- 1Department of Periodontology, Hospital of Stomatology, Jilin University, Changchun, China
- 2Jilin Provincial Key Laboratory of Tooth Development and Bone Remodeling, Jilin University, Changchun, China
- 3Department of Oral and Maxilloficila Surgery, Hospital of Stomatology, Jilin University, Changchun, China
- 4Department of Dental Implant, Hospital of Stomatology, Jilin University, Changchun, China
Oxygen is essential for cell survival and tissue regeneration. Scaffolds releasing oxygen have been hypothesized as an ideal strategy for bone repair. However, excessive oxygen supply will disturb the redox balance, lead to oxidative stress, and affect bone regeneration. In this study, we synthesized a hydrogel from sodium alginate and loaded it calcium peroxide nanoparticles as an oxygen generating material and vitamin C as a pH regulator and antioxidant. The composite hydrogel, with a pH value close to physiological humoral fluid, could release oxygen to alleviate hypoxia in the bone defect and reduce the side effects of excessive hydrogen peroxide. In in vitro experiments, the composite hydrogel promoted the osteogenic differentiation and ALP and mineralization ability of rat bone marrow mesenchymal stem cells in a hypoxic environment (2% O2). In animal experiments, the composite hydrogel was applied in rat skull defect models. It promoted the healing of bone defects. These results suggest that sodium alginate hydrogel releasing oxygen and vitamin C is suitable for cell survival and tissue regeneration in a hypoxic environment and has good application prospects in bone defect repair.
Introduction
Bone loss caused by tumors, inflammation, and trauma is very common in clinical visits (Ferretti et al., 2016; Soluk Tekkesin et al., 2016). The lack of bone not only affects the physical health of patients but also causes serious psychological problems. Autografts, allografts, and xenografts are common methods for bone repair and regeneration (Ehrler and Vaccaro, 2000; Develioglu et al., 2009; Kitzinger et al., 2012). However, the lack of donor sources and immune rejection limit the clinical applications (Brown et al., 2009; Oryan et al., 2014).
Bone tissue engineering (BTE) using scaffolds-seeding cells, genes, proteins, drugs, cytokines, and growth factors to promote bone healing and regeneration is believed to be a promising method for bone repair (Moreno Madrid et al., 2019; Bedair et al., 2020). Currently, the most widely used scaffold materials include polymer hydrogels (Jiang et al., 2019; Edri et al., 2019; Yue et al., 2020), polymer films (Holzwarth and Ma, 2011; Qu et al., 2018; Pillai et al., 2020), and 3D printed polymer blocks (Kankala et al., 2018; Shao et al., 2019; Celikkin et al., 2019; Wu et al., 2020). A hydrogel is a unique scaffold material with three-dimensional hydrophilic network structure and high moisture content of more than 90%. It is similar to the native extracellular matrix and helps to exchange oxygen and substances effectively, thus providing supporting living conditions for cell survival (Yue et al., 2020). Hydrogels have the characteristics of a high biocompatibility, low immunogenicity, and adjustable physical and chemical properties; therefore, they have received increasing attention in tissue engineering medicine (Xavier et al., 2015).
However, one of the main challenges hindering the clinical application of bone tissue engineering scaffolds, including hydrogels, is the lack of an oxygen supply (Alemdar et al., 2016; Kim et al., 2019; Touri et al., 2020). The scaffolds lack a functional vascular network, so cells in the scaffolds can only receive oxygen and nutrition from capillaries in the surrounding tissue. The diffusion of oxygen from capillaries is 100–200 μm in tissues (Carmeliet and Jain, 2000; Farris et al., 2016), but the cells in the central regions of the scaffolds can be several hundred microns away from oxygen sources. Therefore, the simple diffusion of oxygen is insufficient and numerous cells in the center experience hypoxia, which can cause cell death, tissue necrosis, and eventually result in inadequate bone regeneration (Boutilier and St-Pierre, 2000). It has been commonly accepted that a maximum volume of 1 cm3 is the limit to prevent necrotic centers in nonvascularized bone tissue engineered scaffolds (Wendt et al., 2006; Kim et al., 2014). To overcome the insufficient oxygen supply in scaffolds, several strategies have been studied extensively, including promoting the formation of a vascular network in scaffolds by the use of vascular endothelial growth factor, basic fibroblast growth factor, endothelial cells, and tissue-engineered constructs with vascular networks (Yoon et al., 2006; Fuchs et al., 2006; Nillesen et al., 2007; Hong et al., 2017). However, the slow growth of vessels and lack of function of the formed vessels in the scaffolds are the main obstacles to the application of this strategy (Griffith et al., 2005; Camci-Unal et al., 2013).
In recent years, bone tissue engineering scaffolds incorporating oxygen releasing materials have been shown to provide sufficient oxygen for cell survival until blood vessel ingrowth occurs, thus offering an alternative strategy (Lv et al., 2016; Pape et al., 2018). Liquid hydrogen peroxide, which decomposes into oxygen and water, and solid peroxides, such as calcium peroxide, which dissociate into calcium ions and hydrogen peroxide in aqueous solution and subsequently dissociate to form oxygen, are the two main kinds of oxygen releasing materials (Abdi et al., 2011; Khodaveisi et al., 2011). Adequate oxygen release of scaffolds augments extracellular matrix synthesis, angiogenesis, cell differentiation, proliferation, and migration (Rodriguez et al., 2008). However, uncontrolled and excessive oxygen release leads to increased levels of cytotoxic reactive oxygen species (ROS), peroxides, and peroxide byproducts, which can exhaust antioxidants in cell, destroy the redox balance of cells, and cause cell death (Sthijns et al., 2018).
Cells need oxygen to survive, but the supplied oxygen carries a sizeable risk of disturbing the redox balance and leading to oxidative stress. Two effective strategies to solve this problem are limiting the oxygen release and adding antioxidants to the scaffolds. For example, the encapsulation of oxygen-releasing peroxides, such as hydrogen peroxide, in polydimethysiloxane or poly(methylmethacrylate) has been shown to temper excessive and rapid release of oxygen and prevent cytotoxic oxidative stress to cells (Pedraza et al., 2012; Mallepally et al., 2014). In addition, the application of solid peroxide, such as calcium peroxide, is also a good choice for limiting oxygen release (Alemdar et al., 2016; Touri et al., 2020). Unlike hydrogen peroxide, which releases oxygen too quickly, calcium oxide produces oxygen more slowly for a longer duration, which is more conducive to maintaining the redox balance.
Another promising approach is directly incorporating antioxidant enzymes, such as catalase, in scaffolds to maintain the redox balance even in the presence of oxygen (Luo et al., 2017). Vitamin C is an effective and widely used antioxidant. It can neutralize ROS and inhibit the expression of gene-related apoptosis in extravillous trophoblasts (EVTs) under increasing oxygen concentrations (Kawashima et al., 2014). It is also proven that vitamin C compensates for reduced glutathione (GSH) depletion to protect against H2O2-induced cell death (Shang et al., 2003). Vitamin C can also reduce the toxicity of hydrogels, promote the survival of bone marrow mesenchymal cells encapsulated in hydrogels, and promote collagen production and bone regeneration (Yang et al., 2017).
Based on the above background information, we prepared a sodium alginate hydrogel incorporating calcium peroxide nanoparticles and vitamin C as a scaffold material to repair a defect in rat skull and measure the effect of the hydrogel scaffold on bone regeneration.
Experimental Procedures
Materials
Calcium chloride (99.5%), hydrogen peroxide aqueous solution H2O2 (35%), PEG 200, ammonia (25%), and sodium hydroxide (NaOH) were purchased from Beijing Chemical Co., Ltd. The cell culture medium, fetal bovine serum (FBS), trypsin-EDTA, cell counting kit-8 (CCK-8), alizarin red S staining solution (1%, pH 4.2), and hematoxylin-eosin (H&E) staining kit were purchased from Beijing Solarbio Science & Technology Co., Ltd. The primers used in qRT-PCR were purchased from Takara. ALP staining solution was purchased from Sigma-Aldrich.
