 53–64. doi:10.1016/j.addma.2018.12.015
Ganguly, S., and Mondal, A. K. (2018). Influence of SiC Nanoparticles Addition on Microstructure and Creep Behavior of Squeeze-Cast AZ91-Ca-Sb Magnesium alloy. Mater. Sci. Eng. A 718, 377–389. doi:10.1016/j.msea.2018.01.131
Ghali, E. (2011). “Magnesium and Magnesium Alloys,” in Uhlig’s Corrosion Handbook. Editor R. W. Revie 3rd ed. (Hoboken, NJ, USA: John Wiley & Sons). doi:10.1002/9780470872864.ch58
Ghorbanpour, S., McWilliams, B. A., and Knezevic, M. (2019). Effect of Hot Working and Aging Heat Treatments on Monotonic, Cyclic, and Fatigue Behavior of WE43 Magnesium alloy. Mater. Sci. Eng. A 747, 27–41. doi:10.1016/j.msea.2019.01.056
Ghorbanpour, S., Mcwilliams, B. A., and Knezevic, M. (2019). “Effect of Hot Working on the High Cycle Fatigue Behavior of WE43 Rare Earth Magnesium Alloy,” in Magnesium Technology. Editors V. Joshi, J. Jordon, D. Orlov, and N. Neelameggham (Berlin, Germany: Springer Cham), 219–225. doi:10.1007/978-3-030-05789-3_33
Ghorbanpour, S., McWilliams, B. A., and Knezevic, M. (2019). Low‐cycle Fatigue Behavior of Rolled WE43‐T5 Magnesium alloy. Fatigue Fract Eng. Mater. Struct. 42, 1357–1372. doi:10.1111/ffe.12992
Gomes, M. P., Costa, I., Pébère, N., Rossi, J. L., Tribollet, B., and Vivier, V. (2019). On the Corrosion Mechanism of Mg Investigated by Electrochemical Impedance Spectroscopy. Electrochimica Acta 306, 61–70. doi:10.1016/j.electacta.2019.03.080
Gray, J. E., and Luan, B. (2002). Protective Coatings on Magnesium and its Alloys - a Critical Review. J. Alloys Compd. 336, 88–113. doi:10.1016/S0925-8388(01)01899-0
Grote, M., Williams, I., and Preston, J. (2014). Direct Carbon Dioxide Emissions from Civil Aircraft. Atmos. Environ. 95, 214–224. doi:10.1016/j.atmosenv.2014.06.042
Guo, L. F., Yue, T. M., and Man, H. C. (2005). Excimer Laser Surface Treatment of Magnesium alloy WE43 for Corrosion Resistance Improvement. J. Mater. Sci. 40, 3531–3533. doi:10.1007/s10853-005-2888-5
Gupta, M., and Sharon, N. M. L. (2011). Magnesium, Magnesium Alloys, and Magnesium Composites. Hoboken, NJ, USA: John Wiley & Sons.
Gusieva, K., Davies, C. H. J., Scully, J. R., and Birbilis, N. (2015). Corrosion of Magnesium Alloys: the Role of Alloying. Int. Mater. Rev. 60, 169–194. doi:10.1179/1743280414Y.0000000046
Heise, S., Höhlinger, M., Hernández, Y. T., Palacio, J. J. P., Rodriquez Ortiz, J. A., Wagener, V., et al. (2017). Electrophoretic Deposition and Characterization of Chitosan/bioactive Glass Composite Coatings on Mg alloy Substrates. Electrochimica Acta 232, 456–464. doi:10.1016/j.electacta.2017.02.081
Höhlinger, M., Heise, S., Wagener, V., Boccaccini, A. R., and Virtanen, S. (2017). Developing Surface Pre-treatments for Electrophoretic Deposition of Biofunctional Chitosan-Bioactive Glass Coatings on a WE43 Magnesium alloy. Appl. Surf. Sci. 405, 441–448. doi:10.1016/j.apsusc.2017.02.049
Hort, N., Huang, Y., Fechner, D., Störmer, M., Blawert, C., Witte, F., et al. (2010). Magnesium Alloys as Implant Materials - Principles of Property Design for Mg-RE Alloys☆. Acta Biomater. 6, 1714–1725. doi:10.1016/j.actbio.2009.09.010
Hu, Y., Zhang, C., Zheng, T., Pan, F., and Tang, A. (2018). Strengthening Effects of Zn Addition on an Ultrahigh Ductility Mg-Gd-Zr Magnesium Alloy. Materials 11, 1942. doi:10.3390/ma11101942
Huang, C. (2017). Optimisation of Heat Treatment Process for New-Developed Low Cost Creep-Resist Mg Alloy (Mg-3Nd-2Ca). Brisbane, Australia: University of Queensland.
Imwinkelried, T., Beck, S., Iizuka, T., and Schaller, B. (2013). Effect of a Plasmaelectrolytic Coating on the Strength Retention of In Vivo and In Vitro Degraded Magnesium Implants. Acta Biomater. 9, 8643–8649. doi:10.1016/j.actbio.2012.08.047
Incesu, A., and Gungor, A. (2020). Mechanical Properties and Biodegradability of Mg-Zn-Ca Alloys: Homogenization Heat Treatment and Hot Rolling. J. Mater. Sci. Mater. Med. 31, 123. doi:10.1007/s10856-020-06468-5
Jahedi, M., McWilliams, B. A., Kellogg, F. R., Beyerlein, I. J., and Knezevic, M. (2018). Rate and Temperature Dependent Deformation Behavior of As-Cast WE43 Magnesium-Rare Earth alloy Manufactured by Direct-Chill Casting. Mater. Sci. Eng. A 712, 50–64. doi:10.1016/j.msea.2017.11.092
Jahedi, M., McWilliams, B. A., and Knezevic, M. (2018). Deformation and Fracture Mechanisms in WE43 Magnesium-Rare Earth alloy Fabricated by Direct-Chill Casting and Rolling. Mater. Sci. Eng. A 726, 194–207. doi:10.1016/j.msea.2018.04.090
James, M., Kihiu, J. M., Rading, G. O., and Kimotho, J. K. (2011). Use of Magnesium Alloys in Optimizing the Weight of Automobile: Current Trends and Opportunities. Sustain. Res. Innovation Conf. Proc. 3, 4–6.
