- 1Department of Civil and Environmental Engineering, Syracuse University, Syracuse, NY, United States
- 2School of Architecture, Syracuse University, Syracuse, NY, United States
- 3The BioInspired Institute, Syracuse University, Syracuse, NY, United States
Mycelium-based bio-composite materials have been invented and widely applied to different areas, including construction, manufacturing, agriculture, and biomedical. As the vegetative part of a fungus, mycelium has the unique capability to utilize agricultural crop waste (e.g., sugarcane bagasse, rice husks, cotton stalks, straw, and stover) as substrates for the growth of its network, which integrates the wastes from pieces to continuous composites without energy input or generating extra waste. Their low-cost and environmentally friendly features attract interest in their research and commercialization. For example, mycelium-based foam and sandwich composites have been actively developed for construction structures. It can be used as synthetic planar materials (e.g., plastic films and sheets), larger low-density objects (e.g., synthetic foams and plastics), and semi-structural materials (e.g., paneling, flooring, furniture, decking). It is shown that the material function of these composites can be further tuned by controlling the species of fungus, the growing conditions, and the post-growth processing method to meet a specific mechanical requirement in applications (e.g., structural support, acoustic and thermal insulation). Moreover, mycelium can be used to produce chitin and chitosan, which have been applied to clinical trials for wound healing, showing the potential for biomedical applications. Given the strong potential and multiple advantages of such a material, we are interested in studying it in-depth and reviewing the current progress of its related study in this review paper.
Introduction
The construction industry has undertaken significant pressure over the past decade, as the methods of producing construction materials are limited and the demand by the global population is increasing (Madurwar et al., 2013; Pheng and Hou, 2019). The production of traditional construction materials (e.g., steel, concrete) consumes significant energy. It pollutes our environment and this can be measured and tracked by embodied carbon, limiting their massive production and usage (Madurwar et al., 2013; Maraveas, 2020). At the same time, the rapidly increasing global population leads to increasing annual consumption of agricultural products, which generates more byproducts (e.g., rice husks, cotton stalks, and straw), with most of them being tracked as purely agricultural waste largely discarded or burned, generating carbon dioxide, atmospheric particulate matters, and other greenhouse gases (Bhuvaneshwari et al., 2019; Defonseka, 2019; Maraveas, 2020). They have been partly used as an additive to fertilizers, animal bedding, and low-quality building materials for infrastructures (e.g., brick elements and green concrete for low-rise buildings, insulation materials, particleboards for non-structural applications) and fillings for road construction (e.g., the local bitumen road that contains rice hull ash can bear a higher load and have water resistance) (Defonseka, 2019).
In recent years, mycelium gains more interest in academics and commerce studies because of its low energy consumption in growth, zero-byproduct, and broad potential application (Holt et al., 2012; Pelletier et al., 2013; Jones et al., 2017; Nawawi et al., 2020) (Figure 1). Mycelium is the vegetative part of a fungus, consisting of a network of fine white filaments of 1–30 μm in diameter, which spreads out from a single spore into every corner of the substrate (Fricker et al., 2007; Islam et al., 2017). Each mycelium filament is composed of multiple layers that vary in chemical composition, including proteins, glucans, and chitin (Haneef et al., 2017). The substrate composed of organic matter provides nutrition for the growth of the mycelium network. In nature, these organic matters come from the remains of organisms such as plants and animals and their waste products in the environment (Steigerwald, 2014; Swift, 2018). Their elemental composition includes cellulose, tannin, cutin, and lignin, along with other various proteins, lipids, and carbohydrates (Sejian et al., 2015). The general procedure used to grow the mycelium composite is similar to the standard protocol of raising mushrooms, which includes 1) inoculate the culturing dish with mushroom spores and sufficient nutrients and water. The incubation time for the mycelium to completely cover the dish is about 7–14 days 2) Prepare the sterilized growing substrate composed of various organic matters (e.g., brown rice, roasted buckwheat, wheat, and straw) and transfer a small piece of mycelium sample cut from the culturing dish into the growing substrate for further incubation. 3) When the substrate is full of mycelium, it is dried at a high temperature for several hours to inactivate the hyphae and stop the growth process before gaining the mycelium composite. Humidity and temperature are two important factors that can affect mycelium growth during the second stage. High humidity (relatively humidity 98%) and warm room temperature (24–25°) with fresh air provide an excellent environment for growing mycelium (Hoa and Wang, 2015).