Preparation of CaO2 Nanoparticles
The method to prepare calcium peroxide is from the literature (Khodaveisi et al., 2011). We dissolved 6 g of calcium chloride in 60 ml distilled water, and 30 ml ammonia solution (1 mol/L) and 240 ml of PEG 200 were added while stirring at ambient temperature. Then, 30 ml of 30% H2O2 was added to the stirring mixture at the rate of 3 drops per minute. The solution was adjusted to a pH of 10 using ammonia solution, resulting in precipitation of pale-yellow precipitates. The precipitate was separated by centrifuge, washed three times by NaOH solution (pH = 13) and twice by distilled water, and then dried at 80°C for 2 h in an evacuated oven.
Preparation of Polyacrylamide-sodium Alginate Hydrogel
We dissolved 1.169 g of NaCl in 47.83 ml distilled water in a 250 ml beaker, and 1 g of sodium alginate was added to the water several times while stirring continued for 4 h at ambient temperature. The solution in the beaker was placed in a hot water bath (50°C) for 30 min and then removed and rested overnight. 16 g of acrylamide was added to the solution and stirred for 2 h. Finally, 0.016 g of ammonium persulfate, 40 µL of tetramethylethylenediamine, and 0.0096 g of N, N-methylene bisacrylamide were added to the solution and stirred for 15 min in an ice bath. After removing the bubbles by centrifugation, the mixed solution was injected into the glass plate, put into the oven and heated for 3 h. Then, the sodium alginate hydrogel film was obtained.
Preparation of Polyacrylamide-sodium Alginate Hydrogel Loaded With Calcium Peroxide and Vitamin C
The steps were the same as the preparation of sodium alginate hydrogel before centrifugation to remove bubbles. At this time, the pH value of the mixed solution was 9.8. Then, 10 mg of calcium peroxide nanoparticles and 15 mg of vitamin C were added to the mixed solution and stirred well. After this step, the pH of the mixed solution was reduced to 7.4, which is close to the physiological environment. Then, the drug-loaded hydrogels were obtained after centrifugation and heating like before.
Characterization of Calcium Peroxide Nanoparticles and Hydrogels
The morphology of calcium peroxide nanoparticles was observed by scanning electron microscopy (SEM, JSM-6700F), and the crystal structure of calcium peroxide was determined by X-ray diffraction (XRD, PANalytical B.V.). The morphology of the two hydrogel membranes obtained from the methods in Sections Preparation of Polyacrylamide-Sodium Alginate Hydrogel and Preparation of Polyacrylamide-Sodium Alginate Hydrogel Loaded with Calcium Peroxide and Vitamin C were lyophilized and observed by scanning electron microscopy. Fourier transform infrared spectroscopy (FTIR, Nicolet AVATAR 360) of calcium peroxide nanoparticles, vitamin C, sodium alginate hydrogel, and sodium alginate hydrogel loaded with calcium peroxide and vitamin C was performed, and the presence of each component in the composite hydrogel was confirmed by comparing the characteristic peak positions.
Oxygen Generating Capacity and Degradation Test of Composite Hydrogel
First, the oxygen generating capacity and rate after mixing of calcium peroxide nanoparticles and vitamin C were studied in solution. We simultaneously added 10 mg of calcium peroxide nanoparticles and 15 mg of vitamin C to 100 ml of water, and then placed this mixture in a vacuum device with a gas collector connected to a gas chromatograph (GC-7900 gas chromatograph). After removing the air from the vacuum device, the amount of oxygen was detected by chromatographic analysis of the generated gas, which is detected every half hour. After successfully demonstrating the generation of oxygen, sodium alginate hydrogel loaded with calcium peroxide (10 mg) and vitamin C (15 mg) was added into 100 ml simulated body fluid. The amount of oxygen production during hydrogel degradation was measured once a day according to the above method.
The composite hydrogel was made into a circular film with a diameter of 5 mm and a thickness of 1 mm. After weighed, the film were placed in several times volume of water for natural degradation. The pH value of the degrading liquid was detected every 2 days. Then the liquid was discarded and the residual hydrogel was dried by filter paper, weighed and calculated the percentage of total weight.
Cell Cytotoxicity and Proliferation Assay
Rat bone marrow mesenchymal stem cells (rBMSCs) were used for the cell viability assay. rBMSCs were extracted from sterile femur of the rats, and the third passage cells that had grown well were used for further experiments. Due to the short duration of the cell viability assay and the long degradation duration of the solidified hydrogel, we chose the unsolidified hydrogel solution for the cytotoxicity tests. The rBMSCs were seeded in 96-well plates cultured for 24 h in an incubator under normoxic condition (21% O2) and co-cultured with the hydrogels and the composite hydrogels at different doses (0, 25, 50, 100, 200 μg/ml) for another 24 h. Then all the cell culture mediums were changed and the CCK-8 staining solution was added. After 3 h, the optical density values at 490 nm were tested by a microplate reader to further calculate the cell survival rates. In the cell proliferation assay, rBMSCs were seeded in 24-well plates and cultured for 24 h in an incubator (37°C, 2% O2), where cells in the control group were directly inoculated on the plate and cells in the experimental groups were inoculated on hydrogel films and composite hydrogel films of 1 mm thickness, respectively. After culturing for another 24, 48, and 96 h, the cells were stained by CCK-8 solution. The cell survival rates were calculated as before.
mRNA Expression of Osteogenic Genes
The effects of oxygen generating hydrogels on Runx2, OCN, Sp-7, and Col I gene expression in rBMSCs were investigated. In the control group, the rBMSCs were inoculated directly into a 6-well plate. For experimental groups, the hydrogel films and composite hydrogel films (1 mm in thickness) were laid flat on 6-well plates. Then, the rBMSCs were seeded on the hydrogels and cultured for 7 and 14 days in vitro under hypoxia conditions (2% O2). After 7 and 14 days of incubation at 37°C with 2% O2, the total RNA of the rBMSCs was extracted and qRT-PCR tests were performed to investigate the mRNA expression of Runx2, OCN, Sp-7, and Col I.
Alkaline Phosphatase Staining Assay
The effects of oxygen generating hydrogels on osteogenic differentiation of rBMSCs were evaluated through the qualitative and quantitative analysis of ALP activity. The rBMSCs were seeded in 24-well plates and cultured for 24 h in an incubator (37°C, 2% O2). Cells in the control group were directly inoculated on the plate, while cells in the experimental groups were inoculated on hydrogel films and composite hydrogel films of 1 mm in thickness, respectively. After culturing for 7 and 14 days, ALP activity of the three groups was detected qualitatively and quantitatively by an alkaline phosphatase staining kit.
Alizarin Red S Staining Assay
To further evaluate the effect of oxygen generating hydrogels on osteogenic differentiation of rBMSCs, the Alizarin Red S staining assay was used to identify calcium nodules in control and experimental groups. In the control group, the rBMSCs were seeded directly into 12-well plates. For the experimental groups, the rBMSCs were inoculated on hydrogel films and composite hydrogel films (1 mm in thickness). After 21 days of incubation at 37°C with 2% O2, all the mediums in the plates were discarded. Then, all the cells were fixed with 4% paraformaldehyde for 10 min and washed by distilled water. After staining for 1 h with 1% (w/v) alizarin red S solution, the cells were washed by water for three times and photographed by microscope.