Jamesh, M. I., Wu, G., Zhao, Y., Mckenzie, D. R., Bilek, M. M. M., and Chu, P. K. (2015). Electrochemical Corrosion Behavior of Biodegradable Mg-Y-RE and Mg-Zn-Zr Alloys in Ringer's Solution and Simulated Body Fluid. Corrosion Sci. 91, 160–184. doi:10.1016/j.corsci.2014.11.015
Jiang, H. S., Zheng, M. Y., Qiao, X. G., Wu, K., Peng, Q. Y., Yang, S. H., et al. (2017). Microstructure and Mechanical Properties of WE43 Magnesium alloy Fabricated by Direct-Chill Casting. Mater. Sci. Eng. A 684, 158–164. doi:10.1016/j.msea.2016.11.009
Jin, W., Wang, G., Peng, X., Li, W., Qasim, A. M., and Chu, P. K. (2018). Tantalum Nitride Films for Corrosion protection of Biomedical Mg-Y-RE alloy. J. Alloys Compd. 764, 947–958. doi:10.1016/j.jallcom.2018.06.151
Jin, W., Wu, G., Feng, H., Wang, W., Zhang, X., and Chu, P. K. (2015). Improvement of Corrosion Resistance and Biocompatibility of Rare-Earth WE43 Magnesium alloy by Neodymium Self-Ion Implantation. Corrosion Sci. 94, 142–155. doi:10.1016/j.corsci.2015.01.049
Jin, W., Wu, G., Gao, A., Feng, H., Peng, X., and Chu, P. K. (2016). Hafnium-implanted WE43 Magnesium alloy for Enhanced Corrosion protection and Biocompatibility. Surf. Coat. Technol. 306, 11–15. doi:10.1016/j.surfcoat.2016.02.055
Juan, S., Feng, G., Xiaobo, G., and Haiquan, F. (2021). Study on Solid-Solution Interaction and Existing Forms of Alloying Elements in Mg-Al-Zn-Gd alloy. J. Alloys Compd. 854, 156209. doi:10.1016/j.jallcom.2020.156209
Junaid, M., Hashmi, M. Z., Malik, R. N., and Pei, D.-S. (2016). Toxicity and Oxidative Stress Induced by Chromium in Workers Exposed from Different Occupational Settings Around the globe: A Review. Environ. Sci. Pollut. Res. 23, 20151–20167. doi:10.1007/s11356-016-7463-x
Kabirian, F., and Mahmudi, R. (2009). Impression Creep Behavior of a Cast AZ91 Magnesium Alloy. Metall. Mat Trans. A. 40, 116–127. doi:10.1007/s11661-008-9699-7
Kalb, H., Rzany, A., and Hensel, B. (2012). Impact of Microgalvanic Corrosion on the Degradation Morphology of WE43 and Pure Magnesium under Exposure to Simulated Body Fluid. Corrosion Sci. 57, 122–130. doi:10.1016/j.corsci.2011.12.026
Kandalam, S., Agrawal, P., Avadhani, G. S., Kumar, S., and Suwas, S. (2015). Precipitation Response of the Magnesium alloy WE43 in Strained and Unstrained Conditions. J. Alloys Compd. 623, 317–323. doi:10.1016/j.jallcom.2014.09.179
Kang, Y., Huang, Z., Zhao, H., Gan, C., Zhou, N., Zheng, K., et al. (2020). Comparative Study of Hot Deformation Behavior and Microstructure Evolution of As-Cast and Extruded WE43 Magnesium Alloy. Metals 10, 429. doi:10.3390/met10040429
Kang, Y. H., Wang, X. X., Zhang, N., Yan, H., and Chen, R. S. (2017). Effect of Initial Temper on the Creep Behavior of Precipitation-Hardened WE43 alloy. Mater. Sci. Eng. A 689, 419–426. doi:10.1016/j.msea.2017.02.081
Kang, Y. H., Wu, D., Chen, R. S., and Han, E. H. (2014). Microstructures and Mechanical Properties of the Age Hardened Mg-4.2Y-2.5Nd-1Gd-0.6Zr (WE43) Microalloyed with Zn. J. Magnesium Alloys 2, 109–115. doi:10.1016/j.jma.2014.01.010
Kharitonov, D. S., Zimowska, M., Ryl, J., Zieliński, A., Osipenko, M. A., Adamiec, J., et al. (2021). Aqueous Molybdate Provides Effective Corrosion Inhibition of WE43 Magnesium alloy in Sodium Chloride Solutions. Corrosion Sci. 190, 109664. doi:10.1016/j.corsci.2021.109664
Kiani, M., Gandikota, I., Rais-Rohani, M., and Motoyama, K. (2014). Design of Lightweight Magnesium Car Body Structure under Crash and Vibration Constraints. J. Magnesium Alloys 2, 99–108. doi:10.1016/j.jma.2014.05.005
Kiełbus, A. (2018). “Microstructure and Properties of Casting Magnesium Alloys Designed to Work in Elevated Temperature,” in Magnesium Alloys. Editors T. Tański, W. Borek, and M. Król (London, UK: IntechOpen).
