- 1Department of Molecular Biomedical Sciences and Comparative Medicine Institute, North Carolina State University, Raleigh, NC, United States
- 2Joint Department of Biomedical Engineering, University of North Carolina at Chapel Hill & North Carolina State University, Raleigh, NC, United States
Cardiovascular diseases are one of the leading causes of death across the globe. Heart transplantation has been used for end stage heart failure patients. However, due to the lack of donors, this treatment option usually depends on multiple variables and the result varies due to immunological issues. 3D bioprinting is an emerging approach for in vitro generation of functional cardiac tissues for drug screening and cardiac regenerative therapy. There are different techniques such as extrusion, inkjet, or laser-based 3D printing that integrate multiple cell lines with different scaffolds for the construction of complex 3D structures. In this review, we discussed the recent progress and challenges in 3D bioprinting strategies for cardiac tissue engineering, including cardiac patches, in vitro cardiac models, valves, and blood vessels.
Introduction
Cardiovascular diseases (CVD) are common, enervating and fatal malady that devours thousands of life every year (Huang et al., 2021; Mei et al., 2021). Multiple conditions like congenital heart disease, ischemic heart disease and inflammation contribute to the loss of cardiac functions (Liu N. et al., 2021). Due to the limited regenerative capacity of cardiomyocytes (CMs), adult myocardium lacks ability to self-renew after injury (CMs) (Li et al., 2018). The current treatments for myocardial infraction (MI) relieves the symptoms and modestly prolong life rather than restore the lost cardiac tissue. Heart failure (HF), the common outcome of CVD, may require a heart transplant at the end stage (Wang Q. et al., 2021). However, the number of available donors lags far behind the number required by the patients (Liu N. et al., 2021). Allogenic organ transplantation is coupled with the high risk of immune rejections and surgical complications, where the desire for long term recovery of heart function is still unfulfilled.
In regenerative treatment approach, stem cell therapies have been considerably studied for cardiac repair (Berry et al., 2019; Oh et al., 2016; Zhu and Cheng, 2021; Tang J. N. et al., 2018; Tang J. et al., 2018). Mounting evidence suggested that stem cell therapies demonstrate treatment benefit through cardiac function enhancement, infract size reduction, and angiogenesis improvement (Su et al., 2018; Huang et al., 2018; Su et al., 2019; Huang K. et al., 2020; Cheng et al., 2012b). However, they are hampered by the intrinsic limitations after transplantation, such as low cell retention, low survival rate of the engrafted cells and lack of host tissue integration (Wang and Guan, 2010; Ong et al., 2017). Hence, applying contemporary technology to build functional artificial cardiac tissue in vitro has been a Frontier direction in tissue engineering (Tomov et al., 2019).
Cardiovascular tissue engineering encompasses cell biology, material science and biofabrication that facilitates the generation therapeutics for CVD (Noh et al., 2018; Zhu and Cheng, 2021). Within tissue engineering, bioprinting both 2-dimensional (2D) and 3-dimensional (3D) has emerged as a modern fabrication method that utilizes live cells, bioactive molecules, and biomaterials for both in vitro and in vivo applications. Mimetic heart tissues are can fabricated using 3D bioprinting, which successfully capture the intricacy of the native cellular composition and matrix structure. (Bejleri et al., 2018; Ong et al., 2017; Liu et al., 2019; Chingale et al., 2021). These fabricated constructs have been further used for drug screening and regenerative studies (Ong et al., 2017; Liu et al., 2019). For the treatment of aortic valve disease and bypass procedures, 3D bioprinted aortic valves and conduits have been used. (Hockaday et al., 2012; Kang et al., 2016; Pountos et al., 2019). In ischemic events, the functionality of the cardiac tissue can be preserved using 3D cardiac patches (Gaetani et al., 2015; Jang et al., 2017; Izadifar et al., 2018). Also, 3D printed vascular grafts have enhanced structural stability and biocompatibility (Marga et al., 2012; Kucukgul et al., 2015). Using 3D bioprinted cardiac patch, fabricated by human coronary artery endothelial cells, methacrylate collagen micropatterning, and an alginate matrix (Izadifar et al., 2018) showed high cell proliferation, migration and differentiation. This review provides an overview of cardiovascular 3D bioprinting techniques, their potential applications, limitations and also the future prospects of this new technology (Figure 1).
3D Bioprinting Approaches for Cardiovascular Tissues
3D bioprinting is a layer-by-layer additive technology that precisely deposits biomaterials and active cells in accordance with a certain spatial pattern that has high resolution stimulation of the heart (Mir & Nakamura, 2017). High resolution stimulation of is a method of evaluation of the functional attributes of the cardiac tissue and means of induction of various arrhythmia (Issa et al., 2019). Briefly, the 3D bioprinting procedure begins with the gathering of the patient’s data through imaging tools like computed tomography (CT), magnetic resonance imaging (MRI) to design a computer-aided design (CAD) model. This model has a well-defined structure, architecture and porosity (Hockaday et al., 2012; Burke et al., 2017). 3D bioprinting techniques is further bifurcated scaffold-based printing and scaffold free printing (Table 1). The predominant approaches of scaffold based 3D bioprinting are extrusion, inkjet, laser assisted printing and stereolithography (Pountos et al., 2019). Using the various 3D bioprinting approaches, a number of tissue constructs like cartilage (Wang et al., 2016a; Luo et al., 2012; Shi et al., 2017; Cui et al., 2012a), neural tissue (Radulescu et al., 2007; England et al., 2017) heart valve (Hockaday et al., 2012; Duan, et al., 2013) have been successfully constructed.
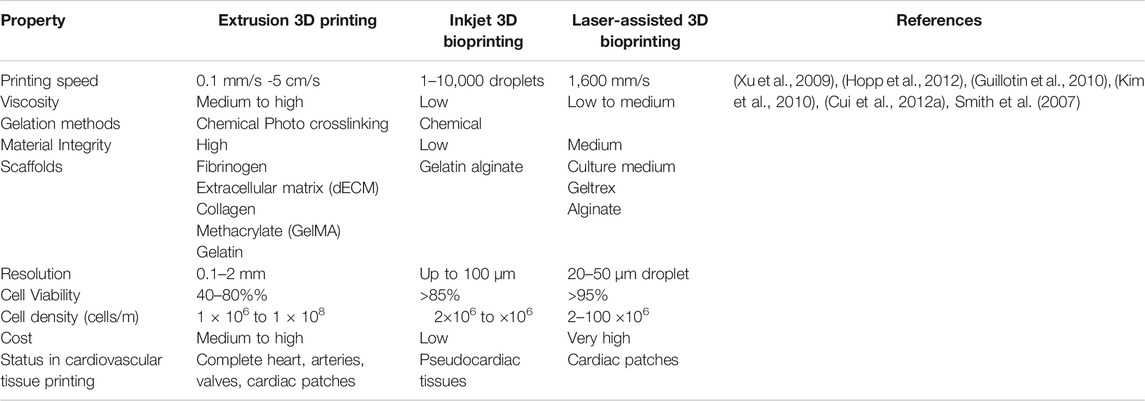
TABLE 1. Comparison Between different scaffold-based 3D printing techniques for cardiovascular applications.
Scaffold-Based 3D Printing
Scaffold based 3D bioprinting is used to generate tissue constructs using cells, biological macromolecules, and structural moieties. The scaffold-based materials have facile surface functionalization and superior physicochemical properties (Sarkhosh-Inanlou et al., 2021). These biomaterials facilitate the reconstruction and repair of various anatomical defects that may occur in the cardiac tissues. The porosity of the scaffolds allows the movement of the biological fluids which in turn amplifies the cell adhesion, proliferation, migration and differentiation (O’Brien, 2011). Furthermore, many scaffolds based 3D printing techniques have been under investigation that exhibit low inflammation and toxicity, and low rejection of scaffold through autoimmunity (O’Brien, 2011; Ng et al., 2012; Tariverdian et al., 2019). Extrusion-based bioprinting, inkjet-based bioprinting and laser-based bioprinting are the three major scaffold-based bioprinting modalities for cardiovascular tissue fabrication (Qasim et al., 2019).
Extrusion-Based 3D Bioprinting
The extrusion-based 3D printing is a facile, less complex and affordable 3D printing approach (Mandrycky et al., 2016; Kato et al., 2021). The cell deposition density usually ranges from 108–109 cells per mL which is crucial for cardiac tissue engineering (Pati et al., 2014). Although extrusion based 3D bioprinting offers high cell densities, due to high stress and dispensing pressure (>6 × 107 mPa s), the cell viability of only about 40–80% (Mandrycky et al., 2016). Promising results are seen in 3D bioprinting of myocardium constructs, heart valves, and blood vessels (Alonzo et al., 2019). In this printing technique, the cells are suspended in a prepolymer solution, loaded in syringes and printed in the form of cylindrical filament precisely into the target 3D cardiac tissue structure by using pneumatic or mechanical forces (Liu et al., 2017; Mistry et al., 2017; Byambaa et al., 2017). To construct durable 3D cardiac tissues, factors like viscosity, shape of the needle, pressure and gauge size should be accurately optimized (Hölzl et al., 2016). Here, the 3D constructs are constructs by sequentially depositing the hydrogel filaments that have the diameters in a range of 150–300 μm (Pereira and Bártolo, 2015). Recently, a 3D human chambered cardiac muscle was constructed using a photo-crosslinking native extracellular matrix (ECM) proteins and human induced pluripotent stem cells (hiPSC)- laden structures (Kupfer et al., 2020). This 3D chamber muscle possessed a continuous action-potential propagation along with macroscale beating. Using freeform reversible embedding of suspending hydrogels (FRESH v2.0), a modified extrusion-based 3D bioprinting technique was developed to bioprint a model of the left ventricle of human heart left ventricle of human heart. Here, collagen type I and human embryonic stem cell–derived cardiac fibroblasts (hESC-CFs) were used (Lee et al., 2019a). Using gelatin methacryloyl (GelMA) as a cell carrier, a combination of cardiac myocytes and cardiac fibroblasts was used to generate a self-contracting cardiac muscle, called engineered heart tissues (EHT) (Koti et al., 2019). Furthermore, GATA binding protein 4 (GATA4) -transfected Mesenchymal Stem Cells (MSCs) that were derived from human umbilical cord-derived (hUC-MSCs) expressed cardiac marker proteins and differentiated further into cells similar to cardiomyocyte. These naked plasmids when encapsulated in polyurethane (PU) hydrogel can upregulate specific cardiac marker genes and indicates as a new approach for in situ cardiac therapeutics (Huang N. C. et al., 2020). A tissue of cardiogenic potential was printed using human cardiac-derived cardiomyocyte progenitor cells (hCMPCs) in alginate scaffold that increase of the early cardiac transcription factor Nkx, Mef-2c, GATA4 also, late cardiac marker Troponin T (TnT) was also highly expressed (Gaetani et al., 2012).