Animal Experiments
Thirty male SD rats of 6-week-old were randomly divided into three groups, namely a control group, hydrogel group, and composite hydrogel group (10 rats/group). The rats were anesthetized, and then we created a critical bone defect with diameter of 5 mm on the left side of the rat skulls. In the hydrogel group, the defects were filled with hydrogel films (5 mm in diameter and 1 mm in thickness). Similarly, the same-sized oxygen generating hydrogel films were filled in the corresponding group. As a control, the defects in the control group had no fillers. The rats in each group were euthanized (5 rats/month) one, two and 3 months after the operation, respectively. The skulls, heart, liver, spleen, and lungs of the rats were surgically removed and fixed with 4% paraformaldehyde solution for further histopathological or CT analysis. Venous blood was taken from rats for the test of the liver and kidney functions. All animal procedures were performed in accordance with the Guidelines for Care and Use of Laboratory Animals of Jilin University and approved by the Animal Ethics Committee of Jilin University.
Statistical Analyses
Statistical differences between distinct groups were analyzed using one-way ANOVA. To determine whether there were significant differences in the data, the Tukey test was utilized as a comparison test. Statistical significance was set to p < 0.05 and <0.01, indicated with single and double asterisks, respectively. All results are presented as mean ± standard deviation (SD).
Results and Discussion
Preparation and Characterization of Calcium Peroxide Nanoparticles
Sufficient oxygen availability is essential for cell survival, differentiation, and function in tissue engineering applications. Hydrogen peroxide and calcium peroxide are the two most commonly used oxygen-producing materials in tissue engineering medicine at present (Coronel et al., 2017). Compared to the bursts of oxygen related by hydrogen peroxide, calcium peroxide releases oxygen relatively mildly and slowly, which is more suitable for tissue engineering medicine. In this study, we synthesized calcium peroxide nanoparticles according to the method reported by literatures (Khodaveisi et al., 2011), which uses calcium chloride, hydrogen peroxide, and PEG200 as raw materials and is a simple and effective synthetic method. As shown in scanning electron microscopy, the morphology of the synthesized products was particles of varying sizes, ranging in size from tens to hundreds of nanometers (Figure 1A). To further verify the elemental composition of the synthesized products, we analyzed the elements mapping of the products by SEM energy spectroscopy. In Supplementary Figure S1, calcium is shown in green and oxygen is shown in yellow, which indicates that the product was composed of calcium and oxygen elements. Then, X-ray diffraction analysis was used to identify the product. As shown in Figure 1B, the characteristic peaks in the XRD spectra of the samples were highly consistent with those of calcium peroxide (Khodaveisi et al., 2011). In addition, according to one study (Sasnovskaya and Razumova, 2002), calcium peroxide will undergo a thermal decomposition reaction at 350°C, generating calcium oxide and oxygen. Therefore, thermogravimetric analysis on the prepared calcium peroxide nanoparticles were performed (Figure 1C). As shown in Figure 1C, the product had a weight loss peak at 350°C, which corresponds exactly to the decomposition of calcium peroxide, which further illustrates that the nanoparticles we prepared were calcium peroxide.
Preparation and Characterization of Oxygen Generating Hydrogel
Hydrogels are ideal scaffolds in tissue engineering medicine, with excellent histocompatibility and similar characteristics to an actual tissue matrix (Buwalda et al., 2017). The loaded drug with different effects can be released during the slow degradation of the hydrogel. Therefore, by adjusting the degradation time and the loaded drugs, the hydrogel can be controlled to meet different needs in tissue engineering medicine (Kolambkar et al., 2011; Buwalda et al., 2017; Qiao et al., 2020). In addition, some cytokines produced by cells encapsulated in hydrogel can be released to the surrounding area through the three-dimensional pore of the hydrogel, and promoting tissue regeneration (Schwieger et al., 2020). In this study, acrylamide and sodium alginate, two hypotoxic organic compounds, was used to synthesize a hydrogel scaffold for the repair of a rat skull defect. As shown in SEM images, the hydrogel had a porous structure (Figure 2A), and the skeleton of the hydrogel also contained pores of different sizes (Figure 2B), which proves that the polyacrylamide-sodium alginate hydrogel was successfully synthesized.
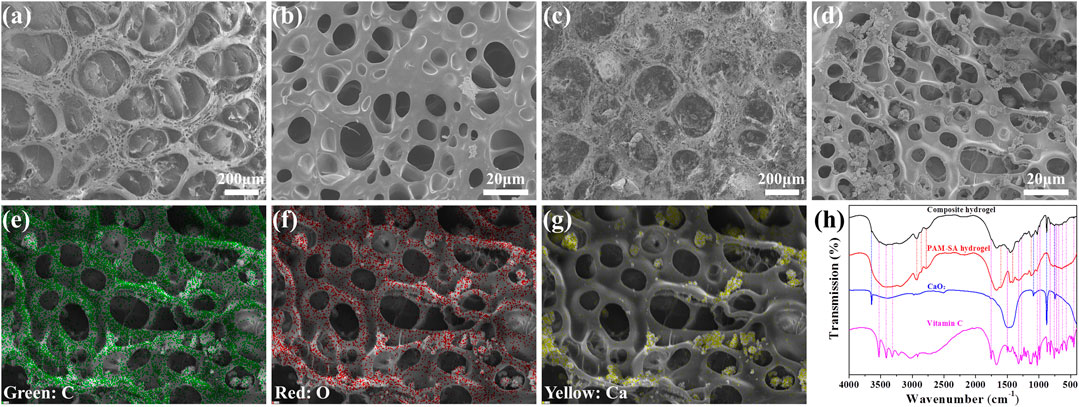
FIGURE 2. (A) SEM image of PAM-SA hydrogel at low magnification. (B) SEM image of PAM-SA hydrogel at a high magnification. (C) SEM images of the composite hydrogel at a low magnification. (D) SEM images of the composite hydrogel at a high magnification. (E) Element distribution mapping image of carbon under SEM. (F) Element distribution mapping image of oxygen under SEM. (G) Element distribution mapping image of calcium under SEM. (H) FTIR spectra of vitamin C, CaO2, the PAM-SA hydrogel, and the composite hydrogel.
To overcome hypoxia during bone regeneration in the bone defect area, calcium peroxide nanoparticles were added into the hydrogel as oxygen generating material. When reacted with water in tissue fluid, calcium peroxide produces hydrogen peroxide and calcium hydroxide, which further generates oxygen and water. However, hydrogen peroxide, a strong peroxide, will consume the surrounding antioxidants, interfere with cellular redox homeostasis, and be harmful to bone regeneration (D’Agostino et al., 2009; Wijeratne et al., 2005). In addition, calcium hydroxide, a product of calcium peroxide, is strongly alkaline and can destroy the acid-base balance of the bone defect area, thus further inhibiting bone regeneration (Kraut, 2010). Therefore, vitamin C was simultaneously added to the hydrogel to overcome the side effects of calcium peroxide, which can catalyze hydrogen peroxide and reduce the production of ROS, and also can neutralize the alkaline environment of calcium hydroxide (Scheme 1).
In the SEM images (Figures 2C,D), nanoparticles of different sizes on the surface of the hydrogel skeleton were clearly observed. Elemental mapping under SEM was used to prove the components of the nanoparticles and hydrogel. As shown in Figures 2E,F, the content of carbon and oxygen in the hydrogel skeleton was high, which was consistent with the main elements of hydrogel. In the position of nanoparticles, the oxygen content was slightly higher than in the hydrogel skeleton (Figure 2F). The content of calcium was very high and no calcium in other places was observed (Figure 2G), which indicates that the nanoparticles were calcium peroxide. In addition, the FTIR of the composite hydrogel contained characteristic peaks of PAM-SA hydrogel, calcium peroxide, and vitamin C, indicating that calcium peroxide and vitamin C were successfully loaded into the hydrogel (Figure 2H).