Kierzek, A., and Adamiec, J. (2011). Design Factors Influencing Weldability of the Mg-4Y-3RE Cast Magnesium alloy. IOP Conf. Ser. Mater. Sci. Eng. 22, 012002. doi:10.1088/1757-899X/22/1/012002
Knapek, M., Minárik, P., Čapek, J., Král, R., Kubásek, J., and Chmelík, F. (2018). Corrosion of Pure Magnesium and a WE43 Magnesium alloy Studied by Advanced Acoustic Emission Analysis. Corrosion Sci. 145, 10–15. doi:10.1016/j.corsci.2018.09.006
Kondori, B., and Mahmudi, R. (2017). Effect of Ca Additions on the Microstructure and Creep Properties of a Cast Mg-Al-Mn Magnesium alloy. Mater. Sci. Eng. A 700, 438–447. doi:10.1016/j.msea.2017.06.007
Kujur, M. S., Manakari, V., Parande, G., Tun, K. S., Mallick, A., and Gupta, M. (2018). Enhancement of thermal, Mechanical, Ignition and Damping Response of Magnesium Using Nano-Ceria Particles. Ceramics Int. 44, 15035–15043. doi:10.1016/j.ceramint.2018.05.133
Kulekci, M. K. (2008). Magnesium and its Alloys Applications in Automotive Industry. Int. J. Adv. Manuf Technol. 39, 851–865. doi:10.1007/s00170-007-1279-2
Le, Q.-C., Zhang, Z.-Q., Shao, Z.-W., Cui, J.-Z., and Xie, Y. (2010). Microstructures and Mechanical Properties of Mg-2%Zn-0.4%RE Alloys. Trans. Nonferrous Met. Soc. China 20, s352–s356. doi:10.1016/S1003-6326(10)60496-7
Lefstad, M., Pedersen, K., and Dumoulin, S. (2012). Up-scaled Equal Channel Angular Pressing of AA6060 and Subsequent Mechanical Properties. Mater. Sci. Eng. A 535, 235–240. doi:10.1016/j.msea.2011.12.073
Leleu, S., Rives, B., Bour, J., Causse, N., and Pébère, N. (2018). On the Stability of the Oxides Film Formed on a Magnesium alloy Containing Rare-Earth Elements. Electrochimica Acta 290, 586–594. doi:10.1016/j.electacta.2018.08.093
Leleu, S., Rives, B., Causse, N., and Pébère, N. (2019). Corrosion Rate Determination of Rare-Earth Mg Alloys in a Na2SO4 Solution by Electrochemical Measurements and Inductive Coupled Plasma-Optical Emission Spectroscopy. J. Magnesium Alloys 7, 47–57. doi:10.1016/j.jma.2018.12.002
Li, F., Peh, W. Y., Nagarajan, V., Ho, M. K., Danno, A., Chua, B. W., et al. (2016). Development of Non-flammable High Strength AZ91 + Ca Alloys via Liquid Forging and Extrusion. Mater. Des. 99, 37–43. doi:10.1016/j.matdes.2016.03.014
Li, G., Zhang, J., Wu, R., Liu, S., Song, B., Jiao, Y., et al. (2019). Improving Age Hardening Response and Mechanical Properties of a New Mg-RE alloy via Simple Pre-cold Rolling. J. Alloys Compd. 777, 1375–1385. doi:10.1016/j.jallcom.2018.11.082
Li, M., Cheng, Y., Zheng, Y. F., Zhang, X., Xi, T. F., and Wei, S. C. (2012). Surface Characteristics and Corrosion Behaviour of WE43 Magnesium alloy Coated by SiC Film. Appl. Surf. Sci. 258, 3074–3081. doi:10.1016/j.apsusc.2011.11.040
Li, Q., Wang, Q., Wang, Y., Zeng, X., and Ding, W. (2007). Effect of Nd and Y Addition on Microstructure and Mechanical Properties of As-Cast Mg-Zn-Zr alloy. J. Alloys Compd. 427, 115–123. doi:10.1016/j.jallcom.2006.02.054
Liu, C., Li, Q., Liang, J., Zhou, J., and Wang, L. (2016). Microstructure and Corrosion Behaviour of Laser Surface Melting Treated WE43 Magnesium alloy. RSC Adv. 6, 30642–30651. doi:10.1039/C5RA27010C
Liu, C., Liang, J., Zhou, J., Li, Q., Peng, Z., and Wang, L. (2016). Characterization and Corrosion Behavior of Plasma Electrolytic Oxidation Coated AZ91-T6 Magnesium alloy. Surf. Coat. Technol. 304, 179–187. doi:10.1016/j.surfcoat.2016.07.021
Liu, D. X., Pang, X., Li, D. L., Guo, C. G., Wongsa-Ngam, J., Langdon, T. G., et al. (2017). Microstructural Evolution and Properties of a Hot Extruded and HPT-Processed Resorbable Magnesium WE43 Alloy. Adv. Eng. Mater. 19, 1600698. doi:10.1002/adem.201600698
Liu, K., Rokhlin, L. L., Elkin, F. M., Tang, D., and Meng, J. (2010). Effect of Ageing Treatment on the Microstructures and Mechanical Properties of the Extruded Mg-7Y-4Gd-1.5Zn-0.4Zr alloy. Mater. Sci. Eng. A 527, 828–834. doi:10.1016/j.msea.2009.10.031
Liu, M., Zanna, S., Ardelean, H., Frateur, I., Schmutz, P., Song, G., et al. (2009). A First Quantitative XPS Study of the Surface Films Formed, by Exposure to Water, on Mg and on the Mg-Al Intermetallics: Al3Mg2 and Mg17Al12. Corrosion Sci. 51, 1115–1127. doi:10.1016/j.corsci.2009.02.017
Liu, Z., Wu, G., Liu, W., Pang, S., and Ding, W. (2013). Microstructure, Mechanical Properties and Fracture Behavior of Peak-Aged Mg34Y32Nd31Gd Alloys under Different Aging Conditions. Mater. Sci. Eng. A 561, 303–311. doi:10.1016/j.msea.2012.09.060
Lukyanova, E. A., Martynenko, N. S., Serebryany, V. N., Belyakov, A. N., Rokhlin, L. L., Dobatkin, S. V., et al. (2017). Structure and Mechanical and Corrosion Properties of a Magnesium Mg-Y-Nd-Zr Alloy after High Pressure Torsion. Russ. Metall. 2017, 912–921. doi:10.1134/S0036029517110088
Lukyanova, E. A., Martynenko, N. S., Shakhova, I., Belyakov, A. N., Rokhlin, L. L., Dobatkin, S. V., et al. (2016). Strengthening of Age-Hardenable WE43 Magnesium alloy Processed by High Pressure Torsion. Mater. Lett. 170, 5–9. doi:10.1016/j.matlet.2016.01.106
Luo, A. A. (2013). Magnesium Casting Technology for Structural Applications. J. Magnesium Alloys 1, 2–22. doi:10.1016/j.jma.2013.02.002
Luo, A. A. (2004). Recent Magnesium alloy Development for Elevated Temperature Applications. Int. Mater. Rev. 49, 13–30. doi:10.1179/095066004225010497
Luo, K., Zhang, L., Wu, G., Liu, W., and Ding, W. (2019). Effect of Y and Gd Content on the Microstructure and Mechanical Properties of Mg-Y-RE Alloys. J. Magnesium Alloys 7, 345–354. doi:10.1016/j.jma.2019.03.002
Luxfer MEL Technologies, Aircraft Interiors (2019). Luxfer MEL Technologies, Aircraft Interiors. Available at: https://www.luxfermeltechnologies.com/markets/aerospace/aircraft-interiors-magnesium-extrusion/(Accessed July 16, 2019).
