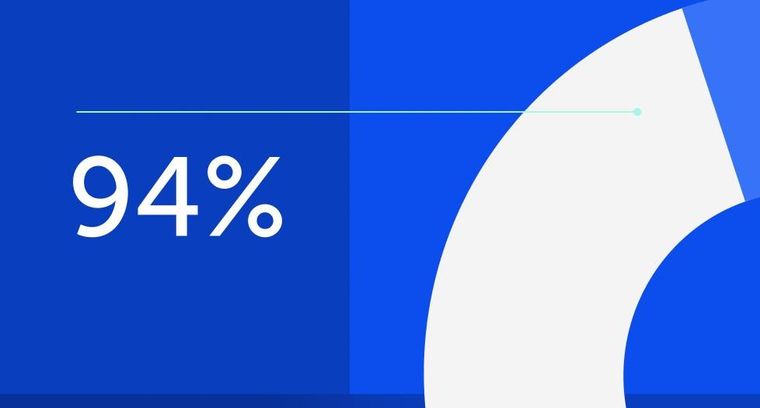
94% of researchers rate our articles as excellent or good
Learn more about the work of our research integrity team to safeguard the quality of each article we publish.
Find out more
REVIEW article
Front. Mater., 05 January 2022
Sec. Structural Materials
Volume 8 - 2021 | https://doi.org/10.3389/fmats.2021.796396
This article is part of the Research TopicHorizons in MaterialsView all 10 articles
Effective mitigation of alkali-silica reaction (ASR) is critical for producing durable concrete. The use of alumina-rich supplementary cementitious materials (SCMs) and chemical admixtures such as lithium salts to prevent expansion caused by ASR was first reported 70 years ago, shortly after the discovery of ASR in 1940s. Despite numerous investigations, the understanding of the mechanisms of Al and Li for mitigating ASR remain partially inexplicit in the case of Al, and hardly understood in the case of Li. This paper reviews the available information on the effect of Al and Li on ASR expansion, the influencing factors, possible mechanisms and limitations. The role of Al in mitigating ASR is likely related to the reduction of dissolution rate of reactive silica. Moreover, the presence of Al may alter the structure of crystalline ASR products to zeolite or its precursor, but such effect seems to be not that significant at ambient conditions due to the slow kinetics of zeolite formation. Several mechanisms for the lithium salts in mitigating ASR have been proposed, but most of them are not conclusive primarily due to the lack of knowledge about the formed reaction products. Combination of Al-rich SCMs and lithium salts may be used as an economic solution for ASR mitigation, although systematic studies are necessary prior to the applications.
Alkali-silica reaction (ASR) is an important durability issue world-wide, which causes significant expansion and deterioration of various concrete infrastructures including dams, pavements, bridges, walls, barriers, and nuclear/power plant structures (Rajabipour et al., 2015; Sims and Poole, 2017). The reaction starts by the dissolution of reactive silica at high pH in presence of alkalis from concrete pore solution followed by formation of alkali-silica gels. With further uptake of Ca, gelation and/or crystallization occur(s) leading to formation of amorphous and/or crystalline ASR products. The nature of the ASR products varies significantly depending on the composition of the pore solution, stage of ASR, and temperature (Shi et al., 2019; Shi et al., 2020a; Shi et al., 2020b). It remains unclear at which steps and by which mechanisms ASR expansion is generated (Shi et al., 2020b). Generally, the expansion due to ASR has been related to swelling of the product in the presence of water as more damage has been observed at high relative humidity (Olafsson, 1986; Kurihara and Katawaki, 1989; Stark, 1991). However, recent work based on synthetic ASR products (Shi et al., 2019) and field ASR products (Leemann et al., 2020) demonstrated that neither amorphous nor crystalline ASR products based on Na, K, Ca and silica swell upon uptake of water. The uptake of water by these ASR products was lower than that of C-S-H, suggesting that not swelling but rather alternative mechanisms are responsible for ASR expansion, indicating an urgent need for further investigations.
To stop ASR in existing structures is challenging and costly and can involve water proofing by coatings and/or slot cutting to release stresses due to concrete expansion of the affected structure. However, in both cases ASR can continue as there can be sufficient water for the ASR even in coated concrete structures and the concrete will continue expanding after slot cutting. In new structures, the use of non-reactive aggregates is a relatively cheap and efficient solution to avoid ASR. However, local aggregates are often used due to environmental and economic reasons, and in remote locations such as for dams may be the only choice.
Effective mitigation of ASR is critical for safe use of reactive aggregates for producing durable concrete. The use of supplementary cementitious materials (SCMs) and chemical admixtures such as lithium salts to prevent expansion caused by ASR was first reported 70 years ago shortly after the discovery of ASR in 1940s. For freshly produced concrete, use of appropriate cement blends such as Portland cement blended with SCMs to lower the pH and alkali concentration in the concrete pore solution is an efficient solution to minimize damage risk of ASR. Lower pH values decreases the dissolution kinetics of silica-containing minerals within the aggregate (Iler, 1979; Bagheri et al., 2021) and lower pH values together with lower alkali concentrations decrease also the risk of the formation of ASR products (Thomas, 2011; Shi and Lothenbach, 2020). Moreover, alumina-rich SCMs such as fly ash, blast furnace slags or metakaolin are found to be more effective than silica-rich SCMs such as silica fume (Aquino et al., 2001; Duchesne and Bérubé, 2001; Kandasamy and Shehata, 2014).
The role of Al in mitigating ASR has been at least partially related to a slowing down of the dissolution of reactive silica (Chappex and Scrivener, 2012b; Bagheri et al., 2022). The presence of Al may also alter the structure of crystalline ASR products to zeolite or its precursor at 80°C, while their formation kinetics at ambient temperatures seem to be too slow to have a relevant effect (Hünger, 2007; Shi et al., 2018; Shi et al., 2021). Thus despite of numerous investigations, the understanding of the mechanisms of Al mitigating ASR remains at least partially inexplicit (Hünger, 2007; Chappex and Scrivener, 2012a).
In contrast to SCMs, which can only be introduced during concrete production, lithium salt solutions can be applied to an expanding concrete structure in addition to being used as an admixture during production. So far, eleven different types of lithium salts have been studied to mitigate ASR including LiCl, Li2CO3, LiF, Li2SiO3, LiNO3, Li2SO4, LiOH, LiNO2, LiBr, LiOH·H2O, LiH2PO4 (McCoy and Caldwell, 1951; Qinghan et al., 1995; Lumley, 1997; Demir and Arslan, 2013). Some difficulties of using these lithium salts are encountered because the dosage of different types of lithium salts varies significantly depending on several factors such as the type of lithium salts, the mineralogy of reactive aggregates, and the alkali content of the concrete. Moreover, the mechanisms of different types of lithium salts are also complicated, since they may result in formation of different types of Li-bearing ASR products and lithium silicates.
Understanding the precise mechanisms of both Al and Li for mitigating ASR is important for optimizing the use of these materials and for ensuring their long-term efficiency in mitigating ASR. This has been a challenge for many decades, primarily due to the difficulties to characterize the ASR products formed in small amounts and volume with conventional laboratory techniques. Moreover, Li-containing reaction products are difficult to be characterized in particular for their chemical compositions. Recent successful synthesis of ASR products at different temperatures resembling to those formed in concrete aggregates under accelerated and field conditions, provide a new opportunity to re-investigate the mechanisms of Al and Li in mitigating ASR (Shi et al., 2019; Shi et al., 2020a; Shi et al., 2021). This review article summaries and critically discusses the available investigations on the effect of Al and Li on ASR expansion, the influencing factors, possible mechanisms and limitations.
Both Al2O3 and SiO2 from Al-rich SCMs can contribute to the reduction of ASR expansion as addressed in the introduction. In order to differentiate between the influence of SiO2 and Al2O3 in SCMs on ASR expansion, few studies (Szeles et al., 2017; Zhou et al., 2019) have isolated the role of Al on ASR expansion by partially replacing Portland cement with pure Al(OH)3 or γ-Al2O3. As demonstrated in Figure 1, replacing 20% OPC with Al(OH)3 significantly reduced the ASR expansion below the threshold level 0.04% at 38°C as specified in ASTM C1293-08b (Szeles et al., 2017). The ASR expansion of the concrete prism was completely suppressed by increasing the replacement level up to 30% over 600 days of exposure. In another study (Zhou et al., 2019) the control concrete using different aggregate composed of 10% crushed fused silica (2.36–4.75 mm) and 90% standard sand (0.15–2.36 mm) showed a comparable ASR expansion to the expansion of the control concrete in Figure 1. However, incorporation of only 10% γ-Al2O3 could already reduce the ASR expansion to the threshold level following either ASTM C227 (at 38°C) or ASTM C1260 (at 80°C). The SEM images from both of the studies demonstrated that the aggregates remain intact for the concrete containing Al in contrast to the reference concrete with significant damage and ASR product formation (Szeles et al., 2017; Zhou et al., 2019). These studies confirmed the role of sole Al in mitigating ASR as previously suggested by other studies based on comparison of the ASR mitigation efficacy between Al-rich SCMs and silica fume (Aquino et al., 2001; Ramlochan et al., 2004). Therefore, it is of significance to elucidate the mechanisms of Al in mitigating ASR in order to predict its long-term efficiency.
FIGURE 1. ASR expansion of concrete prisms containing highly reactive Spratt coarse aggregates and binder materials of control cement (OPC) partially replaced with Al(OH)3. Data from (Szeles et al., 2017).
Several mechanisms of Al in mitigating ASR were proposed in the literature, which include increased alkali fixation, alteration of the ASR products, and reduction of the silica dissolution rates, as discussed in the following.
It was conjectured that the presence of Al in the SCMs possibly enhance the removal of alkalis from the concrete pore solution resulting a reduction of its pH, which would be beneficial for ASR mitigation. Hong and Glasser (Hong and Glasser, 2002) reported that incorporation of Al into C-S-H leads to formation of C-A-S-H phase, which markedly increases its alkali binding capacity. They suggested that this partially contributes to the potential of Al-rich SCMs in reducing pore solution alkalinity and subsequently ASR expansion. Later, Sun et al. (2006) showed significant increases in basal spacings when Al was incorporated into C-S-H phase. They explained that alumina was incorporated into C-S-H and present in the interlayer and that the substitution of Si by Al provides a negative site that must be charge balanced by a net positive charge (e.g., Na+, K+) leading to alkali binding. Thomas (Thomas, 2011) also stated that there is evidence that the alumina content of the SCM also affects its alkali-binding capacity as its silica content.