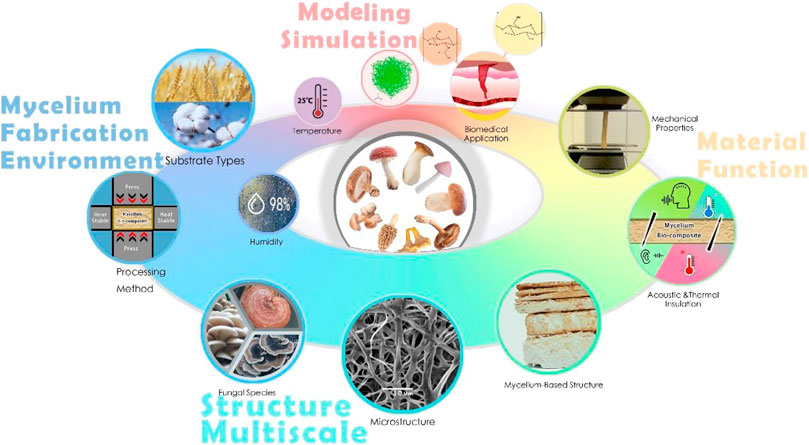
FIGURE 1. The mycelium study, including its multiscale structure, material function, and how environmental factors define these characteristics. It is essential to reveal their relationships by using experiments combined with modeling and simulation methods (the finite element modeling Islam et al., 2017 and mycelium-based structures Haneef et al., 2017 are reused under a creative commons attribution license).
The mycelium-based material can reach specific structures and material functions by controlling the substrate and processing method. Mycelium combines with organic matter generated from agricultural and industrial wastes to form the bio-composite that can be used to produce low-value materials (e.g., gap filling, packaging) and high-value composite materials for structural applications (Holt et al., 2012; Pelletier et al., 2013; Haneef et al., 2017; Islam et al., 2017; Jones et al., 2017). Unlike metal alloy or polymer composite that require energy or complex equipment to melt the raw material and mix different parts, one can uniformly mix various components in the form of small pieces to form the substrate before growing mycelium, which naturally binds and integrates the elements during its growth. Different substrates can achieve specific functions by growing mycelium composites (e.g., structural support, fire resistance, and acoustic insulation). For example, by adding rice husks and glass fines to the substrates, one can significantly increase the fire resistance of the mycelium bio-composite because it can release a lot of char and silica ash to tolerate high temperature during the burning (Bansal et al., 2006; Jones et al., 2018). In addition, mycelium bio-composite can be used as an acoustic insulation material with an outstanding capability of noise absorption. By testing different mycelium bio-composite panels using various substrates, even the worst-performing samples have over 70–75% acoustic absorption at 1,000 Hz. The substrate composed of 50% switchgrass and 50% sorghum leads to the composite of highest acoustic absorption, which can make acoustic panels with economic advantage and capability of biodegradation once exposed to nature (Pelletier et al., 2013).
Two different mycelium-based composite materials have been studied and produced for construction: mycelium-based foam (MBF) and mycelium-based sandwich composites (MBSC) are shown in Figure 2 (Girometta et al., 2019). MBF is made by growing fungi homogenously in agricultural wastes in small pieces (Appels et al., 2019). As the mycelium network grows, it produces fibers that bind these pieces together to form a porous material (Bartnicki-Garcia, 1968; Jiang et al., 2017; Karana et al., 2018). MBSC adds natural fiber fabric (e.g., jute, hemp, and cellulose) as the top and bottom layers aside from the central core as the agricultural wastes combined with mycelium form a sandwich structure of higher bending rigidity (Jiang et al., 2017). Both MBF and MBSC as the “mycelium bricks” or “panels” have shown mechanical strength, are lightweight, and have environmental advantages in packing, building insulation, and interior design in comparison to expanded polystyrene (EPS) foams (Holt et al., 2012; Jones et al., 2017, 2018; Xing et al., 2018; Girometta et al., 2019).
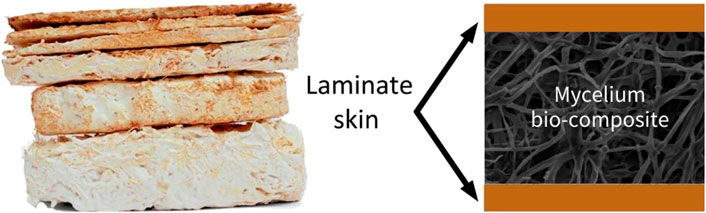
FIGURE 2. A snapshot of the MBF (left) (Karana et al., 2018) (reused under a creative commons attribution license) and a schematic of the MBSC (right).