After loaded with calcium peroxide and vitamin C, the composite hydrogel retained its porous structure. Nitrogen adsorption and desorption experiment results showed an obvious hysteresis, which indicated that there were mesoporous pores in the hydrogel (Supplementary Figure S2A). And the pore diameter of hydrogel structure is in the range of 9–22 nm (Supplementary Figure S2B), specific surface area is 4.7 m2/g. Based on the special structure of hydrogel, its mechanical properties were further studied by rheometer. The storage modulus of the scaffold was larger than the loss modulus, which proved that the gel was successfully prepared. At the same time, the energy storage modulus of the scaffold is high, and the scaffold is in a strong gel state, which is not easy to be destroyed and has certain elasticity (Supplementary Figure S3A). With the increase of shear rate, the stress did not change significantly, and when the fixed shear rate was 10, the stress remained stable for as long as 10 min, indicating that the structure stability of hydrogel was good (Supplementary Figures S3B,C).
When the mass ratio of calcium peroxide to vitamin C was 1:1.5, the pH value of the oxygen generating hydrogel was 7.4, which is close to the pH of physiological body fluids. Similarly, oxygen generation was less and more stable than calcium peroxide alone, as shown in Supplementary Figure S4A. To simulate the oxygen generating capacity in vivo, the composite hydrogel was placed in simulated body fluids, and oxygen levels were measured daily for 12 days. As shown in Supplementary Figure S4B, oxygen was released slowly and steadily with the degradation of the hydrogel, which ensured a stable oxygen supply to the surrounding cells in vivo. The degradation time and the acid-base balance of the composite hydrogel were further tested (Supplementary Figure S5A), and the results showed that the weight of hydrogel decreased gradually with time, and the hydrogel was completely degraded on the 16th day (Supplementary Figure S5B). In the process of hydrogel degradation, the pH value of the degradation products remained neutral, which was close to the pH value of physiological humoral fluid (Supplementary Figure S5C). At the same time, the decrease of hydrogel was accompanied by the release of loading components. Basically corresponding to the degradation data, the release of vitamin C gradually increased over time and was almost complete at 16 days (Supplementary Figure S6).
Cell Survival and Osteogenic Differentiation Genes’ Expression in Hypoxia
In cytotoxicity experiments, the toxicity of the PAM-SA hydrogel and the composite hydrogel compared to rBMSCs were studied in normoxia (21% O2). After 24 h of co-culturing with the PAM-SA hydrogel and the composite hydrogel with different concentrations, the survival rates of rBMSCs were not affected. When the concentration reached 200 μg/ml, the cell survival rates were still above 90%, indicating that the two types of hydrogel had almost no toxicity (Figure 3A).
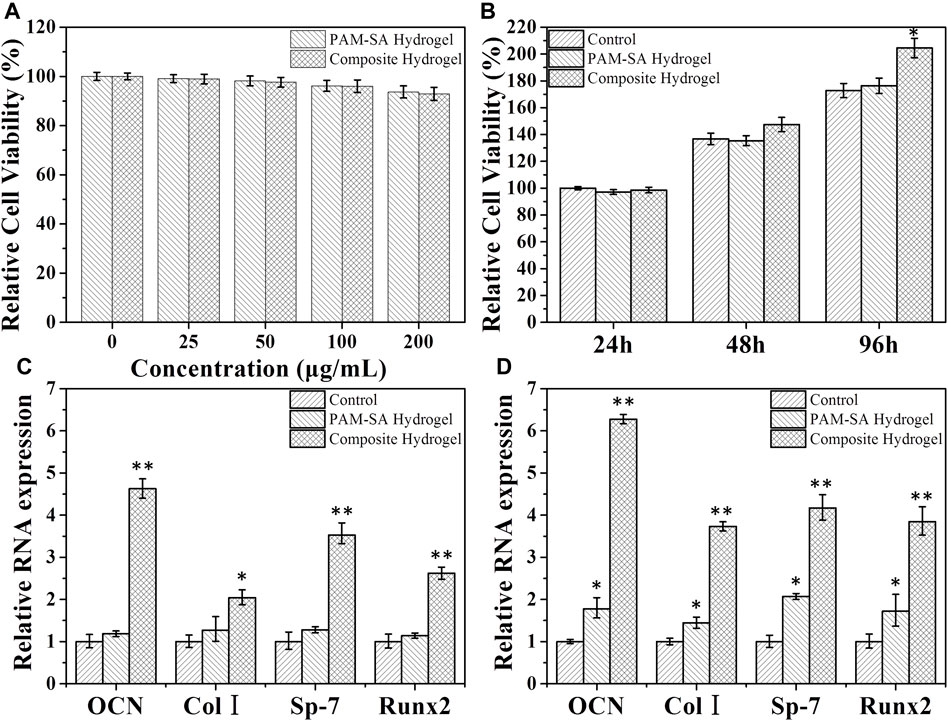
FIGURE 3. (A) Different concentrations of the hydrogel solution were obtained by placing different masses of the hydrogel in the culture medium. The cytotoxicity after a 24 h co-culture with rBMSCs was calculated. (B) The proliferation of rBMSCs co-cultured with different hydrogels for 24, 48, and 96 h in hypoxia (2% O2). The normalized osteoblastic gene expression of rBMSCs after incubation with different hydrogels in hypoxia (2% O2) for 7 days (C) and 14 days (D).
Although hypoxia can inhibit the proliferation and induce apoptosis of most cells, it has little effect on the proliferation of bone marrow mesenchymal stem cells, and even can promote the proliferation of bone marrow mesenchymal stem cells (Li et al., 2015). In our proliferation experiments, the PAM-SA hydrogel and the composite hydrogel were co-cultured with rBMSCs for 24, 48, and 96 h in hypoxia (2% O2). As shown in Figure 3B, hypoxia did not affect the proliferation of rBMSCs, and there were no significant differences among the three groups with respect to the cell viability of rBMSCs in a short time (before 48 h). However, the proliferation of rBMSCs in the composite hydrogel group were accelerated compared to the other two groups after 48 h, which may be associated with the supply of oxygen.
Hypoxia has little effect on the proliferation of bone mesenchymal stem cells, but hypoxia can inhibit the differentiation of bone mesenchymal stem cells, which is crucial for bone regeneration. It has been proven that hypoxia (1% oxygen concentration) can inhibit the osteogenic differentiation of bone mesenchymal stem cells, and when the oxygen concentration increases from 1 to 3%, bone mesenchymal stem cells resume osteogenic differentiation (Holzwarth et al., 2010). In the present study, the results show that the composite hydrogel, with its ability of oxygen generation, could significantly promote the expression of osteogenic differentiation genes of rBMSCs (Figures 3C,D). The results also showed that the PAM-SA hydrogel without an oxygen supply could also promote the osteogenic differentiation genes’ expression of rBMSCs (Figure 3D).
Osteogenic Effects of Oxygen Generating Hydrogel in Hypoxia
ALP staining and alizarin red S staining were performed to measure the potential osteogenic capability of oxygen generating hydrogel in hypoxia (2% O2). The ALP intensity increased with the extension of culture time, and the ALP activity of each group on the 14th day was significantly higher than that on the 7th day (Figures 4A–F). The ALP activity of the oxygen generating hydrogel group was the highest among the three groups, while the ALP activity of the other two groups was not significantly different.