Luxfer MEL Technologies, Engines (2019). Luxfer MEL Technologies, Engines. Available at: https://www.luxfermeltechnologies.com/markets/aerospace/engines/(Accessed July 16, 2019).
Lyon, P., King, J. F., and Fowler, G. A. (1991). “Developments in Magnesium Based Materials and Processes,” in International Gas Turbine and Aeroengine Congress and Exposition, Orlando, FL, USA. doi:10.1115/91-GT-015
Magnesium Alloy Fatigue Data (1995). “Magnesium Alloy Fatigue Data,” in Fatigue Data Book: Light Structural Alloys (Ohio, EUA: ASM International).
Makar, G. L., and Kruger, J. (1993). Corrosion of Magnesium. Int. Mater. Rev. 38, 138–153. doi:10.1179/imr.1993.38.3.138
Malayoğlu, U., and Tekin, K. C. (2015). Wear Behaviour of Plasma Electrolytic Oxide Coatings on E21 and WE43 Mg Alloys. Surf. Eng. 31, 526–533. doi:10.1179/1743294414Y.0000000409
Manakari, V., Parande, G., and Gupta, M. (2017). Selective Laser Melting of Magnesium and Magnesium Alloy Powders: A Review. Metals 7, 2. doi:10.3390/met7010002
Marker, T. R. (2013). Evaluating the Flammability of Various Magnesium Alloys during Laboratory- and Full-Scale Aircraft Fire Tests, Atlantic City, USA.
Martynenko, N. S., Lukyanova, E. A., Serebryany, V. N., Gorshenkov, M. V., Shchetinin, I. V., Raab, G. I., et al. (2018). Increasing Strength and Ductility of Magnesium alloy WE43 by Equal-Channel Angular Pressing. Mater. Sci. Eng. A 712, 625–629. doi:10.1016/j.msea.2017.12.026
Maruyama, K., Suzuki, M., and Sato, H. (2002). Creep Strength of Magnesium-Based Alloys. Metall. Mat Trans. A. 33, 875–882. doi:10.1007/s11661-002-1020-610.1007/s11661-002-0157-7
Mathaudhu, S. N., and Nyberg, E. A. (2014). “Magnesium Alloys in U.S. Military Applications: Past, Current and Future Solutions,” in Essential Readings in Magnesium Technology. Editors S. N. Mathaudhu, A. A. Luo, N. R. Neelameggham, E. A. Nyberg, and W. H. Sillekens (Hoboken, NJ, USA: John Wiley & Sons), 71–76. doi:10.1002/9781118859803.ch10
McClelland, Z., Avery, D. Z., Williams, M. B., Mason, C. J. T., Rivera, O. G., Leah, C., et al. (2019). “Microstructure and Mechanical Properties of High Shear Material Deposition of Rare Earth Magnesium Alloys WE43,” in The Minerals, Metals & Materials Series. Editors V. Joshi, J. Jordon, D. Orlov, and N. Neelameggham (Cham: Springer). doi:10.1007/978-3-030-05789-3_41
Mengucci, P., Barucca, G., Riontino, G., Lussana, D., Massazza, M., Ferragut, R., et al. (2008). Structure Evolution of a WE43 Mg alloy Submitted to Different thermal Treatments. Mater. Sci. Eng. A 479, 37–44. doi:10.1016/j.msea.2007.06.016
Minárik, P., Veselý, J., Čížek, J., Zemková, M., Vlasák, T., Krajňák, T., et al. (2018). Effect of Secondary Phase Particles on thermal Stability of Ultra-fine Grained Mg-4Y-3RE alloy Prepared by Equal Channel Angular Pressing. Mater. Characterization 140, 207–216. doi:10.1016/j.matchar.2018.04.006
Mirza, F. A., and Chen, D. L. (2013). “Fatigue of Magnesium Alloys,” in Aerospace Materials Handbook. Editors S. Zhang, and D. Zhao (Boca Raton, Florida, USA: CRC Press).