As one of the main building blocks of mycelium, chitin is a natural polymer abundantly found in both fungal cell walls and exoskeletons of crustaceans (Jones et al., 2020a). It has been applied to biomedical applications (Morganti and Morganti, 2008; Danti et al., 2019; Jones et al., 2020a; Azimi et al., 2020). Chitin and its derivative chitosan are both long linear macromolecules that can be used to make fibers for wound dressing by electrospinning (Naseri et al., 2014; Danti et al., 2019; Morganti et al., 2019; Azimi et al., 2020), which is a fiber production method that uses the force from an electric field to draw charged polymer chains from solutions to form a continuous fiber as a bundle of aligned chains (Wang et al., 2019). Chitin has been used to produce nonwoven cloths and gels to cover a wound and interact with the open tissue for healing, making it necessary to look into their multiscale structures at the interface with biological tissues (Muzzarelli et al., 2007; Jayakumar et al., 2011; Muzzarelli, 2012). Both crustacean chitin and fungal chitin are applied in wound-dressing research, but there are significant differences in the structure, properties, and processing between them (Morin-Crini et al., 2019; Jones et al., 2020a). Both need to be extracted from the compound, as crustacean chitin often binds with sclerotized proteins and minerals, while fungal chitin binds to other polysaccharides (e.g., glucans) (Muzzarelli, 2011). Highly purified crustacean-derived chitin and chitosan have been used more widely. Still, less research has been done on fungi-derived chitin, even though the extraction process of fungal chitin is more straightforward (Di Mario et al., 2008; Hassainia et al., 2018; Jones et al., 2020a; Azimi et al., 2021). Also, fungi-derived chitin has advantages in quantity and availability, as the growth of mycelium is not subjected to seasonal and regional restrictions as for that of crustaceans (Di Mario et al., 2008; Hassainia et al., 2018).
The rest of the paper is mainly composed of three sections, for which we review. 1) The current state of mycelium study for its structure-function relationship and how environmental factors (e.g., substrate, temperature, humidity, and processing method) are involved in defining the material structure and function; 2) methods to process mycelium and its products from the macrocosmic to the microscopic scales to reach proper material functions that are suitable to wide applications from construction to biomedical; and 3) the possible way to model and optimize the material functions of mycelium-based materials and use them for broader applications.
Mycelium Fabrication Environment
Substrate Types
The substrate for mycelium growth is usually a mixture consisting of agricultural crop waste such as cotton, corn, wheat, and hemp, and flax residues as lignocellulosic wastes (Jiang et al., 2017; Appels et al., 2019; Girometta et al., 2019). The substrate for the mycelium-based foams always uses lignocellulosic waste since fungi can preferentially degrade cellulose or lignin in plant biomass (Girometta et al., 2017). Haneef et al. (2017) stated that a substrate made of the mixture of pure cellulose and potato dextrose broth (PDB) is simple and has advantages in growing mycelium. Cellulose is the most abundant natural polymer available in all hardwoods and crop wastes. It provides the material for mycelium growth, while PDB rich in simple sugars is easily digestible by mycelium and includes energy for mycelium growth. The two components are uniformly ground and mixed with a 1:1 weight ratio to form the substrate, which ensures that the growth process of the mycelium occurs on an invariable platform and produces a uniform material (Haneef et al., 2017).
Humidity and Temperature for Mycelium Growth and Its Water Content
The temperature and humidity are important factors that can affect mycelium growth. The best temperature for growing mycelium is room temperature (24–25°C) (Hoa and Wang, 2015). Moreover, growing mycelium should stay in a relatively high humidity environment. Therefore, humidifiers or sprinkler systems are usually used for mycelium growth. For example, Jiang et al. (2017) created a high humidity environment (up to 98% relative humidity) for the respiration of mycelium fungi by using a semi-permeable polypropylene bag, which provides a high humidity environment and a sterile environment for mycelium growth.
Mycelium, after natural growth, is rich in water (over 60%) (Elsacker et al., 2019). Most of the water must be removed to inactivate its growth and provide a high and reliable mechanical performance. The existing literature does not mention the final percentage of residual moisture in MBF or MBSC, but it needs to be dry enough to terminate the fungal growth (Girometta et al., 2019). The substrate and the species of fungi decided the final mycelium water content. For instance, a substrate made of hemp pulp absorbs more water than that made of cotton wool (Ziegler et al., 2016). In addition, different coatings may affect moisture absorption. It is generally believed that the moisture before deactivation is about 59% (Velasco et al., 2014) or 70–80% (Deacon, 2013), but the residual percentage in the final material recognized by researchers is approximately 10–15% (Deacon, 2013). Therefore, the water content of the final mycelium-based bio-composites is the primary consideration for the mechanics of the mycelium samples.