On contrary, an earlier study from Diamond (Diamond, 1981) showed that class F fly ash reduced hydroxyl and alkali ion concentration of the pore solution by a factor equivalent to its OPC replacement level of 30%, indicating a simple dilution effect. This observation is supported by the studies of Chappex and Scrivener (Chappex and Scrivener, 2012a). In their study, they compared an OPC-metakaolin paste with a paste containing silica fume and an inert filler to maintain an equivalent silica fraction and could thus isolate the effect of alumina. They found that the pore solution alkalinity was reduced with increasing the replacement level of OPC. However, such reduction of pore solution alkalinity was a mere dilution effect, and the alumina did not actively consume hydroxyl ions or alkalis.
Most of the aforementioned studies focused on cement mixtures containing Al-rich SCMs, where the joint effects of alumina and silica in SCMs could not be directly separated. To isolate the role of Al in mitigating ASR, Szeles et al. (2017) replaced OPC with 20% Al(OH)3 and measured the pore solution pH of the cement paste mixtures. They observed that the pH of the Al(OH)3 mixture was initially similar to the 80% of the control mixture, indicating a pure dilution effect. At later ages up to 1 year, slight reduction of pH (0.13 pH unit) was observed beyond the dilution effect, the pH reduction was not significant enough to be the sole mechanism for Al to mitigate ASR. A drop of pH from 12.4 to 11.9 was also recently captured by Shi et al. (2021) during the synthesis of ASR products at 40°C containing SiO2, CaO, and KOH, where Al(OH)3 precipitated as a separate phase. However, the pH increased at 80°C from 12.1 to 12.8, where the formation of an alumino-silicate phase prevented the formation of ASR products. The influence of Al on formation and structure of ASR products will be discussed in detail in the next section.
The above seeming contradictions has been clarified based on synthetic C-S-H (L’Hôpital et al., 2016), where the uptake of K in C-S-H and C-A-S-H at different Ca/Si ratios were compared as shown in Figure 2. The results show that the K/Si ratio in C-S-H is not significantly increased in the presence of aluminum. The scattered results reported in the aforementioned studies was attributed to the experimental error associated with the measurement of alkali concentrations, the synthesis protocol, and equilibration time (L’Hôpital et al., 2016). Therefore, the mechanism of significantly increased alkali fixation with subsequent pH reduction can be excluded.
FIGURE 2. Alkali uptake in C-S-H and C-A-S-H (Al/Si = 0.05, Ca/Si = 0.8) in equilibrium with a solution containing 0.5 mol/L [KOH] equilibrated for 182 days. Reproduced from (L’Hôpital et al., 2016).
For a long period of time, it was debated whether dissolved aluminum ions affect the formation and structure of ASR products or not. Many studies reported the incorporation of Al in ASR products based on scanning electron microscope with energy-dispersive X-ray spectroscopy (SEM/EDS) analysis (Fernandes et al., 2007; Fernandes, 2009; Šachlová et al., 2010; Hagelia and Fernandes, 2012; Leemann and Lura, 2013; Shafaatian et al., 2013; Guo et al., 2018), while many others reported no Al or hardly detectable amounts in ASR products (Aquino et al., 2001; Leemann and Lothenbach, 2008; Katayama, 2012a; Katayama, 2012b; Leemann, 2017). It should be noted that the observation of Al in ASR products could be caused either by the close intermixing with Al-containing phases (hydrated cements or Al-containing minerals within the aggregates) or by cross-contaminations during sample preparation for SEM/EDS analysis. The absence of a significant amount of Al in ASR products was recently confirmed (Shi et al., 2021) based on laboratory synthetized ASR samples, where Al precipitated as a separate phase [gibbsite, Al(OH)3] at 40°C, i.e., under temperatures relevant for field conditions, while the structure and composition of ASR products was not affected by aluminum.
At high temperature (80°C) and high alkali concentration (0.5–1 mol/L NaOH or KOH), however, the formation of zeolites or zeolitic precursors (alkali alumino-silicate phase) was observed in concrete (Hünger, 2007; Shi et al., 2018) as well as in synthetic samples (Shi et al., 2021). Hünger (Hünger, 2007) found an inverse relation between the silica releasing rate of aggregate and the amount of zeolite formed at the same temperature and suggested that the formation of alkali alumino-silicate and zeolite would reduce the concentration of so-called “free” silica available for ASR and thus inhibit ASR. Also laboratory experiments showed the suppression of the formation of ASR products in the presence of sufficient Al. While the formation of alkali alumino-silicate or zeolite could in fact lower the potential to form ASR products at high temperatures (80°C or above), at ambient temperatures the formation of alkali alumino-silicate and zeolite is usually slow (decades) (Sand et al., 1987; Lothenbach et al., 2017). Thus, the formation of alkali alumino-silicate and zeolite is under most conditions too slow to be relevant in suppressing ASR formation in field concretes.
A possibility to prevent ASR is to suppress or strongly slow down the dissolution of SiO2 within the aggregate. Aluminum can drastically reduce silica dissolution rates as aluminum sorbs on the silica surface, which passivates the active silica sites and slows down dissolution (Iler, 1973; Bickmore et al., 2006; Nicoleau et al., 2014). Relatively low concentrations of Al (1–5 mM) can reduce the dissolution rate of silica by as much as 90%. However, the sorption of Al(OH)4- on the surface of silica and the slowing down of silica dissolution silica is more distinct at intermediate pH values (<12), while at pH 13 and above the sorption of Al(OH)4- on silica becomes weak resulting in only feeble suppression of the dissolution rate (Yokoyama et al., 1988; Chappex and Scrivener, 2012b; Nicoleau et al., 2014) as illustrated in Figure 3 (Bagheri et al., 2022). A comparison with the Al concentration in the pore solution of Portland cement and blended cements, show that blending of Portland cement with fly ash or metakaolin could in fact increase the Al concentration to 1 mM and above, i.e., to Al concentrations efficient in slowing down silica dissolution (see Figure 3). Recent study from Zhou et al. (2022) showed that the Al concentration could potentially reach about 80 mM after dissolution of 2 g metakaolin (ca. 1.0 g Al2O3) for 100 days in 75 ml simulated pore solution [0.6M NaOH, Ca(OH)2 saturated], although in a concrete pore solution the concentrations might be significantly lower due to the formation of AFt and AFm phases. In fact, drastic reduction in damage to reactive siliceous aggregates is observed in metakaolin, slag or fly ash blended cements (Chappex and Scrivener, 2012a; Tapas et al., 2021), although this reduction might also relate to the decrease of pH in the pore solution due to the reaction of metakaolin, slag or fly ash.
FIGURE 3. Effect Al concentration on the Si release rate [mol/(m2·s)] of amorphous silica in 100 and 400 mM KOH at 40°C, adapted from (Bagheri et al., 2022). The vertical lines indicate Al concentrations typically observed in the pore solution of Portland cements (≈0.1 mM), in blends with blast furnace slags (≈0.1 mM), with fly ash (≈1 mM) and with metakaolin (≈3 mM) (Deschner et al., 2012; Vollpracht et al., 2016; Avet and Scrivener, 2018).
As Al slows down, but does not prevent silica dissolution, one could expect a slower buildup of ASR with time. However, as demonstrated in Figure 1, the presence of Al(OH)3 or Al-rich SCMs seems to prevent ASR completely up to 700 days (Ramlochan et al., 2004; Szeles et al., 2017), indicating that other effects in addition to a slow down of dissolution could play an important role.
Lithium salts to inhibit ASR were first used 70 years ago by McCoy and Caldwell (McCoy and Caldwell, 1951). McCoy and Caldwell investigated the potential of over 100 different compounds to prevent ASR of mortars containing Pyrex glass as reactive aggregate. All the studied lithium salts containing LiCl, Li2CO3, LiF, Li2SiO3, LiNO3, and Li2SO4 were found to be more effective than the other inorganic salts and the other compounds such as acids, oils, organic chemicals, proteins, and proprietary admixtures. Since even the almost insoluble LiF seemed to be beneficial, they also suggested that the nature of the accompanying anion of the lithium salts was not particularly important. Later, Stark (Stark, 1992) confirmed the effectiveness of LiF and Li2CO3 in inhibiting ASR expansion, and reported that also LiOH seemed to be effective. Several other studies further reported that also LiBr (Qinghan et al., 1995; Bian et al., 1996; Demir and Arslan, 2013; Demir et al., 2018) and LiH2PO4 (Bian et al., 1996) are effective in reducing ASR expansion. Bian et al. (Bian et al., 1996) reported LiCl, LiBr, LiNO2, LiNO3, Li2SO4, and LiH2PO4 actually produced similar suppressive effects on ASR expansion, whereas Li2CO3, LiOH, and LiF only exhibited certain effects at a [Li]/[Na + K] molar ratio of 0.8.
Among all types of lithium salts, LiNO3 emerged as the preferred lithium compound for inhibiting ASR (Diamond, 1999), due to its neutrality, high solubility and good compatibility with other admixtures (Wang et al., 1996). It was also reported that LiNO3 has a benign effect on the concrete properties of strength, electrical resistance, drying shrinkage, and resistance to freezing and thawing, whereas LiOH retard the strength development (Lane and Board, 2002). In Japan the use of lithium nitrite (LiNO2) as an ASR inhibitor has been widely studied (Sakaguchi et al., 1989; Saito et al., 1992; Kobayashi and Takagi, 2020) due to its positive effect on preventing rebar corrosion as well as due to its high solubility.