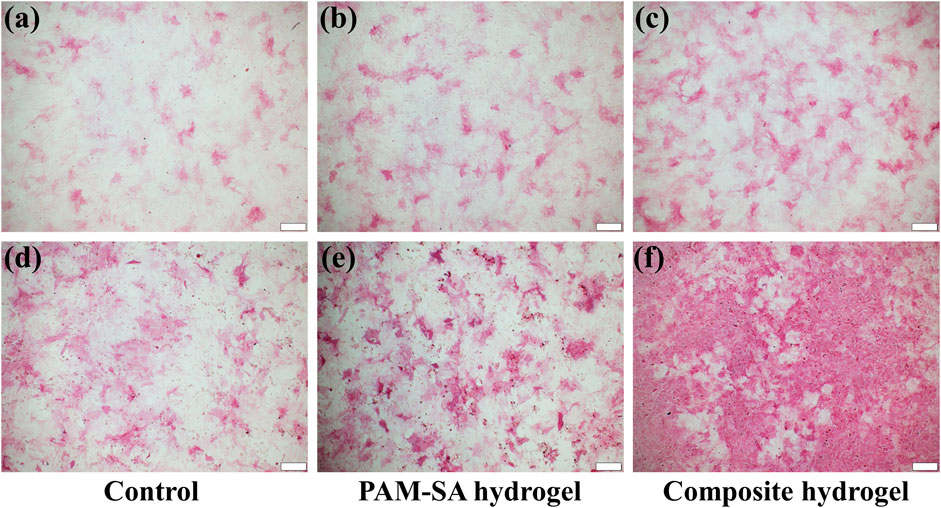
FIGURE 4. ALP-stained photos of rBMSCs on the 7th and 14th days. (A) Control group, 7 days; (B) PAM-SA hydrogel group, 7 days; (C) Composite hydrogel group, 7 days; (D) Control group, 14 days; (E) PAM-SA hydrogel group, 14 days; (F) Composite hydrogel group, 14 days. The scale bar is 100 µm.
Alizarin red S staining was employed to recognize the calcium nodules formed by the rBMSCs (Figures 5A–C). After 21 days of cultivation, increased calcium deposition on the cells was observed in the three groups (Supplementary Figure S7). Compared with the control group, the calcium nodules in the other two groups increased significantly, especially in the oxygen generating hydrogel group, which indicated that sodium alginate hydrogel could promote mineralization of rBMSCs and the oxygen generating hydrogel combined with calcium peroxide and vitamin C had a better outcome in improving the osteogenesis of rBMSCs.
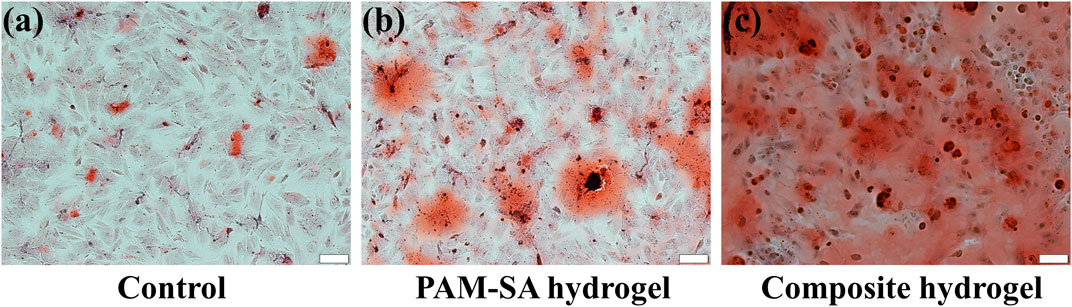
FIGURE 5. Alizarin Red S-stained photos of rBMSCs on the 21st day. (A) Control group; (B) PAM-SA hydrogel group; (C) Composite hydrogel group. The scale bar is 100 µm.
Effects of Oxygen Generating Hydrogel on Bone Generation in vivo
The oxygen generating hydrogels were applied to rat skull defect models for investigating bone regeneration. Micro-CT data showed that after 1 month of operation, there was almost no new bone formation in the control group (Figure 6A). A small amount of new bone had formed with no significant difference at the edge of the bone defects in the hydrogel and the oxygen generating hydrogel groups (Figures 6D,G). In the oxygen generating hydrogel group, new bone formation accelerated notably at 2 months after operation. Some amount of new bone had formed at the edge of the bone defects (Figure 6H) and half of the defect areas had been covered by new generated bone at 3 months after operation (Figure 6I). However, in the control and the hydrogel group, bone formation rates were still slow, and only a small amount of new bone had formed at the edge of the bone defects (Figures 6B,C,E,F), which may be related to hypoxia in the bone defect area. In order to reflect the effect of bone regeneration more directly, we carried out quantitative analysis and calculated the percentage of new bone volume in the total volume of defect. The new bone mass increased with time in all experimental groups. At 3 months, the volume fractions of new bone in the three groups were 13.7, 27.8 and 51.9%, respectively (Supplementary Figure S8). These results suggest that the oxygen generating hydrogel promoted bone regeneration, possibly due to oxygen release, which reduced hypoxia in the bone defect area.
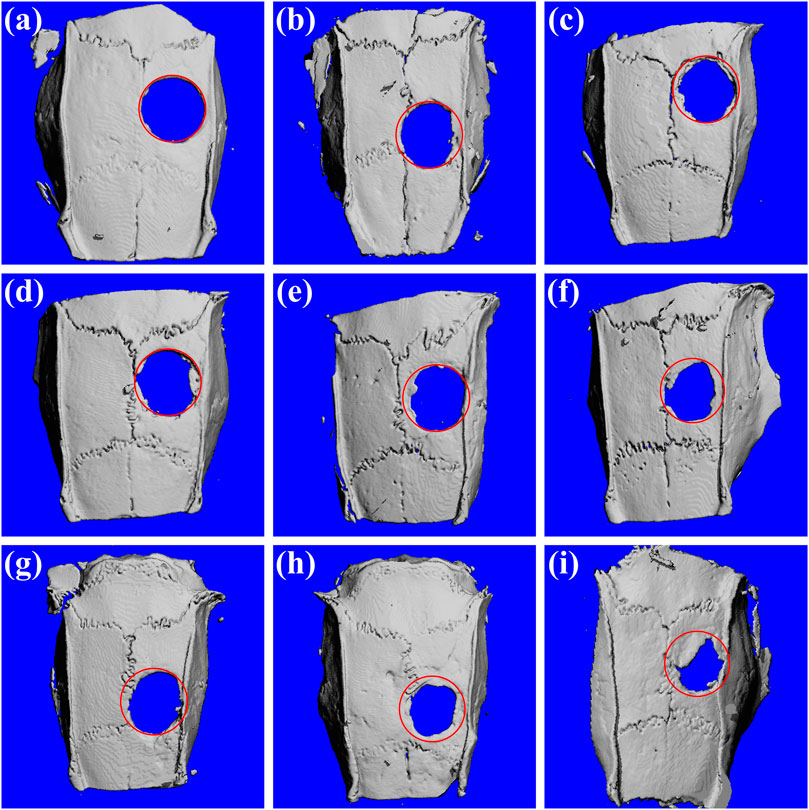
FIGURE 6. Micro-CT images of the rat skulls 1, 2, and 3 months after the surgery. (A) Control group, 1 month; (B) Control group, 2 months; (C) Control group, 3 months; (D) PAM-SA hydrogel group, 1 month; (E) PAM-SA hydrogel group, 2 months; (F) PAM-SA hydrogel group, 3 months; (G) Composite hydrogel group, 1 month; (H) Composite hydrogel group, 2 months; (I) Composite hydrogel group, 3 months.
The possible toxicity of the oxygen generating hydrogel was also analyzed by liver and kidney function tests and histopathological analysis of major organs. After 3 months, the main function indexes of liver and kidney of rats in each group were basically at the same level (Supplementary Figure S9). As shown in Figure 7, there was no histological abnormality in H&E-stained slices of heart, liver, spleen, lung, and kidney among all the three groups, which suggested that the oxygen generating hydrogel used in this work has a good safety profile.
