- 1Department of Stomatology, Shandong Provincial Hospital Affiliated to Shandong First Medical University, Jinan, Shandong, China
- 2ACTV Research Group, Centre for Oral Health Research, Royal Dental Hospital, Melbourne Dental School, The University of Melbourne, Melbourne, VIC, Australia
- 3Centre for Oral Health Research, Royal Dental Hospital, Melbourne Dental School, The University of Melbourne, Melbourne, VIC, Australia
- 4Department of Biomedical Engineering, University of Melbourne, Melbourne, VIC, Australia
Vaccination is one of the most efficacious and cost-effective ways to protect people from infectious diseases and potentially cancer. The shift in vaccine design from disrupted whole pathogens to subunit antigens has brought attention on to vaccine delivery materials. For the last two decades, nanotechnology-based vaccines have attracted considerable attention as delivery vehicles and adjuvants to enhance immunogenicity, exemplified with the current COVID vaccines. The nanoparticle vaccines display unique features in protecting antigens from degradation, controlled antigen release and longer persisting immune response. Due to their size, shape and surface charge, they can be outstanding adjuvants to achieve various immunological effects. With the safety and biodegradable benefit of calcium phosphate nanoparticles (CaP NPs), they are an efficient carrier for vaccine design and adjuvants. Several research groups have studied CaP NPs in the field of vaccination with great advances. Although there are several reports on the overview of CaP NPs, they are limited to the application in biomedicine, drug delivery, bone regeneration and the methodologies of CaP NPs synthesis. Hence, we summarised the basic properties of CaP NPs and the recent vaccine development of CaP NPs in this review.
Introduction
In the last two decades the use of nanoparticle (NP)-based vaccines has emerged as a very promising strategy in vaccine development (Diaz-Arévalo and Zeng, 2020; Butkovich et al., 2021; Petkar et al., 2021; Zaheer et al., 2021). Nanoparticles are synthesised solid cores (particles) with a diameter ranging from 1 nm to 1,000 nm, and have been shown to have significant adjuvant effects as vaccine carriers and delivery vehicles (Peek et al., 2008a; Hamburg, 2012; Gregory et al., 2013; Bolhassani et al., 2014; Sun and Xia, 2016). Previous reports have shown that tumour antigens, targeting ligands, Toll-like receptor (TLR) ligands, and small molecule drugs can be loaded onto NPs for therapeutic applications (Sheen et al., 2014). The relatively small size of NPs allows them to penetrate host-cell walls (Kozlova et al., 2012). Additionally, NPs are easily taken up by antigen-presenting cells (APCs) through multiple phagocytic pathways, and consequently can induce both systemic and mucosal immunity depending on the vaccination site (Rotan et al., 2017). Antigenic material can be enveloped within NPs in order to protect the antigenic cargo from degradation at the site of vaccination, allowing the antigen to be delivered to immune cells such as APCs and subsequently leading to a long-lasting immune response (Bolhassani et al., 2014).
Of the different synthetic antigen delivery carriers currently available, calcium phosphate nanoparticles (CaP NPs) are one of the most promising vaccine transporters and have attracted increasing attention during the past decade (Habraken et al., 2016; Lin et al., 2017). As a natural component of the human body (in bones and teeth) (Habraken et al., 2016), CaP NPs have been studied in biomedical research since 1970 (Habraken et al., 2016; Sokolova and Epple, 2021; Sun et al., 2020) investigating DNA/gene silencing (Lee et al., 2014; Tang et al., 2015; Tang et al., 2018; Hosseini and Epple, 2021), drug delivery (Qi et al., 2013; Jun et al., 2013; Tsikourkitoudi et al., 2020), protein/peptide delivery (Kozlova et al., 2012; Rotan et al., 2017; Sokolova et al., 2017; Temchura et al., 2014a), dental implant and restorations materials (Wu et al., 2015; Zhang et al., 2016; Xu et al., 2011; Balhaddad et al., 2019; Zhou et al., 2020), imaging (Haedicke et al., 2015; Zhang et al., 2017), and bone tissue engineering (Kim et al., 2018; Liang et al., 2018; Levingstone et al., 2019; Sokolova et al., 2020). CaP NPs are non-toxic, biodegradable, cost-effective, and have pH-dependent solubility (Lin et al., 2017; Khalifehzadeh and Arami, 2020a). Most importantly, they provide protection to the antigen cargo from premature enzymatic and proteolytic degradation (Salem, 2015) and prevent elimination by the body (Sharma et al., 2015a; Posadas et al., 2016). Further, they can be functionalised with various molecular adjuvants to enhance active cell targeting and consequently the efficacy of vaccines (Xu et al., 2013; Zilker et al., 2016; Sokolova et al., 2010a; Xu et al., 2014) (Figure 1). Despite the well documented synthesis and applications of CaP NPs, particularly hydroxyapatite (HAP) (Alves Cardoso et al., 2012; Tabakovic et al., 2012; Jun et al., 2013; Lin et al., 2014; Sharma et al., 2015b), there are limited reports on CaP NPs in vaccine development (Lin et al., 2017; Masson et al., 2017). Thus, the aim of this review was to highlight the recent progress and challenges of using CaP NPs as vaccine adjuvants and delivery vehicles in vaccine formulations. To investigate this a systematic review of the literature was conducted using Scopus, Pubmed and Web of Science to identify publications primarily from 2010-2021 but including seminal papers from 2000 focusing on the use of Calcium Phosphate as a delivery vehicle and/or adjuvant for vaccine antigens and vaccine design. Our primary search terms were “calcium phosphate vaccine”; “calcium phosphate adjuvant”; “calcium phosphate nanoparticles or nanomaterials”.
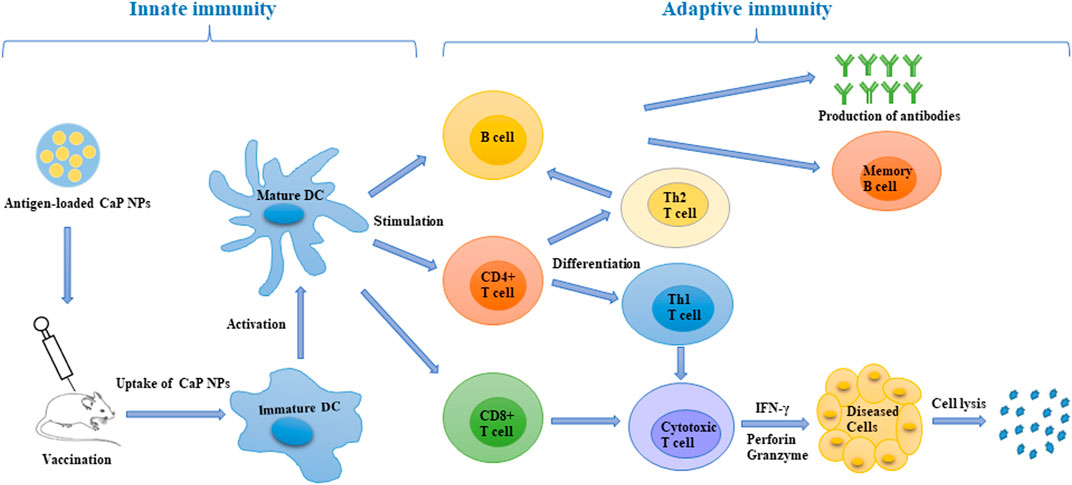
FIGURE 1. Schematic representation of the induction of an immune response after antigen-loaded CaP NP vaccination. CaP NPs can be functionalised with antigen and various molecular adjuvants to enhance active cell targeting. After vaccination, the CaP NP vaccine can be efficiently taken up and processed by DCs, then presented to T cells. This led to the induction of a robust innate and adaptive immune response with CD4+ T cells could differentiate into Th1 cells (drive cellular immunity) and Th2 cells (support humoral immunity). CD8+ T cells could differentiate into cytotoxic T cells, which are able to directly kill cancer and infected cells. DC, dendritic cell; Th, T helper cell; IFN-γ, interferon-γ.
The Importance of Adjuvants, Delivery System and TLR Ligands in Vaccine Design
Adjuvants, delivery systems and TLR ligands are considered three important components for an effective vaccine (Malyala et al., 2009). Non-living vaccine antigens, particularly recombinant subunit vaccines, are in general weakly immunogenic (Mohan et al., 2013). Natural/synthetic molecules such as alum and calcium mineral salts act as immune-stimulators, i.e., adjuvants, that induce the desired potent immune responses to the absorbed/conjugated antigen (Egli et al., 2014). An adjuvant is used as a component of the vaccine that can boost the immunogenicity of vaccine antigens (García and De Sanctis, 2014), while a delivery system ensures optimal delivery of antigen and adjuvant cargo to the desired site or immune cell (Skwarczynski and Toth, 2016). Vaccine adjuvant and delivery systems are not mutually exclusive, and can both act to prevent the antigen from degradation while it is carried to the desired site (Skwarczynski and Toth, 2016). Appropriate and optimised adjuvants play a central role in vaccine design as they can overcome immunosuppression and help induce a strong immune response (Kano et al., 2016).
At present, only a few adjuvants (such as mineral salts, aluminium salts (alum), calcium phosphate, and oil-in-water emulsions (MF59, AS03), cholesterol, phospholipid and polyethylene glycol (PEG) 2000 lipid) have been widely approved for human use (Del Giudice et al., 2018; Thi et al., 2021). These adjuvants are effective at inducing humoral immune response but, with the exception of calcium phosphate materials, have a poor ability to stimulate a cellular immune response, which is critical for viral and tumour eradication (García and De Sanctis, 2014; Skwarczynski and Toth, 2016). With the exception of calcium phosphate, they also have several drawbacks such as severe local tissue irritation, longer inflammatory response and induction of allergic responses in some people (Sharma et al., 2015a). Consequently, there is an urgent need for a novel delivery vehicle and adjuvant to increase cellular and humoral immunogenicity in vaccine design.
Various types of nanocarriers have been used for vaccination, and the most widely studied include polymeric NPs, lipid NPs, inorganic NPs (calcium phosphate, silicate, gold), and their applications in immunotherapy have been widely reviewed in the published literature (Peek et al., 2008b; Park et al., 2013; Smith et al., 2013; Zhao et al., 2014; Zhu et al., 2014; Irvine et al., 2015; Torres-Sangiao et al., 2016; Khalifehzadeh and Arami, 2020a; Mao et al., 2021). Compared with other polymeric NPs, poly (lactic-co-glycolic acid) (PLGA) NPs are the most extensively investigated NPs as vaccine carriers, largely because they have previously been approved by FDA and licensed for human use in medical applications such as bone implants and sutures (Pavot et al., 2014; Vartak and Sucheck, 2016). A number of studies have shown that PLGA NP are effective antigen delivery systems and when co-administered with an adjuvant can be effective vaccine formulations (Gu et al., 2019a; Gu et al., 2019b). However, PLGA NPs vaccines have a short half-life as they are often degraded rapidly, thus losing immunogenicity and effectiveness. Immunogenicity loss during storage has adverse effects on the encapsulated protein antigen that makes the vaccine less effective (Bolhassani et al., 2014). Gold NPs are also suggested as vaccine carriers as their surface can be highly modified with diverse ligands, they are biocompatible, physiologically stable, their size and shape can be easily controlled, and because they are easy to synthesis (Smith et al., 2015). However, there are several disadvantages that limit the potential use of Gold NPs as a cancer therapy. They are non-porous and non-biodegradable, and thus they are not suitable for the time-release of small molecules (Almeida et al., 2014). Although nanocarriers can enhance vaccine efficacy, there remain some potential problems that require further research to overcome.
The induction of a robust and specific cytotoxic T-lymphocyte (CTL) response by vaccination is a challenging goal in viral immunity and also cancer immunotherapy that has the added complication that the tumour microenvironment induces immunosuppression of T cells via a number of pathways (Butterfield, 2015). A key factor in enhancing the efficacy of a vaccine is to increase its binding to host cells and to deliver the antigen cargo to the endocytic pathway of APCs, thus initiating a strong immune response (Zhao et al., 2014). TLRs are a group of transmembrane protein complexes that recognise microbial components called pathogen-associated molecular patterns (PAMPs) in order to initiate an immune response (Akira et al., 2001). The use of TLR agonists as adjuvants has gained increasing interest due to their ability to improve the efficacy and immunogenicity of vaccines formulations (Hansen, 2011; Rahimian et al., 2015; Bruno et al., 2016; Gutjahr et al., 2016; Halliday et al., 2016; Zilker et al., 2016; Gutjahr et al., 2017; Kasturi et al., 2017; Sokolova et al., 2017). Pattern recognition receptors (PRRs), such as TLRs, are expressed on APCs (dendritic cells and macrophages) and mucosal/oral epithelial cells. PRRs play a significant role in inducing and enhancing both innate and adaptive immune responses and are thus being targeted in vaccine design and formulations (Halliday et al., 2016). TLRs facilitate the recognition of pathogens by immune cells, resulting in increased antigen uptake and processing, expression of pro-inflammatory cytokines, chemokines, and co-stimulatory molecules such as CD80, 86 and 40, leading to the induction of a robust innate and adaptive immune response (Blander and Medzhitov, 2006; Temizoz et al., 2016). Several different TLR ligands have been investigated for use as vaccine adjuvants, including ligands for TLR2, TLR3, TLR4, TLR5, TLR7, and TLR9 (Kawai and Akira, 2007; Coffman et al., 2010; Duthie et al., 2011), with the selection of the ligand often dependent on the disease being targeted (Kaur et al., 2018). One of the main challenges in cancer immunotherapy is the difficulty to abrogate the immunosuppressive tumour environment. However, immunosuppression can be overcome by co-immunising an antigen with a TLR ligand, with the benefit of combining mechanisms of up-regulating the production of co-stimulatory molecules, and induction of pro-inflammatory cytokines (Dewitte et al., 2014). Ligation of TLRs 2, 3, 4, 7, and 9 enhances dendritic cells (DCs) activation and antigen phagocytosis, which in turn improves CTL priming (Johnson et al., 2009; Watts et al., 2010). Moreover, the characteristics of CaP NPs and their immunostimulatory activity are controlled by different types of TLR molecular structure. The composition, backbone, sequencing, and concentrations of CpGs all influence the mineralization of CaP NPs (Khalifehzadeh and Arami, 2020b).