Although lithium salts are used to inhibit ASR expansion, Stark et al. (Stark et al., 1993) also reported that insufficient dosages of lithium compounds may even increase the ASR expansion in some aggregates, known as “pessimum effect”. The dosage of lithium salts to be added for inhibiting ASR is commonly expressed as the molar ratio Li/(Na + K). Only at relative high Li/(Na + K) ratio can completely suppress the ASR expansion. A molar ratio of Li/(Na + K) of 0.74 seems to be required to suppress ASR expansion as found in many studies (McCoy and Caldwell, 1951; Bérubé et al., 2004; Leemann et al., 2014), while others also reported that the optimum Li/Na molar ratio is in the range of 0.9–1.2 if LiOH is added (Sakaguchi et al., 1989). In fact, the exact value of the Li/(K + Na) ratio is not fixed but varies depending on the following factors:
a) Type of lithium salts: Thomas et al. (2000) observed that in the case of for LiNO3 a [Li]/[Na + K] molar ratio of 0.74 sufficiently suppressed ASR-induced expansion with most aggregates, whereas higher ratio of [Li]/[Na + K] of 0.85 was required in the case of LiOH·H2O in concrete prisms containing a crushed siltstone aggregate. Collins et al. (2004b) reported that the required minimum threshold [Li2O]/[Na2Oe] ratio varies from 0.5 to 1.0 for each additive examined (LiOH, LiCl, or LiNO3), with respect to the particular reactive aggregate used in their work.
b) Equivalent dosage relates to alkali content: Several studies reported that the minimum Li/(K + Na) ratio required to substantially suppress ASR expansion depends also on the amount of alkali present in the cement. Mo et al. (2003) reported that if the cement contained less than 2.5% Na2O equivalent, a [Li/Na] molar ratio above 0.3 was sufficient to inhibit ASR in long-term by adding LiOH. However, when the cement contained more than 3% Na2O equivalent, a Li/Na ratio of at least 0.6 was needed (Mo et al., 2003). Qinghan et al. (1995) studied the suppressive effect of LiNO2 on mortars containing an andesite sand from Japan following an autoclave test procedure, they reported that the required Li/Na ratios were 0.1, 0.3 and 0.5 and 0.8 corresponding to Na2O equivalent levels of 0.5, 1.0, 1.5%, and 2%. Berra et al. (2003) found a linear relationship between the effective lithium dosage in terms of Li/[Na + K] and the difference between total alkali content and threshold alkali level of the aggregates (alkali reactivity level) as shown in Figure 4.
c) Types of reactive aggregates: Several early studies showed that the dosage of lithium depends also on the aggregates (Lane, 2000; Lane and Board, 2002; Collins et al., 2004b). Lane and coworkers (Lane, 2000; Lane and Board, 2002) studied the efficiency of both LiOH and a commercial LiNO3 solution in mitigating ASR for the concrete prisms containing Pyrex glass and some Virginia reactive aggregates composed of microcrystalline and strained quartz. They observed that [Li]/[Na + K] = 0.925 was required for the two aggregates, and also concluded that both lithium compounds were more effective for the highly reactive aggregates than for the less reactive aggregates. Similar observation is also reported in Drimalas et al. (2012) based on a study on the long-term exposed concrete blocks, using different aggregate types and various dosages of lithium-based salts. The concrete blocks exposed for up to 16 years showed a varying response to lithium based on aggregate types. Tremblay and co-workers (Tremblay et al., 2004a; Tremblay et al., 2007) systematically investigated 12 different aggregates and showed that 50% of the reactive aggregates responded well to the commonly used dosage (0.74 molar ratio). For three other aggregates tested, a higher dosage of LiNO3 ranging from 0.75 to 1.04 was required. For the remaining three aggregates, a 1.11 molar ratio was not sufficient to limit ASR below threshold 0.04% of the concrete prism test. Moreover, they also showed that the response of lithium could not be correlated to the aggregate reactivity level and mineralogy.
FIGURE 4. Relationship between the effective dosage of lithium nitrate (deff), the alkali content of concrete (Lac), and the threshold alkali level (TAL) of the aggregates. Data reproduced from (Berra et al., 2003).
Regardless of the above-mentioned factors, it is generally accepted that a dosage of Li/(Na + K) of >0.6 is required to inhibit ASR (Collins et al., 2004b; Leemann et al., 2014; Islam and Ghafoori, 2016) as illustrated in Figure 5 reproduced based on the data from literature (Qinghan et al., 1995; Thomas et al., 2000; Collins et al., 2004b; Mo et al., 2005; Folliard et al., 2006; Kobayashi and Takagi, 2020) and in Table 1.
FIGURE 5. Expansion measured over an extended time frame as it relates to the Li/Na ratio of the product for various lithium treatments (Qinghan et al., 1995; Thomas et al., 2000; Collins et al., 2004b; Mo et al., 2005; Folliard et al., 2006; Kobayashi amd Takagi, 2020).
TABLE 1. Summary of selected research findings related to the lithium dosage ([Li]/[Na + K] molar ratio) needed to suppress ASR expansion and their test conditions. The table is adapted based on the version published in (Folliard et al., 2006).
The proposed Li/(K + Na) molar ratios from most of the studies discussed above are based on freshly prepared mortars and concretes where lithium salts have been used as admixtures. In practice, lithium salts are often used afterwards to slow down expansion in ASR affected concrete. The determination of the optimum amount of lithium for concrete is thus not straightforward. The even distribution of lithium ions within the entire concrete plays a key role in effectively suppressing ASR expansion. Several methods have been suggested to apply lithium salts to ASR-affected concrete, e.g., sprinkling lithium solution on concrete surface (Zapała-Sławeta and Owsiak, 2018), using electrochemical method by applying voltage at 40 V (Souza et al., 2017), injecting lithium solution into concrete under vacuum (Thomas et al., 2007) or by pressure through drilled holes of a 10–30 mm diameter using a compressor (Kobayashi and Takagi, 2020), or by soaking ASR affected concrete elements in lithium solutions. Most of these methods do not achieve sufficient ingress of the lithium salts into concrete (Thomas et al., 2007). The electrochemical process showed higher Li penetration, but reduced the pH of the pore solution near rebar (cathode) due to electrochemical reduction of water. Surface overlay is commonly used on road and airfield pavements and on highway dividers. In this case, better penetration of Li can be achieved by several applications of smaller amounts, e.g., 0.06 L/m2 to 0.40 L/m2 of 30% LiNO3 solution with multiple applications (Folliard et al., 2003; Thomas et al., 2007). Injection of lithium salts has been applied to almost 100 ASR-affected concrete structures in Japan (Kobayashi and Takagi, 2020). Since it can only prevent further deterioration and not recover the lost performance, Kobayashi and Takagi suggested that it is desirable to perform lithium injection in an as early as possible stage of deterioration (Kobayashi and Takagi, 2020). However, they found that lithium injection into concrete at early deterioration stage, which indeed suppressed the ASR expansion to some extent, resulted in a larger final expansion than that of the concrete treated with lithium after developing ASR cracks. Moreover, injection of lithium also took longer time for concrete at early stage of deterioration, thus the authors suggested that it would be reasonable and economical to apply this treatment only to concrete already suffering ASR with expected further deterioration. Of course, one should avoid over development of ASR cracks, as it will affect the serviceability of concrete structure. An optimization of injection time is therefore very important. For this purpose, precise evaluation of the stage of ASR is critical for lithium treatment.
ASR is a very slow process at ambient temperature, thus many studies applying lithium salts to mitigating ASR expansion are based on accelerated or ultra accelerated (autoclave) testing methods (Ohama et al., 1989; Qinghan et al., 1995; Bian et al., 1996; Mo et al., 2003). Feng et al. (2005) concluded that the results from autoclave methods involving lithium are not directly comparable to those from studies at lower temperatures and pressures. In contrast, Berra et al. (2003) found that LiNO3 was effective at both low (38°C) and high (150°C) temperatures and reported a linear relationship of the effective LiNO3 dosage (Li/[K + Na] molar ratio) between the two temperatures as shown in Figure 6. However, they also found that Li2CO3 was only effective at 38°C.
FIGURE 6. Comparison between the effective dosages of lithium nitrate for mitigating ASR obtained from the ultra-accelerated concrete prism test (150°C) and the concrete prism test at 38°C and 100% RH. Data from (Berra et al., 2003).
Most published studies on ASR with respect to the effect of lithium on silica dissolution is based on the findings of Lawrence and Vivian (Lawrence and Vivian, 1961), who found that the dissolution of reactive silica strongly depended on the type of alkali hydroxides (i.e., NaOH, KOH, or LiOH) following the order LiOH < NaOH < KOH. Twenty years later, Wijnen et al. (1989) reported similar results and suggested that the dissolution rate of reactive silica decreased with an increase in the effective cation radius of the alkaline species following the order K+ < Na+ < Li+. While many later studies supported the observation of reduced silica dissolution in the presence of various lithium compounds (Plettinck et al., 1994; Kurtis and Monteiro, 2003; Collins et al., 2004b; Feng et al., 2005; Tremblay et al., 2010; Rajabipour et al., 2015), others also observed no significant influence of Li on silica dissolution (Dove and Nix, 1997; Dove, 1999; Leemann et al., 2014; Oey et al., 2020; Leemann, 2021; Bagheri et al., 2021). In some cases, even an increase in silica dissolution in presence of lithium was observed (Kurtis and Monteiro, 2003; Collins et al., 2004b; Bagheri et al., 2021). Kurtis and Monteiro (Kurtis and Monteiro, 2003) and Collins et al. (2004b) studied the dissolution of silica gel in simulated pore solutions with and without lithium salts and observed that in the slurries prepared with LiCl and LiNO3, the dissolved Si concentration decreased with increasing lithium dosages, while for LiOH they observed an increase in silica dissolution with increasing lithium dosage. A recent study (Bagheri et al., 2021) indicated that lithium in the absence of calcium could in fact accelerate SiO2 dissolution rate at high pH values by 20–50%.
The above contradictory observations reported in literature are found to be related to the influence of pH and Ca2+ on the effect of Li+ the dissolution rates (Bagheri et al., 2021). Many studies, which investigated the effect of Li on the dissolution of SiO2, were carried out under acidic to neutral conditions (Plettinck et al., 1994; Dove and Nix, 1997; Dove, 1999), where no or only a slightly retarding effect of Li+ in comparison to Na+ or K+ was observed and related to the lower tendency of Li+ to sorb on the SiO2 surface and faster ligand exchange rate (Dove, 1999). Bagheri et al. (2021) investigated the effect of Li+ under high pH conditions (in 400 mM KOH) and found a clear acceleration of SiO2 dissolution in the presence of Li+, which might be related to the ability of Li+ to form surface complexes on silica. It should be noted that lithium can precipitate in the presence of silica as Li2SiO3 (Zhou et al., 2018; Bagheri et al., 2021), which can lower the measured silicon concentration or mass loss in dissolution experiments (Kurtis and Monteiro, 2003; Tremblay et al., 2010; Leemann et al., 2014; Zhou et al., 2018; Oey et al., 2020; Leemann, 2021), leading to an apparent decrease in the observed dissolution rate. In the presence of calcium, the effect of Li on the dissolutions changes drastically. A significant decrease in SiO2 dissolution rate in 100 and 1,000 mM NaOH in the presence of both Li and Ca and the formation of a dense C-S-H containing Li were observed (Leemann et al., 2014; Zhou et al., 2018), indicating a destabilization of Li2SiO3 in the presence of calcium. It has been speculated that this Li containing C-S-H layer is responsible for the slowdown of the silica dissolution in the presence of both Li and Ca. More detailed discussions on influence of the lithium on the change of ASR products and formation of Li-Si complex are presented in the next sections.
The detailed study of the literature has indicated that Li might somewhat accelerate SiO2 dissolution, but only in the absence of calcium, while in the presence of Ca and Li a clear decrease of the silica dissolution has been observed (Leemann et al., 2014; Zhou et al., 2018), which might contribute together with other factors to the lower the expansion observed for Li containing concretes.