Inkjet-Based 3D Bioprinting
Inkjet 3D based bioprinter allows the precise positioning of cells and biomaterials in the targeted cardiac tissue construct using droplets (1–100 pL) with cell densities as high as 10,000–30,000 cells/drop (Calvert, 2007), ejected via thermal (Cui et al., 2012b) or acoustic forces (Xu et al., 2008). In piezoelectric inkjet printer the piezoelectric crystals produce acoustic waves and force the liquid through the nozzle (Pereira and Bártolo, 2015). Whereas, in the thermal inkjet bioprinting, the droplets are expelled out from the nozzle by the pressure generated by the vaporizing the bioink around the heating element (Cui et al., 2012b). The inkjet 3D bioprinter is compatible with most of the biomaterials and has a cell viability of up to 90% (Wang Z. et al., 2021). The inkjet 3D bioprinter is compatible with biomaterials having specific viscosity of 3.5–12 mPa/s where specific viscosity is the increase in the viscosity of the scaffold beyond that of the solvent due to polymer additive (Murphy and Atala, 2014; Chawla et al., 2018). Inkjet printer was used to construct a layer-by-layer 3D bioprinted aortic tissue construct, using Mouse Embryonic Fibroblast (MEF) cell aggregates with hydrogel support. This tissue construct was a hierarchical design of functional cardiac pseudo tissue with balanced porosity, structural support and a beating cell response (Alonzo et al., 2019). In another study, 3D rectangular sheet was fabricated with alternating layers of alginate hydrogels and primary feline adult and H1 cardiomyocytes (Xu et al., 2009). However, due to the noncontact nature of inkjet printers and its dispensing mechanisms, the 3D constructs usually have weak mechanical properties (Lee et al., 2009). Therefore, the inkjet aided bioprinting of the cardiac tissue specifically is still in its infancy stage (Cui and Boland, 2009).
Laser-Assisted 3D Bioprinting
Laser-assisted bioprinting (LAB) facilitates the formation of in vitro tissue constructs. Based on the principle of Laser-Induced Forward Transfer (LIFT), LAB is being progressively used for tissue- and organ-engineering (Barron et al., 2004). LAB exhibits high precision and resolution which can print high cell density (108cells/ml) and viscosity (10–100) μm scaffolds. Using a laser pulse repetition rate of 5 kHz, and speed of 1,600 mm/s. LAB can print bioinks with negligible effect on cell viability and function (Ventura, 2021; Barron et al., 2004; Murphy and Atala, 2014). LIFT has been used to construct a defined patterned cardiac patch seeded with human umbilical vein endothelial cells (huvec) and hMSC on a Polyester urethane urea (PEUU) for cardiac regeneration (Gaebel et al., 2011). Also, laser bioprinting of undifferentiated hiPSCs in combination with different biomaterials like collagen, alginate, hyaluronic acid, fibrinogen, fibrin proved that hiPSCs are sensitive to the biomaterials, not to laser printing. Biomaterials, such as the hyaluronic acid, matrigel support hiPSCs differentiation and can be laser printed without losing their pluripotency (Koch et al., 2018).
Stereolithography
Stereolithography (STL) is a nozzle-free technology based on photo-sensitive polymer formulation. It utilizes ultraviolet (UV) or visible light to cure photosensitive polymers in a layer-by-layer manner (Budharaju et al., 2021). This technique is fast and provides accurate fabrication, that has resolutions ranging from 5to 300 µm (Raman et al., 2016). Photoinitiators molecules are sensitive to different ranges of wavelength and initiate the polymer chains which in turn affects the stiffness and density of the cured resins (Pereira and Bártolo, 2015). Due to the toxic nature of some of the photoinitiators, commonly used least cytotoxic photoinitators are Irgacure 2,959 for UV cross-linkage and eosin Y for visible light (Noshadi et al., 2017). Since, UV light induce mutations, visible light-based photocross-linkage have been used more often in SLA (Ikehata and Ono, 2011). Using STL, a MSCs hydrogel patch was constructed with cross linked poly (ethylene glycol) dimethacrylate (PEGDMA) with desired diameter of microchannel suitable for sustained release of cytokines from cells resulted in enhance cardiac function and minimized cardiac remodeling (Melhem et al., 2017).
Digital Light Processing
Digital light processing (DLP) uses a digital micromirroe device (DMV) chip that is composed of approximately millions of micromirrors that reflects light and project an optical pattern that is dictated by CAD model on the photopolymer solution (Lu et al., 2006). The resolution of this printer controlled by the focal size of the light beam from each micromirror. In DLP printer prints parallely by projecting the entire plane of optical pattern onto the photopolyer solution, thus reducing the time required for the fabrication (Zhang et al., 2012). Compared to the other 3D bioprinting approaches, DLP has significant advantages in prining resolution, efficiency, and working condition (Zhang et al., 2019). Human embryonic stem cell derived cardiomyocytes (hECMs- CMs) and hydrogel were used to fabricate a cardiac tissue using DLP based 3D printing. The DLP facilitated the 3D bioprinting of hECMs- CMs mimicing the multilayed alignment of the myocardium. This tissue construct was furthre used to detect simultaneously both calcium transients and mechanical force that is essential for in vitro disease modeling (Zhang et al., 2012). Recently, using rapid light based 3D printing (DLP), a cardiac tissue construt made of Human induced pluripotent stem cell - derived cardiomyocytes (iPSC-CMs) was used to detect the expression of mature cardiac marker genes (Ma et al., 2019).
Scaffolds for 3D Bioprinting
Cardiac tissue engineering aims to regenerate the injured cardiac tissue by combining cells and highly porous scaffold biomaterials to act as templates for tissue regeneration (O’Brien, 2011). It is of paramount importance that the scaffold possesses native ECM like texture and morphology for precise 3D cardiac construct fabrication (Zhu K. et al., 2017). The 3D scaffolds must have patterned channels that mimic the function of the endothelial network (Usprech et al., 2017). These patterned 3D channels promote the cell migration, physiology, morphology, and phenotype. They also play an important role in stem cell differentiation into cardiomyogenic phenotype (Zhang et al., 2016). In Tissue engineering, scaffold (hydrogel) materials should support cell adhering, cell proliferation along possessing key attributes like printability, degradation kinetics, biocompatible and material biomimicry (Table 2) (Murphy and Atala, 2014; Sahai and Gogoi, 2019). Hydrogels that are currently used in the cardiac regenerative medicine are either naturally derived polymers (like alginate, gelatin, collagen, chitosan, fibrin and hyaluronic acid) or synthetic molecules (polyethylene glycol, PEG) (Murphy and Atala, 2014; Sun et al., 2012; Spiller et al., 2011). Natural polymers provide the advantage in 3D bioprinting as they mimic the ECM both in terms of morphology and bioactivity. The synthetic polymers like hydrogels are also a preferred choice due to the ease of manipulating and tailoring and physical properties as per the target tissue. The challenges with the synthetic polymers include toxic degradation, loss of mechanical properties and poor biocompatibility (Li and Kawashita, 2011; Prasad, 2021).
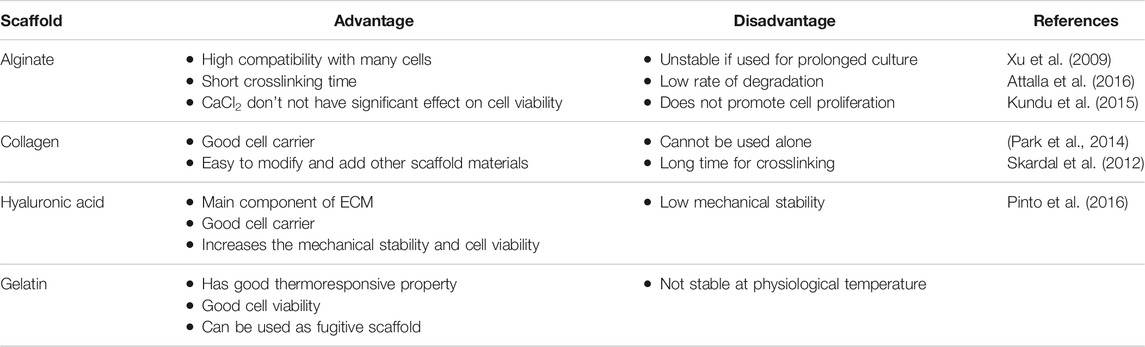
TABLE 2. Advantages and disadvantages of scaffold biomaterials used for 3D bioprinting of cardiovascular tissues.
For precise 3D printing of cardiac constructs, proper selection of scaffold based on the type of 3D bioprinting modality used is very crucial. Hydrogels used for extrusion based 3D bioprinting are usually non-Newtonian fluids, where the viscosity and sheer rate are corelated (Jungst et al., 2016). In addition, the scaffold should possess low surface tension and low adhesion properties along with rapid gelation characteristics (Jia et al., 2016). In inkjet-based 3D printing, the scaffold must have low viscosity and non-fibrous in nature to ensure easy flow through the tubing system. Also, the scaffold must have rheopectic behavior where the viscosity increases with applied shear (Mandrycky et al., 2016). A scaffold with sufficient adhesion and low surface tension characteristics can spread uniformly and adhere is best suited for laser assisted 3D bioprinting. Also, the scaffold chosen should exhibit viscoelasticity as it can enhance cell viability. The viscoelasticity properties of the scaffolds can be altered to achieve high cell densities (Nooranidoost et al., 2019). Also the scaffold should possesses rapid gelation capability, solidifying speed up to 10 s as it helps to maintain structural fidelity. (Wang et al., 2016b). The following functional scaffolds have been used in cardiac 3D printing.
Alginate
Alginate is a common hydrogel used in 3D bioprinting due to its, easy of handling, price, crosslinking, bio-inertness, printability and biocompatibility (Paques et al., 2013; Selcan Gungor-Ozkerim et al., 2018). Alginate is chemical modified to promote desirable cell function and mechanical support (Agarwal et al., 2021). The viscosity of alginate depends on its concentration hence alginate at lower concentrations leads to shape distortion of the 3D constructs which in turn affects cell adhesion, proliferation and distribution of the differentiated cells (Paques et al., 2013). Modifying the alginate surface using peptides sequence (arginine–glycine–aspartic acid) RGD, results in enhanced cell attachment on alginate (Paques et al., 2013). Using chelating agents like ethylenediaminetetraacetic acid (EDTA), alginate can be eliminated after printing the 3D constructs. In clinical trials for patients affected by myocardial infraction, Algisyl-LVR™ has been approved for treatment (Liberski et al., 2016). The human cardiac-derived cardiomyocyte progenitor cells (hCMPCs) printed in alginate scaffold in vitro culture demonstrated increased cardiac commitment of hCMPCs without altering the cell viability and proliferation. A significant increase cardiac marker TnT and cardiac transcription factor Nkx2.5, GATA-4, Mef-2c was observed (Maiullari et al., 2018).
Hyaluronic Acid
Hyaluronic acid (HA) and its derivatives are biocompatible, not toxic and plays a central role in cell support. HA is modified to readily crosslink as it lacks spontaneous ionic/enzymatic gelation and used in 3D bioprinting due to this high viscosity (Duan, et al., 2013). It is a glycosaminoglycan (GAG) found in morphogenesis (Rosines et al., 2007), wound repair (Kikuchi et al., 2005), cell migration and signaling (Lokeshwar and Selzer, 2000). HA is a preferred choice in tissue engineering as its important role during early embryonic development its biocompatible nature and also the ability to control its architecture and degradation (Hölzl et al., 2016). HA displays a slow gelation rate, along easy mechanical and chemical modifications are required to enhance the rheological properties (Hospodiuk et al., 2017). Human cardiosphere-derived cells (CDCs) when delivered with FDA-approved Hyaluronan based scaffold Hystem-C™ hydrogel, showed high cell engraftment and therapeutic efficacy (Cheng et al., 2012a); . A photocrosslinked hydrogel constructed from oxidized and methacrylated HA which enabled the cell-hybrid hydrogel interaction stimulated cell migration, proliferation, GAG secretion. This study provides a proof that the hybrid hydrogels mimic layer specific valve ECM and are a good choice for heart valve tissue engineering (Duan, et al., 2013).