Compared with conventional adjuvants such as aluminium (alum) salts, CaP NPs display higher adjuvanticity and greater affinity to biological materials including proteins, cells and enzymes (Kalita et al., 2007). CaP materials used as adjuvants and have been shown to induce high titre immunoglobulin G1 (IgG1) to absorbed vaccine antigens for diphtheria, tetanus, pertussis, meningococcal, polio, rabies and hepatitis-B (Relyveld, 1986). In comparison with alum, CaP promoted a higher level of antibody response to Bothrops asper snake venom (Olmedo et al., 2014). CaP NPs were found to be more potent compared to alum for inducing a high IgG2a neutralising antibody against Epstein-Barr (EBV) infections (He et al., 2000). In addition, CaP NPs have been shown to be efficient mucosal adjuvants. He et al. reported that herpes simplex virus type 2 (HSV-2) vaccine using CaP NPs as an adjuvant elicited a stronger mucosal and systemic immune response when administered intravaginally and intranasally as compared to Alum (He et al., 2002). In a recent study, Cao et al. found that after 35 days immunization in mice, OVA administered orally via polymer-coated CaP nanocomposites significantly increased systemic and mucosal immune responses compared to antigen OVA alone in vivo (Cao et al., 2021). After 35 days, ELISA results demonstrated that mice vaccinated with CaP-based nanocomposites-OVA had considerably greater faecal antibody levels (about 1.57 fold higher than free OVA), suggesting that CaP has a significant impact on mucosal immunity (Cao et al., 2021). A study by Saeed et al. also showed that the human Enterovirus-71 vaccine encapsulated in CaP NPs had an enhanced antibody responses (IgA and IgG) in rabbits as compared to the standard vaccine (Saeed et al., 2015a). These studies suggested that CaP NPs have great potential as a vaccine adjuvant inducing cellular as well as humoral immunity (He et al., 2000; Lin et al., 2017). CaP, particularly in nanoform, has been suggested to be used instead of alum as a potent adjuvant (Johnson et al., 2020). In addition, CaP can be used as a nanocarrier for antigen delivery. Cao et al. demonstrated that when compared to naked OVA, oral administration of alginate-chitosan-coated CaP@OVA NPs dramatically increased mucosal IgA and serum IgG antibody responses, showing that the CaP-Chi-Alg NP might be a viable oral vaccine delivery strategy (Cao et al., 2020).
Synthesis of CaP NPs
Current strategies of CaP NP preparation are known to generate various sizes and shapes. Synthesis methodologies can be categorized into two groups, including the dry method (Zhang and Zhu, 2006; Fathi and Mohammadi Zahrani, 2009) and wet method chemical routes (Gentile et al., 2015; Sidane et al., 2015). More specifically, the dry method are involving solid-state (Zhang and Zhu, 2006) and mechanochemical (Fathi and Mohammadi Zahrani, 2009) techniques, while wet methods include chemical precipitation (Banik and Basu, 2014; Gentile et al., 2015; Andrade et al., 2016), sol-gel (Sidane et al., 2015), microemulsion (Saha et al., 2009; García et al., 2012; Yang et al., 2012; Hou et al., 2013), hydrothermal (Jin et al., 2015; Sarath Chandra et al., 2015), sonochemical (Utara and Klinkaewnarong, 2015), hydrolysis (Yang and Wang, 2016) and microwave methods (Hassan et al., 2016). Comparison of major methods for synthesis CaP NPs is summarised in Table 1. Various kinds of CaP NPs can be obtained via the different fabrication methods, such as particle size (10 nm–10 mm), size distribution (from narrow to broad), shape (from spherical to rod-shaped), crystallinities (low to high) and compositions (all CaP NPs forms).
Properties of CaP NPs
Safety, Biocompatibility, and Stability
Calcium phosphate is a natural component of the human body, and CaP NPs are reported to be biocompatible, bioresorbable and safe materials and have been shown to have no side effects as a vaccine adjuvant (Goto et al., 1993; He et al., 2000; Viswanathan et al., 2014) and their in vivo use is approved by the FDA (Dorozhkin, 2006). A phase I study revealed that when administered subcutaneously in healthy volunteers, CaP was found to be safe and no toxic effects were induced (Peek et al., 2008b). Viswanathan et al. demonstrated that CaP NPs are suitable for biological applications as CaP NPs did not show any significant cytotoxicity on peripheral blood mononucleated cells (PBMCs) by MTT (a cell proliferation assay) assay (Viswanathan et al., 2014). He et al. also showed that CaP NPs elicited nearly no inflammatory response at the injection site, no local tissue irritation and induced no allergic reaction (He et al., 2000).
In vivo stability of CaP is dependent on the stability of CaP in a biological matrix (Sharma et al., 2015a). CaP stability varies with both the calcium to phosphate ratio in the material and the pH of their environment. Hydroxyapatite (Ca10 (PO4)6(OH)2, Ca/P = 1.67), which is a naturally occurring form of calcium phosphate, has the greatest stability and is known to be quite stable both in vitro and in vivo (Chen et al., 2011). In vitro studies showed that the solubility of CaP decreased as the pH increased from 6.2 to 7.2 (Klein et al., 1990). CaP are stable at human blood (pH 7.4) and their storage stability is reported to be excellent as they are very poor microbial substrates (Sharma et al., 2015a).
CaP NPs have excellent biocompatibility compared to other NPs because they mimic naturally occurring calcium phosphate found in the human body such as teeth (enamel, dentine) and bones. They are well-tolerated and are not recognised as foreign materials by the immune system. CaP NPs’ biocompatibility is also associated with the surface charge of the particles. Chen et al. reported that positively charged HAP has better cytocompatibility compared to negatively charged HAP towards MC3T3-E1 cell lines (osteoblasts) based on MTT and LDH (lactate dehydrogenase, an indicator of cellular toxicity) assays (Chen et al., 2011). The biodegradability of CaP has been shown using HeLa cells (human epithelial cervical cancer cells) that quickly endocytose CaP NPs (diameter 120 nm) (Sokolova et al., 2013), rapidly dissolving in lysosomes (Liu et al., 2014) and the calcium ions actively secreted out of the cell (Dorozhkin and Epple, 2002).
CaP NPs pH-Dependent Solubility
CaP solubility is pH-dependent, it is stable at human blood pH of 7.4 and more alkaline environments but easily dissolves at low pH’s of <5.0. This physical property has specific advantages as a low pH is often found in tumour zones, endosome and lysosome, thus making CaP very suitable for delivery systems of drug and vaccine applications (Sokolova and Epple, 2021). Figure 2 shows a schematic representation of CaP NP cargo release in a low pH environment such as within an endosome/lysosome or at a tumour site. First, CaP NPs enter the tumour site or are endocytosed by the cell. Within the low pH environment of the endosome or tumour site the CaP NPs dissolve and disassemble releasing the cargo at the tumour site or within the endosome. It is proposed that the release of Ca2+ and PO43- ions from the dissolved NPs within the endosome gives rise to high osmotic pressure, causing the endosome to expand and then rupture, releasing the antigen cargo, Ca2+, and PO43- ions into the cytoplasm (Li et al., 2012) (Figure 2). This proposed mechanism is used as an explanation as to why CaP has the ability to act as an adjuvant to induce a cytotoxic T cell response.
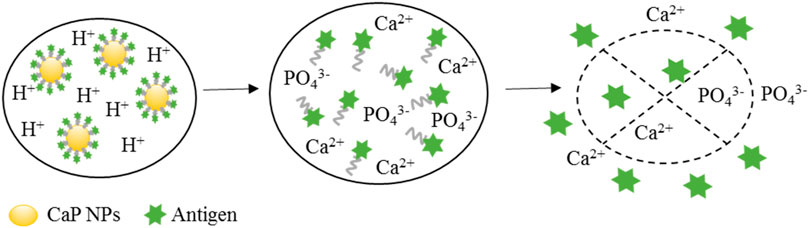
FIGURE 2. The release process of antigen entrapped in antigen-coated CaP NPs in the endosome. There are three steps involved in CaP NP cargo releasing mechanism. CaP NPs can be initially endocytosed by the cell and enter the endosome. Then, the CaP NPs disassemble and dissolve in presence of the low pH environment in the endosome. The released Ca2+ and PO43- ions from the dissolved NPs can give rise to high osmotic pressure, causing the endosome to expand and rupture releasing the antigen cargo into the cytoplasm, where it would then be processed into the MHC class I pathway.
Surface Modification
It is well known that physicochemical properties such as size, shape and surface charge have a crucial role in the efficacy of vaccines (Zhao et al., 2014). Due to the importance of control of NP size and shape in vaccine formulations, different synthetic protocols have been developed to regulate the size and shape of CaP NPs (Sadat-Shojai et al., 2013). For example, Yan et al. (2006) showed that hydrothermal crystallisation of Ca(NO3)2 and (NH4)2HPO4 in the presence of the poly (amidoamine) (PAMAM) dendrimers demonstrating that the size of CaP NPs fabricated decreased from 82, 77, 48 to 38 nm with increasing PAMAM dendrimer generation (G1.0 to G4.0, respectively). Hydrothermal crystallisation of Ca/P using CTAB (cetyltrimethylammonium bromide) as a template was found to result in the size and shape of the formed CaP NPs being able to be controlled by modifying the pH and reaction temperature (Wang et al., 2006).
The surface modification with either hydrophobic or hydrophilic molecules can enhance the versatility of CaP NPs in vaccine applications (Sharma et al., 2015a). The surface of HAP possesses positively charged Ca2+ and negatively charged PO43- sites that provide binding sites for stabilisation as well as bio-conjugation to agents such as polymers (Kandori et al., 2009). For instance, polyethyleneimine (PEI) and poly (ethylene glycol) (PEG) have been added to the surface of formed CaP NPs to increase stabilisation in the physiological environment as well as altering the charge of the NP surface (Ramachandran et al., 2009; Kozlova et al., 2012). Furthermore, the polymers provide a chemical moiety such as an -NH2, -OH or -COOH for conjugation or adsorption of additional agents on the NP surface such as peptide/protein, drugs, DNA/siRNA, or TLR ligands during the fabrication process, thus enhancing the scope of use and immunogenicity (Saeed et al., 2015b). This ability to use the layer-by-layer assembly approach with CaP was used in a multi-shell system to protect biomolecules (antigen and immunostimulants) within from degradation, increase transfection efficiency and targeting to B-cells (Temchura et al., 2014a).
CaP Adjuvanticity
CaP adjuvants, first reported by Relyveld and his colleagues in 1964 (Relyveld et al., 1964), have been used for many years as vaccine adjuvants (Relyveld et al., 1985). CaP adjuvants have been used in diphtheria, pertussis, tetanus and poliomyelitis vaccines in France (Coursaget et al., 1986; Gupta and Siber, 1995; Goto et al., 1997). Several recent papers have highlighted that CaP material should be considered as a replacement of alum salts in vaccine formulations (Sesardic et al., 2007; Issa et al., 2014) due to their distinct advantages in that they are non-toxic, biodegradable, have a low production cost, and have pH-dependent solubility and unlike alum can be readily produced as NPs (Lin et al., 2017). Additionally, CaP adjuvants have been shown to provide protection to the antigen cargo from premature enzymatic and proteolytic degradation (Salem, 2015) and prevent elimination by the reticulohistocytic system (RHS) (Posadas et al., 2016). Another advantage of CaP materials is that they can be functionalised with various molecular adjuvants to enhance active cell targeting and vaccine efficacy (Xu et al., 2013; Xu et al., 2014; Zilker et al., 2016). Due to these properties, CaP nanoparticles have high adjuvanticity and show considerable promise as a vaccine adjuvant.
The Current Strategies for Antigen Loading Onto CaP NPs
In order for NPs to act as effective vectors for the delivery of antigens to the desired site, a strong interaction between NPs and the antigen is required. Currently, there are four approaches to attach antigen on NPs: adsorption; encapsulation; co-mixtures; and chemical conjugation (Zhao et al., 2014) (Figure 3). The advantages and disadvantages of antigen interaction strategies with CaP NPs are summarized in Table 2. Among these, adsorption and encapsulation are the most widely used strategies for antigen loading on CaP NPs, which can be achieved by charge attraction and hydrophobic interaction (Zhao et al., 2014). A draw back of the adsorption method is that it is a weak interaction effected by salt concentrations, and exogenous lipids and proteins displacing the antigen at the site of injection, which can lead to a burst release of antigen from NPs in vivo or sudden release away from the APC thus reducing immunity (Kamaly et al., 2016). Electrostatic attraction between antigen and CaP NPs plays the most important role in surface adsorption. For example, the adsorption of bovine serum albumin (BSA), ovalbumin (OVA) or lysozyme on HAP arises from electrostatic interaction between Ca2+ and PO43− anions of HAP with the COO− and NH4+ cations of the protein antigen (Boonsongrit et al., 2008; Dasgupta et al., 2009; Kandori et al., 2009; Sahdev et al., 2013; Swain and Sarkar, 2013; Ma et al., 2016).
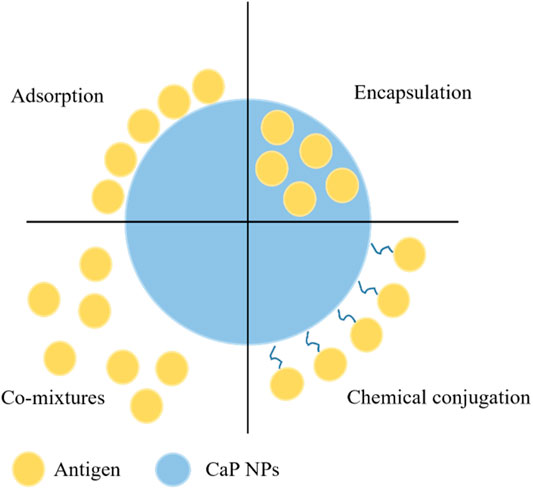
FIGURE 3. The current strategies of antigen interaction with CaP NPs through conjugation, encapsulation, adsorption or simple mixing.