Along with the studies on dissolution of silica in presence of lithium, also changes in the ASR product has been reported in many of the studies mentioned above. Several studies reported that presence of lithium lowers the CaO/SiO2 as well as the (Na + K)/Si ratio in the ASR products (Kawamura and Fuwa, 2003; Feng et al., 2010; Leemann et al., 2014). Feng and co-workers (Feng et al., 2010) observed a dense rigid alkali–silica gel composed of Li with low-Ca contents. The low content of Ca in reaction products was also confirmed in the extensive study by Leemann et al. (2014). They suggested that Ca can be replaced by lithium due to comparable radius of the hydrated cation. It also has been suggested that Li+, due to its smaller ionic radius and higher charge density, is more readily incorporated in ASR products than K+ and Na+ (Kawamura and Fuwa, 2003; Mo, 2005).
In addition to lower the Ca content of ASR products, the precipitation of amorphous Li-Si products has been observed (Sakaguchi et al., 1989; Schneider et al., 2008; Guo et al., 2019). Due to the lack of characterizations of the chemical composition and molecular structure, the amorphous Li-Si products are often described as “Li-Si complex” following the work of Lawrence and Vivian (1961). In some cases, also the precipitation of crystalline Li-containing products has been observed (Mo et al., 2003; Collins et al., 2004a; Feng et al., 2010), generally Li2SiO3, which seems to form in the absence of Ca and at low Ca but high Li contents (Zhou et al., 2018; Bagheri et al., 2021). However, so far no systematic investigations about the formation conditions of the amorphous and crystalline Li-containing products have been conducted. Leemann et al. (2014) found no evidence of crystalline Li2SiO3 formation and argued that crystalline lithium silicate is unlikely to form under dosages of Li/Na + K < 1.0.
Most studies reported that those Li-containing products are not expansive (Sakaguchi et al., 1989; Diamond, 1992; Kawamura et al., 1994; Leemann et al., 2014), although a minimum proportion of lithium must be reached to be non-expansive (Stark, 1992), which could explain the “pessimum” effect of lithium dosages on controlling ASR expansion. However, a recent study showed that formation of crystalline Li2SiO3 could actually cause expansion and cracks in concrete after long periods of exposure (Liu et al., 2019).
Several researchers (Mitchell et al., 2004; Schneider et al., 2008; Leemann et al., 2014) have used nuclear magnetic resonance (NMR) spectroscopy to study the effects of lithium on the chemical structure of ASR gels. The incorporation of Li+ ions into the ASR gel changes its structure from a product with a layered structure (containing mainly Q3 sites) to a product with more disordered networks containing mainly Q1 and Q2 sites. This was put forward as a possible reason, why Li based ASR products show little expansion. It was claimed that expansive gels are typically characterized by large presence of Q3 sites as layered silicates, while the depolymerized products containing mainly Q1 and Q2 sites are not considered to swell upon contact with water (Kirkpatrick et al., 2005; Tambelli et al., 2006). Also Kurtis and coworkers (Kurtis et al., 2000; Kurtis and Monteiro, 2003) suggested that the suppressive effect of lithium on ASR expansion should be attributed to the limitation of ASR gel re-polymerization, rather than reduced dissolution of silica. By dispersing the ASR gel extracted from ASR-affected structure to NaOH solution alone, they observed that ASR is partially dissolved and re-polymerized as a potentially expansive gel. However, when the ASR gel was exposed to the mixture NaOH and LiCl solution, re-polymerization into an expansive gel was limited.
The understanding of ASR mitigation mechanisms by Li is simply based on the assumption that conventional ASR products, which have a layered silicate structure, will swell upon uptake of water while Li-containing ASR products with mainly Q1 and Q2 sites will not swell. However, as recent work based on synthetic ASR products (Shi et al., 2019) and field ASR products (Leemann et al., 2020) demonstrated that both amorphous and crystalline ASR products do not swell upon uptake of water, rather alternative mechanisms seem responsible for ASR expansion as well as their suppression by Li, indicating an urgent need for clarifying the real cause of ASR expansion.
Another mechanism of ASR mitigation suggested for lithium is that these products serve as physical barrier preventing the further dissolution and reaction of reactive silica. This mechanism was first suggested by Lawrence and Vivian (1961), who reported that the lithium silicates formed had low solubility producing a coating effectively protecting the reactive aggregates from further participation in ASR. This observation has been supported by many later studies (Kawamura and Fuwa, 2003; Mitchell et al., 2004; Feng et al., 2010; Leemann et al., 2014; Leemann et al., 2015; Kim and Olek, 2016; Guo et al., 2019). Leemann et al. (2015) observed that the mitigating efficiency of LiNO3 lessens with increasing the specific surface area of the reactive aggregates, due to the increased area to be covered by the lithium products. Based on this finding, Kawamura (2017) assumed that the mitigating effects of lithium on ASR affected structures may be related to the amount of ASR products already formed. Few studies reported that only the Li products containing Ca could serve a physical barrier. Zhou et al. (2018) observed that quartz glass slices immersed in solution containing both LiNO3 and Ca(OH)2 were well protected by the precipitation of a dense layer, while samples were seriously damaged in the solution with only LiNO3 or Ca(OH)2. In contrast, Tremblay et al. (2010) considered the formation of physical barriers unlikely based on detail analysis of the surface and suggested an increased chemical stability of silica due to a presently unknown mechanism as the probable cause.
Several additional mechanisms for the ASR mitigation of lithium have been proposed. Prezzi et al. (1997) introduced an electrical double layer (EDL) theory to explain the suppressive effect of lithium on ASR expansion. ASR gels are negatively charged (Krattiger et al., 2021), and are thus surrounded by a positively charged electrical double layer where cations accumulate. Theoretically, cations with the same valence but smaller hydrated ionic radii will result in a thinner double layer, which would cause based on the swelling theory less gel expansion. As the hydrated radius increases in the order K+ < Na+ < Li+ (Conway, 1981), this would mean that Li would result in higher expansion, which is contradictory to the results generally observed in expansion testing, and thus also to the explanation suggested by (Prezzi et al., 1997). It was also proposed that presence of lithium may reduce the repulsive forces between colloidal ASR gel particles (Mohd et al., 2017). Bian et al. (1996) proposed that the suppressive effect of cations on ASR expansion depended on the ionic surface charge density. The higher value of surface charge density, the stronger the bonding between the cation and anions in the gels, the less tendency to expand. Others (McCormick et al., 1989; Gaboriaud et al., 1999) studied the mitigating mechanisms based on sol-gel principles, they observed that presence of lithium enhanced the formation of large silicate species in solution, which took longer time to destabilize (Gaboriaud et al., 1998).
Based on the literature reviewed above it becomes clear, that the presence of some Ca as well as of sufficient Li are prerequisites for an effective mitigation by Li. Different mechanism such as blocking of dissolution, formation of a non-expansive solid, prevention of swelling due to other reasons have been suggested, however the findings reported in literature are contradictory and the experimental evidence pointing in any direction is circumstantial and inconclusive. This may not be surprising as also the mechanism of ASR expansion itself is under debate since recent investigations have suggested that the swelling theory does not agree with the observed changes in the ASR product (Shi et al., 2019; Leemann et al., 2020; Shi et al., 2020a; Geng et al., 2021).
Analysis of the pore solution of the samples containing lithium can also provide valuable information about the reaction of Li with Si. The addition of some lithium salts (LiF and Li2CO3) can increase the pore solution pH through reaction with Ca(OH)2 forming insoluble CaCO3 or CaF2, while Li+ and OH− remain in solution. However, the pore solution pH is not affected by LiNO3 (Diamond, 1999). Several studies (Sakaguchi et al., 1989; Collins et al., 2004b; Tremblay et al., 2008; Tremblay et al., 2010; Leemann et al., 2014) reported that the Li concentration of the extracted pore solution decreased with time, while the concentration of K and Na remained unchanged. In contrast, the samples without Li showed a decrease of K and Na concentrations (Sakaguchi et al., 1989). Diamond and Ong (1992) reported that even in the samples without reactive aggregates, 40% of Li was absorbed by the cement hydrates after 1 day of hydration, while only 25% NaOH and 20% KOH were incorporated in the C-S-H phase. Similarly (Bérubé et al., 2004), reported that only 35% of the original quantity of lithium left in pore solution in contrast to 55 and 80% for Na and K respectively. Kim and Olek (2015) found that 50% of added lithium is not available in the pore solution but incorporated into cement hydrates. These observations suggest the precipitation of a solid phase containing Li and a stronger interaction of Si with Li than with K and Na.
Only few studies have investigated the long-term effectiveness of lithium salts to inhibit ASR. Mo et al. (2003) studied the long-term effectiveness of LiOH in mitigating ASR by applying a rigorous experimental condition for the mortars, i.e., cured at 80°C for 3 years after being autoclaved for 24 h at 150°C. Under this condition, they found that LiOH was able to inhibit long-term ASR expansion effectively at Li/Na ratio above 0.3 or 0.6, depending on the alkali dosage of cements, i.e., 2.5% or 3.0%. A 6-years experimental study (Ekolu et al., 2017) also showed that LiNO3 with Li/(K + Na) molar ratio of 0.74 is effective in long-term control of delayed ettringite formation (DEF) or combined ASR-DEF mechanism in concretes. However, other researchers (Zapała-Sławeta and Owsiak, 2017) observed that, LiNO3 used at the molar ratio of Li/(K + Na) = 1.0, mitigated ASR only for a limited period of time as shown in Figure 7. A significant increase of expansion was observed from 180 to 540 days. Microscopic observation from this study confirmed that large amount of ASR products were formed with indication of multiple exudations in the presence of LiNO3 at long term. Additionally, DEF was also observed in this study, which possibly contribute the boost expansion after 900 days. Slower DEF than ASR was also observed in (Ekolu et al., 2017). The mechanism for the post formation of ASR products within this period remains unclear, but the authors stated that the ASR products formed seemed to be less viscous. Recently, Liu et al. (2019) showed that high concentration of LiNO3 only inhibit ASR at early stages, and suggested that formation of LiSiO3 could cause expansion and cracking of concrete after long period of time.
FIGURE 7. Mortar bar expansion determined according to ASTM C227. Data from (Zapała-Sławeta and Owsiak, 2017).