Fibrinogen
Fibrinogen is a fibrous plasma protein that is actively involved in wound thrombosis, wound healing, angiogenesis. Fibrinogen is also involved in cell adhesion, migration, proliferation and differentiation (Budharaju et al., 2021). Fibrinogen is often used in 3D printing of cardiac tissues as it undergoes spontaneous gelation in the presence of thrombin and has docking sites for many proteins like VEGF, IL-1, albumin, Von Willebrand factor (Brown and Barker, 2014). By adding FDA approved chemicals like aminocaproic acid, aprotinin during the gel preparation, plasminogen mediated proteolytic degradation of fibrin can be avoided (Wang et al., 2018). Since fibrinogen is less viscous and poses weak rheological properties, fibrinogen is used with other biomaterials like Matrigel, collagen during the printing process (Hölzl et al., 2016). A advanced model of cardiac tissue constructed using HUVECs and iPSC-CMs encapsulated in alginate and PEG-Fibrinogen hydrogel strands demonstrated functional integration of the host’s vasculature into the 3D bioprinted constructs, supplying blood to the implant and preventing necrosis and endorsing heart-like engineered tissue (Maiullari et al., 2018).
Decellularized ECM
Cardiac ECM contains is madeup of fibronectin collagen I, fibrillin 1, and laminin and other tissue specific growth factors (Williams et al., 2014). Decellularized ECM (dECM) has gained attention due to its high biocompatibility, printability and thermoresponsive nature (Garreta et al., 2017). Cardiac dECM can be prepared from porcine sheep by decellularization followed by lyophilization (He and Callanan, 2013). The dECM mimics the architecture of the cardiac ECM and facilitates the process of cell adhesion, proliferation and differentiation and migration (Ott et al., 2008). When myocardial ECM hydrogel was injected into the infract region of rat hearts, the RNA transcriptome analysis revealed the upregulation of neovascularization, cardiac transcription factors and the pathways associated. The factors associated with apoptosis, fibrosis were downregulated (Seif-Naraghi et al., 2013; Spang and Christman, 2018). Using dECM scaffold, a bioconstruct that mimicked native cardiac ECM microenvironment was fabricated that show high cell viability and proliferation of cardiac progenitor cells, that resulted in the increased cardiomyogenic differentiation (Jang et al., 2016).
Gelatin
Gelatin is a derivative of collagen that contains RGD cell-binding motifs like collagen. Gelatin is less immunogenic and takes active part in cell adhesion, differentiation, migration, and proliferation (Davidenko et al., 2016). Gelatin is sensitive to temperature, concentration, solvent and crosslinking agents that in turn affects the thermoresponsive nature of gelatin (Zhu W. et al., 2017). Due to poor mechanical characteristics, gelatin is chemically cross linked with agents like glutaraldehyde before using for 3D bioprinting (Hellio and Djabourov, 2006). Using extrusion-based three-dimensional (3D) bioprinting (Yin et al., 2018) reported accurate deposition of cell-laden GelMA in microarchitectures that showed high cell viability. To build patient specific soft tissue, gelatin-methacrylate gelMA hydrogel using poly -actic-co-glycolic acid nanofiber fragments (PLGA-NF) was fabricated that enhanced the biomechanical properties and stability and also promoted fibroblast proliferation in gelMA/PLGA-NF hydrogel (Ko and Kwon, 2020).
Cell Sources for 3D Printing
For the accurate functioning of the fabricated constructs, the proper choice of cells for tissues is crucial. There are various cell types in tissues and organs that are endowed with specific functions, and these attributes must be recapitulated in the new 3D bioprinted construct. The cells present in the native organ are mainly involved in providing structural support and are actively involved in maintaining the stem cell niche (Murphy and Atala, 2014). For the 3D bioprinted cardiac construct to be functional in the long term, the cardiac tissue construe must maintain homeostasis, respond to tissue injury and self-renew (Budharaju et al., 2021).
Primary Cardiomyocytes
The adult primary cardiomyocytes are terminally differentiated and where the Ca+ regulates the functional characteristics, thus making them an excellent choice for tissue engineering (Foglia and Poss, 2016). By using primary cardiomyocytes and polycaprolactone support frame, a contractile cardiac construct was developed that was structurally organized and scalable The 3D bioprinted cardiac tissue construct was a dense, aligned cardiac muscle bundles that possessed synchronous contraction and responded to known cardiac medications. These medications acted by blocking which notch pathway and promoted improved cardiac tissue formation (Wang et al., 2018). A EHT constructed, contained aligned homologous cardiomyocytes and a synthetic supporting polymer polyethylene vinyl acetate (PEVA) that enhanced the expression of cadherins, integrin proteins, differentiation and maturation with increased functionality (Das et al., 2019).
Stem Cells
Stem cells like iPSCs, ESCs, MSCs (Li et al., 2022), cardiac progenitor cells have been cultured in 2D cell cultures and further differentiated to functional cardiomyocyte (Kattman et al., 2006; Wang X. et al., 2021). The growth factors and differentiation factors present in the matrix of scaffolds play an important role in the cell fate. Among the different proteins in the ECM, integrin is important as it activates transcription factors to future differentiate into cardiomyocyte (Lee, 2018). Mouse ESCs plated on polyglycolic-acid (PGA) material patches and transferred to ischemic and peri-ischemic mice myocardium 3 days after seeding. The survival rate, blood pressure along with ventricular function in the mice heart with ESCs patch was better than the sham operated and cell free patches mice hearts thus demonstrating that ESCs aided the cardiac repair of infracted myocardium (Ke et al., 2005).
hiPSC-Derived Cardiomyocytes
To design an accurate cardiac construct, researchers have integrated stem cells derived human cardiac cells in the construct (Liu et al., 2019). Human induced pluripotent stem cell-derived cardiomyocytes (hiPSC-CMs) are superior to primary cardiomyocytes as they can expand prior to differentiation to cardiomyocytes and also are easy to scale up (Zuppinger, 2019). GelMA-a photopolymerizable ECM has adhesion moieties and natural degradation making it a popular choice for printing encapsulated cells (Yu et al., 2020). To design hiPSC-CMs models, cells have been encapsulated in ECM, Fibrin or gelatin methacrylate (GelMA) (Veldhuizen et al., 2019) to facilitate the formation of connections in 3D space (Ma et al., 2018). The recently developed micro-continuous optical printing system (μCOP) is a perfect 3D bioprinting technique that supports high 3D printing resolution, biocompatibility and speed (Ma et al., 2018; Liu et al., 2020). 3D cardiac tissue that is constructed using hiPSC-CM and human cardiac fibroblasts (HCF) that is coated with (ECM) and plates on the fabricated HBC gel frame, enhances the contractile function and vascular function (Tsukamoto et al., 2020). A study established that a 3D in vitro model of cardiac fibrosis made of TGF-β1 treated 3D cardiac microtissues promoted myofibroblast proliferation and differentiation (Lee et al., 2019b). However, hiPSC-CMs are not preferred for adult cardiac tissue constructs as the new differentiated hiPSC-CMs possess limited tissue alignment and have deficient calcium handling (Ronaldson-Bouchard et al., 2018).
Scaffold–Free Bioprinting
Though scaffolds have been successfully used in tissue engineering, the issues that still persist are low biodegradability and adverse immune response which are life threatening (Gao et al., 2017). Hence, scaffold free printing, has emerged as an alternative for artificial tissue/organ fabrication (Zhu W. et al., 2017). In scaffold-free 3D bioprinting, cell adhesion is crucial as it compensates for the scaffold support. For tissue repair and regeneration, several scaffold free printing methods have be developed like pallet culture (Estes and Guilak, 2011), hanging drop (Lee et al., 2012), hydrodynamic cell trapping (Fu et al., 2014) and spinner flask (Rodday et al., 2011).
Briefly in all these techniques, the cells are outspread in a 3D environment where their differentiation results into a mass due to cell-cell adhesion. After the cells grow in a definite shape, they are placed in a layered pattern with the aid of 3D printer and then cultured with other cells to form a tissue like structure (Tan et al., 2014). A biomaterial free cardiac patch was developed to deliver hiPSC-CMs, fibroblasts (FB) and endothelial cells (EC) as mixed cell spheroids. These 3D printed patches show uniform electrical conduction and also exhibit action potential waveforms as in the ventricle. Upon implantation, vascularization and engraftment with the myocardium was seen (Ong et al., 2017) thus, suggesting a next generation stem cell based treatment for heart failure. A scaffold free cardiac graft patch was developed that was made of spheroids containing CMs, FB and EC that promoted rapid self-organization and vascularization (Noguchi et al., 2016). Though a thicker myocardial graft could not be fabricated, this approach could potentially be developed to treat myocardium injury. In a scaffold free 3D cell sheet, MSCs are seeded and cultivated on temperature-responsive polymer-grafted cell culture dishes (TRCD) at 37°C. At confluency, the cells deposit ECM and form interactions along with forming channels with the neighboring cells. The cells are detached at confluency by reduction of temperature from 37 to 20°C to induce changes in the surface properties, that results in the change from hydrophobic to hydrophilic nature of the culture dished and releases the adherent cells. Spontaneous tissue contraction upon the release from the 2D monolayer resulted in the increase in the thickness of the cell sheet rendering an increase in the volume of the cardiac like tissue construct. This tissue has demonstrated an upregulation in the expression of vascular hepatocyte growth factor (HGF), interleukin -10(IL-10), endothelial growth factor (VEGF) (Bou-Ghannam et al., 2021). The advantage and disadvantages of scaffold-based or scaffold-free bioprinting were summarized in Table 3.
Application
Though the cardiac tissue is complex, 3D bioprinting has emerged as a next generation treatment approach for generating cardiovascular implants that have biomimetic qualities. These 3D implants recapitulate both morphological as well as biochemical attributes of the native cardiac tissue. Here, we discuss the 3D bioprinting strategies used to fabricated cardiac tissues such as myocardium, cardiac valves, and cardiac models for drug screening.
3D Bioprinted Myocardium
The myocardium is an intricately organized muscle layer in the heart wall that plays a crucial role in the contraction and relaxation of the heart. Myocardium is made of nearly 2-4 billon cardiomyocytes (Laflamme and Murry, 2011). During a cardiac injury, there is a loss of cardiomyocytes accompanied with modification in the cardiac ECM (Gaetani et al., 2012). Hence cardiac 3D bioprinted constructs/implants aim in repopulating the cardiomyocytes and ECM for cardiac repair (Mathur et al., 2016). An engineered human myocardium (EHM) that mimicked the postnatal heart both in terms of structural and functional properties was fabricated in vitro under well-defined serum free conditions using ESCs and iPSCs. These EHM exhibited attributes like cardiomyocytes with M bands, systolic twitch, positive force frequency response along with molecular advancement. This EHM can potentially be used for cardiac repair, drug screening and disease modeling (Tiburcy et al., 2017). ‘VentriGel’- a first in man clinical research implant, fabricated from porcine heart-derived dECM-based hydrogel and transendocardially injected in early and late post MI patients with left ventricular dysfunction. Post injection, there was an increase in the vascularization, reduced cardiomyocyte dystrophy and fibrosis (Traverse et al., 2019). Cardiac patches composed of cECM, human cardiac progenitor cells (hCPCs) and gelatin methacrylate (GelMA) were implanted in vivo on rat hearts. After 14 days of implantation, an increase angiogenic potential (>2 fold) along with improved endothelial cell tube formation was observed which indicated that these patches could be used as potential treatment for repairing the damaged myocardium (Bejleri et al., 2018).