Because of the lack of consistent results, we perform our tests to understand the water loss of the pure mycelium network within the mushroom samples after baking for a certain amount of time, as shown in Figure 3. We use king oyster (Pleurotus Eryngii) mushrooms and prepare groups of samples with a total weight of
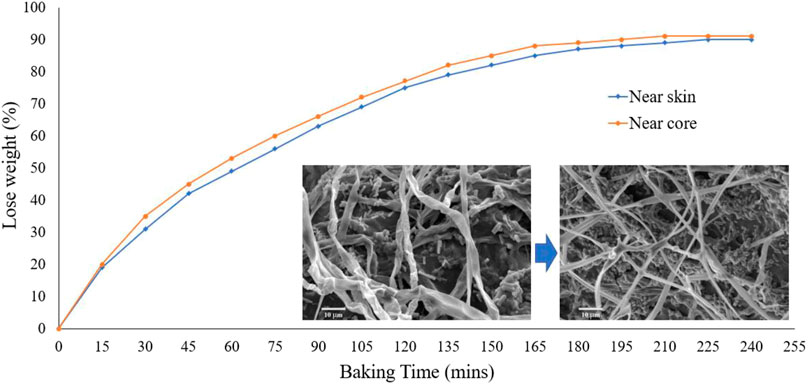
FIGURE 3. The amount of water loss percentage compared to the original weight. Inserted figure (left): the natural mycelium fiber from the skin of king oyster mushroom. Inserted figure (right): baked 30 min of mycelium fiber from the skin of a king oyster mushroom. It is shown that the natural fibers represent tubes with naturally bent overall conformation. In contrast, the dry ones become a flat ribbon curved up in the radial direction with a straight overall conformation, suggesting the more significant bending stiffness of the fiber.
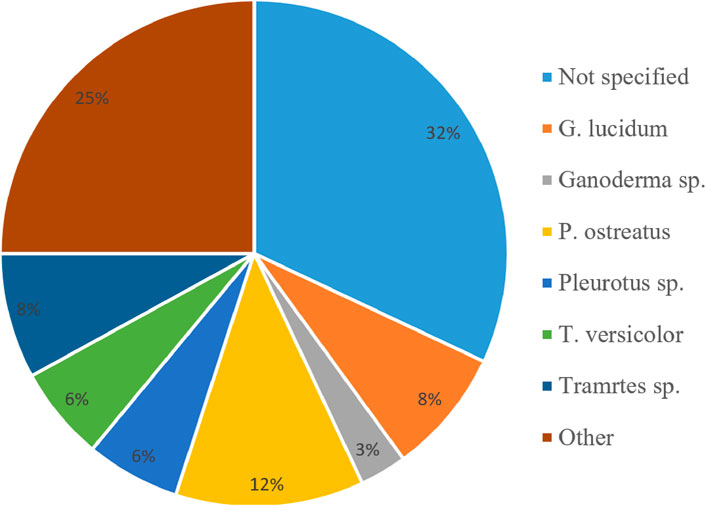
FIGURE 4. The main fungal species used for fabricating mycelium composites in the literature of the current review paper. Structural features of mycelium at different scales.
Fabrication Process
The different fabrication processes will create different functional mycelium bio-composites. The most common method is oven drying to remove the residue water within the mycelium and substrate, producing lightweight and high-strength foams (47) that can be used as the core of sandwich MBSC structures by incorporating natural fiber fabrics on both sides. Besides forming foams, the mycelium plays the role of gluing the core material to the fiber fabrics (though the interface generates during the mycelium growth) to resist delamination at the material interface once the sandwich plate is subjected to shear force in loading, leading to a strong composite board with high bending stiffness (Jiang et al., 2017). Other natural glues (e.g., bioresin) can be added before combining the fabrics to the core part to increase the adhesion of the bio-composites together with mycelium foam. They do not hinder fungus growth through more layers of fiber fabrics, which is critical to forming a robust interface with a large cohesive zone in adhesion that prevents the fabric from easily separating from the foam part through a sharp single crack from defects (Jiang et al., 2019).
The fabrication process that tunes the water content in the mycelium network can significantly affect its mechanics, as shown by former studies. Appels et al. (2019) show that pressing can substantially affect the water content and thus the mechanical properties of mycelium composites. This result is expected because pressing can squeeze water and air out of the porous mycelium network, reduces the porosity of the material, and increases the material density, leading to higher Young’s modulus and strength (Gibson and Ashby, 1982; Dai et al., 2007; Qin et al., 2017). Pressing also helps to reorientate fibers horizontally in the panel plane (Butterfield et al., 1992) and reduce plate thickness during pressing, increasing fiber connection between the walls of the fibers at points of overlap (Carvalho and Costa, 1998). Pressing may also help to reduce large voids as structural defects within the mycelium composite, preventing the crack from generating during a loading (Girometta et al., 2019; Jones et al., 2020b). In comparison to cold pressing, the hot pressing, with the pressure that comes from a pair of hot plates, can further improve the mechanical properties, as shown in an early study (Appels et al., 2019).