In some cases, the use of Al-rich SCMs alone may not be able to fully control the ASR. Therefore, combination of Al-rich SCMs with small dosage of lithium salts to mitigate ASR has also gained some interests. Thomas et al. (2001) studied the efficacy of combinations of fly ash and lithium salts for preventing ASR. Their results indicated that the beneficial effects of lithium and fly ash are cumulative when the materials are combined and, in some cases, there is a synergistic effect. Drimalas et al. (2012) reported that both single use of either 30% class C fly ash or LiNO3 with a Li/(K + Na) molar ratio 0.56 are effective in reducing ASR expansion of concrete containing a highly reactive fine aggregate from Texas. The combinations of lithium and fly ash have shown synergistically beneficial but also detrimental effects (i.e., no synergistic effect and even increase of ASR expansion) may occur. A mixture containing both LiNO3 and 30% class C fly ash showed only a 50% of reduction of the ASR expansion compared to single use of 30% class C fly ash after 3,500 days of outdoor exposure. Venkatanarayanan and Rangaraju (2014) conducted a quantitative analysis of the combined effects of fly ash and lithium admixture in mitigating ASR in mortars containing Spratt aggregate. A linear correlation between the minimum oxide contents (for ASR inhibiting oxides: SiO2, SiO2equi, and SiO2+Al2O3+Fe2O3) or maximum oxide contents (for ASR promoting oxides: CaO, CaOequi, and CaO + MgO + SO3) and the lithium dosage needed to achieve effective ASR mitigation was established as shown in Figure 8. The results show that use of lithium nitrate is not needed for mortars containing fly ashes with less than 14.40% CaO. It was expected that the correlation could be used to optimize the lithium dosage as a function of fly ash composition to provide an economic solution for ASR mitigation.
FIGURE 8. Correlation of chemical contents of fly ash and lithium dosage required to achieve ASR mitigation at 25% fly ash replacement level. Data reproduced from (Venkatanarayanan and Rangaraju, 2014).
The efficiency of Al in mitigating ASR expansion has been directly confirmed by replacing Portland cement with 20% Al(OH)3 following ASTM C1293 or 10% γ-Al2O3 following ASTM C 1260 or ASTM C227. The presence of Al leads to a slower SiO2 dissolution and thus a slower formation of reaction products. Sorption of Al(OH)4- on the surface of silica and the slowing down of silica dissolution silica is more distinct at intermediate pH values (<12), while at pH 13 and above the sorption of Al(OH)4- on silica becomes weak resulting in only feeble suppression of the dissolution rate. As the sorption of Al(OH)4- on SiO2 only slows down, but does not prevent silica dissolution, although the presence of Al(OH)3 or Al-rich SCMs seems to prevent ASR completely up to 700 days, additional not yet well-understood effects could play an important role.
Uptake of alkalis by C-S-H is not significantly increased in the presence of Al, such that the mechanism of significantly increased alkali fixation by C-A-S-H with subsequent pH reduction can be excluded.
The structure and composition of ASR products are not affected by the presence of Al at ambient conditions, while at higher temperature such as 80°C, formation of alkali alumino-silicates or zeolites could lower the potential to form ASR products. However, the formation of alkali alumino-silicate and zeolite is under most conditions expected to be too slow to be relevant in suppressing ASR formation in field concretes.
Different lithium salts, such as LiNO3, LiNO2, Li2CO3, LiF, LiOH, LiOH·H2O, Li2SiO3, Li2SO4, LiCl, LiBr, and LiH2PO4, have been found to be effective in mitigating ASR at a certain dosage. The use of some lithium salt such as Li2CO3 and LiF as well as LiOH results an increase of pore solution pH. Preferably, LiNO3 and LiNO2 are used due to their high solubility and good compatibility with other admixtures. LiNO2 can have in addition a positive effect on preventing rebar corrosion.
The effective dosage of lithium depends on the type of lithium salts, alkali content of the cements and reactivity of the aggregates. Lithium salts are more effective in mitigating ASR for the highly reactive aggregates than for the less reactive aggregates. Generally, high alkali content in the cement result in high effective Li dosages needed. A linear correlation between the effective lithium dosage and the difference between total alkali content and threshold alkali level of the aggregates has been established by some authors, while others did not find a correlation between alkali reactivity of the aggregates and effective lithium dosage. More investigations will be needed to clarify those effects.
LiNO3 was found to be effective at both low (38°C) and high (150°C) temperatures, while Li2CO3 was only effective at 38°C, due to unknown reasons. In laboratory studies, lithium salts are often used as an admixture, while in practice they are rather applied to already ASR-affected structures. The transferability from pre-treatment laboratory results to post-exposure treatment on concrete structure will also need further research.
Various mechanisms have been proposed to explain why lithium salts mitigate ASR. Lithium might somewhat accelerate SiO2 dissolution, but only in the absence of calcium, while in the presence of Ca and Li a clear decrease of the silica dissolution has been observed which might contribute together with other factors to the lower the expansion observed for Li containing concretes. Li can replace Ca, K and Na in ASR products, thus alter their composition and structure although it remains unclear how that affects expansion. In addition, Li can also react with Si to form amorphous and/or crystalline lithium silicates. Different mechanism such as blocking of dissolution, formation of a non-expansive solid, prevention of swelling due to other reasons have been suggested, however the findings reported in literature are contradictory and the experimental evidences are not conclusive, indicating more systematic research will be needed.
The combination of Al-rich SCMs such as fly ash and lithium could be very efficient in preventing ASR. A correlation of the CaO content of fly ash and the required lithium dosage could be established. Such relationships could be used to optimize the lithium dosage as a function of fly ash composition to provide an economic solution for ASR mitigation.
ZS and BL contributed to the conception and design of the review. ZS wrote the first draft of the manuscript. BL contributed with writing, reviewing and editing of the manuscript.
The partial financial contribution of SNF Sinergia project: alkali-silica reaction in concrete (ASR), grant number CRSII5_17108; is gratefully acknowledged.
ZS was employed by Global R&D, HeidelbergCement AG.
The remaining author declares that the research was conducted in the absence of any commercial or financial relationships that could be construed as a potential conflict of interest.
All claims expressed in this article are solely those of the authors and do not necessarily represent those of their affiliated organizations, or those of the publisher, the editors and the reviewers. Any product that may be evaluated in this article, or claim that may be made by its manufacturer, is not guaranteed or endorsed by the publisher.
ASR, alkali-silica reaction; C-S-H, calcium-silicate-hydrate; C-A-S-H, calcium-alumina-silicate-hydrate; OPC, ordinary Portland cement; SCMs, supplementary cementitious materials; SEM/EDS, scanning electron microscopy with energy dispersive spectroscopy; TAL, threshold alkali level; DEF, delayed ettringite formation.
Aquino, W., Lange, D. A., and Olek, J. (2001). The Influence of Metakaolin and Silica Fume on the Chemistry of Alkali-Silica Reaction Products. Cem. Concr. Compos. 23 (6), 485–493. doi:10.1016/s0958-9465(00)00096-2
Avet, F., and Scrivener, K. (2018). Investigation of the Calcined Kaolinite Content on the Hydration of Limestone Calcined Clay Cement (LC3). Cem. Concr. Res. 107, 124–135. doi:10.1016/j.cemconres.2018.02.016
Bagheri, M., Lothenbach, B., Shakoorioskooie, M., and Scrivener, K. (2022). Effect of Different Ions on Dissolution Rates of Silica and Feldspars at High pH. Cem. Concr. Res 152, 106644. doi:10.1016/j.cemconres.2021.106644
Bagheri, M., Lothenbach, B., Shakoorioskooie, M., Leemann, A., and Scrivener, K. (2021). Use of Scratch Tracking Method to Study the Dissolution of alpine Aggregates Subject to Alkali Silica Reaction. Cem. Concr. Compos. 124, 104260. doi:10.1016/j.cemconcomp.2021.104260
Bérubé, M. A., Tremblay, C., Fournier, B., Thomas, M. D., and Stokes, D. B. (2004). Influence of Lithium-Based Products Proposed for Counteracting ASR on the Chemistry of Pore Solution and Cement Hydrates. Cem. Concr. Res. 34 (9), 1645–1660. doi:10.1016/j.cemconres.2004.03.025
Berra, M., Mangialardi, T., and Paolini, A. E. (2003). Use of Lithium Compounds to Prevent Expansive Alkali-Silica Reactivity in concrete. Adv. Cem. Res. 15 (4), 145–154. doi:10.1680/adcr.2003.15.4.145
Bian, Q., Nishibayashi, S., Kuroda, T., Wu, X., and Tang, M. (1996). “Various Chemicals in Suppressing Expansion Due to Alkali–Silica Reaction,” in Proceedings of the 10th International Conference on Alkali–Aggregate Reaction, Melbourne, Australia, August 18–24, 1996 (CSIRO Division of Building Construction and Engineering), 868.
Bickmore, B. R., Nagy, K. L., Gray, A. K., and Brinkerhoff, A. R. (2006). The Effect of Al(OH)4− on the Dissolution Rate of Quartz. Geochim. Cosmochim. Acta 70 (2), 290–305. doi:10.1016/j.gca.2005.09.017
Chappex, T., and Scrivener, K. (2012a). Alkali Fixation of C-S-H in Blended Cement Pastes and its Relation to Alkali Silica Reaction. Cem. Concr. Res. 42 (8), 1049–1054. doi:10.1016/j.cemconres.2012.03.010
Chappex, T., and Scrivener, K. L. (2012b). The Influence of Aluminium on the Dissolution of Amorphous Silica and its Relation to Alkali Silica Reaction. Cem. Concr. Res. 42 (12), 1645–1649. doi:10.1016/j.cemconres.2012.09.009
Collins, C. L., Ideker, J. H., and Kurtis, K. E. (2004a). Laser Scanning Confocal Microscopy for In Situ Monitoring of Alkali-Silica Reaction. J. Microsc. 213 (2), 149–157. doi:10.1111/j.1365-2818.2004.01280.x
Collins, C. L., Ideker, J. H., Willis, G. S., and Kurtis, K. E. (2004b). Examination of the Effects of LiOH, LiCl, and LiNO3 on Alkali-Silica Reaction. Cem. Concr. Res. 34 (8), 1403–1415. doi:10.1016/j.cemconres.2004.01.011
Conway, B. E. (1981). Ionic Hydration in Chemistry and Biophysics. Amsterdam, Netherlands: Elsevier Scientific Publishing.
Demir, İ., and Arslan, M. (2013). The Mechanical and Microstructural Properties of Li2SO4, LiNO3, Li2CO3 and LiBr Added Mortars Exposed to Alkali-Silica Reaction. Constr. Build. Mater. 42, 64–77. doi:10.1016/j.conbuildmat.2012.12.059
Demir, İ., Sevim, Ö., and Kalkan, İ. (2018). Microstructural Properties of Lithium-Added Cement Mortars Subjected to Alkali–Silica Reactions. Sādhanā 43 (7), 1–10. doi:10.1007/s12046-018-0901-3
Deschner, F., Winnefeld, F., Lothenbach, B., Seufert, S., Schwesig, P., Dittrich, S., et al. (2012). Hydration of Portland Cement with High Replacement by Siliceous Fly Ash. Cem. Concr. Res. 42 (10), 1389–1400. doi:10.1016/j.cemconres.2012.06.009
Diamond, S., and Ong, S. (1992). “The Mechanism of Lithium Effects on ASR,” in Proc. of 9th International Conference on Alkali-Aggregate Reaction, Westminster, London, July 27–31, 269–278.