Encapsulation and conjugation can be an appropriate alternative to achieve steady antigen release and delivery of all of the antigen to the APC. In general, encapsulation can be achieved by antigens mixed with chemical reagents and solvents during NP synthesis route, resulting in interaction that is strong, similar to that of chemical conjugation. This method can enhance antigen transfection efficiency as the antigen is not released until the dissolution of NPs, thus delivering the antigen at the desired site. Dasgupta et al. encapsulated BSA protein by first incorporating it with the Ca2+ solution prior to mixing with the PO43− aqueous solution during a precipitation synthesis, resulting in 50–60 nm NPs containing up to 24% by weight BSA protein (Dasgupta et al., 2010). Similarly, Li et al. used the water-in-oil micro-emulsion method to incorporate siRNA in CaP NPs, by adding the siRNA to the PO43− aqueous solution phase, this resulted in nanoparticles of 60–80 nm in diameter with an 39.8 ± 2.8% encapsulation efficiency of siRNA (Li et al., 2010). Chiu et al. (2012) used a hybrid-encapsulating stabilisation process whereby the antigenic protein was expressed with peptides that bound to and stabilised calcium phosphate. In this method the protein was mixed with the PO43− aqueous solution phase and upon addition of the Ca2+ phase the protein stabilised the calcium-phosphate nucleation to form nanoparticles (60–70 nm) with an amorphous calcium phosphate core with a protein shell.
Chemical conjugation has also been used to incorporate antigenic molecules such as proteins and peptides by covalently linking them to the surface of the NP (Slütter et al., 2010). Ramachandran et al. functionalised the surface of CaP NPs using carbodiimide chemistry to covalently link diamino-PEG and then loaded the PEG-CaP particles with insulin for an oral delivery therapeutic (Ramachandran et al., 2009). In another approach Kozlova et al. synthesised CaP NPs coated with a silica shell that was functionalized by thiol/amino groups or salinization, to covalently attaching antibodies and antigens (Kozlova et al., 2012). The approach of antigen-conjugated NPs has some significant advantages for mucosal immunisation as the conjugated antigen has been found to be protected from proteases in the mucosal tissue (Navarro-Tovar et al., 2016). CaP NPs act as an immune potentiator (adjuvant) as well as a delivery system and conjugation between antigens, adjuvants and NPs has been shown to give better immunity in comparison to a mixed solution of the formulation (Nembrini et al., 2011; Zhao et al., 2017). A Hen Egg Lysozyme (HEL) and TLR9 ligand CaP NP conjugated was found to elicit a significantly higher humoral immune response than non-conjugated NPs (Zilker et al., 2016).
CaP NPs Uptake and Immunogenicity
CaP NPs Cellular Uptake
The effective uptake of NPs by DCs is crucial to induce a protective immune response. By using CaP NPs as adjuvants and antigen carriers, the efficiency of delivering antigen to DCs has been shown to be greatly enhanced compared with antigen alone (Dorozhkin, 2006). As previously stated, the ability of NP uptake is influenced by particle size, shape, surface charge, and composition. Elucidating the effect of these parameters is essential for the development of effective, novel NP vaccines.
It has been widely accepted that the size of NPs plays a critical role in vaccine immunogenicity (He et al., 2010; Knuschke et al., 2014a; Sharma et al., 2015b; Hayashi et al., 2016). Foge et al. have shown that size is the most critical factor that affects NP uptake (Hayashi et al., 2016). At a size range of 20–100 nm, NPs can easily enter lymph system and home to lymph nodes (Knuschke et al., 2014b). Once localised in the lymph nodes, NP-released antigens can directly activate B cells and be effectively phagocytosed by DCs, resulting in the induction of both long-lasting cell-mediated and humoral immunity. Larger NPs (∼200 nm) that do not readily enter the lymph system are efficiently taken up by cells (DCs) at a distal site (injection site) via endocytosis or micropinocytosis before the DCs migrate to the lymph nodes and inducing a robust immune response (Knuschke et al., 2014b). In vitro studies have suggested that NPs of a size below 500 nm are optimal for DC uptake (Foged et al., 2005) and nano-sized particles have been shown to readily penetrate cells and induce higher immune responses (Sharma et al., 2015a). Studies have found that tumour-associated antigen conjugated to nano-rather than micron sized particles elicited higher antibody responses which significantly inhibited the generation of human gastric cancer cells (Li et al., 2008; Hou et al., 2009; Saeed et al., 2015b). Similarly, a recent study compared different sized CaP NPs, and the size range between 100 and 400 nm was found to elicit significantly stronger antibody responses compared to smaller or larger particles (Hayashi et al., 2016).
Apart from size effects, the surface charge of NPs has also been shown to significantly effect immune responses. It has been reported that positively charged CaP NPs are more readily taken up by cells than those with a negative or neutral charge (Chen et al., 2011). The possible reason may be due to the negatively-charged cell membrane, and thus the NPs and cell are electrostatically attracted to each other (Chen et al., 2011). In addition to size and surface charge, particle shape plays a significant role in the stimulation of immune responses (Hu et al., 2007; Ramesh et al., 2007; Hayashi et al., 2016). Ramesh et al. observed that knife-shaped CaP NPs are more stimulatory than both plate-like and spherical NPs (Ramesh et al., 2007). Rod-shaped CaP NPs generated greater IL-1β production in vitro than spherical NPs, but both induced similar humoral immunity to attached antigen in vivo (Hayashi et al., 2016). Cell adhesion and proliferation may also be influenced by the composition of NPs. Hu et al. observed that compared with amorphous calcium phosphate (ACP), more bone marrow mesenchymal stem cells adsorb and proliferate on HAP film and that cellular differentiation was significantly promoted by HAP, indicating the CaP NPs that are HAP rather than ACP or other calcium/phosphate forms are more immunostimulatory (Hu et al., 2007). The cellular uptake and subsequent immunity developed from that, for different size, shape and formulation of CaP NPs is divergent and it remains that the antigen being delivered also plays a significant role in the immune response elicited. An advantage of CaP nanomaterials is that they are cheap, and their formulations easily modified, thus allowing different particles to be used as delivery/adjuvants for the antigen and to investigate which targets the cargo to the desired immune cell which is rarely done in the field.
Targeting the Innate Immune System
The innate immune system plays a significant role in the induction and development of adaptive immunity to an antigen (Iwasaki and Medzhitov, 2010). It is well established that when the innate immune response is triggered by microbial molecules it can induce and recruit the cellular components of the adaptive immune system and aid in development of immune memory (Medzhitov, 2007). Pattern-recognition receptors (PRRs) on the surface of APCs (particularly DCs) recognise microbial associated molecules, which results in the induction of inflammatory responses and induction of adaptive immune responses (Iwasaki and Medzhitov, 2015). In the last decade, the adjuvant effect of CaP NPs on the innate immune system has been investigated (Kozlova et al., 2014a). Behera et al. demonstrated that Labeo rohita H. S-layer protein (of Aeromonas hydrophila) loaded CaP NPs elicited a robust innate and adaptive immune responses in a fish model following parenteral immunisation (Behera and Swain, 2011). Further, the antigen loaded CaP NPs induced significantly stronger respiratory burst and myeloperoxidase activity compared with negative control groups (Behera and Swain, 2011). Matesanz et al. reported that BSA loaded nano-sized CaP produced a rapid stimulation of the innate immune response in mice (Matesanz et al., 2014). Further, macrophage proliferation and phagocytic activity were greatly decreased, while the generation of inflammatory cytokines such as IL-6, TNF-α was strongly enhanced (Matesanz et al., 2014). Tai et al. found that β-tricalcium phosphate (β-TCP) may also have an immunostimulatory effect on macrophages. Mice injected with β-TCP NPs elicited extensive migration of mouse monocyte/macrophage cells to the injection site, and that it significantly enhanced the production of macrophage inflammatory protein 1 alpha (MIP-1α), suggesting a novel role for β-TCP in vaccine applications (Tai et al., 2014).
As mentioned previously, the innate immune system depends on the recognition of pathogen-associated molecular patterns (PAMPs) by PRRs such as TLRs. TLR activation can elicit inflammatory cytokine production, enhance macrophage phagocytosis activity, and enhance antigen presentation (Krutzik et al., 2005; Moresco et al., 2011). Consequently, TLR agonists in vaccine development have been widely investigated in preclinical studies (Luo et al., 2015). Sokolova et al. evaluated the adjuvant effect of CaP encapsulated with TLR ligands CpG and polyriboinosinic: polyribocytidylic acid (poly (I: C)) in a mouse model. They showed that TLR functionalized NPs elicited high levels of the innate inflammatory cytokines IL-12p70 and TNF-α, which resulted in DC maturation and rapid CD4+ T cell activation (Sokolova et al., 2010a). Similarly, other researchers have developed a flagellin-functionalized CaP NPs and studied the immunostimulatory effect on the innate immunity both in vitro and in vivo in mice (Kozlova et al., 2014b). Flagellin is a PAMP that can be recognised by TLR5 on the host cell surface (Hayashi et al., 2001). Compared to free flagellin, flagellin-functionalized CaP NPs produced a high-level of the proinflammatory cytokines IL-1β from bone marrow-derived macrophages (Kozlova et al., 2014a). These findings suggest that CaP NPs could effectively activate the innate immune system and that TLR ligand functionalisation enhances this ability. These key findings should be taken into account in designing CaP NP vaccine formulations, as the addition of TLR ligands will have not only an additional adjuvanticity effect but also a “depot” effect allowing the vaccine to be located in a set area, the ‘injection’ site long enough for dendritic cells and other antigen presenting cells to sequester the vaccine. Furthermore, CaP can be formulated in a number of different ways depending on the Ca to P ratio and this as highlighted above can have significant impact on immunogenicity. Thus although, hydroxyapatite maybe the most stable CaP formulation other CaP formulations such as β-TCP need to be considered.
Targeting the Adaptive Immune System
Effects of CaP NPs on Humoral Immunity
The capability of vaccines to induce humoral immunity plays an essential role in the elimination of many microbial infections. Some earlier studies have shown that CaP NPs can elicit stronger and longer-lived antibody responses (IgG, IgG1) when compared to traditional adjuvants such as aluminium salts (He et al., 2000; Goyal et al., 2009; Behera and Swain, 2011; Sahdev et al., 2013). Viswanathan et al. developed a Newcastle disease virus (avian avulavirus 1)-conjugated CaP NP vaccine and evaluated its efficacy in chickens (Viswanathan et al., 2014). They found that the vaccine induced higher and earlier humoral immunity (IgA antibody) than live-attenuated viral commercial vaccines (Viswanathan et al., 2014). In a separate study CaP NP were used to encapsulated a DNA vaccine to foot and mouth disease virus that was found to elicit a strong humoral immune response (neutralizing antibodies) providing protection for mice and guinea pigs upon infection with live virus (Joyappa et al., 2009). In a recent study, Temchura et al. developed and tested CaP NPs functionalized with Hen Egg Lysozyme (HEL), which effectively targeted and activated B cells in vitro and in vivo using BCR-transgenic B-cells from SW-HEL mice as a model. These NPs significantly increased the expression of B cell activation markers and were 100 times more effective in the maturation of B cells than soluble antigen protein (Temchura et al., 2014b). Similar studies have shown that nano-sized CaP coated with an inactivated human enterovirus-71 virus (HEV-71) led to a higher antibody response (IgM and IgG) than the unadsorbed vaccine alone or micro-sized CaP NPs in rabbits via intradermal injection (Saeed et al., 2015b). Moreover, Chua et al. developed a novel vaccine that used OVA as a model antigen and chitosan and HAP NPs as the delivery vehicles. After immunisation with a single dose of vaccine, a strong antibody response (IgG1) was induced in mice, which persisted for 12 months (Chua et al., 2015). Additionally, Zilker et al. (Zilker et al., 2016) optimised the administration route of CaP-HEL NPs without additional adjuvants and demonstrated that intramuscular immunisation elicited a higher antibody response (IgG) in comparison to the monovalent antigen. In this report, the authors also compared different types of TLR ligands for CaP-HEL, and reported that TLR ligands loaded with CaP-HEL gave higher IgG antibody levels than when CaP-HEL was used without TLR ligands. This study demonstrated that CaP-HEL NPs functionalised with different TLR ligands modulated the IgG isotype response, and the production of mucosal IgA antibodies. A novel CaP vaccine loaded with three Brucella antigens (FliC, 7α-HSDH, BhuA) and two multi-epitopes (poly B and poly T) was found to induce a significantly enhance the cellular and humoral immune responses to the antigens. Furthermore, the ratio of antigen specific IgG2a to IgG1 was increased (Sadeghi et al., 2020). Recently, Dodangeh et al. designed a novel multi-epitope (MIC3, ROP8, and SAG1) vaccine with CaP adjuvants. They found that compared to control mice, animals vaccinated with MRS-CaP vaccine elicited greater humoral (IgG1 and IgG2a) and T helper type 1 cell-mediated immunological responses (Dodangeh et al., 2021). Taken as a whole, these findings demonstrate that CaP NPs loaded with antigens and TLR ligands are promising vaccine formulations for effective induction and modulation of an antibody response.
Effects of CaP NPs on T Cell-Mediated Immunity
A potent T cell immune response is indispensable to clearing and recovering from infectious disease. There are several studies that have demonstrated that CaP NPs loaded with viral and bacterial antigens are able to activate protective T cell responses against viral and bacterial infections (Sokolova et al., 2010b; Xu et al., 2013; Zhou et al., 2014; Rojas-Sánchez et al., 2020). A novel HAP vaccine loaded with malaria antigen (merozoite surface protein-119, MSP-119) was found to elicit a vigorous T cell response and secretion of IL-2 and IFN-γ in mice (Goyal et al., 2009). In a separate study hemagglutinin (HA) loaded CaP NPs functionalised with TLR9 ligand (CpG) were found to induce DCs maturation and a strong antigen specific CD4+ T cell proliferative response (Sokolova et al., 2010a). In a following study it was found that immunisation of mice with TLR functionalized CaP NPs were effectively taken up by DCs as well as inducing high production of CD4+ and CD8+ effector T cells (Knuschke et al., 2013). Others have reported that CaP loaded with model antigen ovalbumin (OVA), combined with a fusion protein induced a robust and long-lasting CD8+ T cell responses in mice that were characterised by the high production of IFN-γ and TNF-α (Zhou et al., 2014). Interestingly it has been shown that magnesium (Mg) and zinc (Zn) loaded HAP NPs could effectively promote Th1 and Th2 immunity in vitro, and enhance the secretion of type 1 and 2 cytokines from bone marrow dendritic cells (BMDCs), suggesting these mixed ion (Zn, Mg) CaP particles can be used as human adjuvants (Wang et al., 2016). Furthermore, in a recent study, CaP NPs encapsulating the TLR9 ligand, CpG, combined with vial peptides from murine leukaemia Friend retrovirus (FV) were shown strongly enhanced anti-viral immunity (Knuschke et al., 2016). Li et al. developed a CpG-functionalized silica-coated CaP NP (SCP) vaccine and evaluated its effectiveness against human immunodeficiency virus type 1 (HIV-1) infection (Li et al., 2021). When compared to naked DNA vaccination, SCP-based DNA immunisation was found to generate significantly broader humoral and a more robust cellular immune responses in vivo (Li et al., 2021).