Multiscale Structure of Mycelium
Fungal Species
The mechanical properties of the mycelium bio-composite are determined mainly by species of fungus, which can be introduced by using different types of spores during the first stage of the incubation of mycelium. Based on the other species of fungus, its productivity, the thickness of mycelium fiber, the microstructure, and the surface topography are different (Haneef et al., 2017; Jiang et al., 2017; Appels et al., 2019; Girometta et al., 2019; Jones et al., 2020b). In fungus taxonomy, mycelium hyphae can be categorized by generative, skeletal, and binding hyphae (Corner, 1953; Jones et al., 2017). Generative hyphae are relatively undifferentiated and can develop reproductive structures. They are typically thin-walled, with occasionally thickened walls, usually have frequent septa (i.e., cell walls that separate the cells), and may have clamp connections (i.e., the unique hook-like structure for hyphal cell growing). Skeletal hyphae are thicker, longer, and rarely branched. They have few septa and lack clamp connections. Binding hyphae are thick-walled, often solid, and often branched (Ryvarden, 1991; Pegler, 1996; Ko and Jung, 1999). Based on the three different hyphal types, the mycelium network can be divided into three categories, which are monomitic, dimitic, and trimitic (Pegler, 1996). Monomitic species comprise only generative hyphae, dimitic species form two hyphal types (usually generative and skeletal), and trimitic species contain all three principle hyphal types (Webster and Weber, 2007). These mycelium networks have very different structures and mechanical properties, such as monomitic species, are suggested to provide worse mechanical performance than dimitic and trimitic hyphal species (Bayer and McIntyre, 2012, 2015). For example, trimitic species such as T. Versicolor exhibit higher tensile (0.04 MPa) and flexural strengths (0.22 MPa) than monomitic species, such as P. Ostreatus (0.01 MPa tensile strength, 0.06 MPa flexural strength), when grown on rapeseed straw (Jones et al., 2020b).
Figure 4 shows the common fungal species reported in the literature as we reviewed here. From these species, Ganoderma lucidum (25%, by the number of studies) and Pleurotus ostreatus (12%) were found to be the most common (Attias et al., 2020). These fungi are composed of trimitic and monomitic species. There is tremendous scientific significance in these two species due to the essential chemicals they produced, including a variety of enzymes that can efficiently degrade plant components difficult to hydrolyze, including lignin (Islam et al., 2015; Petre, 2015; Haneef et al., 2017). However, many publications do not reveal the species of fungus for the composite production (32%), making it hard to fully reproduce the work by overlooking the type of mycelium network, for example (Parisi et al., 2016; Ziegler et al., 2016; Dahmen, 2017; Jiang et al., 2017).
The complex material functions of mycelium attribute to its complex network structure at different scales. The mycelium mechanical properties are controlled by branched filaments and the topology of the network structures (Islam et al., 2017). Figure 6 shows the general structure of mycelium from macroscale to nanoscale. Since the mycelium has a symbiosis relationship with the substrate, it produces the branch fiber network structure, increasing the contact area with the complex porous substrate. The mycelium network grows from a spore by extending through the cell membrane and cell wall at the tip of a mycelium fiber. Every single mycelium fiber is composed of an array of slender cells separated by cross walls, so-called septum, and enclosed within the same cell wall. Tiny holes in the septum allow for the rapid flow of nutrients, water, and other small molecules from cell to cell along with the mycelium fiber. The cell wall protects the mycelium and provides mechanical strength, and is composed of a layer of chitin, a layer of glucans, and a layer of proteins (e.g., mannoproteins and hydrophobins) on the cell membrane (Haneef et al., 2017). Chitin is a complex polysaccharide, a polymer of N-acetylglucosamine that locates on the cell membrane and plays an essential role in giving structural strength to the cell walls of fungi. We cultured the mycelium network using an agar plate as the inoculated substrate for 7–14 days and took a sample to show the pure mycelium network without other substrate fibers clearly. The SEM images show that the mycelium network comprises many mycelium fibers with a diameter of about 2 μm. After that, we migrate the mycelium network to substrate in the lab to generate the mycelium composite and mushrooms (e.g., the king oyster mushroom as shown in the image at the upper right of Figure 5), which allows us to perform the mechanical tests and microscopic images of the mycelium network at a large scale. The rectangular figure at the bottom shows the nanostructure of the α-chitin. Two primary polymorphic forms of chitin exist, α and β, with α-chitin the most common polymorph for both crustacean and fungal chitin and β-chitin occurring only in squid pen, sea tube worms, and some algae (centric diatom) (Rinaudo, 2006).