Diamond, S. (1981). Effects of Two Danish Flyashes on Alkali Contents of Pore Solutions of Cement-Flyash Pastes. Cement Concrete Res. 11 (3), 383–394. doi:10.1016/0008-8846(81)90110-1
Diamond, S. (1992). “The Mechanisms of Lithium Effects on ASR,” in Proc. of 9th International Conference on Alkali-Aggregate Reaction, Westminster, London, July 27-31, 269.
Diamond, S. (1999). Unique Response of LiNO3 as an Alkali Silica Reaction-Preventive Admixture. Cem. Concr. Res. 29 (8), 1271–1275. doi:10.1016/s0008-8846(99)00115-5
Dove, P. M., and Nix, C. J. (1997). The Influence of the Alkaline Earth Cations, Magnesium, Calcium, and Barium on the Dissolution Kinetics of Quartz. Geochim. Cosmochim. Acta 61 (16), 3329–3340. doi:10.1016/s0016-7037(97)00217-2
Dove, P. M. (1999). The Dissolution Kinetics of Quartz in Aqueous Mixed Cation Solutions. Geochim. Cosmochim. Acta 63 (22), 3715–3727. doi:10.1016/s0016-7037(99)00218-5
Drimalas, T., Ideker, J. H., Bentivegna, A. F., Folliard, K. J., Fournier, B., and Thomas, M. D. A. (2012). The Long-Term Monitoring of Large-Scale Concrete Specimens Containing Lithium Salts to Mitigate Alkali-Silica Reaction. Spec. Publ. 289, 1–17.
Duchesne, J., and Bérubé, M.-A. (2001). Long-term Effectiveness of Supplementary Cementing Materials against Alkali-Silica Reaction. Cem. Concr. Res. 31 (7), 1057–1063. doi:10.1016/s0008-8846(01)00538-5
Durand, B. (2000). “More Results about the Use of Lithium Salts and mineral Admixtures to Inhibit ASR in concrete,” in Proceedings of the 11th International Conference on Alkali–Aggregate Reaction, Quebec, Canada, June 11–16.
Ekolu, S., Rakgosi, G., and Hooton, D. (2017). Long-term Mitigating Effect of Lithium Nitrate on Delayed Ettringite Formation and ASR in Concrete - Microscopic Analysis. Mater. Charact. 133, 165–175. doi:10.1016/j.matchar.2017.09.025
Feng, X., Thomas, M. D. A., Bremner, T. W., Balcom, B. J., and Folliard, K. J. (2005). Studies on Lithium Salts to Mitigate ASR-Induced Expansion in New concrete: a Critical Review. Cem. Concr. Res. 35 (9), 1789–1796. doi:10.1016/j.cemconres.2004.10.013
Feng, X., Thomas, M. D. A., Bremner, T. W., Folliard, K. J., and Fournier, B. (2010). New Observations on the Mechanism of Lithium Nitrate against Alkali Silica Reaction (ASR). Cem. Concr. Res. 40 (1), 94–101. doi:10.1016/j.cemconres.2009.07.017
Fernandes, I., Noronha, F., and Teles, M. (2007). Examination of the concrete from an Old Portuguese Dam: Texture and Composition of Alkali–Silica Gel. Mater. Charact. 58 (11–12), 1160–1170. doi:10.1016/j.matchar.2007.04.007
Fernandes, I. (2009). Composition of Alkali-Silica Reaction Products at Different Locations within concrete Structures. Mater. Charact. 60 (7), 655–668. doi:10.1016/j.matchar.2009.01.011
Folliard, K. J., Thomas, M. D. A., and Kurtis, K. E. (2003). Guidelines for the Use of Lithium to Mitigate or Prevent ASR. Washington, DC: US Department of Transportation, Federal Highway Administration (FHWA).
Folliard, K. J., Thomas, M. D. A., Fournier, B., Kurtis, K. E., and Ideker, J. H. (2006). Interim Recommendations for the Use of Lithium to Mitigate or Prevent Alkali-Silica Reaction (ASR). United States: Federal Highway AdministrationOffice of Infrastructure.
Gaboriaud, F., Chaumont, D., Nonat, A., Hanquet, B., and Craeivich, A. (1998). Study of the Influence of Alkaline Ions (Li, Na and K) on the Structure of the Silicate Entities In Silico Alkaline Sol and on the Formation of the Silico-Calco-Alkaline Gel. J. Sol-Gel Sci. Technol. 13 (1–3), 353–358. doi:10.1023/a:1008644405473
Gaboriaud, F., Nonat, A., Chaumont, D., Craievich, A., and Hanquet, B. (1999). 29Si NMR and Small-Angle X-ray Scattering Studies of the Effect of Alkaline Ions (Li+, Na+, and K+) In Silico-alkaline Sols. J. Phys. Chem. B 103 (12), 2091–2099. doi:10.1021/jp984074x
Geng, G., Barbotin, S., Shakoorioskooie, M., Shi, Z., Leemann, A., Sanchez, D. F., et al. (2021). An In-Situ 3D Micro-XRD Investigation of Water Uptake by Alkali-Silica-Reaction (ASR) Product. Cem. Concr. Res. 141, 106331. doi:10.1016/j.cemconres.2020.106331
Guo, S., Dai, Q., Sun, X., Xiao, X., Si, R., and Wang, J. (2018). Reduced Alkali-Silica Reaction Damage in Recycled Glass Mortar Samples with Supplementary Cementitious Materials. J. Clean. Prod. 172, 3621–3633. doi:10.1016/j.jclepro.2017.11.119
Guo, S., Dai, Q., and Si, R. (2019). Effect of Calcium and Lithium on Alkali-Silica Reaction Kinetics and Phase Development. Cem. Concr. Res. 115, 220–229. doi:10.1016/j.cemconres.2018.10.007
Hagelia, P., and Fernandes, I. (2012). “On the AAR Susceptibility of Granitic and Quartzitic Aggregates in View of Petrographic Characteristics and Accelerated Testing,” in The 14th International Conference on Alkali-Aggregate Reactions in Concrete, Austin, Texas, USA, May 20–25, 10.
Hong, S.-Y., and Glasser, F. P. (2002). Alkali Sorption by C-S-H and C-A-S-H Gels. Cem. Concr. Res. 32 (7), 1101–1111. doi:10.1016/s0008-8846(02)00753-6
Hünger, K.-J. (2007). The Contribution of Quartz and the Role of Aluminum for Understanding the AAR with Greywacke. Cem. Concr. Res. 37 (8), 1193–1205. doi:10.1016/j.cemconres.2007.05.009
Iler, R. K. (1973). Effect of Adsorbed Alumina on the Solubility of Amorphous Silica in Water. J. Colloid Interf. Sci. 43 (2), 399–408. doi:10.1016/0021-9797(73)90386-x
Iler, K. R. (1979). The Chemistry of Silica. Solubility, Polymerization, Colloid and Surface Properties and Biochemistry of Silica. John Wiley & Sons.
Islam, M. S., and Ghafoori, N. (2016). Experimental Study and Empirical Modeling of Lithium Nitrate for Alkali-Silica Reactivity. Constr. Build. Mater. 121, 717–726. doi:10.1016/j.conbuildmat.2016.06.026
Kandasamy, S., and Shehata, M. H. (2014). The Capacity of Ternary Blends Containing Slag and High-Calcium Fly Ash to Mitigate Alkali Silica Reaction. Cem. Concr. Compos. 49, 92–99. doi:10.1016/j.cemconcomp.2013.12.008
Katayama, T. (2012a). “Late-expansive ASR in a 30-year Old PC Structure in Eastern Japan,”. Paper in Proc. 14th International Conference on Alkali-Aggregate Reaction (ICAAR), Austin, Texas, USA, May 20–25, 862–873.
Katayama, T. (2012b). “ASR Gels and Their Crystalline Phases in concrete—universal Products in Alkali–Silica, Alkali–Silicate and Alkali–Carbonate Reactions,” in Proceedings of the 14th International Conference on Alkali Aggregate Reactions (ICAAR), Austin, Texas, May 20–25, 1–12.
Kawamura, M., and Fuwa, H. (2003). Effects of Lithium Salts on ASR Gel Composition and Expansion of Mortars. Cem. Concr. Res. 33 (6), 913–919. doi:10.1016/s0008-8846(02)01092-x
Kawamura, M., Takeuchi, K., and Sugiyama, A. (1994). Mechanisms of Expansion of Mortars Containing Reactive Aggregate in NaCl Solution. Cem. Concr. Res. 24 (4), 621–632. doi:10.1016/0008-8846(94)90186-4
Kawamura, M. (2017). A Discussion of the Paper "ASR Prevention - Effect of Aluminum and Lithium Ions on the Reaction Products" by Andreas Leemann, Laetitia Bernard, Salaheddine Alahrache, Frank Winnefeld. Cem. Concr. Res., 102, 225–226. doi:10.1016/j.cemconres.2017.05.012
Kim, T., and Olek, J. (2015). Modeling of Early Age Loss of Lithium Ions from Pore Solution of Cementitious Systems Treated with Lithium Nitrate. Cem. Concr. Res. 67, 204–214. doi:10.1016/j.cemconres.2014.10.010
Kim, T., and Olek, J. (2016). The Effects of Lithium Ions on Chemical Sequence of Alkali-Silica Reaction. Cem. Concr. Res. 79, 159–168. doi:10.1016/j.cemconres.2015.09.013
Kirkpatrick, R. J., Kalinichev, A. G., Hou, X., and Struble, L. (2005). Experimental and Molecular Dynamics Modeling Studies of Interlayer Swelling: Water Incorporation in Kanemite and ASR Gel. Mat. Struct. 38 (4), 449–458. doi:10.1007/bf02482141
Kobayashi, K., and Takagi, Y. (2020). Penetration of Pressure-Injected Lithium Nitrite in concrete and ASR Mitigating Effect. Cem. Concr. Compos. 114, 103709. doi:10.1016/j.cemconcomp.2020.103709
Krattiger, N., Lothenbach, B., and Churakov, S. V. (2021). Sorption and Electrokinetic Properties of ASR Product and C-S-H: A Comparative Modelling Study. Cem. Concr. Res. 146, 106491. doi:10.1016/j.cemconres.2021.106491
Kurihara, T., and Katawaki, K. (1989). “Effects of Moisture Control and Inhibition on Alkali Silica Reaction,” in Proc., 8th Int. Conf. on Alkali Aggregate Reaction in Concrete, Kyoto, Japan, July 17–20, 629–634.
Kurtis, K. E., and Monteiro, P. J. M. (2003). Chemical Additives to Control Expansion of Alkali-Silica Reaction Gel: Proposed Mechanisms of Control. J. Mater. Sci. 38 (9), 2027–2036. doi:10.1023/a:1023549824201
Kurtis, K. E., Monteiro, P. J. M., and Meyer-Ilse, W. (2000). Examination of the Effect of LiCl on ASR Gel Expansion. California: Ernest Orlando Lawrence Berkeley National Lab.