Mokhtarishirazabad, M., Azadi, M., Hossein Farrahi, G., Winter, G., and Eichlseder, W. (2013). Improvement of High Temperature Fatigue Lifetime in AZ91 Magnesium alloy by Heat Treatment. Mater. Sci. Eng. A 588, 357–365. doi:10.1016/j.msea.2013.09.067
Montemor, M. F. (2014). Functional and Smart Coatings for Corrosion protection: A Review of Recent Advances. Surf. Coat. Technol. 258, 17–37. doi:10.1016/j.surfcoat.2014.06.031
Montemor, M. F., Simões, A. M., and Carmezim, M. J. (2007). Characterization of Rare-Earth Conversion Films Formed on the AZ31 Magnesium alloy and its Relation with Corrosion protection. Appl. Surf. Sci. 253, 6922–6931. doi:10.1016/j.apsusc.2007.02.019
Morozov, Y., Calado, L. M., Shakoor, R. A., Raj, R., Kahraman, R., Taryba, M. G., et al. (2019). Epoxy Coatings Modified with a New Cerium Phosphate Inhibitor for Smart Corrosion protection of Steel. Corrosion Sci. 159, 108128. doi:10.1016/j.corsci.2019.108128
Moosbrugger C., (Editor) (2017). “Introduction to Magnesium Alloys,” Engineering Properties of Magnesium Alloys (Russell, OH, USA: ASM International).
Mouritz, A. P. (2012). Introduction to Aerospace Materials. Sawston, USA: Woodhead Publishing Limited.
Natta, M. G. L.-B. (2001). Evidence of Two Anodic Processes in the Polarization Curves of Magnesium in Aqueous media. Corrosion 57, 712–720. doi:10.5006/1.3290399
Nie, J.-F. (2012). Precipitation and Hardening in Magnesium Alloys. Metall. Mat Trans. A. 43, 3891–3939. doi:10.1007/s11661-012-1217-2
Nie, J. F., and Muddle, B. C. (2000). Characterisation of Strengthening Precipitate Phases in a Mg-Y-Nd alloy. Acta Materialia 48, 1691–1703. doi:10.1016/S1359-6454(00)00013-6
Nie, J. F., Xiao, X. L., Luo, C. P., and Muddle, B. C. (2001). Characterisation of Precipitate Phases in Magnesium Alloys Using Electron Microdiffraction. Micron 32, 857–863. doi:10.1016/S0968-4328(00)00094-9
Ning, Z. L., Yi, J. Y., Qian, M., Sun, H. C., Cao, F. Y., Liu, H. H., et al. (2014). Microstructure and Elevated Temperature Mechanical and Creep Properties of Mg-4Y-3Nd-0.5Zr alloy in the Product Form of a Large Structural Casting. Mater. Des. 60, 218–225. doi:10.1016/j.matdes.2014.03.062
Ninlachart, J., Karmiol, Z., Chidambaram, D., and Raja, K. S. (2017). Effect of Heat Treatment Conditions on the Passivation Behavior of WE43C Mg-Y-Nd alloy in Chloride Containing Alkaline Environments. J. Magnesium Alloys 5, 147–165. doi:10.1016/j.jma.2017.03.003
Nordlien, J. H., Nisancioglu, K., Ono, S., and Masuko, N. (1997). Morphology and Structure of Water‐Formed Oxides on Ternary MgAl Alloys. J. Electrochem. Soc. 144, 461–466. doi:10.1149/1.1837432
Oshibe, N., Marukawa, E., Yoda, T., and Harada, H. (2019). Degradation and Interaction with Bone of Magnesium alloy WE43 Implants: A Long-Term Follow-Up In Vivo Rat Tibia Study. J. Biomater. Appl. 33, 1157–1167. doi:10.1177/0885328218822050
Pachla, W., Mazur, A., Skiba, J., Kulczyk, M., and Przybysz, S. (2012). Wrought Magnesium Alloys ZM21, ZW3 and WE43 Processed by Hydrostatic Extrusion with Back Pressure. Arch. Metall. Mater. 57, 485–493. doi:10.2478/v10172-012-0050-3
Pai, B. C., Pillai, U. T. S., Manikandan, P., and Srinivasan, A. (2012). Modification of AZ91 Mg Alloys for High Temperature Applications. Trans. Indian Inst. Met. 65, 601–606. doi:10.1007/s12666-012-0166-1
Pan, F., Yang, M., and Chen, X. (2016). A Review on Casting Magnesium Alloys: Modification of Commercial Alloys and Development of New Alloys. J. Mater. Sci. Technol. 32, 1211–1221. doi:10.1016/j.jmst.2016.07.001
Penghuai, F., Liming, P., Haiyan, J., Wenjiang, D., and Chunquan, Z. (2014). Tensile Properties of High Strength Cast Mg Alloys at Room Temperature: A Review. China Foundry 11, 277–286.