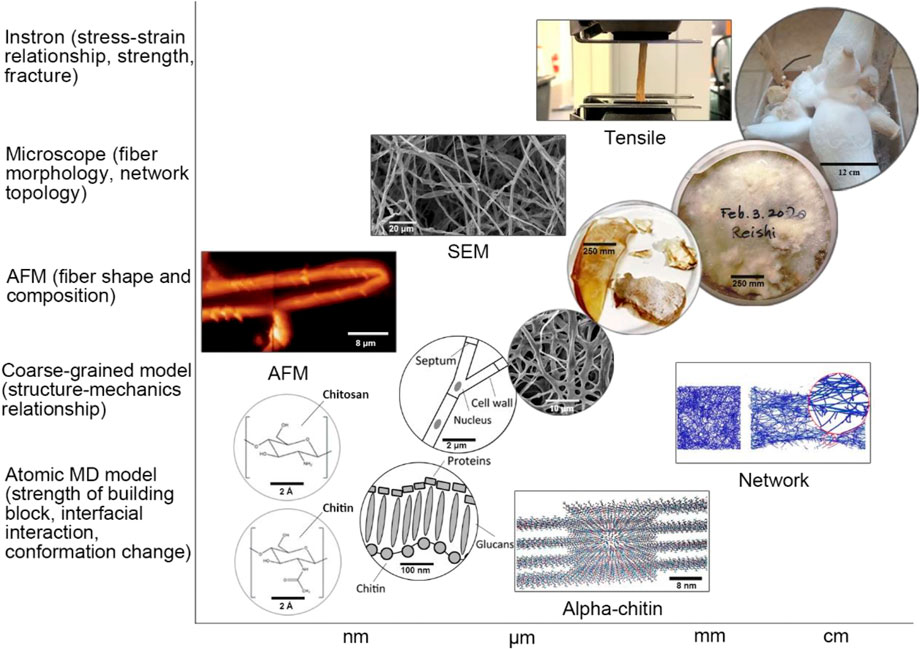
FIGURE 5. Multiscale structure of the mycelium. From the bottom left, the figure shows the molecular formula of chitin and chitosan, followed by two figures showing the structure of the single mycelium and mycelium cell wall and the SEM image of the mycelium network, as well as two figures showing the wet and dry mycelium samples in the culturing disk. The last figure at the right-up corner shows the cultured king oyster mushroom. We can use different research methods to study the structure-function relationship of the mycelium network at different scale levels, as noted at the axis of the plot (The AFM figure is reproduced under a creative commons attribution license) (Haneef et al., 2017).
Protein
Although mushrooms are a rich source of many proteins, not many proteins have been identified. Enzymes involved in the degradation of lignocellulose are among the most investigated groups of proteins from fungi (Erjavec et al., 2012). Laccases, peroxidases, oxidases, cellulases, and different glycosidases are content in other species of fungi to participate in degradation (Baldrian and Valášková, 2008; Hatakka and Hammel, 2011). The principle that enzymes degrade lignocellulose in fungi is the oxidative and hydrolytic enzymes cooperate. Fungi have two types of degradation systems: intracellular and the outer cell envelope layer, which is essential for polysaccharide degradation. Moreover, in the extracellular, hydrolytic enzymes are responsible for polysaccharide degradation, and oxidative enzymes are responsible for degrading lignin and open phenyl rings (Sánchez, 2009; Andlar et al., 2018). Mainly, there are three groups of fungi with different effects and degradation mechanisms of lignocellulose, as soft-rot, white-rot, and brown-rot fungi (Sánchez, 2009).
Soft-rot fungi can degrade surface polysaccharide layers of plants and mostly are ascomycete fungi. Peroxidases are involved in lignin modifications and laccases production, leading to the darkening and softening of the wood. These enzymes have limited degrading functions (Woiciechowski et al., 2013). White-rot fungi can degrade lignin, cellulose, and hemicellulose. The degradation of lignin is more effective than brown-rot and soft-rot fungi. The wood changes its texture and becomes moist, soft, and silky. Its color becomes white or yellow (Couturier and Berrin, 2013). Brown-rot fungi are basidiomycetes that have a different function from soft-rot fungi about degrading lignin. It can rapidly metabolize cellulose and hemicellulose and only slightly modify lignin. Due to the oxidizing reaction of lignin, the wood residue exhibits a cube shape and has a brown color (Andlar et al., 2018). The disruption of the lignocellulose matrix by brown-rot fungi can be demonstrated using iron-dependent Fenton chemistry known as the chelator-mediated Fenton system (Arantes and Goodell, 2014).
Hydrophobins are one of the other important groups of proteins unique to fungi. Hydrophobins are localized on the outer surface of filamentous fungi cell walls (Whiteford and Spanu, 2002). They are essential to the growth of fungi and the interaction between fungi with their surrounding environment by facilitating aerial development (fungi prone to grow upwards) and contribute to the attachment of fungi to solid supports (Linder et al., 2005). In addition, hydrophobins make fungi hydrophobic by assembling into an amphipathic membrane, as the hydrophobic side is exposed to the exterior and the hydrophilic surface is combined with the cell wall polysaccharides (Whiteford and Spanu, 2002).
Glucans
The most abundant polysaccharides in the cell walls of fungi are glucans. They are essential to integrate functional proteins and skeletal chitin and form fungal cell walls’ most critical structural components. Glucans in the fungi are connected through alpha (α) or beta (β) linkages. Alpha 1,3 are the most abundant alpha-glucans. They provide resistance to the large deformation of cell walls in the form of structural microfibrils. The structure of beta-glucans is more complex. They mainly contain β 1,3 and β 1,6 linkages, forming secondary microfibrils (Ruiz-Herrera and Ortiz-Castellanos, 2019).