L’Hôpital, E., Lothenbach, B., Scrivener, K., and Kulik, D. A. (2016). Alkali Uptake in Calcium Alumina Silicate Hydrate (CASH). Cem. Concr. Res. 85, 122–136. doi:10.1016/j.cemconres.2016.03.009
Lane, D. S., and Board, V. C. T. (2002). Laboratory Investigation of Lithium-Bearing Compounds for Use in concrete. Charlottesville, VA: Virginia Transportation Research Council.
Lane, D. S. (2000). “Preventive Measures for ASR Used in Virginia, USA,” in Proceedings of the 11th International Conference on Alkali–Aggregate Reaction, Quebec, Canada, June 11–16 (Quebec: Centre de Recherche Interuniversitaire Sur Le Beton), 693.
Lawrence, M., and Vivian, H. E. (1961). Reactions of Various Alkalis with Silica. Aust. J. Appl. Sci. 12, 96–103.
Leemann, A., and Lothenbach, B. (2008). The Influence of Potassium-Sodium Ratio in Cement on concrete Expansion Due to Alkali-Aggregate Reaction. Cem. Concr. Res. 38 (10), 1162–1168. doi:10.1016/j.cemconres.2008.05.004
Leemann, A., and Lura, P. (2013). E-modulus of the Alkali-Silica-Reaction Product Determined by Micro-Indentation. Constr. Build. Mater. 44, 221–227. doi:10.1016/j.conbuildmat.2013.03.018
Leemann, A., Lörtscher, L., Bernard, L., Le Saout, G., Lothenbach, B., and Espinosa-Marzal, R. M. (2014). Mitigation of ASR by the Use of LiNO3-Characterization of the Reaction Products. Cem. Concr. Res. 59, 73–86. doi:10.1016/j.cemconres.2014.02.003
Leemann, A., Bernard, L., Alahrache, S., and Winnefeld, F. (2015). ASR Prevention - Effect of Aluminum and Lithium Ions on the Reaction Products. Cem. Concr. Res. 76, 192–201. doi:10.1016/j.cemconres.2015.06.002
Leemann, A., Shi, Z., Wyrzykowski, M., and Winnefeld, F. (2020). Moisture Stability of Crystalline Alkali-Silica Reaction Products Formed in concrete Exposed to Natural Environment. Mater. Des. 195, 109066. doi:10.1016/j.matdes.2020.109066
Leemann, A. (2017). Raman Microscopy of Alkali-Silica Reaction (ASR) Products Formed in concrete. Cem. Concr. Res. 102, 41–47. doi:10.1016/j.cemconres.2017.08.014
Leemann, A. (2021). “Impact of Different Added Alkalis on concrete Expansion Due to ASR,” in Proceedings of the 16th International Conference on Alkali-Aggregate Reaction in Concrete. Editors A. Lopes Batista, A. Santos Silva, I. Fernandes, L. Oliveira Santos, J. Custódio, and C. Serra, 175–184.
Liu, J., Yu, L., and Deng, M. (2019). Effect of LiNO3 on Expansion of Alkali-Silica Reaction in Rock Prisms and Concrete Microbars Prepared by Sandstone. Materials 12 (7), 1150. doi:10.3390/ma12071150
Lothenbach, B., Bernard, E., and Mäder, U. (2017). Zeolite Formation in the Presence of Cement Hydrates and Albite. Phys. Chem. Earth, Parts A/B/C 99, 77–94. doi:10.1016/j.pce.2017.02.006
Lumley, J. S. (1997). ASR Suppression by Lithium Compounds. Cem. Concr. Res. 27 (2), 235–244. doi:10.1016/s0008-8846(97)00003-3
McCormick, A. V., Bell, A. T., and Radke, C. J. (1989). Evidence from Alkali-Metal NMR Spectroscopy for Ion Pairing in Alkaline Silicate Solutions. J. Phys. Chem. 93 (5), 1733–1737. doi:10.1021/j100342a013
McCoy, W. J., and Caldwell, A. G. (1951). New Approach to Inhibiting Alkali-Aggregate Expansion. J. Proc. 47 (5), 693–706.
Mitchell, L. D., Beaudoin, J. J., and Grattan-Bellew, P. (2004). The Effects of Lithium Hydroxide Solution on Alkali Silica Reaction Gels Created with Opal. Cem. Concr. Res. 34 (4), 641–649. doi:10.1016/j.cemconres.2003.10.011
Mo, X., Yu, C., and Xu, Z. (2003). Long-term Effectiveness and Mechanism of LiOH in Inhibiting Alkali-Silica Reaction. Cem. Concr. Res. 33 (1), 115–119. doi:10.1016/s0008-8846(02)00934-1
Mo, X., Jin, T., Li, G., Wang, K., Xu, Z., and Tang, M. (2005). Alkali-aggregate Reaction Suppressed by Chemical Admixture at 80 °C. Constr. Build. Mater. 19 (6), 473–479. doi:10.1016/j.conbuildmat.2004.07.012
Mo, X. (2005). Laboratory Study of LiOH in Inhibiting Alkali-Silica Reaction at 20 °C: a Contribution. Cem. Concr. Res. 35 (3), 499–504. doi:10.1016/j.cemconres.2004.06.003
Mohd, I., Yasutaka, S., Hidenori, H., and Yamamoto, D. (2017). An Experimental Study on Mitigating Alkali Silica Reaction by Using Lithium Hydroxide Monohydrate. AIP Conf. Proc. 1903 (1), 30005. doi:10.1063/1.5011512
Nicoleau, L., Schreiner, E., and Nonat, A. (2014). Ion-specific Effects Influencing the Dissolution of Tricalcium Silicate. Cement Concrete Res. 59, 118–138. doi:10.1016/j.cemconres.2014.02.006
Oey, T., La Plante, E. C., Falzone, G., Hsiao, Y.-H., Wada, A., Monfardini, L., et al. (2020). Calcium Nitrate: A Chemical Admixture to Inhibit Aggregate Dissolution and Mitigate Expansion Caused by Alkali-Silica Reaction. Cem. Concr. Compos. 110, 103592. doi:10.1016/j.cemconcomp.2020.103592
Ohama, Y., Demura, K., and Kakegawa, M. (1989). “Inhibiting ASR with Chemical Admixtures,” in Proceedings of the 8th International Conference of Alkali–Aggregate Reaction, Kyoto, Japan, July 17–20.
Olafsson, H. (1986). “The Effect of Relative Humidity and Temperature on Alkali Expansion of Mortar Bars,” in Proc., 7th Int. Conf. on Alkali Aggregate Reaction in Concrete, Ottawa, Canada, August, 461–465.
Plettinck, S., Chou, L., and Wollast, R. (1994). Kinetics and Mechanisms of Dissolution of Silica at Room Temperature and Pressure. Mineral. Mag. 58A (2), 728–729. doi:10.1180/minmag.1994.58a.2.116
Prezzi, M., Monteiro, P. J. M., and Sposito, G. (1997). The Alkali–Silica Reaction: Part I. Use of the Double-Layer Theory to Explain the Behavior of Reaction-Product Gels. ACI Mater. J. 94 (1), 10–17.
Qinghan, B., Nishibayashi, S., Xuequan, W., Yoshino, A., Hong, Z., Tiecheng, W., et al. (1995). Preliminary Study of Effect of LiNO2 on Expansion of Mortars Subjected to Alkali-Silica Reaction. Cem. Concr. Res. 25 (8), 1647–1654. doi:10.1016/0008-8846(95)00161-1
Rajabipour, F., Giannini, E., Dunant, C., Ideker, J. H., and Thomas, M. D. A. (2015). Alkali-Silica Reaction: Current Understanding of the Reaction Mechanisms and the Knowledge Gaps. Cem. Concr. Res. 76, 130–146. doi:10.1016/j.cemconres.2015.05.024
Ramlochan, T., Thomas, M. D. A., and Hooton, R. D. (2004). The Effect of Pozzolans and Slag on the Expansion of Mortars Cured at Elevated Temperature: Part II: Microstructural and Microchemical Investigations. Cem. Concr. Res. 34 (8), 1341–1356. doi:10.1016/j.cemconres.2003.12.026
Šachlová, Š., Přikryl, R., and Pertold, Z. (2010). Alkali-Silica Reaction Products: Comparison between Samples from concrete Structures and Laboratory Test Specimens. Mater. Charact. 61 (12), 1379–1393. doi:10.1016/j.matchar.2010.09.010
Saito, M., Kitagawa, A., and Hasaba, S. (1992). Effectiveness of Lithium Nitrite in Suppressing Alkali-Aggregate Expansion. J. Soc. Mater. Sci. Jpn. 41 (468), 1375–1381. doi:10.2472/jsms.41.1375
Sakaguchi, T., Takakura, M., Kitagawa, A., Hori, T., Tomozawa, F., and Abe, M. (1989). “The Inhibiting Effect of Lithium Compounds on Alkali-Silica Reaction,” in 8th International Conference on Alkali-Aggregate Reaction, Kyoto, Japan, July 17–20, 229–234.