Pereira, G. S., Koga, G. Y., Avila, J. A., Bittencourt, I. M., Fernandez, F., Miyazaki, M. H., et al. (2021). Corrosion Resistance of WE43 Mg alloy in Sodium Chloride Solution. Mater. Chem. Phys. 272, 124930. doi:10.1016/j.matchemphys.2021.124930
Pereira, P. H. R., Huang, Y., Kawasaki, M., and Langdon, T. G. (2017). An Examination of the Superplastic Characteristics of Al-Mg-Sc Alloys after Processing. J. Mater. Res. 32, 4541–4553. doi:10.1557/jmr.2017.286
Pinto, R., Carmezim, M. J., Ferreira, M. G. S., and Montemor, M. F. (2010). A Two-step Surface Treatment, Combining Anodisation and Silanisation, for Improved Corrosion protection of the Mg alloy WE54. Prog. Org. Coat. 69, 143–149. doi:10.1016/j.porgcoat.2010.04.014
Plaass, C., Ettinger, S., Sonnow, L., Koenneker, S., Noll, Y., Weizbauer, A., et al. (2016). Early Results Using a Biodegradable Magnesium Screw for Modified Chevron Osteotomies. J. Orthop. Res. 34, 2207–2214. doi:10.1002/jor.23241
Polmear, I. J. (1994). Magnesium Alloys and Applications. Mater. Sci. Technol. 10, 1–16. doi:10.1179/mst.1994.10.1.1
Prabhu, T. R., Vedantam, S., and Singh, V. (2017). “Magnesium Alloys,” in Aerospace Materials and Material Technologies. Editors N. E. Prasad, and R. J. H. Wanhill (Basingstoke, USA: Springer Nature). doi:10.1007/978-981-10-2134-3_1
Riontino, G., Massazza, M., Lussana, D., Mengucci, P., Barucca, G., and Ferragut, R. (2008). A Novel thermal Treatment on a Mg-4.2Y-2.3Nd-0.6Zr (WE43) alloy. Mater. Sci. Eng. A 494, 445–448. doi:10.1016/j.msea.2008.04.043
Rzychoń, T., and Kiełbus, A. (2007). Microstructure of WE43 Casting Magnesium alloy. J. Achievements Mater. Manufacturing Eng. 21, 31–34.
Rzychoń, T., Michalska, J., and Kiełbus, A. (2007). Effect of Heat Treatment on Corrosion Resistance of WE54 alloy. J. Achievements Mater. Manufacturing Eng. 20, 191–194.
Salandari-Rabori, A., Zarei-Hanzaki, A., Abedi, H. R., Lecomte, J. S., and Khatami-Hamedani, H. (2018). Micro and Macro Texture Evolution during Multiaxial Forging of a WE43 Magnesium alloy. J. Alloys Compd. 739, 249–259. doi:10.1016/j.jallcom.2017.12.181
Santamaria, M., Di Quarto, F., Zanna, S., and Marcus, P. (2007). Initial Surface Film on Magnesium Metal: A Characterization by X-ray Photoelectron Spectroscopy (XPS) and Photocurrent Spectroscopy (PCS). Electrochimica Acta 53, 1314–1324. doi:10.1016/j.electacta.2007.03.019
Shaat, M. (2018). Effects of Processing Conditions on Microstructure and Mechanical Properties of Equal-Channel-Angular-Pressed Titanium. Mater. Sci. Technol. 34, 1149–1167. doi:10.1080/02670836.2018.1478481
Shi, X., Zhu, Y., Zhang, S., Zhao, R., Zhang, R., Chen, L., et al. (2020). Characteristics of Selenium-Containing Coatings on WE43 Magnesium alloy by Micro-arc Oxidation. Mater. Lett. 261, 126944. doi:10.1016/j.matlet.2019.126944
Smola, B., Joska, L., Březina, V., Stulíková, I., and Hnilica, F. (2012). Microstructure, Corrosion Resistance and Cytocompatibility of Mg-5Y-4Rare Earth-0.5Zr (WE54) alloy. Mater. Sci. Eng. C 32, 659–664. doi:10.1016/j.msec.2012.01.003
Society of Automotive Engineers (2015). Performance Standard for Seats in Civil Rotorcraft, Transport Aircraft, and General Aviation Aircraft - AS8049C.
Soltan, A., Dargusch, M. S., Shi, Z., Gerrard, D., and Atrens, A. (2019). Understanding the Corrosion Behaviour of the Magnesium Alloys EV31A, WE43B, and ZE41A. Mater. Corrosion 70, 1527–1552. doi:10.1002/maco.201910845
Song, G., Atrens, A., and Dargusch, M. (1999). Influence of Microstructure on the Corrosion of Diecast AZ91D. Corrosion Sci. 41, 249–273. doi:10.1016/S0010-938X(98)00121-8
Song, G. L., and Atrens, A. (1999). Corrosion Mechanisms of Magnesium Alloys. Adv. Eng. Mater. 1, 11–33. doi:10.1002/(sici)1527-2648(199909)1:1<11:aid-adem11>3.0.co;2-n
StJohn, D. H., Qian, M., Easton, M. A., Cao, P., and Hildebrand, Z. (2005). Grain Refinement of Magnesium Alloys. Metall. Mat Trans. A. 36, 1669–1679. doi:10.1007/s11661-005-0030-6
Su, Z. J., Liu, C. M., Wang, Y. C., and Shu, X. (2013). Effect of Y Content on Microstructure and Mechanical Properties of Mg-2·4Nd-0·2Zn-0·4Zr Alloys. Mater. Sci. Technol. 29, 148–155. doi:10.1179/1743284712Y.0000000149
Sudholz, A. D., Gusieva, K., Chen, X. B., Muddle, B. C., Gibson, M. A., and Birbilis, N. (2011). Electrochemical Behaviour and Corrosion of Mg-Y Alloys. Corrosion Sci. 53, 2277–2282. doi:10.1016/j.corsci.2011.03.010
Suzuki, M., Kimura, T., Koike, J., and Maruyama, K. (2004). Effects of Zinc on Creep Strength and Deformation Substructures in Mg-Y alloy. Mater. Sci. Eng. A 387-389, 706–709. doi:10.1016/j.msea.2003.12.071