Chitin
Chitin is the innermost layer of the fungi cell wall that can provide reinforcement and strength. Chitin is a biopolymer composed of [β(1–4) linked N-acetyl-2-amino-2-deoxy-d-glucose] units (Dhillon et al., 2013). Chitin is a structural polymer made by the smaller monomers to form strong fibers. When secreted inside or outside of cells in an organized way, the fibers form weak bonds between each other, which increases the strength of the entire structure (Karana et al., 2018). The development of the application for chitosan has expanded rapidly in recent years, especially in wound healing (Jones et al., 2020a). Even though chitin can be obtained from crustacean shells, the fungi still show many advantages (Dhillon et al., 2013), especially because they are not limited to season and location. Table 1 summarized the advantages and disadvantages of obtaining chitosan from crustacean shells and fungi.
Some evidence shows that the linkages between chitin and glucan in the fungi are covalent bonds (Sietsma and Wessels, 1979; Kollár et al., 1997; Heux et al., 2000). The insoluble glucans in mushrooms, yeast, and hyphae have slight differences. However, β-glucans exhibiting a (1,3) or (1,6) branching for the backbone are associated with chitin in mycelium (Latgé, 2007). These are called β-(1–3)-(1–6)-glucans, which have chemical structures very similar to cellulose, which is β-(1–4)-glucan (Zhou et al., 2021). The location of chitin in different fungi is different; it is concentrated in the bud scar in yeast and the cell wall of most other fungi (Bartnicki-Garcia and Nickerson, 1962; Karimi and Zamani, 2013). Especially in the fungal species of Zygomycota, chitin and chitosan are simultaneously co-synthesized (Bartnicki-Garcia and Nickerson, 1962; Karimi and Zamani, 2013). Compared with fungal chitin, the crustacean chitin contains minerals that require an acidic extraction step for removal, thus degrading the chitin in the process. Crustacean chitin typically binds with sclerotized proteins and minerals and contains minimal residual protein. Such a difference makes the isolation procedure for fungal chitin nanofibers very simple, requiring just brief mechanical agitation in a kitchen blender after a mild alkaline treatment to remove proteins (Fazli Wan Nawawi et al., 2019; Nawawi et al., 2020). However, the glucan associates with fungal chitin can occur in quantities exceeding the chitin content itself (Hackman, 1960; Attwood and Zola, 1967; Kramer et al., 1995; Percot et al., 2003; Muzzarelli, 2011). Moreover, the extracted chitin can be of different secondary structures (as α, β, and γ chitin), except for the most common polymorph α-chitin, squid pen, sea tube worms, and some algae contain the β-chitin (Rinaudo, 2006). Figure 6 shows the molecular structure of α chitin and β chitin. The main difference between α chitin and β chitin is the secondary structure, as the neighboring chains of the α chitin are in antiparallel directions. In contrast, the chains are parallel in the β chitin (Figure 6) (Rinaudo, 2006). Moreover, the γ chitin has chains both in parallel and antiparallel (Rinaudo, 2006). Such a structural difference causes the adjacent amide groups between the neighboring chains in parallel for the α chitin, but they are not parallel for the β chitin, associating with the flexibility of the β chitin (Elieh-Ali-Komi and Hamblin, 2016).
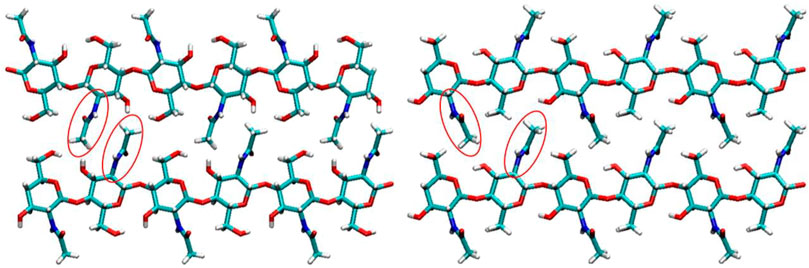
FIGURE 6. The molecular structure of α chitin (left) and β chitin (right). Each atom is colored according to its type, with red for oxygen, cyan for carbon, blue for nitrogen, and white for hydrogen.
Material Functions
Mechanical Properties
The mechanical properties of mycelium-based bio-composites are crucial for their application to engineering fields. Since the network structure of mycelium within the composited is primarily determined by the species of the fungi and the substrate that are used to manufacture mycelium, it can be very different by comparing different studies. Table 2 summarizes the test results for the MBF and MBSC in various studies. We can see the material density significantly deviate from one test to another. Generally, the higher material density leads to a high Young’s modulus and strength, as is shown in most cellular materials (Gibson, 2012), while the mechanics of the mycelium composites seem to be very different once compared across various studies. The substrate is one of the important reasons that affects the density of the mycelium-based composite. Typically, the higher proportion of grain (fibers, husks, or wood pulp) contained in the substrate will lead to a higher density (Arifin and Yusuf, 2013; Dhillon et al., 2013). Another reason is the different mycelium species used in various studies.