3D Bioprinted Cardiac Valves
Apart from myocardial damage, cardiac valve dysfunction represents another major reason for heart failure (Howell and Butcher, 2012). The cardiac valves are mainly made of valve interstitial cells (VICs), SMCs, and valvular endothelial cells (VECs) (Klebe, 1988). In cardiac valve diseases, the valves are either incapable to close or are too contracted to open entirely. In such conditions, valve replacement surgery is the only option where prosthetic valves are employed. Though the prosthetic valves have high longevity, thrombogenicity is a major issue (Maxson et al., 2019). A 3D bioprinted aortic heart valve scaffold was fabricated using rat MSCs onto gelatin support gel. In vivo studies proved that this scaffold was capable of alteration and an increase in elastin, vimentin, alpha SMA, and CD31 was observed in all 12 weeks (Maxson et al., 2019). 3D bioprinted aortic valve conduit constructed directly by encapsulating aortic root sinus smooth muscle cells (SMC) and aortic valve leaflet interstitial cells (VIC) within alginate/gelatin hydrogel discs improved cell viability, migration and proliferation (Duan et al., 2013).
3D Bioprinted Blood Vessels
Impaired blood circulation to heart underlies enervating conditions like ischemia, heart failure and stroke (Mozaffarian et al., 2015). Functional vascular network facilitates the adequate delivery of oxygen, nutrients, unwanted metabolite removal and constant circulation of immune cells which in turn plays a crucial role cardiovascular regeneration (Duan, 2017). 3D bioprinted viable cardiac tissue constructs require imbedded blood vessel networks for nutrient transport and cellular waste disposal (Jafarkhani et al., 2019). Using bone marrow MSCs and sodium alginate/gelatin, a cardiac patch was designed and implanted on the injured heart. 5-azacytidine (5-Aza) induced differentiation of MSCs leds to morphological changes and expression of α-striate muscle actine antibody (α-SCA) and desmin antibody (DES) and other proteins. This cardiac patch repairs the injured cardiomyocytes by generating new cardiomyocytes, rather, than increasing the blood supply and increasing the cardiac functionality by promoting vascular proliferation. Also, the implanted cardiac patch demonstrated improved epicardial activation that promoted angiogenesis and EMT through WT1 (Wilms tumor protein 1)-mediated Wnt/β-catenin signaling pathway (Liu X. et al., 2021). Using the in vivo priming strategy, bone marrow MSCs were primed in vivo by genetically induced hepatocyte growth factor -expressing MSCs (HGF-eMSCs). These MSCs and HGF-eMSCs were encapsulated within a 3D cardiac patch. On implantation on MI induced heart, an improvement in cardiac function, prevented apoptosis along with the enhancement of vessel formation was observed post MI (Park et al., 2020).
3D Bioprinted for Drug Screening In Vitro
3D printing offers a great platform in pharmaceutics for drug discovery and development (Sharma et al., 2021; Vijayavenkataraman et al., 2018). In cardiac regenerative medicine, efforts are mainly focus on recreating biomimetic microtissues of left ventricular myocardium as it is the primary pumping chamber and site for pathologies (Ma et al., 2018). Recently, an µCOP system was adopted to construct a scalable 3D model that mimicked the function and microarchitecture of the ventricular myocardium (Liu et al., 2020) that could be used in drug screening. A customizable force computing asymmetric system was designed that directly printed encapsulated cardiomyocyte. Here the NMVCMs formed a contracting tissue that possessed improved alignment physiological responsiveness to ionotropic stimulation (Liu et al., 2020). Using microfluid technology 3D endothelialized microfibrous scaffold with precisely controlled macroscale microfibers and seeded cardiomyocytes that induced the formatin of myocardium that possessed spontaneous and synchrous contraction (Zhang et al., 2016; Veldhuizen et al., 2019).
Limitations
The innovations in 3D printing in tissue engineering for cardiovascular repair and regenerative research has made tremendous stride in the recent years. The advantage of 3D bioprinting approach is its ability of accurately printing high resolution constructs of different biomaterials, cells, and therapeutics for the fabrication of highly complex 3D cardiac constructs. Despite of the significant progress and sophistication in 3D bioprinting in tissue engineering, the fabrication of a fully functional heart is yet to achieve. In 3D bioprinting, one of the major challenges is the printing resolution. For the fabricated new tissue to be closely mimetic to the native tissue, the ideal resolution much be comparable to the cell size. For the clinical application, a multilayered tissue is required. It is a challenge to generate controlled vascular network for the survival for the cells (Lovett et al., 2009). In the recent years, the 3D bioprinting technique has advanced in achieving structural complexity, but the 3D bioprinting of soft materials is still immature (Lee and Dai, 2017). Another limitation of the 3D bioprinting is that there is no standard and accepted method to access the accuracy and efficiency of the fabricated models which results in the variability. Some of current biomaterials used do not truly replicated the mechanical properties of the human heart limiting the evaluation of the tissue behavior (Harb et al., 2019). Another issue in 3D bioprinting is the determination of scaffold and optimizing the process parameters. Also, the present polymeric materials lack appropriate conductivity and weak mechanical strength as compared to native cardiomyocytes. For CTE engineers, degradability, and biocompatibility of scaffold along with cells interaction and migration in the scaffolds remains an important challenge. In the present times, 3D bioprinted of cardiac tissue is still in infancy, in the coming years, once the above issues are addressed, 3D bioprinting will enable the translation of technology to personalize therapeutic and pharmaceutical applications.
Prospects
Cardiovascular constructs (vasculature constructs, heart valves, myocardium), have been successfully 3D bioprinted with discrete structure and function. However, all these techniques are still in infancy and face several challenge which hinder the accurate construction of cardiac analogs with full functionality and complex micro-architecture (Cui et al., 2018). The recent advancements on the field of 3D printing and tissue engineering have shown to provide a platform for studying and understanding the unknown mechanism of development of diseases and its responsiveness to new developing drugs. This science continues to grow and evolve with the addition of new advanced technologies like bioelectronics, next generation sequencing etc. For instance, the convergence of bioelectronics and 3D printing helps to generate a functional live tissues like cardiac muscles with electrophysiological signals (Ershad et al., 2019). Also, the integration of biosensors and engineered tissue devices further helps in real-time monitoring of parameters which in turn can provide insight in morphogenesis, pathogenesis, and drug responsive remodeling processes in disease conditions (Ni et al., 2017). Using patient driven stem cells, 3D printed cardiac tissues can be used to study the in vitro responses of individual characteristics (Sun, 2020). This facilitates higher treatment efficacy by enabling personalized prescriptions and treatments.
Conclusion
The 3D bioprinting technology is novel and cutting-edge. it is one of the most popular tissue engineering methods as it facilitates the rapid creation of complex biostructures. Currently, significant efforts are being made to develop the technique to improve printing accuracy and speed, as well as the capacity to capture the tissue’s complexity. Future advancements in the field of 3D bioprinting will offer new doors and speed up cardiac regenerative medicine research.
Author Contributions
MC wrote the text of this review paper with guidance from KH and KC. All authors have reviewed the final version and approve of the content in this manuscript.
Funding
This work was supported by grants from the National Institutes of Health (R01 HL154154, HL137093, HL144002, HL146153, HL154154, and HL147357 to KC) and the American Heart Association (19EIA34660286 to KC, and 21CDA855570 to KH).
Conflict of Interest
The authors declare that the research was conducted in the absence of any commercial or financial relationships that could be construed as a potential conflict of interest.
Publisher’s Note
All claims expressed in this article are solely those of the authors and do not necessarily represent those of their affiliated organizations, or those of the publisher, the editors and the reviewers. Any product that may be evaluated in this article, or claim that may be made by its manufacturer, is not guaranteed or endorsed by the publisher.
References
Agarwal, T., Fortunato, G. M., Hann, S. Y., Ayan, B., Vajanthri, K. Y., Presutti, D., et al. (2021). Recent Advances in Bioprinting Technologies for Engineering Cardiac Tissue. Mater. Sci. Eng. C 124, 112057. doi:10.1016/j.msec.2021.112057
Alonzo, M., AnilKumar, S., Roman, B., Tasnim, N., and Joddar, B. (2019). 3D Bioprinting of Cardiac Tissue and Cardiac Stem Cell Therapy. Translational Res. 211, 64–83. doi:10.1016/j.trsl.2019.04.004