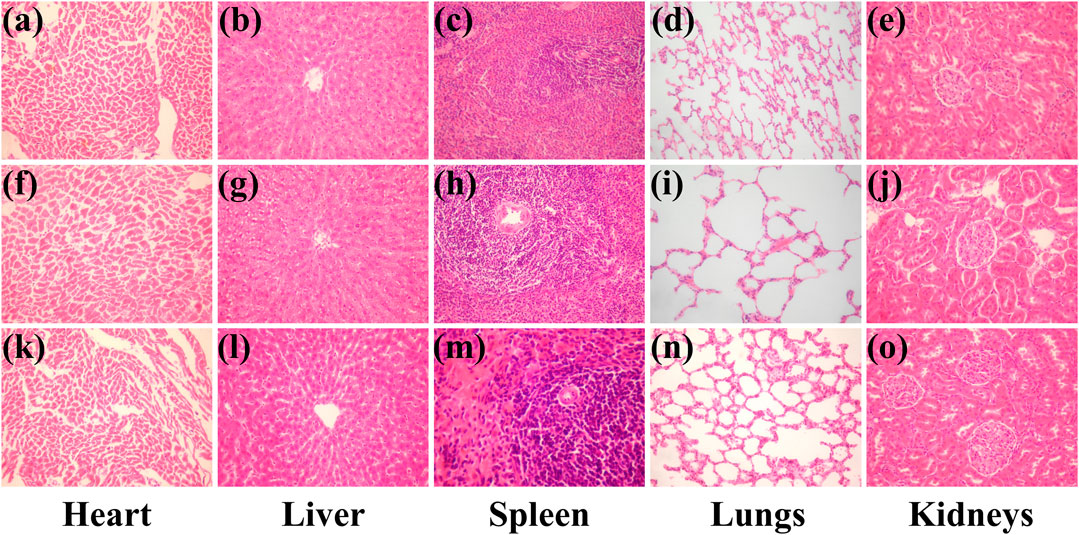
FIGURE 7. H&E-stained primary organ slices 3 months after the surgery. (A–E) Control group; (F–J) PAM-SA hydrogel group; (K–O) composite group. Magnification: 200 times.
Conclusion
This study investigated how an oxygen and vitamin C releasing hydrogel affects bone regeneration. The composite hydrogel was synthesized from sodium alginate, a non-toxic organic compound. It was loaded with calcium peroxide nanoparticles, which acted as oxygen generating material, and vitamin C, which served as a pH regulator and antioxidant. The composite hydrogel was porous with a pH value close to that of the physiological humoral fluid; it could release oxygen to alleviate hypoxia in the bone defect area and reduce the side effects of excessive hydrogen peroxide. In vitro experiments showed that the composite hydrogel promoted osteogenic differentiation, ALP activity, and mineralization ability of rBMSCs in hypoxia. The composite hydrogel was applied in rat skull defect models for bone regeneration investigation. The results showed that the composite hydrogel promoted the healing of bone defects. Hence, the system of sodium alginate hydrogel releasing oxygen and vitamin C can be regarded as an effective method for accelerated repairing of bone.
Data Availability Statement
The original contributions presented in the study are included in the article/Supplementary Materials, further inquiries can be directed to the corresponding authors.
Ethics Statement
The animal study was reviewed and approved by the Animal Ethics Committee of Jilin University.
Author Contributions
YZ and BS proposed and supervised the project. BZ and BS designed and performed the experiments and co-wrote the paper. JH, FW and RX participated in most experiments. All authors have given approval to the final version of the manuscript.
Funding
This work was supported by the Science and Technology Development Program of Jilin Province (Nos. 20210203090SF, 20200403119SF), the Science and Technology Project of the Education Department of Jilin Province (Nos. JJKH20201113KJ, JJKH20211219KJ).
Conflict of Interest
The authors declare that the research was conducted in the absence of any commercial or financial relationships that could be construed as a potential conflict of interest.
Publisher’s Note
All claims expressed in this article are solely those of the authors and do not necessarily represent those of their affiliated organizations, or those of the publisher, the editors and the reviewers. Any product that may be evaluated in this article, or claim that may be made by its manufacturer, is not guaranteed or endorsed by the publisher.
Supplementary Material
The Supplementary Material for this article can be found online at: https://www.frontiersin.org/articles/10.3389/fmats.2021.758599/full#supplementary-material
References
Abdi, S. I. H., Ng, S. M., and Lim, J. O. (2011). An Enzyme-Modulated Oxygen-Producing Micro-system for Regenerative Therapeutics. Int. J. Pharmaceutics 409 (1-2), 203–205. doi:10.1016/j.ijpharm.2011.02.041
Alemdar, N., Leijten, J., Camci-Unal, G., Hjortnaes, J., Ribas, J., Paul, A., et al. (20162017). Oxygen-Generating Photo-Cross-Linkable Hydrogels Support Cardiac Progenitor Cell Survival by Reducing Hypoxia-Induced Necrosis. ACS Biomater. Sci. Eng. 3, 1964–1971. doi:10.1021/acsbiomaterials.6b00109
Bedair, T. M., Lee, C. K., Kim, D.-S., Baek, S.-W., Bedair, H. M., Joshi, H. P., et al. (2020). Magnesium Hydroxide-Incorporated PLGA Composite Attenuates Inflammation and Promotes BMP2-Induced Bone Formation in Spinal Fusion. J. Tissue Eng. 11, 204173142096759. doi:10.1177/2041731420967591
Boutilier, R. G., and St-Pierre, J. (2000). Surviving Hypoxia without Really Dying. Comp. Biochem. Physiol. A: Mol. Integr. Physiol. 126, 481–490. doi:10.1016/S1095-6433(00)00234-8
Brown, B. N., Valentin, J. E., Stewart-Akers, A. M., Mccabe, G. P., and Badylak, S. F. (2009). Macrophage Phenotype and Remodeling Outcomes in Response to Biologic Scaffolds with and without a Cellular Component. Biomaterials 30, 1482–1491. doi:10.1016/j.biomaterials.2008.11.040
Buwalda, S. J., Vermonden, T., and Hennink, W. E. (2017). Hydrogels for Therapeutic Delivery: Current Developments and Future Directions. Biomacromolecules 18, 316–330. doi:10.1021/acs.biomac.6b01604
Camci-Unal, G., Alemdar, N., Annabi, N., and Khademhosseini, A. (2013). Oxygen-releasing Biomaterials for Tissue Engineering. Polym. Int. 62, 843–848. doi:10.1002/pi.4502
Carmeliet, P., and Jain, R. K. (2000). Angiogenesis in Cancer and Other Diseases. Nature 407, 249–257. doi:10.1038/35025220
Celikkin, N., Mastrogiacomo, S., Walboomers, X., and Swieszkowski, W. (2019). Enhancing X-ray Attenuation of 3d Printed Gelatin Methacrylate (Gelma) Hydrogels Utilizing Gold Nanoparticles for Bone Tissue Engineering Applications. Polymers 11, 367–380. doi:10.3390/polym11020367
Coronel, M. M., Geusz, R., and Stabler, C. L. (2017). Mitigating Hypoxic Stress on Pancreatic Islets via In Situ Oxygen Generating Biomaterial. Biomaterials 129, 139–151. doi:10.1016/j.biomaterials.2017.03.018
D'Agostino, D. P., Olson, J. E., and Dean, J. B. (2009). Acute Hyperoxia Increases Lipid Peroxidation and Induces Plasma Membrane Blebbing in Human U87 Glioblastoma Cells. Neuroscience 159, 1011–1022. doi:10.1016/j.neuroscience.2009.01.062
Develioglu, H., Unver Saraydin, S., and Kartal, U. (2009). The Bone-Healing Effect of a Xenograft in a Rat Calvarial Defect Model. Dent. Mater. J. 28, 396–400. doi:10.4012/dmj.28.396
Edri, R., Gal, I., Noor, N., Harel, T., Fleischer, S., Adadi, N., et al. (2019). Personalized Hydrogels for Engineering Diverse Fully Autologous Tissue Implants. Adv. Mater. 31, 1–9. doi:10.1002/adma.201803895
Ehrler, D. M., and Vaccaro, A. R. (2000). The Use of Allograft Bone in Lumbar Spine Surgery. Clin. Orthopaedics Relat. Res. 371, 38–45. doi:10.1097/00003086-200002000-00005