These studies indicated that different kinds of adjuvant used to functionalise or coat CaP NPs can strongly affect T-cell immunity. Knuschke et al. has compared CpG and poly (I:C) functionalized NPs with CaP NPs in vitro, and observed that CpG-CaP NPs elicited a more robust T cell-mediated immunity as compared to the other functionalised and bear CaP NPs (Knuschke et al., 2014c). In another study, CaP NPs encapsulating both CpG and poly (I:C) ligands and the antigen hemagglutinin were found to elicit DC maturation and T cell activation significantly (Sokolova et al., 2010b) (Figure 4). Sokolova et al. demonstrated CaP NPs functionalised with TLR ligand poly (I:C) resulted in a pronounced immunostimulation to liver cells both in vitro and in vivo (Sokolova et al., 2017). From these studies it appears that combining TLR ligands targeting TLR9 and TLR3 singularly or together in a CaP NP formulation enhances CTL immunity by aiding DC targeting and CD4+ and CD8+ T cell activation. However, these are only two TLR ligands and vaccine formulations, other than CaP NPs have also used ligands for other pattern recognition receptor’s such as TLR2 (Palmitoyl moieties), TLR4 (LPS), TLR5 (flagellin) and NOD1/2 (peptidoglycan) that can be used in future CaP NP studies to enhance targeting, activation and to direct immunity to the desired outcome.
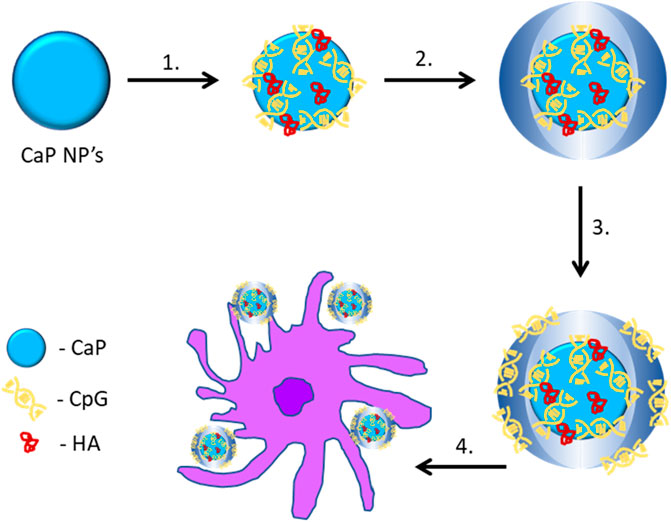
FIGURE 4. Triple-shell calcium phosphate nanoparticles loaded with an antigen and a TLR ligand (CpG or poly (I:C)) for cellular uptake. 1) Functionalisation of the CaP NPs with CpG and HA to form single-shell nanoparticles. 2) Calcium and phosphate solution added to form double-shell nanoparticles. 3) CpG or poly (I:C) added to form triple-shell nanoparticles. 4) CaP NPs phagocytosed by dendritic cells (Sokolova et al., 2010b). CaP, calcium phosphate; HA, hemagglutinin.
The potential of CaP as a vaccine carrier and adjuvant has been further explored in cancer vaccines (Xu et al., 2014; Zhao et al., 2015; Khalifehzadeh and Arami, 2020a; Scheffel et al., 2020). It is known that the induction of a potent CTL response is crucial for the eradication of cancer cells and prevent cancer recurrence (Rosenberg et al., 2004; Thomas and Prendergast, 2016). Xu et al. studied the effect of NPs on CTL responses in a melanoma mouse model of cancer. Prior to the induction of the disease, mice were immunised with either free melanoma antigen (Tyrosinase-related protein 2, p-Trp2), or free TLR 9 ligand (CpG), or NPs loaded with lipid-calcium-phosphate (LCP) NPs containing p-Trp2 peptide and TLR 9 ligand (Trp2-TLR LCP) (Xu et al., 2013). They showed the mice vaccinated with the Trp2-TLR LCP induced a robust and specific IFN-γ CTL response in vivo which inhibited tumour growth and lung metastasis (Xu et al., 2013). However, the vaccine was found to be less effective in a late-stage melanoma, despite a robust CTL response due to the immune suppressive tumour microenvironment. To overcome this a novel LCP system was trialled that incorporated Transforming Growth Factor-β (TGF-β) siRNA to contribute to tumour immunosuppression. This approach resulted in decreasing levels of TGF-β in the tumour microenvironment and a significant inhibition of tumour growth with increased levels of CD8+ T cells compared with vaccine formulations without the TGF-β siRNA (Xu et al., 2014). The anti-tumour effect on advanced stage melanoma has also been improved by a combination of chemotherapeutic agents and LCP (Zhao et al., 2015). Additionally, combinatorial immunotherapy can enhance anticancer efficacy. In a recent study lipid-dendrimer-CaP NPs functionalised with siRNA, plasmid DNA and a hepatocellular carcinoma-targeting peptide were found to increase tumoral infiltration, elicit a strong CD8+ T cell response, and suppress hepatocellular carcinoma progression (Huang et al., 2020). Sun et al. formulated mannose-and bisphosphonate (BP)-modified CaP NPs loaded with OVA-encoded plasmid DNA. They found that in C57BL/6J mice, MB-CaP-OVA vaccination greatly suppresses the development of OVA-expressing E.G7 tumour cells (Sun et al., 2021a). Another type of CaP, β-TCP, has also shown anti-tumour activity in vivo and has immune-stimulatory effects on immune cells (Naito et al., 2010). Tai et al. studied the in vivo and in vitro effect of β-TCP in C57bl/6 mice and a macrophage cell lines (J774A.1 cells), respectively. They found that vaccination with β-TCP elicited substantial migration of abundant neutrophils to the injection area in immunocompetent B6 mice. Additionally, β-TCP elicited upregulation of surface marker (CD86) expression and secretion of TNF-α and MIP-1α in mouse monocyte/macrophage cells, indicating the different forms of calcium/phosphate may have significant effects on the immune system and the response induced (Tai et al., 2014).
Conclusion
In conclusion, the interaction between CaP NPs and the immune system has attracted a high level of interest in the last two decades. As demonstrated by the numerous examples presented in this review (Table 3), CaP NPs can be used as an effective vaccine adjuvant and delivery vehicles for vaccine formulations to achieve immunity. CaP NPs are non-toxic, biodegradable, cost-effective, and have pH-by altering their physicochemical properties such as size, shape and surface charge (Chiu et al., 2012; Hayashi et al., 2016). Additionally, CaP NPs can be functionalised with various molecular adjuvants to enhance active immune cell targeting and consequently the efficacy of vaccines (Xu et al., 2013; Zilker et al., 2016). CaP NPs have also been shown to be efficient mucosal vaccine adjuvants to enhance immunity (He et al., 2002). Further, CaP NPs can be modified with either hydrophobic or hydrophilic molecules to deliver peptide, protein or DNA vaccine cargo and thus have great potential as a universal adjuvant as they are able to elicit humoral and cellular immunity (Saeed et al., 2015b). Although CaP NPs are an attractive material for vaccine development, there are currently several challenges that have limited their clinical uptake (Tsiourvas et al., 2011). The major ones being typically low antigen loading capacity and rapid aggregation of CaP NPs (Huang et al., 2017). These issues are currently being investigated and in a recent study, adenosine triphosphate (ATP) was used as a dual functional agent to stabilise CaP and act as an adjuvant resulting in ATP-modified CaP NPs were found to be an effective nano-delivery method and nano-adjuvant for cancer DNA vaccinations (Sun et al., 2021b). Even though there are some challenges for NPs to be used as vaccine vehicles, the use of CaP NPs will be a promising new platform and carrier for developing effective vaccines against cancer and infectious diseases.
Author Contributions
Conceptualization, ZS and NO’B-S, writing and original draft preparation ZS; writing—review and editing ZS, NO’B-S, JH, JL, WL, MM, and AO’C supervision of ZS by NO’B-S, JH, JL, WL, MM, and AO’C. All authors have read and agreed to the published version of the article.
Funding
The National Health and Medical Research Council (NHMRC) of Australia and Australian Research Council (ARC) are thanked for financial support over many years for the peptide chemistry and chemical biology studies reported in the authors’ laboratories. NO’B-S is the recipient of NHMRC funding (APP1142472, APP1158841, APP1185426), ARC funding (DP210102781, DP160101312, LE200100163), Cancer Council Victoria funding (APP1163284) and Australian Dental Research Funding in antimicrobial materials and research is supported by the Centre for Oral Health Research in the Basic and Clinical Oral Sciences Division at The Melbourne Dental School.
Conflict of Interest
The authors declare that the research was conducted in the absence of any commercial or financial relationships that could be construed as a potential conflict of interest.
Publisher’s Note
All claims expressed in this article are solely those of the authors and do not necessarily represent those of their affiliated organizations, or those of the publisher, the editors and the reviewers. Any product that may be evaluated in this article, or claim that may be made by its manufacturer, is not guaranteed or endorsed by the publisher.
References
Akira, S., Takeda, K., and Kaisho, T. (2001). Toll-like Receptors: Critical Proteins Linking Innate and Acquired Immunity. Nat. Immunol. 2 (8), 675–680. doi:10.1038/90609
Almeida, J. P. M., Figueroa, E. R., and Drezek, R. A. (2014). Gold Nanoparticle Mediated Cancer Immunotherapy. Nanomedicine: Nanotechnology, Biol. Med. 10 (3), 503–514. doi:10.1016/j.nano.2013.09.011
Alves Cardoso, D., Jansen, J. A., and G. Leeuwenburgh, S. C. (2012). Synthesis and Application of Nanostructured Calcium Phosphate Ceramics for Bone Regeneration. J. Biomed. Mater. Res. 100B (8), 2316–2326. doi:10.1002/jbm.b.32794
Andrade, F. A. C., Vercik, L. C. d. O., Monteiro, F. J., and Rigo, E. C. d. S. (2016). Preparation, Characterization and Antibacterial Properties of Silver Nanoparticles-Hydroxyapatite Composites by a Simple and Eco-Friendly Method. Ceramics Int. 42 (2, Part A), 2271–2280. doi:10.1016/j.ceramint.2015.10.021
Balhaddad, A. A., Kansara, A. A., Hidan, D., Weir, M. D., Xu, H. H. K., and Melo, M. A. S. (2019). Toward Dental Caries: Exploring Nanoparticle-Based Platforms and Calcium Phosphate Compounds for Dental Restorative Materials. Bioactive Mater. 4, 43–55. doi:10.1016/j.bioactmat.2018.12.002
Banik, M., and Basu, T. (2014). Calcium Phosphate Nanoparticles: a Study of Their Synthesis, Characterization and Mode of Interaction with salmon Testis DNA. Dalton Trans. 43 (8), 3244–3259. doi:10.1039/c3dt52522h
Behera, T., and Swain, P. (2011). Antigen Adsorbed Calcium Phosphate Nanoparticles Stimulate Both Innate and Adaptive Immune Response in Fish, Labeo Rohita H. Cell Immunol. 271 (2), 350–359. doi:10.1016/j.cellimm.2011.07.015