Attalla, R., Ling, C., and Selvaganapathy, P. (2016). Fabrication and Characterization of Gels with Integrated Channels Using 3D Printing with Microfluidic Nozzle for Tissue Engineering Applications. Biomed. Microdevices 18 (1), 17. doi:10.1007/s10544-016-0042-6
Barron, J. A., Ringeisen, B. R., Kim, H., Spargo, B. J., and Chrisey, D. B. (2004). Application of Laser Printing to Mammalian Cells. Thin Solid Films 453-454, 383–387. doi:10.1016/j.tsf.2003.11.161
Bejleri, D., Streeter, B. W., Nachlas, A. L. Y., Brown, M. E., Gaetani, R., Christman, K. L., et al. (2018). A Bioprinted Cardiac Patch Composed of Cardiac‐Specific Extracellular Matrix and Progenitor Cells for Heart Repair. Adv. Healthc. Mater. 7 (23), 1800672. doi:10.1002/adhm.201800672
Berry, J. L., Zhu, W., Tang, Y. L., Krishnamurthy, P., Ge, Y., Cooke, J. P., et al. (2019). Convergences of Life Sciences and Engineering in Understanding and Treating Heart Failure. Circ. Res. 124 (1), 161–169. doi:10.1161/circresaha.118.314216
Bou-Ghannam, S., Kim, K., Grainger, D. W., and Okano, T. (2021). 3D Cell Sheet Structure Augments Mesenchymal Stem Cell Cytokine Production. Sci. Rep. 11 (1), 8170. doi:10.1038/s41598-021-87571-7
Brown, A. C., and Barker, T. H. (2014). Fibrin-based Biomaterials: Modulation of Macroscopic Properties through Rational Design at the Molecular Level. Acta Biomater. 10 (4), 1502–1514. doi:10.1016/j.actbio.2013.09.008
Budharaju, H., Subramanian, A., and Sethuraman, S. (2021). Recent Advancements in Cardiovascular Bioprinting and Bioprinted Cardiac Constructs. Biomater. Sci. 9 (6), 1974–1994. doi:10.1039/d0bm01428a
Burke, M., Carter, B. M., and Perriman, A. W. (2017). Bioprinting: Uncovering the Utility Layer-By-Layer. J. 3D Printing Med. 1 (3), 165–179. doi:10.2217/3dp-2017-0006
Byambaa, B., Annabi, N., Yue, K., Trujillo-de Santiago, G., Alvarez, M. M., Jia, W., et al. (2017). Bioprinted Osteogenic and Vasculogenic Patterns for Engineering 3D Bone Tissue. Adv. Healthc. Mater. 6 (16). doi:10.1002/adhm.201700015
Chawla, S., Midha, S., Sharma, A., and Ghosh, S. (2018). Silk‐Based Bioinks for 3D Bioprinting. Adv. Healthc. Mater. 7 (8), 1701204. doi:10.1002/adhm.201701204
Cheng, K., Blusztajn, A., Shen, D., Li, T.-S., Sun, B., Galang, G., et al. (2012a). Functional Performance of Human Cardiosphere-Derived Cells Delivered in an In Situ Polymerizable Hyaluronan-Gelatin Hydrogel. Biomaterials 33 (21), 5317–5324. doi:10.1016/j.biomaterials.2012.04.006
Cheng, K., Shen, D., Smith, J., Galang, G., Sun, B., Zhang, J., et al. (2012b). Transplantation of Platelet Gel Spiked With Cardiosphere-Derived Cells Boosts Structural and Functional Benefits Relative to Gel Transplantation Alone in Rats With Myocardial Infarction. Biomaterials 33, 2872–2879. doi:10.1016/j.biomaterials.2011.12.040
Chingale, M., Zhu, D., Cheng, K., and Huang, K. (2021). Bioengineering Technologies for Cardiac Regenerative Medicine. Front. Bioeng. Biotechnol. 9, 361. doi:10.3389/fbioe.2021.681705
Cui, H., Miao, S., Esworthy, T., Zhou, X., Lee, S.-j., Liu, C., et al. (2018). 3D Bioprinting for Cardiovascular Regeneration and Pharmacology. Adv. Drug Deliv. Rev. 132, 252–269. doi:10.1016/j.addr.2018.07.014
Cui, X., Breitenkamp, K., Finn, M. G., Lotz, M., and D'Lima, D. D. (2012a). Direct Human Cartilage Repair Using Three-Dimensional Bioprinting Technology. Tissue Eng. Part. A. 18 (11–12), 1304–1312. doi:10.1089/ten.TEA.2011.0543
Cui, X., Boland, T., D.D'Lima, D., and K. Lotz, M. (2012b). Thermal Inkjet Printing in Tissue Engineering and Regenerative Medicine. Ddf 6 (2), 149–155. doi:10.2174/187221112800672949
Cui, X., and Boland, T. (2009). Human Microvasculature Fabrication Using thermal Inkjet Printing Technology. Biomaterials 30 (31), 6221–6227. doi:10.1016/j.biomaterials.2009.07.056
Das, S., Kim, S.-W., Choi, Y.-J., Lee, S., Lee, S.-H., Kong, J.-S., et al. (2019). Decellularized Extracellular Matrix Bioinks and the External Stimuli to Enhance Cardiac Tissue Development In Vitro. Acta Biomater. 95, 188–200. doi:10.1016/j.actbio.2019.04.026
Davidenko, N., Schuster, C. F., Bax, D. V., Farndale, R. W., Hamaia, S., Best, S. M., et al. (2016). Evaluation of Cell Binding to Collagen and Gelatin: a Study of the Effect of 2D and 3D Architecture and Surface Chemistry. J. Mater. Sci. Mater. Med. 27 (10), 148. doi:10.1007/s10856-016-5763-9
Duan, B., Hockaday, L. A., Kang, K. H., and Butcher, J. T. (2013). 3D Bioprinting of Heterogeneous Aortic Valve Conduits with Alginate/gelatin Hydrogels. J. Biomed. Mater. Res. 101A (5), 1255–1264. doi:10.1002/jbm.a.34420
Duan, B. (2017). State-of-the-art Review of 3D Bioprinting for Cardiovascular Tissue Engineering. Ann. Biomed. Eng. 45 (1), 195–209. doi:10.1007/s10439-016-1607-5
England, S., Rajaram, A., Schreyer, D. J., and Chen, X. (2017). Bioprinted Fibrin-Factor XIII-Hyaluronate Hydrogel Scaffolds with Encapsulated Schwann Cells and Their In Vitro Characterization for Use in Nerve Regeneration. Bioprinting 5, 1–9. doi:10.1016/j.bprint.2016.12.001
Ershad, F., Sim, K., Thukral, A., Zhang, Y. S., and Yu, C. (2019). Invited Article: Emerging Soft Bioelectronics for Cardiac Health Diagnosis and Treatment. APL Mater. 7 (3), 031301. doi:10.1063/1.5060270
Estes, B. T., and Guilak, F. (2011). “Three-dimensional Culture Systems to Induce Chondrogenesis of Adipose-Derived Stem Cells,” in Adipose-Derived Stem Cells: Methods and Protocols. Editors J. M. Gimble, and B. A. Bunnell (Totowa: Humana Press), 201–217. doi:10.1007/978-1-61737-960-4_15
Foglia, M. J., and Poss, K. D. (2016). Building and Re-building the Heart by Cardiomyocyte Proliferation. Development 143 (5), 729–740. doi:10.1242/dev.132910
Fu, C.-Y., Tseng, S.-Y., Yang, S.-M., Hsu, L., Liu, C.-H., and Chang, H.-Y. (2014). A Microfluidic Chip with a U-Shaped Microstructure Array for Multicellular Spheroid Formation, Culturing and Analysis. Biofabrication 6 (1), 015009. doi:10.1088/1758-5082/6/1/015009
Gaebel, R., Ma, N., Liu, J., Guan, J., Koch, L., Klopsch, C., et al. (2011). Patterning Human Stem Cells and Endothelial Cells with Laser Printing for Cardiac Regeneration. Biomaterials 32 (35), 9218–9230. doi:10.1016/j.biomaterials.2011.08.071
Gaetani, R., Doevendans, P. A., Metz, C. H. G., Alblas, J., Messina, E., Giacomello, A., et al. (2012). Cardiac Tissue Engineering Using Tissue Printing Technology and Human Cardiac Progenitor Cells. Biomaterials 33 (6), 1782–1790. doi:10.1016/j.biomaterials.2011.11.003
Gaetani, R., Feyen, D. A. M., Verhage, V., Slaats, R., Messina, E., Christman, K. L., et al. (2015). Epicardial Application of Cardiac Progenitor Cells in a 3D-Printed Gelatin/hyaluronic Acid Patch Preserves Cardiac Function after Myocardial Infarction. Biomaterials 61, 339–348. doi:10.1016/j.biomaterials.2015.05.005
Gao, L., Kupfer, M. E., Jung, J. P., Yang, L., Zhang, P., Da Sie, Y., et al. (2017). Myocardial Tissue Engineering with Cells Derived from Human-Induced Pluripotent Stem Cells and a Native-like, High-Resolution, 3-dimensionally Printed Scaffold. Circ. Res. 120 (8), 1318–1325. doi:10.1161/circresaha.116.310277
Garreta, E., Oria, R., Tarantino, C., Pla-Roca, M., Prado, P., Fernández-Avilés, F., et al. (2017). Tissue Engineering by Decellularization and 3D Bioprinting. Mater. Today 20 (4), 166–178. doi:10.1016/j.mattod.2016.12.005
Guillotin, B., Souquet, A., Catros, S., Duocastella, M., Pippenger, B., Bellance, S., et al. (2010). Laser Assisted Bioprinting of Engineered Tissue with High Cell Density and Microscale Organization. Biomaterials 31 (28), 7250–7256. doi:10.1016/j.biomaterials.2010.05.055
Gungor-Ozkerim, P. S., Inci, I., Zhang, Y. S., Khademhosseini, A., and Dokmeci, M. R. (2018). Bioinks for 3D Bioprinting: An Overview. Biomater. Sci. 6 (5), 915–946. doi:10.1039/c7bm00765e
Harb, S. C., Rodriguez, L. L., Vukicevic, M., Kapadia, S. R., and Little, S. H. (2019). Three-dimensional Printing Applications in Percutaneous Structural Heart Interventions. Circ. Cardiovasc. Imaging 12 (10), e009014. doi:10.1161/CIRCIMAGING.119.009014
He, M., and Callanan, A. (2013). Comparison of Methods for Whole-Organ Decellularization in Tissue Engineering of Bioartificial Organs. Tissue Eng. B: Rev. 19 (3), 194–208. doi:10.1089/ten.teb.2012.0340
Hellio, D., and Djabourov, M. (2006). Physically and Chemically Crosslinked Gelatin Gels. Macromol. Symp. 241 (1), 23–27. doi:10.1002/masy.200650904
Hockaday, L. A., Kang, K. H., Colangelo, N. W., Cheung, P. Y. C., Duan, B., Malone, E., et al. (2012). Rapid 3D Printing of Anatomically Accurate and Mechanically Heterogeneous Aortic Valve Hydrogel Scaffolds. Biofabrication 4 (3), 035005. doi:10.1088/1758-5082/4/3/035005
Hölzl, K., Lin, S., Tytgat, L., Vlierberghe, S. V., Gu, L., and Ovsianikov, A. (2016). Bioink Properties before, during and after 3D Bioprinting. Biofabrication 8 (3), 032002.
Hopp, B., Smausz, T., Szabó, G., Kolozsvari, L., Nogradi, A., Kafetzopoulos, D., et al. (2012). Femtosecond Laser Printing of Living Cells Using Absorbing Film-Assisted Laser-Induced Forward Transfer. Opt. Eng. 51 (1), 014302. doi:10.1117/1.oe.51.1.014302
Hospodiuk, M., Dey, M., Sosnoski, D., and Ozbolat, I. T. (2017). The Bioink: A Comprehensive Review on Bioprintable Materials. Biotechnol. Adv. 35 (2), 217–239. doi:10.1016/j.biotechadv.2016.12.006
Howell, E. J., and Butcher, J. T. (2012). Valvular Heart Diseases in the Developing World: Developmental Biology Takes center Stage. J. Heart Valve Dis. 21 (2), 234–240.