Farris, A. L., Rindone, A. N., and Grayson, W. L. (2016). Oxygen Delivering Biomaterials for Tissue Engineering. J. Mater. Chem. B 4, 3422–3432. doi:10.1039/C5TB02635K
Ferretti, C., Muthray, E., Rikhotso, E., Reyneke, J., and Ripamonti, U. (2016). Reconstruction of 56 Mandibular Defects with Autologous Compressed Particulate Corticocancellous Bone Grafts. Br. J. Oral Maxill. Surg. 54, 322–326. doi:10.1016/j.bjoms.2015.12.014
Fuchs, S., Motta, A., Migliaresi, C., and Kirkpatrick, C. J. (2006). Outgrowth Endothelial Cells Isolated and Expanded from Human Peripheral Blood Progenitor Cells as a Potential Source of Autologous Cells for Endothelialization of Silk Fibroin Biomaterials. Biomaterials 27, 5399–5408. doi:10.1016/j.biomaterials.2006.06.015
Griffith, C. K., Miller, C., Sainson, R. C. A., Calvert, J. W., Jeon, N. L., Hughes, C. C. W., et al. (2005). Diffusion Limits of an In Vitro Thick Prevascularized Tissue. Tissue Eng. 11, 257–266. doi:10.1089/ten.2005.11.257
Holzwarth, C., Vaegler, M., Gieseke, F., Pfister, S. M., Handgretinger, R., Kerst, G., et al. (2010). Low Physiologic Oxygen Tensions Reduce Proliferation and Differentiation of Human Multipotent Mesenchymal Stromal Cells. BMC Cell Biol 11, 11. doi:10.1186/1471-2121-11-11
Holzwarth, J. M., and Ma, P. X. (2011). Biomimetic Nanofibrous Scaffolds for Bone Tissue Engineering. Biomaterials 32, 9622–9629. doi:10.1016/j.biomaterials.2011.09.009
Hong, S., Jung, B. Y., and Hwang, C. (2017). Multilayered Engineered Tissue Sheets for Vascularized Tissue Regeneration. Tissue Eng. Regen. Med. 14 (4), 371–381. doi:10.1007/s13770-017-0049-y
Jiang, L. B., Su, D. H., Ding, S. L., Zhang, Q. C., Li, Z. F., Chen, F. C., et al. (2019). Salt‐Assisted Toughening of Protein Hydrogel with Controlled Degradation for Bone Regeneration. Adv. Funct. Mater. 29, 1901314. doi:10.1002/adfm.201901314
Kankala, R., Xu, X.-M., Liu, C.-G., Chen, A.-Z., and Wang, S.-B. (20182018). 3D-Printing of Microfibrous Porous Scaffolds Based on Hybrid Approaches for Bone Tissue Engineering. Polymers 10, 807. doi:10.3390/polym10070807
Kawashima, A., Sekizawa, A., Koide, K., Hasegawa, J., Satoh, K., Arakaki, T., et al. (2014). Vitamin C Induces the Reduction of Oxidative Stress and Paradoxically Stimulates the Apoptotic Gene Expression in Extravillous Trophoblasts Derived from First-Trimester Tissue. Reprod. Sci. 22, 783–790. doi:10.1177/1933719114561561
Khodaveisi, J., Banejad, H., Afkhami, A., Olyaie, E., Lashgari, S., and Dashti, R. (2011). Synthesis of Calcium Peroxide Nanoparticles as an Innovative Reagent for In Situ Chemical Oxidation. J. Hazard. Mater. 192, 1437–1440. doi:10.1016/j.jhazmat.2011.06.060
Kim, H. Y., Kim, S. Y., Lee, H.-Y., Lee, J. H., Rho, G.-J., Lee, H.-J., et al. (2019). Oxygen-releasing Microparticles for Cell Survival and Differentiation Ability under Hypoxia for Effective Bone Regeneration. Biomacromolecules 20, 1087–1097. doi:10.1021/acs.biomac.8b01760
Kim, J., Andersson, K.-E., Jackson, J. D., Lee, S. J., Atala, A., and Yoo, J. J. (2014). Downregulation of Metabolic Activity Increases Cell Survival under Hypoxic Conditions: Potential Applications for Tissue Engineering. Tissue Eng. A 20, 2265–2272. doi:10.1089/ten.TEA.2013.0637
Kitzinger, H. B., Karle, B., Prommersberger, K.-J., van Schoonhoven, J., and Frey, M. (2012). Four-corner Arthrodesis - Does the Source of Graft Affect Bony union Rate? Iliac Crest versus Distal Radius Bone Graft. J. Plast. Reconstr. Aesthet. Surg. 65, 379–383. doi:10.1016/j.bjps.2011.09.043
Kolambkar, Y. M., Dupont, K. M., Boerckel, J. D., Huebsch, N., Mooney, D. J., Hutmacher, D. W., et al. (2011). An Alginate-Based Hybrid System for Growth Factor Delivery in the Functional Repair of Large Bone Defects. Biomaterials 32, 65–74. doi:10.1016/j.biomaterials.2010.08.074
Kraut, J. A. (2010). Disturbances of Acid-Base Balance and Bone Disease in End-Stage Renal Disease. Semin. Dial. 13, 261–266. doi:10.1046/j.1525-139x.2000.00070.x
Le Pape, F., Richard, G., Porchet, E., Sourice, S., Dubrana, F., Férec, C., et al. (2018). Adhesion, Proliferation and Osteogenic Differentiation of Human Mscs Cultured under Perfusion with a marine Oxygen Carrier on an Allogenic Bone Substitute. Artif. Cell Nanomedicine, Biotechnol. 46, 95–107. doi:10.1080/21691401.2017.1365724
Li, L., Li, L., Zhang, Z., and Jiang, Z. (2015). Hypoxia Promotes Bone Marrow-Derived Mesenchymal Stem Cell Proliferation through apelin/APJ/autophagy Pathway. Acta Biochim. Biophys. Sinica 47, 362–367. doi:10.1093/abbs/gmv014
Luo, L., O'Reilly, A. R., Thorpe, S. D., Buckley, C. T., and Kelly, D. J. (2017). Engineering Zonal Cartilaginous Tissue by Modulating Oxygen Levels and Mechanical Cues through the Depth of Infrapatellar Fat Pad Stem Cell Laden Hydrogels. J. Tissue Eng. Regen. Med. 11, 2613–2628. doi:10.1002/term.2162
Lv, X., Li, Z., Chen, S., Xie, M., Huang, J., Peng, X., et al. (2016). Structural and Functional Evaluation of Oxygenating Keratin/silk Fibroin Scaffold and Initial Assessment of Their Potential for Urethral Tissue Engineering. Biomaterials 84, 99–110. doi:10.1016/j.biomaterials.2016.01.032
Mallepally, R. R., Parrish, C. C., Mc Hugh, M. A. M., and Ward, K. R. (2014). Hydrogen Peroxide Filled Poly(methyl Methacrylate) Microcapsules: Potential Oxygen Delivery Materials. Int. J. Pharmaceutics 475, 130–137. doi:10.1016/j.ijpharm.2014.08.052
Moreno Madrid, A. P., Vrech, S. M., Sanchez, M. A., and Rodriguez, A. P. (2019). Advances in Additive Manufacturing for Bone Tissue Engineering Scaffolds. Mater. Sci. Eng. C 100, 631–644. doi:10.1016/j.msec.2019.03.037
Nillesen, S. T. M., Geutjes, P. J., Wismans, R., Schalkwijk, J., Daamen, W. F., and van Kuppevelt, T. H. (2007). Increased Angiogenesis and Blood Vessel Maturation in Acellular Collagen-Heparin Scaffolds Containing Both FGF2 and VEGF. Biomaterials 28, 1123–1131. doi:10.1016/j.biomaterials.2006.10.029