Blander, J. M., and Medzhitov, R. (2006). Toll-dependent Selection of Microbial Antigens for Presentation by Dendritic Cells. Nature 440 (7085), 808–812. doi:10.1038/nature04596
Bolhassani, A., Javanzad, S., Saleh, T., Hashemi, M., Aghasadeghi, M. R., and Sadat, S. M. (2014). Polymeric Nanoparticles. Hum. Vaccin. Immunother. 10 (2), 321–332. doi:10.4161/hv.26796
Boonsongrit, Y., Abe, H., Sato, K., Naito, M., Yoshimura, M., Ichikawa, H., et al. (2008). Controlled Release of Bovine Serum Albumin from Hydroxyapatite Microspheres for Protein Delivery System. Mater. Sci. Eng. B 148 (1–3), 162–165. doi:10.1016/j.mseb.2007.09.006
Bruno, C., Agnolon, V., Berti, F., Bufali, S., O’Hagan, D. T., and Baudner, B. C. (2016). The Preparation and Characterization of PLG Nanoparticles with an Entrapped Synthetic TLR7 Agonist and Their Preclinical Evaluation as Adjuvant for an Adsorbed DTaP Vaccine. Eur. J. Pharmaceutics Biopharmaceutics 105, 1–8. doi:10.1016/j.ejpb.2016.05.013
Butkovich, N., Li, E., Ramirez, A., Burkhardt, A. M., and Wang, S. W. (2021). Advancements in Protein Nanoparticle Vaccine Platforms to Combat Infectious Disease. Wiley Interdiscip. Rev. Nanomed Nanobiotechnol 13 (3), e1681. doi:10.1002/wnan.1681
Cao, P., Han, F. Y., Grøndahl, L., Xu, Z. P., and Li, L. (2020). Enhanced Oral Vaccine Efficacy of Polysaccharide-Coated Calcium Phosphate Nanoparticles. ACS Omega 5 (29), 18185–18197. doi:10.1021/acsomega.0c01792
Cao, P., Wang, J., Sun, B., Rewatkar, P., Popat, A., Fu, C., et al. (2021). Enhanced Mucosal Transport of Polysaccharide–Calcium Phosphate Nanocomposites for Oral Vaccination. ACS Appl. Bio Mater. 4 (11), 7865–7878. doi:10.1021/acsabm.1c00798
Chen, L., Mccrate, J. M., Lee, J. C.-M., and Li, H. (2011). The Role of Surface Charge on the Uptake and Biocompatibility of Hydroxyapatite Nanoparticles with Osteoblast Cells. Nanotechnology 22 (10), 105708. doi:10.1088/0957-4484/22/10/105708
Chiu, D., Zhou, W., Kitayaporn, S., Schwartz, D. T., Murali-Krishna, K., Kavanagh, T. J., et al. (2012). Biomineralization and Size Control of Stable Calcium Phosphate Core-Protein Shell Nanoparticles: Potential for Vaccine Applications. Bioconjug. Chem. 23 (3), 610–617. doi:10.1021/bc200654v
Cho, J. S., Lee, J.-C., and Rhee, S.-H. (2016). Effect of Precursor Concentration and spray Pyrolysis Temperature upon Hydroxyapatite Particle Size and Density. J. Biomed. Mater. Res. 104 (2), 422–430. doi:10.1002/jbm.b.33406
Chua, B. Y., Sekiya, T., Al Kobaisi, M., Short, K. R., Mainwaring, D. E., and Jackson, D. C. (2015). A Single Dose Biodegradable Vaccine Depot that Induces Persistently High Levels of Antibody over a Year. Biomaterials 53, 50–57. doi:10.1016/j.biomaterials.2015.02.066
Coffman, R. L., Sher, A., and Seder, R. A. (2010). Vaccine Adjuvants: Putting Innate Immunity to Work. Immunity 33 (4), 492–503. doi:10.1016/j.immuni.2010.10.002
Coursaget, P., Yvonnet, B., Relyveld, E. H., Barres, J. L., Diop-Mar, I., and Chiron, J. P. (1986). Simultaneous Administration of Diphtheria-Tetanus-Pertussis-Polio and Hepatitis B Vaccines in a Simplified Immunization Program: Immune Response to Diphtheria Toxoid, Tetanus Toxoid, Pertussis, and Hepatitis B Surface Antigen. Infect. Immun. 51 (3), 784–787. doi:10.1128/iai.51.3.784-787.1986
Dasgupta, S., Bandyopadhyay, A., and Bose, S. (2009). Reverse Micelle-Mediated Synthesis of Calcium Phosphate Nanocarriers for Controlled Release of Bovine Serum Albumin. Acta Biomater. 5 (8), 3112–3121. doi:10.1016/j.actbio.2009.04.031
Dasgupta, S., Banerjee, S. S., Bandyopadhyay, A., and Bose, S. (2010). Zn- and Mg-Doped Hydroxyapatite Nanoparticles for Controlled Release of Protein. Langmuir 26 (7), 4958–4964. doi:10.1021/la903617e
Del Giudice, G., Rappuoli, R., and Didierlaurent, A. M. (2018). Correlates of Adjuvanticity: A Review on Adjuvants in Licensed Vaccines. Semin. Immunol. 39, 14–21. doi:10.1016/j.smim.2018.05.001
Dewitte, H., Verbeke, R., Breckpot, K., De Smedt, S. C., and Lentacker, I. (2014). Nanoparticle Design to Induce Tumor Immunity and challenge the Suppressive Tumor Microenvironment. Nano Today 9 (6), 743–758. doi:10.1016/j.nantod.2014.10.001
Diaz-Arévalo, D., and Zeng, M. (2020). Nanoparticle-based Vaccines: Opportunities and Limitations. Nanopharmaceuticals, 135–150. Nanoparticle-based vaccines. doi:10.1016/b978-0-12-817778-5.00007-5
Dodangeh, S., Fasihi-Ramandi, M., Daryani, A., Valadan, R., Asgarian-Omran, H., Hosseininejad, Z., et al. (2021). Protective Efficacy by a Novel Multi-Epitope Vaccine, Including MIC3, ROP8, and SAG1, against Acute Toxoplasma Gondii Infection in BALB/c Mice. Microb. Pathogenesis 153, 104764. doi:10.1016/j.micpath.2021.104764
Dorozhkin, S. V. (2006). Calcium Phosphates and Human Beings. J. Chem. Educ. 83 (5), 713–719. doi:10.1021/ed083p713
Dorozhkin, S. V., and Epple, M. (2002). Biological and Medical Significance of Calcium Phosphates. Angew. Chem. Int. Ed. 41 (17), 3130–3146. doi:10.1002/1521-3773(20020902)41:17<3130:aid-anie3130>3.0.co;2-1
Duthie, M. S., Windish, H. P., Fox, C. B., and Reed, S. G. (2011). Use of Defined TLR Ligands as Adjuvants within Human Vaccines. Immunol. Rev. 239 (1), 178–196. doi:10.1111/j.1600-065x.2010.00978.x
Egli, A., Santer, D., Barakat, K., Zand, M., Levin, A., Vollmer, M., et al. (2014). Vaccine Adjuvants-Uunderstanding Molecular Mechanisms to Improve Vaccines. Swiss Med. Wkly 144, w13940. doi:10.4414/smw.2014.13940
Fathi, M. H., and Mohammadi Zahrani, E. (2009). Mechanical Alloying Synthesis and Bioactivity Evaluation of Nanocrystalline Fluoridated Hydroxyapatite. J. Cryst. Growth 311 (5), 1392–1403. doi:10.1016/j.jcrysgro.2008.11.100
Foged, C., Brodin, B., Frokjaer, S., and Sundblad, A. (2005). Particle Size and Surface Charge Affect Particle Uptake by Human Dendritic Cells in an In Vitro Model. Int. J. Pharmaceutics 298 (2), 315–322. doi:10.1016/j.ijpharm.2005.03.035
García, A., and De Sanctis, J. B. (2014). An Overview of Adjuvant Formulations and Delivery Systems. Apmis 122 (4), 257–267. doi:10.1111/apm.12143
García, C., García, C., and Paucar, C. (2012). Controlling Morphology of Hydroxyapatite Nanoparticles through Hydrothermal Microemulsion Chemical Synthesis. Inorg. Chem. Commun. 20, 90–92. doi:10.1016/j.inoche.2012.02.024
Gentile, P., Wilcock, C., Miller, C., Moorehead, R., and Hatton, P. (2015). Process Optimisation to Control the Physico-Chemical Characteristics of Biomimetic Nanoscale Hydroxyapatites Prepared Using Wet Chemical Precipitation. Materials 8 (1996-19445), 2297–2310. doi:10.3390/ma8052297
Goto, N., Kato, H., Maeyama, J., Shibano, M., Saito, T., Yamaguchi, J., et al. (1997). Local Tissue Irritating Effects and Adjuvant Activities of Calcium Phosphate and Aluminium Hydroxide with Different Physical Properties. Vaccine 15 (12), 1364–1371. doi:10.1016/s0264-410x(97)00054-6
Goto, N., Kato, H., Maeyama, J.-i., Eto, K., and Yoshihara, S. (1993). Studies on the Toxicities of Aluminium Hydroxide and Calcium Phosphate as Immunological Adjuvants for Vaccines. Vaccine 11 (9), 914–918. doi:10.1016/0264-410x(93)90377-a
Goyal, A. K., Khatri, K., Mishra, N., Mehta, A., Vaidya, B., Tiwari, S., et al. (2009). Development of Self-Assembled Nanoceramic Carrier Construct(s) for Vaccine Delivery. J. Biomater. Appl. 24 (1), 65–84. doi:10.1177/0885328209104018
Gregory, A. E., Titball, R., and Williamson, D. (2013). Vaccine Delivery Using Nanoparticles. Front. Cel. Infect. Microbiol. 3, 13. doi:10.3389/fcimb.2013.00013
Gu, P., Liu, Z., Sun, Y., Ou, N., Hu, Y., Liu, J., et al. (2019). Angelica Sinensis Polysaccharide Encapsulated into PLGA Nanoparticles as a Vaccine Delivery and Adjuvant System for Ovalbumin to Promote Immune Responses. Int. J. Pharmaceutics 554, 72–80. doi:10.1016/j.ijpharm.2018.11.008
Gu, P., Wusiman, A., Wang, S., Zhang, Y., Liu, Z., Hu, Y., et al. (2019). Polyethylenimine-coated PLGA Nanoparticles-Encapsulated Angelica Sinensis Polysaccharide as an Adjuvant to Enhance Immune Responses. Carbohydr. Polym. 223, 115128. doi:10.1016/j.carbpol.2019.115128
Gupta, R. K., and Siber, G. R. (1995). Adjuvants for Human Vaccines-Current Status, Problems and Future Prospects. Vaccine 13 (14), 1263–1276. doi:10.1016/0264-410x(95)00011-o
Gutjahr, A., Phelip, C., Coolen, A. L., Monge, C., Boisgard, A. S., Paul, S., et al. (2016). Biodegradable Polymeric Nanoparticles-Based Vaccine Adjuvants for Lymph Nodes Targeting. Vaccines (Basel) 4 (4), 34. doi:10.3390/vaccines4040034
Gutjahr, A., Papagno, L., Nicoli, F., Lamoureux, A., Vernejoul, F., Lioux, T., et al. (2017). Cutting Edge: A Dual TLR2 and TLR7 Ligand Induces Highly Potent Humoral and Cell-Mediated Immune Responses. J.I. 198 (11), 4205–4209. doi:10.4049/jimmunol.1602131
Habraken, W., Habibovic, P., Epple, M., and Bohner, M. (2016). Calcium Phosphates in Biomedical Applications: Materials for the Future? Mater. Today 19 (2), 69–87. doi:10.1016/j.mattod.2015.10.008
Haedicke, K., Kozlova, D., Gräfe, S., Teichgräber, U., Epple, M., and Hilger, I. (2015). Multifunctional Calcium Phosphate Nanoparticles for Combining Near-Infrared Fluorescence Imaging and Photodynamic Therapy. Acta Biomater. 14, 197–207. doi:10.1016/j.actbio.2014.12.009
Halliday, A., Turner, J. D., Guimarães, A., Bates, P. A., and Taylor, M. J. (2016). The TLR2/6 Ligand PAM2CSK4 Is a Th2 Polarizing Adjuvant in Leishmania Major and Brugia malayi Murine Vaccine Models. Parasites Vectors 9, 96. doi:10.1186/s13071-016-1381-0
Hamburg, M. A. (2012). FDA's Approach to Regulation of Products of Nanotechnology. Science 336 (6079), 299–300. doi:10.1126/science.1205441
Hansen, J. (2011). Adjuvants for Vaccines against Cancer: An Investigation of CAF-Based Adjuvants Incorporating TLR Ligands for CTL-Induction against Cancer. PhD Thesis (Copenhagen: University of Copenhagen).