Huang, K., Hu, S., and Cheng, K. (2018). A New Era of Cardiac Cell Therapy: Opportunities and Challenges. Adv. Healthc. Mater., 1801011. doi:10.1002/adhm.201801011
Huang, K., Ozpinar, E. W., Su, T., Tang, J., Shen, D., Qiao, L., et al. (2020). An Off-The-Shelf Artificial Cardiac Patch Improves Cardiac Repair After Myocardial Infarction in Rats and Pigs. Sci. Transl. Med. 12, eaat9683. doi:10.1126/scitranslmed.aat9683
Huang, N.-C., Lee, C.-M., and Hsu, S.-h. (2020). Effective Naked Plasmid DNA Delivery into Stem Cells by Microextrusion-Based Transient-Transfection System for In Situ Cardiac Repair. Cytotherapy 22 (2), 70–81. doi:10.1016/j.jcyt.2019.12.003
Huang, S., Lei, D., Yang, Q., Yang, Y., Jiang, C., Shi, H., et al. (2021). A Perfusable, Multifunctional Epicardial Device Improves Cardiac Function and Tissue Repair. Nat. Med. 27 (3), 480–490. doi:10.1038/s41591-021-01279-9
Ikehata, H., and Ono, T. (2011). The Mechanisms of UV Mutagenesis. Jrr 52 (2), 115–125. doi:10.1269/jrr.10175
Issa, Z. F., Miller, J. M., and Zipes, D. P. (2019). “Electrophysiological Testing,” in Clinical Arrhythmology and Electrophysiology. Editors Z. F. Issa, J. M. Miller, and D. P. Zipes. Third Edition (Elsevier), 81–124. doi:10.1016/b978-0-323-52356-1.00004-9
Izadifar, M., Chapman, D., Babyn, P., Chen, X., and Kelly, M. E. (2018). UV-assisted 3D Bioprinting of Nanoreinforced Hybrid Cardiac Patch for Myocardial Tissue Engineering. Tissue Eng. C: Methods 24 (2), 74–88. doi:10.1089/ten.tec.2017.0346
Jafarkhani, M., Salehi, Z., Salehi, Z., Aidun, A., and Shokrgozar, M. A. (2019). Bioprinting in Vascularization Strategies. ibj 23 (1), 9–20. doi:10.29252/ibj.23.1.9
Jang, J., Kim, T. G., Kim, B. S., Kim, S.-W., Kwon, S.-M., and Cho, D.-W. (2016). Tailoring Mechanical Properties of Decellularized Extracellular Matrix Bioink by Vitamin B2-Induced Photo-Crosslinking. Acta Biomater. 33, 88–95. doi:10.1016/j.actbio.2016.01.013
Jang, J., Park, H.-J., Kim, S.-W., Kim, H., Park, J. Y., Na, S. J., et al. (2017). 3D Printed Complex Tissue Construct Using Stem Cell-Laden Decellularized Extracellular Matrix Bioinks for Cardiac Repair. Biomaterials 112, 264–274. doi:10.1016/j.biomaterials.2016.10.026
Jia, W., Gungor-Ozkerim, P. S., Zhang, Y. S., Yue, K., Zhu, K., Liu, W., et al. (2016). Direct 3D Bioprinting of Perfusable Vascular Constructs Using a Blend Bioink. Biomaterials 106, 58–68. doi:10.1016/j.biomaterials.2016.07.038
Jungst, T., Smolan, W., Schacht, K., Scheibel, T., and Groll, J. (2016). Strategies and Molecular Design Criteria for 3D Printable Hydrogels. Chem. Rev. 116 (3), 1496–1539. doi:10.1021/acs.chemrev.5b00303
Kang, H.-W., Lee, S. J., Ko, I. K., Kengla, C., Yoo, J. J., and Atala, A. (2016). A 3D Bioprinting System to Produce Human-Scale Tissue Constructs with Structural Integrity. Nat. Biotechnol. 34 (3), 312–319. doi:10.1038/nbt.3413
Kato, B., Wisser, G., Agrawal, D. K., Wood, T., and Thankam, F. G. (2021). 3D Bioprinting of Cardiac Tissue: Current Challenges and Perspectives. J. Mater. Sci. Mater. Med. 32 (5), 54. doi:10.1007/s10856-021-06520-y
Kattman, S. J., Huber, T. L., and Keller, G. M. (2006). Multipotent Flk-1+ Cardiovascular Progenitor Cells Give Rise to the Cardiomyocyte, Endothelial, and Vascular Smooth Muscle Lineages. Dev. Cel 11 (5), 723–732. doi:10.1016/j.devcel.2006.10.002
Kikuchi, S., Griffin, C. T., Wang, S.-S., and Bissell, D. M. (2005). Role of CD44 in Epithelial Wound Repair. J. Biol. Chem. 280 (15), 15398–15404. doi:10.1074/jbc.m414048200
Kim, J. D., Choi, J. S., Kim, B. S., Chan Choi, Y., and Cho, Y. W. (2010). Piezoelectric Inkjet Printing of Polymers: Stem Cell Patterning on Polymer Substrates. Polymer 51 (10), 2147–2154. doi:10.1016/j.polymer.2010.03.038
Klebe, R. (1988). Cytoscribing: A Method for Micropositioning Cells and the Construction of Two- and Three-Dimensional Synthetic Tissues. Exp. Cel Res. 179 (2), 362–373. doi:10.1016/0014-4827(88)90275-3
Ko, Y.-G., and Kwon, O. H. (2020). Reinforced Gelatin-Methacrylate Hydrogels Containing Poly(lactic-Co-Glycolic Acid) Nanofiber Fragments for 3D Bioprinting. J. Ind. Eng. Chem. 89, 147–155. doi:10.1016/j.jiec.2020.04.021
Koch, L., Deiwick, A., Franke, A., Schwanke, K., Haverich, A., Zweigerdt, R., et al. (2018). Laser Bioprinting of Human Induced Pluripotent Stem Cells-The Effect of Printing and Biomaterials on Cell Survival, Pluripotency, and Differentiation. Biofabrication 10 (3), 035005. doi:10.1088/1758-5090/aab981
Koti, P., Muselimyan, N., Mirdamadi, E., Asfour, H., and Sarvazyan, N. A. (2019). Use of GelMA for 3D Printing of Cardiac Myocytes and Fibroblasts. J. 3D Printing Med. 3 (1), 11–22. doi:10.2217/3dp-2018-0017
Kucukgul, C., Ozler, S. B., Inci, I., Karakas, E., Irmak, S., Gozuacik, D., et al. (2015). 3D Bioprinting of Biomimetic Aortic Vascular Constructs with Self-Supporting Cells. Biotechnol. Bioeng. 112 (4), 811–821. doi:10.1002/bit.25493
Kundu, J., Shim, J.-H., Jang, J., Kim, S.-W., and Cho, D.-W. (2015). An Additive Manufacturing-Based PCL-Alginate-Chondrocyte Bioprinted Scaffold for Cartilage Tissue Engineering. J. Tissue Eng. Regen. Med. 9 (11), 1286–1297. doi:10.1002/term.1682
Laflamme, M. A., and Murry, C. E. (2011). Heart Regeneration. Nature 473 (7347), 326–335. doi:10.1038/nature10147
Lee, K., Kim, C., Young Yang, J., Lee, H., Ahn, B., Xu, L., et al. (2012). Gravity-oriented Microfluidic Device for Uniform and Massive Cell Spheroid Formation. Biomicrofluidics 6 (1), 014114. doi:10.1063/1.3687409
Lee, A., Hudson, A. R., Shiwarski, D. J., Tashman, J. W., Hinton, T. J., Yerneni, S., et al. (2019a). 3D Bioprinting of Collagen to Rebuild Components of the Human Heart. Science 365 (6452), 482–487. doi:10.1126/science.aav9051
Lee, M.-O., Jung, K. B., Jo, S.-J., Hyun, S.-A., Moon, K.-S., Seo, J.-W., et al. (2019b). Modelling Cardiac Fibrosis Using Three-Dimensional Cardiac Microtissues Derived from Human Embryonic Stem Cells. J. Biol. Eng. 13 (1), 15. doi:10.1186/s13036-019-0139-6
Lee, R. T. (2018). Adult Cardiac Stem Cell Concept and the Process of Science. Circulation 138 (25), 2940–2942. doi:10.1161/circulationaha.118.036407
Lee, V. K., and Dai, G. (2017). Printing of Three-Dimensional Tissue Analogs for Regenerative Medicine. Ann. Biomed. Eng. 45 (1), 115–131. doi:10.1007/s10439-016-1613-7
Lee, W., Debasitis, J. C., Lee, V. K., Lee, J.-H., Fischer, K., Edminster, K., et al. (2009). Multi-layered Culture of Human Skin Fibroblasts and Keratinocytes through Three-Dimensional Freeform Fabrication. Biomaterials 30 (8), 1587–1595. doi:10.1016/j.biomaterials.2008.12.009
Li, J., Lv, Y., Zhu, D., Mei, X., Huang, K., Wang, X., et al. (2022). Intrapericardial Hydrogel Injection Generates High Cell Retention and Augments Therapeutic Effects of Mesenchymal Stem Cells in Myocardial Infarction. Chem. Eng. J. 427, 131581. doi:10.1016/j.cej.2021.131581
Li, Y., He, L., Huang, X., Bhaloo, S. I., Zhao, H., Zhang, S., et al. (2018). Genetic Lineage Tracing of Nonmyocyte Population by Dual Recombinases. Circulation 138 (8), 793–805. doi:10.1161/circulationaha.118.034250
Li, Z., and Kawashita, M. (2011). Current Progress in Inorganic Artificial Biomaterials. J. Artif. Organs 14 (3), 163–170. doi:10.1007/s10047-011-0585-5
Liberski, A., Latif, N., Raynaud, C., Bollensdorff, C., and Yacoub, M. (2016). Alginate for Cardiac Regeneration: From Seaweed to Clinical Trials. gcsp 2016. doi:10.21542/gcsp.2016.4
Liu, J., He, J., Liu, J., Ma, X., Chen, Q., Lawrence, N., et al. (2019). Rapid 3D Bioprinting of In Vitro Cardiac Tissue Models Using Human Embryonic Stem Cell-Derived Cardiomyocytes. Bioprinting 13, e00040. doi:10.1016/j.bprint.2019.e00040
Liu, J., Miller, K., Ma, X., Dewan, S., Lawrence, N., Whang, G., et al. (2020). Direct 3D Bioprinting of Cardiac Micro-tissues Mimicking Native Myocardium. Biomaterials 256, 120204. doi:10.1016/j.biomaterials.2020.120204
Liu, N., Ye, X., Yao, B., Zhao, M., Wu, P., Liu, G., et al. (2021). Advances in 3D Bioprinting Technology for Cardiac Tissue Engineering and Regeneration. Bioactive Mater. 6 (5), 1388–1401. doi:10.1016/j.bioactmat.2020.10.021
Liu, W., Heinrich, M. A., Zhou, Y., Akpek, A., Hu, N., Liu, X., et al. (2017). Extrusion Bioprinting of Shear-Thinning Gelatin Methacryloyl Bioinks. Adv. Healthc. Mater. 6 (12). doi:10.1002/adhm.201601451
Liu, X., Xu, M., Li, P., Zhao, S., Yang, G., Zhang, W., et al. (2021). Implantation and Repair of 3D Printed Myocardial Patch in Rabbit Model of Myocardial Infarction. Bioprinting 24, e00165. doi:10.1016/j.bprint.2021.e00165
Lokeshwar, V. B., and Selzer, M. G. (2000). Differences in Hyaluronic Acid-Mediated Functions and Signaling in Arterial, Microvessel, and Vein-Derived Human Endothelial Cells. J. Biol. Chem. 275 (36), 27641–27649. doi:10.1074/jbc.m003084200
Lovett, M., Lee, K., Edwards, A., and Kaplan, D. L. (2009). Vascularization Strategies for Tissue Engineering. Tissue Eng. Part B: Rev. 15 (3), 353–370. doi:10.1089/ten.teb.2009.0085
Lu, Y., Mapili, G., Suhali, G., Chen, S., and Roy, K. (2006). A Digital Micro-mirror Device-Based System for the Microfabrication of Complex, Spatially Patterned Tissue Engineering Scaffolds. J. Biomed. Mater. Res. 77A (2), 396–405. doi:10.1002/jbm.a.30601
Luo, Y., Wu, C., Lode, A., and Gelinsky, M. (2012). Hierarchical Mesoporous Bioactive Glass/alginate Composite Scaffolds Fabricated by Three-Dimensional Plotting for Bone Tissue Engineering. Biofabrication 5 (1), 015005. doi:10.1088/1758-5082/5/1/015005