Szakács, G., Wiese, B., Mendis, C. L., Tolnai, D., Stark, A., Schell, N., et al. (2014). “In Situ Synchrotron Radiation Diffraction during Solidification of Mg4Y and Mg4YxGd Alloys (X=1,4 wt.%),” in Magnesium Technology. Editors M. Alderman, M. V. Manuel, N. Hort, and N. R. Neelameggham (San Diego, California, USA: Springer International Publishers), 213–218. doi:10.1007/978-3-319-48231-6
Tan, Q., Yin, Y., Mo, N., Zhang, M., and Atrens, A. (2019). Recent Understanding of the Oxidation and Burning of Magnesium Alloys. Surf. Innov. 7, 71–92. doi:10.1680/jsuin.18.00062
Tekin, K. C., Malayoğlu, U., and Shrestha, S. (2013). Electrochemical Behavior of Plasma Electrolytic Oxide Coatings on Rare Earth Element Containing Mg Alloys. Surf. Coat. Technol. 236, 540–549. doi:10.1016/j.surfcoat.2013.10.051
Toda-Caraballo, I., Galindo-Nava, E. I., and Rivera-Díaz-del-Castillo, P. E. J. (2014). Understanding the Factors Influencing Yield Strength on Mg Alloys. Acta Materialia 75, 287–296. doi:10.1016/j.actamat.2014.04.064
Torkian, A., Faraji, G., and Karimpour, M. (2018). Mechanical Properties and Microstructure of WE43 Mg Alloy Processed by Warm ECAP Followed by Extrusion. Arch. Metall. Mater. 63, 1093–1100. doi:10.24425/123781
Tun, K. S., Jayaramanavar, P., Nguyen, Q. B., Chan, J., Kwok, R., and Gupta, M. (2012). Investigation into Tensile and Compressive Responses of Mg-ZnO Composites. Mater. Sci. Technol. 28, 582–588. doi:10.1179/1743284711Y.0000000108
Walter, R., and Kannan, M. B. (2011). In-vitro Degradation Behaviour of WE54 Magnesium alloy in Simulated Body Fluid. Mater. Lett. 65, 748–750. doi:10.1016/j.matlet.2010.11.051
Wang, B. J., Xu, D. K., Wang, S. D., and Han, E. H. (2019). Fatigue Crack Initiation of Magnesium Alloys under Elastic Stress Amplitudes: A Review. Front. Mech. Eng. 14, 113–127. doi:10.1007/s11465-018-0482-1
Wang, L., Jiang, J., Ma, A., Li, Y., and Song, D. (2017). A Critical Review of Mg-Based Hydrogen Storage Materials Processed by Equal Channel Angular Pressing. Metals 7, 324. doi:10.3390/met7090324
Wang, Z., Wang, J.-G., Chen, Z.-Y., Zha, M., Wang, C., Liu, S., et al. (2019). Effect of Ce Addition on Modifying the Microstructure and Achieving a High Elongation with a Relatively High Strength of As-Extruded AZ80 Magnesium Alloy. Materials 12, 76. doi:10.3390/ma12010076
Westengen, H., and Aune, T. K. (2006). “Magnesium Casting Alloys,” in Magnesium Technology - Metallurgy, Design Data, Applications. Editors H. E. Friedrich, and B. L. Mordike (Berlin, Germany: Springer-Verlag Berlin Heidelberg).
Witecka, A., Valet, S., Basista, M., and Boccaccini, A. R. (2021). Electrophoretically Deposited High Molecular Weight Chitosan/bioactive Glass Composite Coatings on WE43 Magnesium alloy. Surf. Coat. Technol. 418, 127232. doi:10.1016/j.surfcoat.2021.127232
Witte, F., Hort, N., Vogt, C., Cohen, S., Kainer, K. U., Willumeit, R., et al. (2008). Degradable Biomaterials Based on Magnesium Corrosion. Curr. Opin. Solid State. Mater. Sci. 12, 63–72. doi:10.1016/j.cossms.2009.04.001
Xia, S. J., Yue, R., Rateick, R. G., and Birss, V. I. (2004). Electrochemical Studies of AC/DC Anodized Mg Alloy in NaCl Solution. J. Electrochem. Soc. 151, B179–B187. doi:10.1149/1.1646139
Xiang, C., Gupta, N., Coelho, P., and Cho, K. (2018). Effect of Microstructure on Tensile and Compressive Behavior of WE43 alloy in as Cast and Heat Treated Conditions. Mater. Sci. Eng. A 710, 74–85. doi:10.1016/j.msea.2017.10.084
Xie, J., Zhang, J., You, Z., Liu, S., Guan, K., Wu, R., et al. (2021). Towards Developing Mg Alloys with Simultaneously Improved Strength and Corrosion Resistance via RE Alloying. J. Magnesium Alloys 9, 41–56. doi:10.1016/j.jma.2020.08.016
Xu, D., Zhao, K., Yang, C., Li, H., and Zhang, J. (2018). Effect of Heat Treatment on Microstructure and Mechanical Properties of the AZ31/WE43 Bimetal Composites. Metals 8, 971. doi:10.3390/met8110971
Yan, J., Sun, Y., Xue, F., Xue, S., and Tao, W. (2008). Microstructure and Mechanical Properties in Cast Magnesium-Neodymium Binary Alloys. Mater. Sci. Eng. A 476, 366–371. doi:10.1016/j.msea.2007.05.058
Yang, C., Gupta, N., Ding, H., and Xiang, C. (2020). Effect of Microstructure on Corrosion Behavior of We43 Magnesium alloy in as Cast and Heat-Treated Conditions. Metals 10, 1552. doi:10.3390/met10111552
Yang, L., Li, Y., Qian, B., and Hou, B. (2015). Polyaspartic Acid as a Corrosion Inhibitor for WE43 Magnesium alloy. J. Magnesium Alloys 3, 47–51. doi:10.1016/j.jma.2014.12.009
You, S., Huang, Y., Kainer, K. U., and Hort, N. (2017). Recent Research and Developments on Wrought Magnesium Alloys. J. Magnesium Alloys 5, 239–253. doi:10.1016/j.jma.2017.09.001
Yu, K., Li, W., Wang, R., Wang, B., and Li, C. (2008). Effect of T5 and T6 Tempers on a Hot-Rolled WE43 Magnesium Alloy. Mater. Trans. 49, 1818–1821. doi:10.2320/matertrans.MRA2008602