Hassan, M. N., Mahmoud, M. M., El-Fattah, A. A., and Kandil, S. (2016). Microwave-assisted Preparation of Nano-Hydroxyapatite for Bone Substitutes. Ceramics Int. 42 (3), 3725–3744. doi:10.1016/j.ceramint.2015.11.044
Hayashi, F., Smith, K. D., Ozinsky, A., Hawn, T. R., Yi, E. C., Goodlett, D. R., et al. (2001). The Innate Immune Response to Bacterial Flagellin Is Mediated by Toll-like Receptor 5. Nature 410 (6832), 1099–1103. doi:10.1038/35074106
Hayashi, M., Aoshi, T., Kogai, Y., Nomi, D., Haseda, Y., Kuroda, E., et al. (2016). Optimization of Physiological Properties of Hydroxyapatite as a Vaccine Adjuvant. Vaccine 34, 306–312. doi:10.1016/j.vaccine.2015.11.059
He, C., Hu, Y., Yin, L., Tang, C., and Yin, C. (2010). Effects of Particle Size and Surface Charge on Cellular Uptake and Biodistribution of Polymeric Nanoparticles. Biomaterials 31 (13), 3657–3666. doi:10.1016/j.biomaterials.2010.01.065
He, Q., Mitchell, A., Morcol, T., and Bell, S. J. D. (2002). Calcium Phosphate Nanoparticles Induce Mucosal Immunity and protection against Herpes Simplex Virus Type 2. Clin. Vaccin. Immunol 9 (5), 1021–1024. doi:10.1128/cdli.9.5.1021-1024.2002
He, Q., Mitchell, A. R., Johnson, S. L., Wagner-Bartak, C., Morcol, T., and Bell, S. J. D. (2000). Calcium Phosphate Nanoparticle Adjuvant. Clin. Diagn. Lab. Immunol. 7 (6), 899–903. doi:10.1128/cdli.7.6.899-903.2000
Hosseini, S., and Epple, M. (2021). Suppositories with Bioactive Calcium Phosphate Nanoparticles for Intestinal Transfection and Gene Silencing. Nano Select 2 (3), 561–572. doi:10.1002/nano.202000150
Hou, C. H., Hou, S. M., Hsueh, Y. S., Lin, J., Wu, H. C., and Lin, F. H. (2009). The In Vivo Performance of Biomagnetic Hydroxyapatite Nanoparticles in Cancer Hyperthermia Therapy. Biomaterials 30 (23–24), 3956–3960. doi:10.1016/j.biomaterials.2009.04.020
Hou, S., Ma, H., Ji, Y., Hou, W., and Jia, N. (2013). A Calcium Phosphate Nanoparticle-Based Biocarrier for Efficient Cellular Delivery of Antisense Oligodeoxynucleotides. ACS Appl. Mater. Inter. 5 (3), 1131–1136. doi:10.1021/am3028926
Hu, Q., Tan, Z., Liu, Y., Tao, J., Cai, Y., Zhang, M., et al. (2007). Effect of Crystallinity of Calcium Phosphate Nanoparticles on Adhesion, Proliferation, and Differentiation of Bone Marrow Mesenchymal Stem Cells. J. Mater. Chem. 17 (44), 4690–4698. doi:10.1039/b710936a
Huang, K. W., Hsu, F. F., Qiu, J. T., Chern, G. J., Lee, Y. A., Chang, C. C., et al. (2020). Highly Efficient and Tumor-Selective Nanoparticles for Dual-Targeted Immunogene Therapy against Cancer. Sci. Adv. 6 (3), eaax5032. doi:10.1126/sciadv.aax5032
Huang, X., Andina, D., Ge, J., Labarre, A., Leroux, J.-C., and Castagner, B. (2017). Characterization of Calcium Phosphate Nanoparticles Based on a PEGylated Chelator for Gene Delivery. ACS Appl. Mater. Inter. 9 (12), 10435–10445. doi:10.1021/acsami.6b15925
Irvine, D. J., Hanson, M. C., Rakhra, K., and Tokatlian, T. (2015). Synthetic Nanoparticles for Vaccines and Immunotherapy. Chem. Rev. 115 (19), 11109–11146. doi:10.1021/acs.chemrev.5b00109
Issa, A. M., Salim, M. S., Zidan, H., Mohamed, A. F., and Farrag, A.-R. H. (2014). Evaluation of the Effects of Aluminum Phosphate and Calcium Phosphate Nanoparticles as Adjuvants in Vaccinated Mice. Ijcea 5 (5), 367–373. doi:10.7763/ijcea.2014.v5.411
Iwasaki, A., and Medzhitov, R. (2015). Control of Adaptive Immunity by the Innate Immune System. Nat. Immunol. 16 (4), 343–353. doi:10.1038/ni.3123
Iwasaki, A., and Medzhitov, R. (2010). Regulation of Adaptive Immunity by the Innate Immune System. Science 327 (5963), 291–295. doi:10.1126/science.1183021
Jin, X., Zhuang, J., Zhang, Z., Guo, H., and Tan, J. (2015). Hydrothermal Synthesis of Hydroxyapatite Nanorods in the Presence of Sodium Citrate and its Aqueous Colloidal Stability Evaluation in Neutral pH. J. Colloid Interf. Sci. 443, 125–130. doi:10.1016/j.jcis.2014.12.010
Johnson, L., Duschl, A., and Himly, M. (2020). Nanotechnology-Based Vaccines for Allergen-specific Immunotherapy: Potentials and Challenges of Conventional and Novel Adjuvants under Research. Vaccines 8 (2), 237. doi:10.3390/vaccines8020237
Johnson, S., Zhan, Y., Sutherland, R. M., Mount, A. M., Bedoui, S., Brady, J. L., et al. (2009). Selected Toll-like Receptor Ligands and Viruses Promote Helper-independent Cytotoxic T Cell Priming by Upregulating CD40L on Dendritic Cells. Immunity 30 (2), 218–227. doi:10.1016/j.immuni.2008.11.015
Joyappa, D. H., Kumar, C. A., Banumathi, N., Reddy, G. R., and Suryanarayana, V. V. (2009). Calcium Phosphate Nanoparticle Prepared with Foot and Mouth Disease Virus P1-3CD Gene Construct Protects Mice and guinea Pigs against the challenge Virus. Vet. Microbiol. 139 (1–2), 58–66. doi:10.1016/j.vetmic.2009.05.004
Jun, W., Lin, L., Yurong, C., and Juming, Y. (2013). Recent Advances of Calcium Phosphate Nanoparticles for Controlled Drug Delivery. Mrmc 13 (10), 1501–1507. doi:10.2174/13895575113139990059
Kalita, S. J., Bhardwaj, A., and Bhatt, H. A. (2007). Nanocrystalline Calcium Phosphate Ceramics in Biomedical Engineering. Mater. Sci. Eng. C 27 (3), 441–449. doi:10.1016/j.msec.2006.05.018
Kamaly, N., Yameen, B., Wu, J., and Farokhzad, O. C. (2016). Degradable Controlled-Release Polymers and Polymeric Nanoparticles: Mechanisms of Controlling Drug Release. Chem. Rev. 116 (4), 2602–2663. doi:10.1021/acs.chemrev.5b00346
Kandori, K., Mizumoto, S., Toshima, S., Fukusumi, M., and Morisada, Y. (2009). Effects of Heat Treatment of Calcium Hydroxyapatite Particles on the Protein Adsorption Behavior. J. Phys. Chem. B 113 (31), 11016–11022. doi:10.1021/jp904481z
Kano, Y., Iguchi, T., Matsui, H., Adachi, K., Sakoda, Y., Miyakawa, T., et al. (2016). Combined Adjuvants of poly(I:C) Plus LAG ‐3‐Ig Improve Antitumor Effects of Tumor‐specific T Cells, Preventing Their Exhaustion. Cancer Sci. 107 (4), 398–406. doi:10.1111/cas.12861
Kasturi, S. P., Kozlowski, P. A., Nakaya, H. I., Burger, M. C., Russo, P., Pham, M., et al. (2017). Adjuvanting a Simian Immunodeficiency Virus Vaccine with Toll-like Receptor Ligands Encapsulated in Nanoparticles Induces Persistent Antibody Responses and Enhanced Protection in TRIM5α Restrictive Macaques. J. Virol. 91 (4). doi:10.1128/JVI.01844-16
Kaur, A., Poonam, P., Patil, M. T., Mehta, S. K., and Salunke, D. B. (2018). An Efficient and Scalable Synthesis of Potent TLR2 Agonistic PAM2CSK4. RSC Adv. 8 (18), 9587–9596. doi:10.1039/c8ra01387j
Kavitha, M., Subramanian, R., Vinoth, K. S., Narayanan, R., Venkatesh, G., and Esakkiraja, N. (2015). Optimization of Process Parameters for Solution Combustion Synthesis of Strontium Substituted Hydroxyapatite Nanocrystals Using Design of Experiments Approach. Powder Techn. 271, 167–181. doi:10.1016/j.powtec.2014.10.046
Kawai, T., and Akira, S. (2007). Signaling to NF-Κb by Toll-like Receptors. Trends Mol. Med. 13 (11), 460–469. doi:10.1016/j.molmed.2007.09.002
Khalifehzadeh, R., and Arami, H. (2020). Biodegradable Calcium Phosphate Nanoparticles for Cancer Therapy. Adv. Colloid Interf. Sci. 279, 102157. doi:10.1016/j.cis.2020.102157
Khalifehzadeh, R., and Arami, H. (2020). The CpG Molecular Structure Controls the Mineralization of Calcium Phosphate Nanoparticles and Their Immunostimulation Efficacy as Vaccine Adjuvants. Nanoscale 12 (17), 9603–9615. doi:10.1039/c9nr09782a
Kim, M. H., Kim, B. S., Park, H., Lee, J., and Park, W. H. (2018). Injectable Methylcellulose Hydrogel Containing Calcium Phosphate Nanoparticles for Bone Regeneration. Int. J. Biol. Macromolecules 109, 57–64. doi:10.1016/j.ijbiomac.2017.12.068
Klein, C. P. A. T., de Blieck-Hogemrst, J. M. A., Wolket, J. G. C., and de Groot, K. (1990). Studies of the Solubility of Different Calcium Phosphate Ceramic Particles In Vitro. Biomaterials 11 (7), 509–512. doi:10.1016/0142-9612(90)90067-z
Knuschke, T., Bayer, W., Rotan, O., Sokolova, V., Wadwa, M., Kirschning, C. J., et al. (2014). Prophylactic and Therapeutic Vaccination with a Nanoparticle-Based Peptide Vaccine Induces Efficient Protective Immunity during Acute and Chronic Retroviral Infection. Nanomedicine: Nanotechnology, Biol. Med. 10 (8), 1787–1798. doi:10.1016/j.nano.2014.06.014
Knuschke, T., Bayer, W., Rotan, O., Sokolova, V., Wadwa, M., Kirschning, C. J., et al. (2014). Prophylactic and Therapeutic Vaccination with a Nanoparticle-Based Peptide Vaccine Induces Efficient Protective Immunity during Acute and Chronic Retroviral Infection. Nanomedicine: Nanotechnology, Biol. Med. 10 (8), 1787–1798. doi:10.1016/j.nano.2014.06.014
Knuschke, T., Epple, M., and Westendorf, A. M. (2014). The Type of Adjuvant Strongly Influences the T-Cell Response during Nanoparticle-Based Immunization. Hum. Vaccin. Immunother. 10 (1), 164–169. doi:10.4161/hv.26203
Knuschke, T., Rotan, O., Bayer, W., Sokolova, V., Hansen, W., Sparwasser, T., et al. (2016). Combination of Nanoparticle-Based Therapeutic Vaccination and Transient Ablation of Regulatory T Cells Enhances Anti-viral Immunity during Chronic Retroviral Infection. Retrovirology 13, 24. doi:10.1186/s12977-016-0258-9
Knuschke, T., Sokolova, V., Rotan, O., Wadwa, M., Tenbusch, M., Hansen, W., et al. (2013). Immunization with Biodegradable Nanoparticles Efficiently Induces Cellular Immunity and Protects against Influenza Virus Infection. J.I. 190 (12), 6221–6229. doi:10.4049/jimmunol.1202654
Kozlova, D., Chernousova, S., Knuschke, T., Buer, J., Westendorf, A. M., and Epple, M. (2012). Cell Targeting by Antibody-Functionalized Calcium Phosphatenanoparticles. J. Mater. Chem. 22 (2), 396–404. doi:10.1039/c1jm14683a
Kozlova, D., Sokolova, V., Zhong, M., Zhang, E., Yang, J., Li, W., et al. (2014). Calcium Phosphate Nanoparticles Show an Effective Activation of the Innate Immune Response In Vitro and In Vivo after Functionalization with Flagellin. Virol. Sin. 29 (1), 33–39. doi:10.1007/s12250-014-3379-0
Kozlova, D., Sokolova, V., Zhong, M., Zhang, E., Yang, J., Li, W., et al. (2014). Calcium Phosphate Nanoparticles Show an Effective Activation of the Innate Immune Response In Vitro and In Vivo after Functionalization with Flagellin. Virol. Sin. 29 (1), 33–39. doi:10.1007/s12250-014-3379-0
Krutzik, S. R., Tan, B., Li, H., Ochoa, M. T., Liu, P. T., Sharfstein, S. E., et al. (2005). TLR Activation Triggers the Rapid Differentiation of Monocytes into Macrophages and Dendritic Cells. Nat. Med. 11 (6), 653–660. doi:10.1038/nm1246
Lee, M. S., Lee, J. E., Byun, E., Kim, N. W., Lee, K., Lee, H., et al. (2014). Target-specific Delivery of siRNA by Stabilized Calcium Phosphate Nanoparticles Using Dopa-Hyaluronic Acid Conjugate. J. Controlled Release 192, 122–130. doi:10.1016/j.jconrel.2014.06.049
Levingstone, T. J., Herbaj, S., and Dunne, N. J. (2019). Calcium Phosphate Nanoparticles for Therapeutic Applications in Bone Regeneration. Nanomaterials 9 (11), 1570. doi:10.3390/nano9111570
Li, J., Chen, Y.-C., Tseng, Y.-C., Mozumdar, S., and Huang, L. (2010). Biodegradable Calcium Phosphate Nanoparticle with Lipid Coating for Systemic siRNA Delivery. J. Controlled Release 142 (3), 416–421. doi:10.1016/j.jconrel.2009.11.008
Li, J., Yang, Y., and Huang, L. (2012). Calcium Phosphate Nanoparticles with an Asymmetric Lipid Bilayer Coating for siRNA Delivery to the Tumor. J. Controlled Release 158 (1), 108–114. doi:10.1016/j.jconrel.2011.10.020
Li, J., Yin, Y., Yao, F., Zhang, L., and Yao, K. (2008). Effect of Nano- and Micro-hydroxyapatite/chitosan-gelatin Network Film on Human Gastric Cancer Cells. Mater. Lett. 62 (17–18), 3220–3223. doi:10.1016/j.matlet.2008.02.072
Li, S., Wang, B., Jiang, S., Pan, Y., Shi, Y., Kong, W., et al. (2021). Surface-Functionalized Silica-Coated Calcium Phosphate Nanoparticles Efficiently Deliver DNA-Based HIV-1 Trimeric Envelope Vaccines against HIV-1. ACS Appl. Mater. Inter. 13 (45), 53630–53645. doi:10.1021/acsami.1c16989
Liang, K., Xiao, S., Weir, M. D., Bao, C., Liu, H., Cheng, L., et al. (2018). Poly (Amido Amine) Dendrimer and Dental Adhesive with Calcium Phosphate Nanoparticles Remineralized Dentin in Lactic Acid. J. Biomed. Mater. Res. 106 (6), 2414–2424. doi:10.1002/jbm.b.34050
Lin, K., Wu, C., and Chang, J. (2014). Advances in Synthesis of Calcium Phosphate Crystals with Controlled Size and Shape. Acta Biomater. 10 (10), 4071–4102. doi:10.1016/j.actbio.2014.06.017
Lin, Y., Wang, X., Huang, X., Zhang, J., Xia, N., and Zhao, Q. (2017). Calcium Phosphate Nanoparticles as a New Generation Vaccine Adjuvant. Expert Rev. Vaccin. 16 (9), 895–906. doi:10.1080/14760584.2017.1355733
Liu, Z., Xiao, Y., Chen, W., Wang, Y., Wang, B., Wang, G., et al. (2014). Calcium Phosphate Nanoparticles Primarily Induce Cell Necrosis through Lysosomal Rupture: the Origination of Material Cytotoxicity. J. Mater. Chem. B 2 (22), 3480–3489. doi:10.1039/c4tb00056k
Luo, Y. H., Chang, L. W., and Lin, P. (2015). Metal-Based Nanoparticles and the Immune System: Activation, Inflammation, and Potential Applications. Biomed. Res. Int. 2015, 143720. doi:10.1155/2015/143720
Ma, Y., Zhang, J., Guo, S., Shi, J., Du, W., Wang, Z., et al. (2016). Biomimetic Mineralization of Nano-Sized, Needle-like Hydroxyapatite with Ultrahigh Capacity for Lysozyme Adsorption. Mater. Sci. Eng. C 68, 551–556. doi:10.1016/j.msec.2016.06.021
Malyala, P., O'Hagan, D. T., and Singh, M. (2009). Enhancing the Therapeutic Efficacy of CpG Oligonucleotides Using Biodegradable Microparticles. Adv. Drug Deliv. Rev. 61 (3), 218–225. doi:10.1016/j.addr.2008.12.009
Mao, L., Chen, Z., Wang, Y., and Chen, C. (2021). Design and Application of Nanoparticles as Vaccine Adjuvants against Human corona Virus Infection. J. Inorg. Biochem. 219, 111454. doi:10.1016/j.jinorgbio.2021.111454
Masson, J.-D., Thibaudon, M., Bélec, L., and Crépeaux, G. (2017). Calcium Phosphate: a Substitute for Aluminum Adjuvants? Expert Rev. Vaccin. 16 (3), 289–299. doi:10.1080/14760584.2017.1244484
Matesanz, M. C., Feito, M. J., Oñaderra, M., Ramírez-Santillán, C., da Casa, C., Arcos, D., et al. (2014). Early In Vitro Response of Macrophages and T Lymphocytes to Nanocrystalline Hydroxyapatites. J. Colloid Interf. Sci. 416, 59–66. doi:10.1016/j.jcis.2013.10.045
Medzhitov, R. (2007). Recognition of Microorganisms and Activation of the Immune Response. Nature 449 (7164), 819–826. doi:10.1038/nature06246
Mohan, T., Verma, P., and Rao, D. N. (2013). Novel Adjuvants & Delivery Vehicles for Vaccines Development: a Road Ahead. Indian J. Med. Res. 138 (5), 779–795.