Ma, X., Dewan, S., Liu, J., Tang, M., Miller, K. L., Yu, C., et al. (2019). 3D Printed Micro-scale Force Gauge Arrays to Improve Human Cardiac Tissue Maturation and Enable High Throughput Drug Testing. Acta Biomater. 95, 319–327. doi:10.1016/j.actbio.2018.12.026
Ma, X., Liu, J., Zhu, W., Tang, M., Lawrence, N., Yu, C., et al. (2018). 3D Bioprinting of Functional Tissue Models for Personalized Drug Screening and In Vitro Disease Modeling. Adv. Drug Deliv. Rev. 132, 235–251. doi:10.1016/j.addr.2018.06.011
Maiullari, F., Costantini, M., Milan, M., Pace, V., Chirivì, M., Maiullari, S., et al. (2018). A Multi-Cellular 3D Bioprinting Approach for Vascularized Heart Tissue Engineering Based on HUVECs and iPSC-Derived Cardiomyocytes. Sci. Rep. 8 (1), 13532. doi:10.1038/s41598-018-31848-x
Mandrycky, C., Wang, Z., Kim, K., and Kim, D.-H. (2016). 3D Bioprinting for Engineering Complex Tissues. Biotechnol. Adv. 34 (4), 422–434. doi:10.1016/j.biotechadv.2015.12.011
Marga, F., Jakab, K., Khatiwala, C., Shepherd, B., Dorfman, S., Hubbard, B., et al. (2012). Toward Engineering Functional Organ Modules by Additive Manufacturing. Biofabrication 4 (2), 022001. doi:10.1088/1758-5082/4/2/022001
Mathur, A., Ma, Z., Loskill, P., Jeeawoody, S., and Healy, K. E. (2016). In Vitro cardiac Tissue Models: Current Status and Future Prospects. Adv. Drug Deliv. Rev. 96, 203–213. doi:10.1016/j.addr.2015.09.011
Maxson, E. L., Young, M. D., Noble, C., Go, J. L., Heidari, B., Khorramirouz, R., et al. (2019). In Vivo remodeling of a 3D-Bioprinted Tissue Engineered Heart Valve Scaffold. Bioprinting 16, e00059. doi:10.1016/j.bprint.2019.e00059
Mei, X., Zhu, D., Li, J., Huang, K., Hu, S., Li, Z., et al. (2021). A Fluid-Powered Refillable Origami Heart Pouch for Minimally Invasive Delivery of Cell Therapies in Rats and Pigs. Med 2 (11), 1253–1268. doi:10.1016/j.medj.2021.10.001
Melhem, M. R., Park, J., Knapp, L., Reinkensmeyer, L., Cvetkovic, C., Flewellyn, J., et al. (2017). 3D Printed Stem-Cell-Laden, Microchanneled Hydrogel Patch for the Enhanced Release of Cell-Secreting Factors and Treatment of Myocardial Infarctions. ACS Biomater. Sci. Eng. 3 (9), 1980–1987. doi:10.1021/acsbiomaterials.6b00176
Mistry, P., Aied, A., Alexander, M., Shakesheff, K., Bennett, A., and Yang, J. (2017). Bioprinting Using Mechanically Robust Core-Shell Cell-Laden Hydrogel Strands. Macromol Biosci. 17 (6). doi:10.1002/mabi.201600472
Mozaffarian, D., Benjamin, E. J., Go, A. S., Arnett, D. K., Blaha, M. J., Cushman, M., et al. (2015). Heart Disease and Stroke Statistics--2015 Update: a Report from the American Heart Association. Circulation 131 (4), e29–322. doi:10.1161/CIR.0000000000000152
Murphy, S. V., and Atala, A. (2014). 3D Bioprinting of Tissues and Organs. Nat. Biotechnol. 32 (8), 773–785. doi:10.1038/nbt.2958
Ng, R., Zang, R., Yang, K. K., Liu, N., and Yang, S.-T. (2012). Three-dimensional Fibrous Scaffolds with Microstructures and Nanotextures for Tissue Engineering. RSC Adv. 2 (27), 10110–10124. doi:10.1039/c2ra21085a
Ni, Y., Ji, R., Long, K., Bu, T., Chen, K., and Zhuang, S. (2017). A Review of 3D-Printed Sensors. Appl. Spectrosc. Rev. 52 (7), 623–652. doi:10.1080/05704928.2017.1287082
Noh, S., Myung, N., Park, M., Kim, S., Zhang, S.-U., and Kang, H.-W. (2018). “3D Bioprinting for Tissue Engineering,” in Clinical Regenerative Medicine in Urology. Editor B. W. Kim (Springer), 105–123. doi:10.1007/978-981-10-2723-9_5
Nooranidoost, M., Izbassarov, D., Tasoglu, S., and Muradoglu, M. (2019). A Computational Study of Droplet-Based Bioprinting: Effects of Viscoelasticity. Phys. Fluids 31 (8), 081901. doi:10.1063/1.5108824
Noshadi, I., Hong, S., Sullivan, K. E., Shirzaei Sani, E., Portillo-Lara, R., Tamayol, A., et al. (2017). In Vitro and In Vivo Analysis of Visible Light Crosslinkable Gelatin Methacryloyl (GelMA) Hydrogels. Biomater. Sci. 5 (10), 2093–2105. doi:10.1039/c7bm00110j
Oh, H., Ito, H., and Sano, S. (2016). Challenges to success in Heart Failure: Cardiac Cell Therapies in Patients with Heart Diseases. J. Cardiol. 68 (5), 361–367. doi:10.1016/j.jjcc.2016.04.010
Ong, C. S., Fukunishi, T., Zhang, H., Huang, C. Y., Nashed, A., Blazeski, A., et al. (2017). Biomaterial-free Three-Dimensional Bioprinting of Cardiac Tissue Using Human Induced Pluripotent Stem Cell Derived Cardiomyocytes. Sci. Rep. 7 (1), 4566. doi:10.1038/s41598-017-05018-4
Ott, H. C., Matthiesen, T. S., Goh, S.-K., Black, L. D., Kren, S. M., Netoff, T. I., et al. (2008). Perfusion-decellularized Matrix: Using Nature's Platform to Engineer a Bioartificial Heart. Nat. Med. 14 (2), 213–221. doi:10.1038/nm1684
Paques, J. P., van der Linden, E., van Rijn, C. J. M., and Sagis, L. M. C. (2013). Alginate Submicron Beads Prepared through W/o Emulsification and Gelation with CaCl2 Nanoparticles. Food Hydrocolloids 31 (2), 428–434. doi:10.1016/j.foodhyd.2012.11.012
Park, B. W., Jung, S. H., Das, S., Lee, S. M., Park, J. H., Kim, H., et al. (2020). In Vivo priming of Human Mesenchymal Stem Cells with Hepatocyte Growth Factor-Engineered Mesenchymal Stem Cells Promotes Therapeutic Potential for Cardiac Repair. Sci. Adv. 6 (13), eaay6994. doi:10.1126/sciadv.aay6994
Park, J. Y., Choi, J.-C., Shim, J.-H., Lee, J.-S., Park, H., Kim, S. W., et al. (2014). A Comparative Study on Collagen Type I and Hyaluronic Acid Dependent Cell Behavior for Osteochondral Tissue Bioprinting. Biofabrication 6 (3), 035004. doi:10.1088/1758-5082/6/3/035004
Pati, F., Jang, J., Ha, D.-H., Won Kim, S., Rhie, J.-W., Shim, J.-H., et al. (2014). Printing Three-Dimensional Tissue Analogues with Decellularized Extracellular Matrix Bioink. Nat. Commun. 5 (1), 3935. doi:10.1038/ncomms4935
Pereira, R. F., and Bártolo, P. J. (2015). 3D Bioprinting of Photocrosslinkable Hydrogel Constructs. J. Appl. Polym. Sci. 132 (48). doi:10.1002/app.42458
Pinto, A. R., Ilinykh, A., Ivey, M. J., Kuwabara, J. T., D’Antoni, M. L., Debuque, R., et al. (2016). Revisiting Cardiac Cellular Composition. Circ. Res. 118 (3), 400–409. doi:10.1161/circresaha.115.307778
Pountos, I., Tellisi, N., and Ashammakhi, N. (2019). “Potential Clinical Applications of Three-Dimensional Bioprinting,” in 3D Bioprinting in Medicine: Technologies, Bioinks, and Applications. Editor M. Guvendiren, 101–125. doi:10.1007/978-3-030-23906-0_3
Prasad, A. (2021). State of Art Review on Bioabsorbable Polymeric Scaffolds for Bone Tissue Engineering. Mater. Today Proc. 44, 1391–1400. doi:10.1016/j.matpr.2020.11.622
Qasim, M., Haq, F., Kang, M. H., and Kim, J. H. (2019). 3D Printing Approaches for Cardiac Tissue Engineering and Role of Immune Modulation in Tissue Regeneration. Ijn Vol. 14, 1311–1333. doi:10.2147/ijn.s189587
Radulescu, D., Dhar, S., Young, C. M., Taylor, D. W., Trost, H.-J., Hayes, D. J., et al. (2007). Tissue Engineering Scaffolds for Nerve Regeneration Manufactured by Ink-Jet Technology. Mater. Sci. Eng. C 27 (3), 534–539. doi:10.1016/j.msec.2006.05.050
Raman, R., Bhaduri, B., Mir, M., Shkumatov, A., Lee, M. K., Popescu, G., et al. (2016). High-resolution Projection Microstereolithography for Patterning of Neovasculature. Adv. Healthc. Mater. 5 (5), 610–619. doi:10.1002/adhm.201500721
Rodday, B., Hirschhaeuser, F., Walenta, S., and Mueller-Klieser, W. (2011). Semiautomatic Growth Analysis of Multicellular Tumor Spheroids. J. Biomol. Screen. 16 (9), 1119–1124. doi:10.1177/1087057111419501
Ronaldson-Bouchard, K., Ma, S. P., Yeager, K., Chen, T., Song, L., Sirabella, D., et al. (2018). Advanced Maturation of Human Cardiac Tissue Grown from Pluripotent Stem Cells. Nature 556 (7700), 239–243. doi:10.1038/s41586-018-0016-3
Rosines, E., Schmidt, H. J., and Nigam, S. K. (2007). The Effect of Hyaluronic Acid Size and Concentration on Branching Morphogenesis and Tubule Differentiation in Developing Kidney Culture Systems: Potential Applications to Engineering of Renal Tissues. Biomaterials 28 (32), 4806–4817. doi:10.1016/j.biomaterials.2007.07.034
Sahai, N., and Gogoi, M. (2019). “Techniques and Software Used in 3D Printing for Nanomedicine Applications,” in 3D Printing Technology in Nanomedicine. Editors N. Ahmad, P. Gopinath, and R. Dutta, 23–41. doi:10.1016/b978-0-12-815890-6.00002-5
Sarkhosh-Inanlou, R., Shafiei-Irannejad, V., Azizi, S., Jouyban, A., Ezzati-Nazhad Dolatabadi, J., Mobed, A., et al. (2021). Applications of Scaffold-Based Advanced Materials in Biomedical Sensing. Trac Trends Anal. Chem. 143, 116342. doi:10.1016/j.trac.2021.116342
Seif-Naraghi, S. B., Singelyn, J. M., Salvatore, M. A., Osborn, K. G., Wang, J. J., Sampat, U., et al. (2013). Safety and Efficacy of an Injectable Extracellular Matrix Hydrogel for Treating Myocardial Infarction. Sci. Transl Med. 5 (173), 173ra25. doi:10.1126/scitranslmed.3005503
Sharma, P., Wang, X., Ming, C. L. C., Vettori, L., Figtree, G., Boyle, A., et al. (2021). Considerations for the Bioengineering of Advanced Cardiac In Vitro Models of Myocardial Infarction. Small (Weinheim an Der Bergstrasse, Germany) 17 (15), e2003765. doi:10.1002/smll.202170067
Shi, W., Sun, M., Hu, X., Ren, B., Cheng, J., Li, C., et al. (2017). Structurally and Functionally Optimized Silk-Fibroin-Gelatin Scaffold Using 3D Printing to Repair Cartilage Injury In Vitro and In Vivo. Adv. Mater. 29 (29), 1701089. doi:10.1002/adma.201701089
Skardal, A., Mack, D., Kapetanovic, E., Atala, A., Jackson, J. D., Yoo, J., et al. (2012). Bioprinted Amniotic Fluid-Derived Stem Cells Accelerate Healing of Large Skin Wounds. STEM CELLS Translational Med. 1 (11), 792–802. doi:10.5966/sctm.2012-0088