Oryan, A., Alidadi, S., Moshiri, A., and Maffulli, N. (2014). Bone Regenerative Medicine: Classic Options, Novel Strategies, and Future Directions. J. Orthop. Surg. Res. 9, 18. doi:10.1186/1749-799X-9-18
Pedraza, E., Coronel, M. M., Fraker, C. A., Ricordi, C., and Stabler, C. L. (2012). Preventing Hypoxia-Induced Cell Death in Beta Cells and Islets via Hydrolytically Activated, Oxygen-Generating Biomaterials. Proc. Natl. Acad. Sci. 109, 4245–4250. doi:10.1073/pnas.1113560109
Pillai, M. M., Sathishkumar, G., Houshyar, S., Senthilkumar, R., Quigley, A., Shanthakumari, S., et al. (2020). Nanocomposite-Coated Silk-Based Artificial Conduits: The Influence of Structures on Regeneration of the Peripheral Nerve. ACS Appl. Bio Mater. 3, 4454–4464. doi:10.1021/acsabm.0c00430
Qiao, S., Wu, D., Li, Z., Zhu, Y., Zhan, F., Lai, H., et al. (2020). The Combination of Multi-Functional Ingredients-Loaded Hydrogels and Three-Dimensional Printed Porous Titanium Alloys for Infective Bone Defect Treatment. J. Tissue Eng. 11 (2), 204173142096579. doi:10.1177/2041731420965797
Qu, Y., Wang, B., Chu, B., Liu, C., Rong, X., Chen, H., et al. (2018). Injectable and Thermosensitive Hydrogel and PDLLA Electrospun Nanofiber Membrane Composites for Guided Spinal Fusion. ACS Appl. Mater. Inter. 10, 4462–4470. doi:10.1021/acsami.7b17020
Rodriguez, P. G., Felix, F. N., Woodley, D. T., and Shim, E. K. (2008). The Role of Oxygen in Wound Healing: a Review of the Literature. Dermatol. Surg. 34, 1159–1169. doi:10.1111/j.1524-4725.2008.34254.x
Sasnovskaya, V. D., and Razumova, A. P. (2002). Sodium Chlorate Decomposition in the Naclo3-Cao2(cao)-Mg Systems. Russ. J. Inorg. Chem. 47 (6), 794–798.
Schwieger, J., Hamm, A., Gepp, M. M., Schulz, A., Hoffmann, A., Lenarz, T., et al. (2020). Alginate-encapsulated Brain-Derived Neurotrophic Factor-Overexpressing Mesenchymal Stem Cells Are a Promising Drug Delivery System for protection of Auditory Neurons. J. Tissue Eng. 11 (8), 2041731420911313. doi:10.1177/2041731420911313
Shang, F., Lu, M., Dudek, E., Reddan, J., and Taylor, A. (2003). Vitamin C and Vitamin E Restore the Resistance of GSH-Depleted Lens Cells to H2O2. Free Radic. Biol. Med. 34, 521–530. doi:10.1016/s0891-5849(02)01304-7
Shao, H., He, J., Lin, T., Zhang, Z., Zhang, Y., and Liu, S. (2019). 3D Gel-Printing of Hydroxyapatite Scaffold for Bone Tissue Engineering. Ceramics Int. 45 (1), 1163–1170. doi:10.1016/j.ceramint.2018.09.300
Soluk Tekkesin, M., Tuna, E. B., Olgac, V., Aksakallı, N., and Alatlı, C. (2016). Odontogenic Lesions in a Pediatric Population: Review of the Literature and Presentation of 745 Cases. Int. J. Pediatr. Otorhinolaryngol. 86, 196–199. doi:10.1016/j.ijporl.2016.05.010
Sthijns, M. M. J. P. E., van Blitterswijk, C. A., and LaPointe, V. L. S. (2018). Redox Regulation in Regenerative Medicine and Tissue Engineering: The Paradox of Oxygen. J. Tissue Eng. Regen. Med. 12, 2013–2020. doi:10.1002/term.2730
Touri, M., Moztarzadeh, F., Abu Osman, N. A., Dehghan, M. M., Brouki Milan, P., Farzad-Mohajeri, S., et al. (2020). Oxygen-releasing Scaffolds for Accelerated Bone Regeneration. ACS Biomater. Sci. Eng. 6, 2985–2994. doi:10.1021/acsbiomaterials.9b01789
Wendt, D., Stroebel, S., Jakob, M., John, G. T., and Martin, I. (2006). Uniform Tissues Engineered by Seeding and Culturing Cells in 3d Scaffolds under Perfusion at Defined Oxygen Tensions. Biorheology 43, 481–488. doi:10.1016/j.bbmt.2005.09.011
Wijeratne, S. S. K., Cuppett, S. L., and Schlegel, V. (2005). Hydrogen Peroxide Induced Oxidative Stress Damage and Antioxidant Enzyme Response in Caco-2 Human colon Cells. J. Agric. Food Chem. 53, 8768–8774. doi:10.1021/jf0512003
Wu, Z., Meng, Z., Wu, Q., Zeng, D., Guo, Z., Yao, J., et al. (2020). Biomimetic and Osteogenic 3d Silk Fibroin Composite Scaffolds with Nano Mgo and Mineralized Hydroxyapatite for Bone Regeneration. J. Tissue Eng. 11, 204173142096779. doi:10.1177/2041731420967791
Xavier, J. R., Thakur, T., Desai, P., Jaiswal, M. K., Sears, N., Cosgriff-Hernandez, E., et al. (2015). Bioactive Nanoengineered Hydrogels for Bone Tissue Engineering: a Growth-factor-free Approach. ACS Nano 9, 3109–3118. doi:10.1021/nn507488s
Yang, H., Wu, S., Feng, R., Huang, J., Liu, L., Liu, F., et al. (2017). Vitamin C Plus Hydrogel Facilitates Bone Marrow Stromal Cell-Mediated Endometrium Regeneration in Rats. Stem Cell Res Ther 8, 267. doi:10.1186/s13287-017-0718-8
Yoon, J. J., Chung, H. J., Lee, H. J., and Park, T. G. (2006). Heparin-immobilized Biodegradable Scaffolds for Local and Sustained Release of Angiogenic Growth Factor. J. Biomed. Mater. Res. 79A, 934–942. doi:10.1002/jbm.a.30843
Keywords: hydrogel, oxygen supply, vitamin C, bone regeneration, hypoxia
Citation: Zhao B, He J, Wang F, Xing R, Sun B and Zhou Y (2021) Polyacrylamide-Sodium Alginate Hydrogel Releasing Oxygen and Vitamin C Promotes Bone Regeneration in Rat Skull Defects. Front. Mater. 8:758599. doi: 10.3389/fmats.2021.758599
Received: 26 August 2021; Accepted: 12 October 2021;
Published: 17 November 2021.
Edited by:
Hae-Won Kim, Institute of Tissue Regeneration Engineering (ITREN), South KoreaCopyright © 2021 Zhao, He, Wang, Xing, Sun and Zhou. This is an open-access article distributed under the terms of the Creative Commons Attribution License (CC BY). The use, distribution or reproduction in other forums is permitted, provided the original author(s) and the copyright owner(s) are credited and that the original publication in this journal is cited, in accordance with accepted academic practice. No use, distribution or reproduction is permitted which does not comply with these terms.
*Correspondence: Bin Sun, c3VuYmluMDZAc29odS5jb20=; Yanmin Zhou, emhvdXltNjJAMTI2LmNvbQ==