Moresco, E. M. Y., LaVine, D., and Beutler, B. (2011). Toll-like Receptors. Curr. Biol. 21 (13), R488–R493. doi:10.1016/j.cub.2011.05.039
Naito, Y., Nagata, T., Tachibana, S., Okimoto, M., Ohara, N., Hakamatsuka, Y., et al. (2010). Locally Applied TCP Inhibits Tumor Growth via Possible Activation of Macrophages. J. Biomed. Mater. Res. A. 92 (2), 542–547. doi:10.1002/jbm.a.32367
Navarro-Tovar, G., Palestino, G., and Rosales-Mendoza, S. (2016). An Overview on the Role of Silica-Based Materials in Vaccine Development. Expert Rev. Vaccin. 15 (11), 1449–1462. doi:10.1080/14760584.2016.1188009
Nembrini, C., Stano, A., Dane, K. Y., Ballester, M., van der Vlies, A. J., Marsland, B. J., et al. (2011). Nanoparticle Conjugation of Antigen Enhances Cytotoxic T-Cell Responses in Pulmonary Vaccination. Proc. Natl. Acad. Sci. 108 (44), E989–E997. doi:10.1073/pnas.1104264108
Olmedo, H., Herrera, M., Rojas, L., Villalta, M., Vargas, M., Leiguez, E., et al. (2014). Comparison of the Adjuvant Activity of Aluminum Hydroxide and Calcium Phosphate on the Antibody Response towardsBothrops Aspersnake Venom. J. Immunotoxicology 11 (1), 44–49. doi:10.3109/1547691x.2013.772267
Park, Y.-M., Lee, S. J., Kim, Y. S., Lee, M. H., Cha, G. S., Jung, I. D., et al. (2013). Nanoparticle-based Vaccine Delivery for Cancer Immunotherapy. Immune Netw. 13 (5), 177–183. doi:10.4110/in.2013.13.5.177
Pavot, V., Berthet, M., Rességuier, J., Legaz, S., Handké, N., Gilbert, S. C., et al. (2014). Poly(lactic Acid) and Poly(lactic-Co-Glycolic Acid) Particles as Versatile Carrier Platforms for Vaccine Delivery. Nanomedicine 9 (17), 2703–2718. doi:10.2217/nnm.14.156
Peek, L. J., Middaugh, C. R., and Berkland, C. (2008). Nanotechnology in Vaccine Delivery. Adv. Drug Deliv. Rev. 60 (8), 915–928. doi:10.1016/j.addr.2007.05.017
Peek, L. J., Middaugh, C. R., and Berkland, C. (2008). Nanotechnology in Vaccine Delivery. Adv. Drug Deliv. Rev. 60 (8), 915–928. doi:10.1016/j.addr.2007.05.017
Petkar, K. C., Patil, S. M., Chavhan, S. S., Kaneko, K., Sawant, K. K., Kunda, N. K., et al. (2021). An Overview of Nanocarrier-Based Adjuvants for Vaccine Delivery. Pharmaceutics 13 (4), 455. doi:10.3390/pharmaceutics13040455
Posadas, I., Monteagudo, S., and Ceña, V. (2016). Nanoparticles for Brain-specific Drug and Genetic Material Delivery, Imaging and Diagnosis. Nanomedicine 11 (7), 833–849. doi:10.2217/nnm.16.15
Qi, C., Zhu, Y.-J., Zhao, X.-Y., Lu, B.-Q., Tang, Q.-L., Zhao, J., et al. (2013). Highly Stable Amorphous Calcium Phosphate Porous Nanospheres: Microwave-Assisted Rapid Synthesis Using ATP as Phosphorus Source and Stabilizer, and Their Application in Anticancer Drug Delivery. Chem. Eur. J. 19 (3), 981–987. doi:10.1002/chem.201202829
Rahimian, S., Fransen, M. F., Kleinovink, J. W., Christensen, J. R., Amidi, M., Hennink, W. E., et al. (2015). Polymeric Nanoparticles for Co-delivery of Synthetic Long Peptide Antigen and Poly IC as Therapeutic Cancer Vaccine Formulation. J. Controlled Release 203, 16–22. doi:10.1016/j.jconrel.2015.02.006
Ramachandran, R., Paul, W., and Sharma, C. P. (2009). Synthesis and Characterization of PEGylated Calcium Phosphate Nanoparticles for Oral Insulin Delivery. J. Biomed. Mater. Res. 88B (1), 41–48. doi:10.1002/jbm.b.31241
Ramesh, M., Turner, L. F., Yadav, R., Rajan, T. V., Vella, A. T., and Kuhn, L. T. (2007). Effects of the Physico-Chemical Nature of Two Biomimetic Crystals on the Innate Immune Response. Int. Immunopharmacology 7 (13), 1617–1629. doi:10.1016/j.intimp.2007.08.011
Relyveld, E. H., Henocq, E., and Raynaud, M. (1964). Study of the Antidiphtheria Vaccination of Allergic Subjects with A Pure Anatoxin Absorbed on Calcium Phosphate. Bull. World Health Organ. 30, 321–325.
Relyveld, E. H., Ickovic, M. R., Hénocq, E., and Garcelon, M. (1985). Calcium Phosphate Adjuvanted Allergens. Ann. Allergy 54 (6), 521–529.
Relyveld, E. H. (1986). Preparation and Use of Calcium Phosphate Adsorbed Vaccines. Dev. Biol. Stand. 65, 131–136.
Rojas-Sánchez, L., Zhang, E., Sokolova, V., Zhong, M., Yan, H., Lu, M., et al. (2020). Genetic Immunization against Hepatitis B Virus with Calcium Phosphate Nanoparticles In Vitro and In Vivo. Acta Biomater. 110, 254–265. doi:10.1016/j.actbio.2020.04.021
Rosenberg, S. A., Yang, J. C., and Restifo, N. P. (2004). Cancer Immunotherapy: Moving beyond Current Vaccines. Nat. Med. 10 (9), 909–915. doi:10.1038/nm1100
Rotan, O., Severin, K. N., Pöpsel, S., Peetsch, A., Merdanovic, M., Ehrmann, M., et al. (2017). Uptake of the Proteins HTRA1 and HTRA2 by Cells Mediated by Calcium Phosphate Nanoparticles. Beilstein J. Nanotechnol. 8, 381–393. doi:10.3762/bjnano.8.40
Sadat-Shojai, M., Khorasani, M.-T., Dinpanah-Khoshdargi, E., and Jamshidi, A. (2013). Synthesis Methods for Nanosized Hydroxyapatite with Diverse Structures. Acta Biomater. 9 (8), 7591–7621. doi:10.1016/j.actbio.2013.04.012
Sadeghi, Z., Fasihi-Ramandi, M., and Bouzari, S. (2020). Nanoparticle-Based Vaccines for Brucellosis: Calcium Phosphate Nanoparticles-Adsorbed Antigens Induce Cross Protective Response in Mice. Ijn Vol. 15, 3877–3886. doi:10.2147/ijn.s249942
Saeed, M. I., Omar, A. R., Hussein, M. Z., Elkhidir, I. M., and Sekawi, Z. (2015). Development of Enhanced Antibody Response toward Dual Delivery of Nano-Adjuvant Adsorbed humanEnterovirus-71vaccine Encapsulated Carrier. Hum. Vaccin. Immunother. 11 (10), 2414–2424. doi:10.1080/21645515.2015.1052918
Saeed, M. I., Omar, A. R., Hussein, M. Z., Elkhidir, I. M., and Sekawi, Z. (2015). Systemic Antibody Response to Nano-Size Calcium Phospate Biocompatible Adjuvant Adsorbed HEV-71 Killed Vaccine. Clin. Exp. Vaccin. Res 4 (1), 88–98. doi:10.7774/cevr.2015.4.1.88
Saha, S. K., Banerjee, A., Banerjee, S., and Bose, S. (2009). Synthesis of Nanocrystalline Hydroxyapatite Using Surfactant Template Systems: Role of Templates in Controlling Morphology. Mater. Sci. Eng. C 29 (7), 2294–2301. doi:10.1016/j.msec.2009.05.019
Sahdev, P., Podaralla, S., Kaushik, R. S., and Perumal, O. (2013). Calcium Phosphate Nanoparticles for Transcutaneous Vaccine Delivery. J. Biomed. Nanotechnology 9 (1), 132–141. doi:10.1166/jbn.2013.1545
Salem, A. K. (2015). Nanoparticles in Vaccine Delivery. Aaps j 17 (2), 289–291. doi:10.1208/s12248-015-9720-1
Sarath Chandra, V., Elayaraja, K., Thanigai Arul, K., Ferraris, S., Spriano, S., Ferraris, M., et al. (2015). Synthesis of Magnetic Hydroxyapatite by Hydrothermal-Microwave Technique: Dielectric, Protein Adsorption, Blood Compatibility and Drug Release Studies. Ceramics Int. 41 (10, Part A), 13153–13163. doi:10.1016/j.ceramint.2015.07.088
Scheffel, F., Knuschke, T., Otto, L., Kollenda, S., Sokolova, V., Cosmovici, C., et al. (2020). Effective Activation of Human Antigen-Presenting Cells and Cytotoxic CD8+ T Cells by a Calcium Phosphate-Based Nanoparticle Vaccine Delivery System. Vaccines 8 (1), 110. doi:10.3390/vaccines8010110
Sesardic, D., Rijpkema, S., and Patel, B. P. (2007). New Adjuvants: EU Regulatory Developments. Expert Rev. Vaccin. 6 (5), 849–861. doi:10.1586/14760584.6.5.849
Sharma, S., Verma, A., Teja, B. V., Pandey, G., Mittapelly, N., Trivedi, R., et al. (2015). An Insight into Functionalized Calcium Based Inorganic Nanomaterials in Biomedicine: Trends and Transitions. Colloids Surf. B: Biointerfaces 133, 120–139. doi:10.1016/j.colsurfb.2015.05.014
Sharma, S., Verma, A., Teja, B. V., Pandey, G., Mittapelly, N., Trivedi, R., et al. (2015). An Insight into Functionalized Calcium Based Inorganic Nanomaterials in Biomedicine: Trends and Transitions. Colloids Surf. B: Biointerfaces 133, 120–139. doi:10.1016/j.colsurfb.2015.05.014
Sheen, M. R., Lizotte, P. H., Toraya-Brown, S., and Fiering, S. (2014). Stimulating Antitumor Immunity with Nanoparticles. WIREs Nanomed Nanobiotechnol 6 (5), 496–505. doi:10.1002/wnan.1274
Sidane, D., Khireddine, H., Yala, S., Ziani, S., Bir, F., and Chicot, D. (2015). Morphological and Mechanical Properties of Hydroxyapatite Bilayer Coatings Deposited on 316L SS by Sol-Gel Method. Metall. Materi Trans. B 46 (5), 2340–2347. doi:10.1007/s11663-015-0397-8
Skwarczynski, M., and Toth, I. (2016). Peptide-based Synthetic Vaccines. Chem. Sci. 7 (2), 842–854. doi:10.1039/c5sc03892h
Slütter, B., Soema, P. C., Ding, Z., Verheul, R., Hennink, W., and Jiskoot, W. (2010). Conjugation of Ovalbumin to Trimethyl Chitosan Improves Immunogenicity of the Antigen. J. Controlled Release 143 (2), 207–214. doi:10.1016/j.jconrel.2010.01.007
Smith, D. M., Simon, J. K., and Baker Jr, J. R. (2013). Applications of Nanotechnology for Immunology. Nat. Rev. Immunol. 13 (8), 592–605. doi:10.1038/nri3488
Smith, J. D., Morton, L. D., and Ulery, B. D. (2015). Nanoparticles as Synthetic Vaccines. Curr. Opin. Biotechnol. 34, 217–224. doi:10.1016/j.copbio.2015.03.014
Sokolova, V., and Epple, M. (2021). Biological and Medical Applications of Calcium Phosphate Nanoparticles. Chem. Eur. J. 27 (27), 7471–7488. doi:10.1002/chem.202005257
Sokolova, V., Knuschke, T., Kovtun, A., Buer, J., Epple, M., and Westendorf, A. M. (2010). The Use of Calcium Phosphate Nanoparticles Encapsulating Toll-like Receptor Ligands and the Antigen Hemagglutinin to Induce Dendritic Cell Maturation and T Cell Activation. Biomaterials 31 (21), 5627–5633. doi:10.1016/j.biomaterials.2010.03.067
Sokolova, V., Knuschke, T., Kovtun, A., Buer, J., Epple, M., and Westendorf, A. M. (2010). The Use of Calcium Phosphate Nanoparticles Encapsulating Toll-like Receptor Ligands and the Antigen Hemagglutinin to Induce Dendritic Cell Maturation and T Cell Activation. Biomaterials 31 (21), 5627–5633. doi:10.1016/j.biomaterials.2010.03.067
Sokolova, V., Kostka, K., Shalumon, K. T., Prymak, O., Chen, J.-P., and Epple, M. (2020). Synthesis and Characterization of PLGA/HAP Scaffolds with DNA-Functionalised Calcium Phosphate Nanoparticles for Bone Tissue Engineering. J. Mater. Sci. Mater. Med. 31 (11), 102. doi:10.1007/s10856-020-06442-1
Sokolova, V., Kozlova, D., Knuschke, T., Buer, J., Westendorf, A. M., and Epple, M. (2013). Mechanism of the Uptake of Cationic and Anionic Calcium Phosphate Nanoparticles by Cells. Acta Biomater. 9 (7), 7527–7535. doi:10.1016/j.actbio.2013.02.034
Sokolova, V., Shi, Z., Huang, S., Du, Y., Kopp, M., Frede, A., et al. (2017). Delivery of the TLR Ligand poly(I:C) to Liver Cells In Vitro and In Vivo by Calcium Phosphate Nanoparticles Leads to a Pronounced Immunostimulation. Acta Biomater. 64, 401–410. doi:10.1016/j.actbio.2017.09.037