Sand, L. B., Sacco, A., Thompson, R. W., and Dixon, A. G. (1987). Large Zeolite Crystals: Their Potential Growth in Space. Zeolites 7 (5), 387–392. doi:10.1016/0144-2449(87)90001-7
Schneider, J. F., Hasparyk, N. P., Silva, D. A., and Monteiro, P. J. M. (2008). Effect of Lithium Nitrate on the Alkali-Silica Reaction Gel. J. Am. Ceram. Soc. 91 (10), 3370–3374. doi:10.1111/j.1551-2916.2008.02638.x
Shafaatian, S. M. H., Akhavan, A., Maraghechi, H., and Rajabipour, F. (2013). How Does Fly Ash Mitigate Alkali-Silica Reaction (ASR) in Accelerated Mortar Bar Test (ASTM C1567)? Cem. Concr. Compos. 37, 143–153. doi:10.1016/j.cemconcomp.2012.11.004
Shi, Z., and Lothenbach, B. (2020). The Combined Effect of Potassium, Sodium and Calcium on the Formation of Alkali-Silica Reaction Products. Cem. Concr. Res. 127, 105914. doi:10.1016/j.cemconres.2019.105914
Shi, Z., Shi, C., Zhang, J., Wan, S., Zhang, Z., and Ou, Z. (2018). Alkali-silica Reaction in Waterglass-Activated Slag Mortars Incorporating Fly Ash and Metakaolin. Cem. Concr. Res. 108, 10–19. doi:10.1016/j.cemconres.2018.03.002
Shi, Z., Geng, G., Leemann, A., and Lothenbach, B. (2019). Synthesis, Characterization, and Water Uptake Property of Alkali-Silica Reaction Products. Cem. Concr. Res. 121, 58–71. doi:10.1016/j.cemconres.2019.04.009
Shi, Z., Leemann, A., Rentsch, D., and Lothenbach, B. (2020a). Synthesis of Alkali-Silica Reaction Product Structurally Identical to that Formed in Field concrete. Mater. Des. 190, 108562. doi:10.1016/j.matdes.2020.108562
Shi, Z., Park, S., Lothenbach, B., and Leemann, A. (2020b). Formation of Shlykovite and ASR-P1 in concrete under Accelerated Alkali-Silica Reaction at 60 and 80 °C. Cem. Concr. Res. 137, 106213. doi:10.1016/j.cemconres.2020.106213
Shi, Z., Ma, B., and Lothenbach, B. (2021). Effect of Al on the Formation and Structure of Alkali-Silica Reaction Products. Cem. Concr. Res. 140, 106311. doi:10.1016/j.cemconres.2020.106311
Sims, I., and Poole, A. B. (2017). Alkali-Aggregate Reaction in Concrete: A World Review. CRC Press. doi:10.1201/9781315708959
Souza, L. M. S., Polder, R. B., and Çopuroğlu, O. (2017). Lithium Migration in a Two-Chamber Set-Up as Treatment against Expansion Due to Alkali-Silica Reaction. Constr. Build. Mater. 134, 324–335. doi:10.1016/j.conbuildmat.2016.12.052
Stark, D., Morgan, B., and Okamoto, P. (1993). Eliminating or Minimizing Alkali-Silica Reactivity. Issue SHRP-C-343.
Stark, D. (1991). The Moisture Condition of Field concrete Exhibiting Alkali-Silica Reactivity. Spec. Publ. 126, 973–988.
Stark, D. C. (1992). “Lithium Salt Admixtures--An Alternative Method to Prevent Expansive Alkali-Silica Reactivity,” in The 9th International Conference on Alkali-Aggregate Reactions in Concrete, Westminster, London, July 27–31, 1017–1025.
Sun, G. K., Young, J. F., and Kirkpatrick, R. J. (2006). The Role of Al in C-S-H: NMR, XRD, and Compositional Results for Precipitated Samples. Cem. Concr. Res. 36 (1), 18–29. doi:10.1016/j.cemconres.2005.03.002
Szeles, T., Wright, J., Rajabipour, F., and Stoffels, S. (2017). Mitigation of Alkali-Silica Reaction by Hydrated Alumina. Transp. Res. Rec. 2629 (1), 15–23. doi:10.3141/2629-04
Tambelli, C. E., Schneider, J. F., Hasparyk, N. P., and Monteiro, P. J. M. (2006). Study of the Structure of Alkali-Silica Reaction Gel by High-Resolution NMR Spectroscopy. J. Non-Cryst. Sol. 352 (32–35), 3429–3436. doi:10.1016/j.jnoncrysol.2006.03.112
Tapas, M. J., Sofia, L., Vessalas, K., Thomas, P., Sirivivatnanon, V., and Scrivener, K. (2021). Efficacy of SCMs to Mitigate ASR in Systems with Higher Alkali Contents Assessed by Pore Solution Method. Cem. Concr. Res. 142, 106353. doi:10.1016/j.cemconres.2021.106353
Thomas, M., Hooper, T., and Stokes, D. (2000). “Use of Lithium Containing Compounds to Control Expansion in Concrete Due to Alkali-Silica Reaction,” in Proc. of 11th International Conference on Alkali-Aggregate Reaction, Quebec, Canada, June 11–16 (Quebec: Centre de Recherche Interuniversitaire Sur Le Beton), 783.
Thomas, M., Baxter, S., Stokes, D., and Hill, R. (2001). “The Combined Use of Fly Ash and Lithium Nitrate for Controlling Expansion Due to Alkali-Silica Reaction,” in International Center for Aggregates Research 9th Annual Symposium: Aggregates-Concrete, Bases and FinesInternational Center for Aggregates Research (ICAR), Austin, Texas, April 22–25 (University of Texas at Austin; Texas A&M University System; Aggregates Foundation for Technology, R, Final Draft).
Thomas, M. D. A., Fournier, B., Folliard, K. J., Ideker, J. H., and Resendez, Y. (2007). The Use of Lithium to Prevent or Mitigate Alkali-Silica Reaction in concrete Pavements and Structures. Virginia: Turner-Fairbank Highway Research Center.
Thomas, M. (2011). The Effect of Supplementary Cementing Materials on Alkali-Silica Reaction: A Review. Cem. Concr. Res. 41 (12), 1224–1231. doi:10.1016/j.cemconres.2010.11.003
Tremblay, C., Bérubé, M. A., Fournier, B., and Thomas, M. D. A. (2004a). “Performance of Lithium-Based Products against ASR: Effect of Aggregate Type and Reactivity, and Reaction Mechanisms,”. Suppl. Papers in Proceedings of the Seventh CANMET/ACI International Conference on Recent Advances in Concrete Technology, Las Vegas, USA, 26–29 May, 247–267.
Tremblay, C., Bérubé, M. A., Fournier, B., Thomas, M. D. A., and Stokes, D. B. (2004b). “Performance of lithium-based products against ASR: application to Canadian reactive aggregates, reaction mechanisms and testing,” in Proceedings of the 12th International Conference on Alkali-Aggregate Reaction (AAR) in Concrete, Beijing (China). Editors M. Tang, and Deng (October 15–19: International Academic Publishers, Beijing World Publishing Corp), 668–677.
Tremblay, C., Bérubé, M.-A., Fournier, B., Thomas, M. D. A., and Folliard, K. J. (2007). Effectiveness of Lithium-Based Products in concrete Made with Canadian Natural Aggregates Susceptible to Alkali-Silica Reactivity. ACI Mater. J. 104 (2), 195–205.
Tremblay, C., Bérubé, M. A., Fournier, B., Thomas, M. D., Folliard, K. J., and Nkinamubanzi, P. C. (2008). Use of the Accelerated Mortar Bar Test to Evaluate the Effectiveness of LiNO 3 against Alkali-Silica Reaction—Part 2: Comparison with Results from the concrete Prism Test. J. ASTM Int. 5 (8), 1–21. doi:10.1520/JAI101352
Tremblay, C., Bérubé, M. A., Fournier, B., Thomas, M. D., and Folliard, K. J. (2010). Experimental Investigation of the Mechanisms by Which LiNO3 Is Effective against ASR. Cem. Concr. Res. 40 (4), 583–597. doi:10.1016/j.cemconres.2009.09.022
Venkatanarayanan, H. K., and Rangaraju, P. R. (2014). Effectiveness of Lithium Nitrate in Mitigating Alkali-Silica Reaction in the Presence of Fly Ashes of Varying Chemical Compositions. J. Mater. Civil Eng. 26 (7), 4014021. doi:10.1061/(asce)mt.1943-5533.0000908
Vollpracht, A., Lothenbach, B., Snellings, R., and Haufe, J. (2016). The Pore Solution of Blended Cements: a Review. Mater. Struct. 49 (8), 3341–3367. doi:10.1617/s11527-015-0724-1
Wang, H., Stokes, D. B., and Tang, F. (1996). “Compatibility of Lithium-Based Admixtures with Other concrete Admixtures,” in Proceedings of the 10th International Conference on Alkali–Aggregate Reaction, Melbourne, Australia, August 18–23 (CSIRO Division of Building Construction and Engineering), 884–891.
Wijnen, P. W. J. G., Beelen, T. P. M., De Haan, J. W., Rummens, C. P. J., Van de Ven, L. J. M., and Van Santen, R. A. (1989). Silica Gel Dissolution in Aqueous Alkali Metal Hydroxides Studied by 29Si NMR. J. Non-Cryst. Sol. 109 (1), 85–94. doi:10.1016/0022-3093(89)90446-8
Yokoyama, T., Kinoshita, S., Wakita, H., and Tarutani, T. (1988). 27Al NMR Study on the Interaction between Aluminate and Silicate Ions in Alkaline Solution. Bcsj 61 (3), 1002–1004. doi:10.1246/bcsj.61.1002
Zapała-Sławeta, J., and Owsiak, Z. (2017). Effect of Lithium Nitrate on the Reaction between Opal Aggregate and Sodium and Potassium Hydroxides in concrete over a Long Period of Time. Bull. Polish Acad. Sci. Tech. Sci. 65, 773–778. doi:10.1515/bpasts-2017-0085
Zapała-Sławeta, J., and Owsiak, Z. (2018). The Use of Lithium Compounds for Inhibiting Alkali-Aggregate Reaction Effects in Pavement Structures. MS&E 356 (1), 12008. doi:10.1088/1757-899x/356/1/012008
Zhou, B., Mao, Z., and Deng, M. (2018). Reaction of Quartz Glass in Lithium-Containing Alkaline Solutions with or without Ca. R. Soc. Open Sci. 5 (9), 180797. doi:10.1098/rsos.180797
Zhou, J., Zheng, K., Liu, Z., Chen, L., and Lippiatt, N. (2019). Use of γ-Al2O3 to Prevent Alkali-Silica Reaction by Altering Solid and Aqueous Compositions of Hydrated Cement Paste. Cem. Concr. Res. 124, 105817. doi:10.1016/j.cemconres.2019.105817
Keywords: alkali silica reaction, supplementary cementitious materials, expansion, aluminium, lithium salts
Citation: Shi Z and Lothenbach B (2022) Role of Aluminum and Lithium in Mitigating Alkali-Silica Reaction—A Review. Front. Mater. 8:796396. doi: 10.3389/fmats.2021.796396
Received: 16 October 2021; Accepted: 09 December 2021;
Published: 05 January 2022.
Edited by:
Jie-Sheng Chen, Shanghai Jiao Tong University, ChinaReviewed by:
Linwen Yu, Chongqing University, ChinaCopyright © 2022 Shi and Lothenbach. This is an open-access article distributed under the terms of the Creative Commons Attribution License (CC BY). The use, distribution or reproduction in other forums is permitted, provided the original author(s) and the copyright owner(s) are credited and that the original publication in this journal is cited, in accordance with accepted academic practice. No use, distribution or reproduction is permitted which does not comply with these terms.
*Correspondence: Barbara Lothenbach, YmFyYmFyYS5sb3RoZW5iYWNoQGVtcGEuY2g=
Disclaimer: All claims expressed in this article are solely those of the authors and do not necessarily represent those of their affiliated organizations, or those of the publisher, the editors and the reviewers. Any product that may be evaluated in this article or claim that may be made by its manufacturer is not guaranteed or endorsed by the publisher.
Research integrity at Frontiers
Learn more about the work of our research integrity team to safeguard the quality of each article we publish.