Yuwono, J. A., Birbilis, N., Taylor, C. D., Williams, K. S., Samin, A. J., and Medhekar, N. V. (2019). Aqueous Electrochemistry of the Magnesium Surface: Thermodynamic and Kinetic Profiles. Corrosion Sci. 147, 53–68. doi:10.1016/j.corsci.2018.10.014
Zeng, Z., Stanford, N., Davies, C. H. J., Nie, J.-F., and Birbilis, N. (2019). Magnesium Extrusion Alloys: a Review of Developments and Prospects. Int. Mater. Rev. 64, 27–62. doi:10.1080/09506608.2017.1421439
Zhang, J., Niu, X., Qiu, X., Liu, K., Nan, C., Tang, D., et al. (2009). Effect of yttrium-rich misch metal on the microstructures, mechanical properties and corrosion behavior of die cast AZ91 alloy. J. Alloys Compd. 471, 322–330. doi:10.1016/j.jallcom.2008.03.089
Zhang, T., Meng, G., Shao, Y., Cui, Z., and Wang, F. (2011). Corrosion of Hot Extrusion AZ91 Magnesium alloy. Part II: Effect of Rare Earth Element Neodymium (Nd) on the Corrosion Behavior of Extruded alloy. Corrosion Sci. 53, 2934–2942. doi:10.1016/j.corsci.2011.05.035
Zhang, X., Wu, G., Peng, X., Li, L., Feng, H., Gao, B., et al. (2015). Mitigation of Corrosion on Magnesium Alloy by Predesigned Surface Corrosion. Sci. Rep. 5, 17399. doi:10.1038/srep17399
Zhang, Y., Gao, M., Etim, I. P., Tan, L., and Yang, K. (2020). Optimising the Torsional Properties and Corrosion Resistance of Biodegradable WE43 Mg alloy by ECAP and Subsequent Ageing. Mater. Technol. 35, 402–410. doi:10.1080/10667857.2019.1688539
Zhao, H. D., Qin, G. W., Ren, Y. P., Pei, W. L., Chen, D., and Guo, Y. (2011). The Maximum Solubility of Y in α-Mg and Composition Ranges of Mg24Y5−x and Mg2Y1−x Intermetallic Phases in Mg-Y Binary System. J. Alloys Compd. 509, 627–631. doi:10.1016/j.jallcom.2010.09.120
Zhao, Y., Wu, G., Lu, Q., Wu, J., Xu, R., Yeung, K. W. K., et al. (2013). Improved Surface Corrosion Resistance of WE43 Magnesium alloy by Dual Titanium and Oxygen Ion Implantation. Thin Solid Films 529, 407–411. doi:10.1016/j.tsf.2012.05.046
Zhilyaev, A., and Langdon, T. (2008). Using High-Pressure Torsion for Metal Processing: Fundamentals and Applications. Prog. Mater. Sci. 53, 893–979. doi:10.1016/j.pmatsci.2008.03.002
Zomorodian, A., Garcia, M. P., Moura E Silva, T., Fernandes, J. C. S., Fernandes, M. H., and Montemor, M. F. (2013). Corrosion Resistance of a Composite Polymeric Coating Applied on Biodegradable AZ31 Magnesium alloy. Acta Biomater. 9, 8660–8670. doi:10.1016/j.actbio.2013.02.036
Zomorodian, A., Santos, C., Carmezim, M. J., Silva, T. M. e., Fernandes, J. C. S., and Montemor, M. F. (2015). "In-vitro" Corrosion Behaviour of the Magnesium alloy with Al and Zn (AZ31) Protected with a Biodegradable Polycaprolactone Coating Loaded with Hydroxyapatite and Cephalexin. Electrochimica Acta 179, 431–440. doi:10.1016/j.electacta.2015.04.013
Zucchi, F., Grassi, V., Frignani, A., Monticelli, C., and Trabanelli, G. (2006). Electrochemical Behaviour of a Magnesium alloy Containing Rare Earth Elements. J. Appl. Electrochem. 36, 195–204. doi:10.1007/s10800-005-9053-3
Zucchi, F., Grassi, V., Frignani, A., Monticelli, C., and Trabanelli, G. (2006). Influence of a Silane Treatment on the Corrosion Resistance of a WE43 Magnesium alloy. Surf. Coat. Technol. 200, 4136–4143. doi:10.1016/j.surfcoat.2005.02.073
Zumdick, N. A., Jauer, L., Kersting, L. C., Kutz, T. N., Schleifenbaum, J. H., and Zander, D. (2019). Additive Manufactured WE43 Magnesium: A Comparative Study of the Microstructure and Mechanical Properties with Those of Powder Extruded and As-Cast WE43. Mater. Characterization 147, 384–397. doi:10.1016/j.matchar.2018.11.011
Keywords: magnesium alloys, rare earth elements, corrosion, corrosion protection, coatings
Citation: Calado LM, Carmezim MJ and Montemor MF (2022) Rare Earth Based Magnesium Alloys—A Review on WE Series. Front. Mater. 8:804906. doi: 10.3389/fmats.2021.804906
Received: 29 October 2021; Accepted: 27 December 2021;
Published: 19 January 2022.
Edited by:
Yingwei Song, Institute of Metals Research (CAS), ChinaReviewed by:
Quantong Jiang, Institute of Oceanology (CAS), ChinaBo Song, Southwest University, China
Jinhui Liu, Zhengzhou University, China
Copyright © 2022 Calado, Carmezim and Montemor. This is an open-access article distributed under the terms of the Creative Commons Attribution License (CC BY). The use, distribution or reproduction in other forums is permitted, provided the original author(s) and the copyright owner(s) are credited and that the original publication in this journal is cited, in accordance with accepted academic practice. No use, distribution or reproduction is permitted which does not comply with these terms.
*Correspondence: Lénia M. Calado, bGVuaWFjYWxhZG9AdGVjbmljby51bGlzYm9hLnB0