Sun, B., and Xia, T. (2016). Nanomaterial-based Vaccine Adjuvants. J. Mater. Chem. B 4 (33), 5496–5509. doi:10.1039/c6tb01131d
Sun, B., Zhao, X., Gu, W., Cao, P., Movahedi, F., Wu, Y., et al. (2021). ATP Stabilised and Sensitised Calcium Phosphate Nanoparticles as Effective Adjuvants for a DNA Vaccine against Cancer. J. Mater. Chem. B 9 (36), 7435–7446. doi:10.1039/d1tb01408k
Sun, B., Zhao, X., Wu, Y., Cao, P., Movahedi, F., Liu, J., et al. (2021). Mannose-Functionalized Biodegradable Nanoparticles Efficiently Deliver DNA Vaccine and Promote Anti-tumor Immunity. ACS Appl. Mater. Inter. 13 (12), 14015–14027. doi:10.1021/acsami.1c01401
Sun, R., Åhlén, M., Tai, C. W., Bajnóczi, É. G., Kleijne, F., Ferraz, N., et al. (2020). Highly Porous Amorphous Calcium Phosphate for Drug Delivery and Bio-Medical Applications. Nanomaterials (Basel) 10 (1), 20. doi:10.3390/nano10010020
Swain, S. K., and Sarkar, D. (2013). Study of BSA Protein Adsorption/release on Hydroxyapatite Nanoparticles. Appl. Surf. Sci. 286, 99–103. doi:10.1016/j.apsusc.2013.09.027
Tabakovic, A., Kester, M., and Adair, J. H. (2012). Calcium Phosphate-Based Composite Nanoparticles in Bioimaging and Therapeutic Delivery Applications. Wiley Interdiscip. Rev. Nanomed Nanobiotechnol 4 (1), 96–112. doi:10.1002/wnan.163
Tai, S., Cheng, J.-Y., Ishii, H., Akimoto, S., Satoh, T., Yamamoto, K., et al. (2014). Characterization of Beta-Tricalcium Phosphate as a Novel Immunomodulator. Int. Immunopharmacology 19 (1), 45–51. doi:10.1016/j.intimp.2013.12.024
Tang, J., Howard, C. B., Mahler, S. M., Thurecht, K. J., Huang, L., and Xu, Z. P. (2018). Enhanced Delivery of siRNA to Triple Negative Breast Cancer Cells In Vitro and In Vivo through Functionalizing Lipid-Coated Calcium Phosphate Nanoparticles with Dual Target Ligands. Nanoscale 10 (9), 4258–4266. doi:10.1039/c7nr08644j
Tang, J., Li, L., Howard, C. B., Mahler, S. M., Huang, L., and Xu, Z. P. (2015). Preparation of Optimized Lipid-Coated Calcium Phosphate Nanoparticles for Enhanced In Vitro Gene Delivery to Breast Cancer Cells. J. Mater. Chem. B 3 (33), 6805–6812. doi:10.1039/c5tb00912j
Temchura, V. V., Kozlova, D., Sokolova, V., Überla, K., and Epple, M. (2014). Targeting and Activation of Antigen-specific B-Cells by Calcium Phosphate Nanoparticles Loaded with Protein Antigen. Biomaterials 35 (23), 6098–6105. doi:10.1016/j.biomaterials.2014.04.010
Temchura, V. V., Kozlova, D., Sokolova, V., Überla, K., and Epple, M. (2014). Targeting and Activation of Antigen-specific B-Cells by Calcium Phosphate Nanoparticles Loaded with Protein Antigen. Biomaterials 35 (23), 6098–6105. doi:10.1016/j.biomaterials.2014.04.010
Temizoz, B., Kuroda, E., and Ishii, K. J. (2016). Vaccine Adjuvants as Potential Cancer Immunotherapeutics. Intimm 28 (7), 329–338. doi:10.1093/intimm/dxw015
Thi, T. T. H., Suys, E. J. A., Lee, J. S., Nguyen, D. H., Park, K. D., and Truong, N. P. (2021). Lipid-Based Nanoparticles in the Clinic and Clinical Trials: From Cancer Nanomedicine to COVID-19 Vaccines. Vaccines 9 (4), 359. doi:10.3390/vaccines9040359
Thomas, S., and Prendergast, G. C. (2016). Cancer Vaccines: A Brief Overview. Methods Mol. Biol. 1403, 755–761. doi:10.1007/978-1-4939-3387-7_43
Torres-Sangiao, E., Holban, A. M., and Gestal, M. C. (2016). Advanced Nanobiomaterials: Vaccines, Diagnosis and Treatment of Infectious Diseases. Molecules 21 (7), 867. doi:10.3390/molecules21070867
Tsikourkitoudi, V., Karlsson, J., Merkl, P., Loh, E., Henriques-Normark, B., and Sotiriou, G. A. (2020). Flame-Made Calcium Phosphate Nanoparticles with High Drug Loading for Delivery of Biologics. Molecules 25 (7), 1747. doi:10.3390/molecules25071747
Tsiourvas, D., Tsetsekou, A., Kammenou, M.-I., and Boukos, N. (2011). Controlling the Formation of Hydroxyapatite Nanorods with Dendrimers. J. Am. Ceram. Soc. 94 (7), 2023–2029. doi:10.1111/j.1551-2916.2010.04342.x
Utara, S., and Klinkaewnarong, J. (2015). Sonochemical Synthesis of Nano-Hydroxyapatite Using Natural Rubber Latex as a Templating Agent. Ceramics Int. 41 (10, Part B), 14860–14867. doi:10.1016/j.ceramint.2015.08.018
Vartak, A., and Sucheck, S. (2016). Recent Advances in Subunit Vaccine Carriers. Vaccines 4 (2), 12. doi:10.3390/vaccines4020012
Viswanathan, K., Gopinath, V. P., and Raj, G. D. (2014). Formulation of Newcastle Disease Virus Coupled Calcium Phosphate Nanoparticles: an Effective Strategy for Oculonasal Delivery to Chicken. Colloids Surf. B: Biointerfaces 116, 9–16. doi:10.1016/j.colsurfb.2013.12.017
Wang, X., Li, X., Ito, A., Watanabe, Y., Sogo, Y., Hirose, M., et al. (2016). Rod-shaped and Substituted Hydroxyapatite Nanoparticles Stimulating Type 1 and 2 Cytokine Secretion. Colloids Surf. B: Biointerfaces 139, 10–16. doi:10.1016/j.colsurfb.2015.12.004
Wang, Y., Chen, J., Wei, K., Zhang, S., and Wang, X. (2006). Surfactant-assisted Synthesis of Hydroxyapatite Particles. Mater. Lett. 60 (27), 3227–3231. doi:10.1016/j.matlet.2006.02.077
Watts, C., West, M. A., and Zaru, R. (2010). TLR Signalling Regulated Antigen Presentation in Dendritic Cells. Curr. Opin. Immunol. 22 (1), 124–130. doi:10.1016/j.coi.2009.12.005
Wu, J., Weir, M. D., Melo, M. A. S., and Xu, H. H. K. (2015). Development of Novel Self-Healing and Antibacterial Dental Composite Containing Calcium Phosphate Nanoparticles. J. Dentistry 43 (3), 317–326. doi:10.1016/j.jdent.2015.01.009
Xu, H. H. K., Moreau, J. L., Sun, L., and Chow, L. C. (2011). Nanocomposite Containing Amorphous Calcium Phosphate Nanoparticles for Caries Inhibition. Dental Mater. 27 (8), 762–769. doi:10.1016/j.dental.2011.03.016
Xu, Z., Ramishetti, S., Tseng, Y.-C., Guo, S., Wang, Y., and Huang, L. (2013). Multifunctional Nanoparticles Co-delivering Trp2 Peptide and CpG Adjuvant Induce Potent Cytotoxic T-Lymphocyte Response against Melanoma and its Lung Metastasis. J. Controlled Release 172 (1), 259–265. doi:10.1016/j.jconrel.2013.08.021
Xu, Z., Wang, Y., Zhang, L., and Huang, L. (2014). Nanoparticle-Delivered Transforming Growth Factor-β siRNA Enhances Vaccination against Advanced Melanoma by Modifying Tumor Microenvironment. ACS Nano 8 (4), 3636–3645. doi:10.1021/nn500216y
Yan, S.-J., Zhou, Z.-H., Zhang, F., Yang, S.-P., Yang, L.-Z., and Yu, X.-B. (2006). Effect of Anionic PAMAM with Amido Groups Starburst Dendrimers on the Crystallization of Ca10(PO4)6(OH)2 by Hydrothermal Method. Mater. Chem. Phys. 99 (1), 164–169. doi:10.1016/j.matchemphys.2005.10.009
Yang, H., and Wang, Y. (2016). Morphology Control of Hydroxyapatite Microcrystals: Synergistic Effects of Citrate and CTAB. Mater. Sci. Eng. C 62, 160–165. doi:10.1016/j.msec.2016.01.052
Yang, Y., Li, J., Liu, F., and Huang, L. (2012). Systemic Delivery of siRNA via LCP Nanoparticle Efficiently Inhibits Lung Metastasis. Mol. Ther. 20 (3), 609–615. doi:10.1038/mt.2011.270
Zaheer, T., Pal, K., and Zaheer, I. (2021). Topical Review on Nano-Vaccinology: Biochemical Promises and Key Challenges. Process Biochem. 100, 237–244. doi:10.1016/j.procbio.2020.09.028
Zhang, H. G., and Zhu, Q. (2006). Preparation of Fluoride-Substituted Hydroxyapatite by a Molten Salt Synthesis Route. J. Mater. Sci. Mater. Med. 17 (8), 691–695. doi:10.1007/s10856-006-9679-7
Zhang, L., Weir, M. D., Chow, L. C., Antonucci, J. M., Chen, J., and Xu, H. H. K. (2016). Novel Rechargeable Calcium Phosphate Dental Nanocomposite. Dental Mater. 32 (2), 285–293. doi:10.1016/j.dental.2015.11.015
Zhang, M., Zhang, L., Chen, Y., Li, L., Su, Z., and Wang, C. (2017). Precise Synthesis of Unique Polydopamine/mesoporous Calcium Phosphate Hollow Janus Nanoparticles for Imaging-Guided Chemo-Photothermal Synergistic Therapy. Chem. Sci. 8 (12), 8067–8077. doi:10.1039/c7sc03521g
Zhao, L., Seth, A., Wibowo, N., Zhao, C.-X., Mitter, N., Yu, C., et al. (2014). Nanoparticle Vaccines. Vaccine 32 (3), 327–337. doi:10.1016/j.vaccine.2013.11.069
Zhao, Y., Huo, M., Xu, Z., Wang, Y., and Huang, L. (2015). Nanoparticle Delivery of CDDO-Me Remodels the Tumor Microenvironment and Enhances Vaccine Therapy for Melanoma. Biomaterials 68, 54–66. doi:10.1016/j.biomaterials.2015.07.053
Zhao, Z., Hu, Y., Hoerle, R., Devine, M., Raleigh, M., Pentel, P., et al. (2017). A Nanoparticle-Based Nicotine Vaccine and the Influence of Particle Size on its Immunogenicity and Efficacy. Nanomedicine: Nanotechnology, Biol. Med. 13 (2), 443–454. doi:10.1016/j.nano.2016.07.015
Zhou, W., Moguche, A. O., Chiu, D., Murali-Krishna, K., and Baneyx, F. (2014). Just-in-time Vaccines: Biomineralized Calcium Phosphate Core-Immunogen Shell Nanoparticles Induce Long-Lasting CD8+ T Cell Responses in Mice. Nanomedicine: Nanotechnology, Biol. Med. 10 (3), 571–578. doi:10.1016/j.nano.2013.11.007
Zhou, W., Peng, X., Zhou, X., Weir, M. D., Melo, M. A. S., Tay, F. R., et al. (2020). In Vitro evaluation of Composite Containing DMAHDM and Calcium Phosphate Nanoparticles on Recurrent Caries Inhibition at Bovine Enamel-Restoration Margins. Dental Mater. 36 (10), 1343–1355. doi:10.1016/j.dental.2020.07.007
Zhu, M., Wang, R., and Nie, G. (2014). Applications of Nanomaterials as Vaccine Adjuvants. Hum. Vaccin. Immunother. 10 (9), 2761–2774. doi:10.4161/hv.29589
Keywords: calcium phosphate nanoparticles, antigen delivery, adjuvant, functionalisation, immunogenicity, innate immunity, humoral immunity, cellular immunity
Citation: Sun Z, Li W, Lenzo JC, Holden JA, McCullough MJ, O’Connor AJ and O’Brien-Simpson NM (2021) The Potential of Calcium Phosphate Nanoparticles as Adjuvants and Vaccine Delivery Vehicles. Front. Mater. 8:788373. doi: 10.3389/fmats.2021.788373
Received: 02 October 2021; Accepted: 30 November 2021;
Published: 22 December 2021.
Edited by:
Kytai Truong Nguyen, University of Texas at Arlington, United StatesReviewed by:
Sankar Renu, Upkara Inc., United StatesOlga Kammona, Centre for Research and Technology Hellas (CERTH), Greece
Copyright © 2021 Sun, Li, Lenzo, Holden, McCullough, O’Connor and O’Brien-Simpson. This is an open-access article distributed under the terms of the Creative Commons Attribution License (CC BY). The use, distribution or reproduction in other forums is permitted, provided the original author(s) and the copyright owner(s) are credited and that the original publication in this journal is cited, in accordance with accepted academic practice. No use, distribution or reproduction is permitted which does not comply with these terms.
*Correspondence: Neil M. O’Brien-Simpson, bmVpbC5vYnNAdW5pbWVsYi5lZHUuYXU=