Smith, C. M., Christian, J. J., Warren, W. L., and Williams, S. K. (2007). Characterizing Environmental Factors that Impact the Viability of Tissue-Engineered Constructs Fabricated by a Direct-Write Bioassembly Tool. Tissue Eng. 13 (2), 373–383. doi:10.1089/ten.2006.0101
Spang, M. T., and Christman, K. L. (2018). Extracellular Matrix Hydrogel Therapies: In Vivo Applications and Development. Acta Biomater. 68, 1–14. doi:10.1016/j.actbio.2017.12.019
Spiller, K. L., Maher, S. A., and Lowman, A. M. (2011). Hydrogels for the Repair of Articular Cartilage Defects. Tissue Eng. Part B: Rev. 17 (4), 281–299. doi:10.1089/ten.teb.2011.0077
Su, J., Huang, K., Daniele, M. A., Hensley, M. T., Young, A. T., Tang, J., et al. (2018). Cardiac Stem Cell Patch Integrated with Microengineered Blood Vessels Promotes Cardiomyocyte Proliferation and Neovascularization after Acute Myocardial Infarction. ACS Appl. Mater. Interfaces 10, 33088–33096. doi:10.1021/acsami.8b13571
Su, T., Huang, K., Ma, H., Liang, H., Dinh, P.-U., Chen, J., et al. (2019). Platelet-Inspired Nanocells for Targeted Heart Repair After Ischemia/Reperfusion Injury. Adv. Funct. Mater. 29, 1803567. doi:10.1002/adfm.201803567
Sun, J., Wang, J., Cheng, W., Zhang, J., Li, X., Zhang, S., et al. (2012). Chitosan Functionalized Ionic Liquid as a Recyclable Biopolymer-Supported Catalyst for Cycloaddition of CO2. Green. Chem. 14 (3), 654–660. doi:10.1039/c2gc16335g
Sun, Z. (2020). Clinical Applications of Patient-specific 3D Printed Models in Cardiovascular Disease: Current Status and Future Directions. Biomolecules 10 (11), 1577. doi:10.3390/biom10111577
Tan, Y., Richards, D. J., Trusk, T. C., Visconti, R. P., Yost, M. J., Kindy, M. S., et al. (2014). 3D Printing Facilitated Scaffold-free Tissue Unit Fabrication. Biofabrication 6 (2), 024111. doi:10.1088/1758-5082/6/2/024111
Tang, J.-N., Cores, J., Huang, K., Cui, X.-L., Luo, L., Zhang, J.-Y., et al. (2018). Concise Review: Is Cardiac Cell Therapy Dead? Embarrassing Trial Outcomes and New Directions for the Future. Stem Cell Translational Med. 7 (4), 354–359. doi:10.1002/sctm.17-0196
Tang, J., Su, T., Huang, K., Dinh, P.-U., Wang, Z., Vandergriff, A., et al. (2018). Targeted Repair of Heart Injury by Stem Cells Fused with Platelet Nanovesicles. Nat. Biomed. Eng. 2 (1), 17–26. doi:10.1038/s41551-017-0182-x
Tariverdian, T., Sefat, F., Gelinsky, M., and Mozafari, M. (2019). “Scaffold for Bone Tissue Engineering,” in Handbook of Tissue Engineering Scaffolds: Volume One. Editors M. Mozafari, F. Sefat, and A. Atala, 189–209. doi:10.1016/b978-0-08-102563-5.00010-1
Tiburcy, M., Hudson, J. E., Balfanz, P., Schlick, S., Meyer, T., Chang Liao, M.-L., et al. (2017). Defined Engineered Human Myocardium with Advanced Maturation for Applications in Heart Failure Modeling and Repair. Circulation 135 (19), 1832–1847. doi:10.1161/circulationaha.116.024145
Tomov, M. L., Gil, C. J., Cetnar, A., Theus, A. S., Lima, B. J., Nish, J. E., et al. (2019). Engineering Functional Cardiac Tissues for Regenerative Medicine Applications. Curr. Cardiol. Rep. 21 (9), 105. doi:10.1007/s11886-019-1178-9
Traverse, J. H., Henry, T. D., Dib, N., Patel, A. N., Pepine, C., Schaer, G. L., et al. (2019). First-in-man Study of a Cardiac Extracellular Matrix Hydrogel in Early and Late Myocardial Infarction Patients. JACC: Basic Translational Sci. 4 (6), 659–669. doi:10.1016/j.jacbts.2019.07.012
Tsukamoto, Y., Akagi, T., and Akashi, M. (2020). Vascularized Cardiac Tissue Construction with Orientation by Layer-By-Layer Method and 3D Printer. Sci. Rep. 10 (1), 5484. doi:10.1038/s41598-020-59371-y
Usprech, J., Romero, D. A., Amon, C. H., and Simmons, C. A. (2017). Combinatorial Screening of 3D Biomaterial Properties that Promote Myofibrogenesis for Mesenchymal Stromal Cell-Based Heart Valve Tissue Engineering. Acta Biomater. 58, 34–43. doi:10.1016/j.actbio.2017.05.044
Veldhuizen, J., Migrino, R. Q., and Nikkhah, M. (2019). Three-dimensional Microengineered Models of Human Cardiac Diseases. J. Biol. Eng. 13 (1), 29. doi:10.1186/s13036-019-0155-6
Ventura, R. D. (2021). An Overview of Laser-Assisted Bioprinting (LAB) in Tissue Engineering Applications. Med. Lasers 10 (2), 76–81. doi:10.25289/ml.2021.10.2.76
Vijayavenkataraman, S., Yan, W.-C., Lu, W. F., Wang, C.-H., and Fuh, J. Y. H. (2018). 3D Bioprinting of Tissues and Organs for Regenerative Medicine. Adv. Drug Deliv. Rev. 132, 296–332. doi:10.1016/j.addr.2018.07.004
Wang, F., and Guan, J. (2010). Cellular Cardiomyoplasty and Cardiac Tissue Engineering for Myocardial Therapy. Adv. Drug Deliv. Rev. 62 (7), 784–797. doi:10.1016/j.addr.2010.03.001
Wang, Q., Song, Y., Chen, J., Li, Q., Gao, J., Tan, H., et al. (2021). Direct In Vivo Reprogramming with Non-viral Sequential Targeting Nanoparticles Promotes Cardiac Regeneration. Biomaterials 276, 121028. doi:10.1016/j.biomaterials.2021.121028
Wang, X.-F., Song, Y., Liu, Y.-S., Sun, Y.-c., Wang, Y.-g., Wang, Y., et al. (2016a). Osteogenic Differentiation of Three-Dimensional Bioprinted Constructs Consisting of Human Adipose-Derived Stem Cells In Vitro and In Vivo. PLOS ONE 11 (6), e0157214. doi:10.1371/journal.pone.0157214
Wang, X., Hu, S., Li, J., Zhu, D., Wang, Z., Cores, J., et al. (2021). Extruded Mesenchymal Stem Cell Nanovesicles Are Equally Potent to Natural Extracellular Vesicles in Cardiac Repair. ACS Appl. Mater. Inter. 13 (47), 55767–55779. doi:10.1021/acsami.1c08044
Wang, Z., Jin, X., Dai, R., Holzman, J. F. J., and Kim, K. (2016b). An Ultrafast Hydrogel Photocrosslinking Method for Direct Laser Bioprinting. RSC Adv. 6 (25), 21099–21104. doi:10.1039/c5ra24910d
Wang, Z., Lee, S. J., Cheng, H.-J., Yoo, J. J., and Atala, A. (2018). 3D Bioprinted Functional and Contractile Cardiac Tissue Constructs. Acta Biomater. 70, 48–56. doi:10.1016/j.actbio.2018.02.007
Wang, Z., Wang, L., Li, T., Liu, S., Guo, B., Huang, W., et al. (2021). 3D Bioprinting in Cardiac Tissue Engineering. Theranostics 11 (16), 7948–7969. doi:10.7150/thno.61621
Williams, C., Quinn, K. P., Georgakoudi, I., and Black, L. D. (2014). Young Developmental Age Cardiac Extracellular Matrix Promotes the Expansion of Neonatal Cardiomyocytes In Vitro. Acta Biomater. 10 (1), 194–204. doi:10.1016/j.actbio.2013.08.037
Xu, T., Baicu, C., Aho, M., Zile, M., and Boland, T. (2009). Fabrication and Characterization of Bio-Engineered Cardiac Pseudo Tissues. Biofabrication 1 (3), 035001. doi:10.1088/1758-5082/1/3/035001
Xu, T., Kincaid, H., Atala, A., and Yoo, J. J. (2008). High-throughput Production of Single-Cell Microparticles Using an Inkjet Printing Technology. J. Manufacturing Sci. Eng. 130 (2). doi:10.1115/1.2903064
Yin, J., Yan, M., Wang, Y., Fu, J., and Suo, H. (2018). 3D Bioprinting of Low-Concentration Cell-Laden Gelatin Methacrylate (GelMA) Bioinks with a Two-step Cross-Linking Strategy. ACS Appl. Mater. Inter. 10 (8), 6849–6857. doi:10.1021/acsami.7b16059
Yu, C., Miller, K. L., Schimelman, J., Wang, P., Zhu, W., Ma, X., et al. (2020). A Sequential 3D Bioprinting and Orthogonal Bioconjugation Approach for Precision Tissue Engineering. Biomaterials 258, 120294. doi:10.1016/j.biomaterials.2020.120294
Zhang, A. P., Qu, X., Soman, P., Hribar, K. C., Lee, J. W., Chen, S., et al. (2012). Rapid Fabrication of Complex 3D Extracellular Microenvironments by Dynamic Optical Projection Stereolithography. Adv. Mater. 24 (31), 4266–4270. doi:10.1002/adma.201202024
Zhang, J., Hu, Q., Wang, S., Tao, J., and Gou, M. (2019). Digital Light Processing Based Three-Dimensional Printing for Medical Applications. Int. J. Bioprint 6 (1), 242. doi:10.18063/ijb.v6i1.242
Zhang, Y. S., Arneri, A., Bersini, S., Shin, S.-R., Zhu, K., Goli-Malekabadi, Z., et al. (2016). Bioprinting 3D Microfibrous Scaffolds for Engineering Endothelialized Myocardium and Heart-On-A-Chip. Biomaterials 110, 45–59. doi:10.1016/j.biomaterials.2016.09.003
Zhu, D., and Cheng, K. (2021). Cardiac Cell Therapy for Heart Repair: Should the Cells Be Left Out. Cells 10 (3), 641. doi:10.3390/cells10030641
Zhu, K., Shin, S. R., van Kempen, T., Li, Y. C., Ponraj, V., Nasajpour, A., et al. (2017). Gold Nanocomposite Bioink for Printing 3D Cardiac Constructs. Adv. Funct. Mater. 27 (12), 1605352. doi:10.1002/adfm.201605352
Zhu, W., Qu, X., Zhu, J., Ma, X., Patel, S., Liu, J., et al. (2017). Direct 3D Bioprinting of Prevascularized Tissue Constructs with Complex Microarchitecture. Biomaterials 124, 106–115. doi:10.1016/j.biomaterials.2017.01.042
Keywords: 3D bioprinting, scaffolds-based 3D bioprinting, 3D cardiac patch, cardiac regeneration, cardiac repair
Citation: Chingale M, Cheng K and Huang K (2022) 3D Bioprinting Technology – One Step Closer Towards Cardiac Tissue Regeneration. Front. Mater. 8:804134. doi: 10.3389/fmats.2021.804134
Received: 10 November 2021; Accepted: 31 December 2021;
Published: 02 February 2022.
Edited by:
Masoud Mozafari, University of Toronto, CanadaReviewed by:
Guohao Dai, Northeastern University, United StatesManashjit Gogoi, North Eastern Hill University, India
Copyright © 2022 Chingale, Cheng and Huang. This is an open-access article distributed under the terms of the Creative Commons Attribution License (CC BY). The use, distribution or reproduction in other forums is permitted, provided the original author(s) and the copyright owner(s) are credited and that the original publication in this journal is cited, in accordance with accepted academic practice. No use, distribution or reproduction is permitted which does not comply with these terms.
*Correspondence: Ke Huang, a2h1YW5nN0BuY3N1LmVkdQ==; Ke Cheng, a2VfY2hlbmdAbmNzdS5lZHU=