TABLE 2. The mechanical properties of mycelium-based composites. MBF = mycelium-based foam; MBSC = mycelium-based sandwich composite.
Compressive strength is the capacity of a material or structure to withstand loads tending to compress the material, which is an essential feature for the mycelium-based composite that can be used as package and construction material. López-Nava et al. (2016) focus on characterizing the mechanical property of MBF (substrate: common wheat stalks; fungi: Pleurotus sp). They stated that the compressive strength of MBFs is always lower than synthetic polymer foam of the same density as the water absorption can significantly affect the compressive strength, and both the substrate and mycelium absorb a large amount of water. Moreover, Silverman (2018) stated that by using the fiber (e.g., psyllium husk) in the substrate, MBF of higher compressive strength can be obtained from different species of mushrooms. Besides using fabric to increase the compressive strength, they also use chicken feathers in the substrate. The feathers will not be degraded during mycelium growth since they are primarily composed of keratin protein of durability. Still, they are lightweight, hydrophobic, and can provide structural support for the composite and contribute to its mechanics. They reported that the compressive strength of the composite significantly increases with the same density (Silverman, 2018).
Flexural strength is the stress at the fracture point for the sample in bending. It is also called modulus of rupture, or bend strength, or transverse rupture strength. López-Nava et al. investigated that the range of the flexural strength of MBF is lower than the synthetic polymer foams with the same density, while the tensile strength is much larger than the synthetic ones. (López-Nava et al., 2016). However, Appels et al. (2019) get the opposite result. The authors tested the mycelium-based bio-composite, which is created by Trametes multicolor and Pleurotus ostreatus grows on the rapeseed straw and beech sawdust. The results show that its flexural strength is larger than that of the synthetic polymer foam (Appels et al., 2019). The authors suggest that the effect is caused by the contrasting mechanics between the substrate and the mycelium fibers. The mycelium fibers are more elastic than the colonized substrate particles and, therefore, will contribute to the flexibility of the composite and may only rupture at a high strain. Moreover, the authors think that the tensile strength is not significantly affected by the species of substrate and fungi but can be affected by the pressing method, as heat pressing can substantially increase the tensile strength (Appels et al., 2019).
As mentioned previously, density is one of the essential factors that can affect mechanical properties. Islam et al. (2017) test the mycelium samples by using tension and compression. In the tension test, the mycelium shows the linear elastic at the low strain and then yields and undergoes strain hardening before rupture. In the compression test, the mycelium shows behavior like the open-cell foam. The stress-strain curve shows the linear-elastic at the beginning, followed by a plateau with a softened response. The mycelium exhibits strain-related hysteresis and stress softening effects between each cycle when subjected to continuous loading and unloading cycles (with their mechanical features summarized in Table 2) (Islam et al., 2017).
Ziegler et al. (2016) reported an MBSC with a core made of hemp pith and cotton mat. The surface binding fabric is made of a generic, such as a natural fiber fabric like burlap. As mentioned before, the authors use the same approach as Jiang et al. (2017). They put the natural fiber fabric on both sides of the pre-inoculated active mycelium-based bio-composite foam. The mycelium as a natural glue that will continue growing to connect both sides of the natural fiber fabric. The fiber surface increases the compressive strength and gives a high tensile strength to MBSC. However, Young’s modulus does not achieve the highest value of MBF (Ziegler et al., 2016) (Table 2). Jiang et al. (2017) discussed using different fibers from the MBF surface to make the MBSC. By forming a tight mycelium net, the fungus was able to cement the fabric layers firmly. The results show that flax, rather than jute or cellulose, is more efficient for colonization and yields higher mycelium production. The ultimate strength and yield stress of the samples produced with flax surface layers (35 and 27 kPa, respectively) are almost double that of the samples produced with jute (20 and 12 kPa, respectively) or cellulose surface layers (16 and 15 kPa, respectively) (Jiang et al., 2017).
Researchers have tested the mechanical properties of the mycelium fibrous film. Haneef et al. (2017) used G. lucidum and P. ostreatus to grow on the pure cellulose and cellulose-potato dextrose broth (PDB), which can get four different combinations of the mycelium. Generally, P. ostreatus fibers are stiffer (i.e., higher Young’s modulus as summarized in Table 3) than G. lucidum fibers, which have a lower critical strain which refers to the percentage of elongation of the material at the break. On the other hand, critical stress, which refers to the ultimate stress level at the break, is hardly affected by the mycelium species (Haneef et al., 2017). It is also noted that the existence of PDB can make the mycelium fibrous film softer but more stretchable (i.e., lower Young’s modulus and higher critical strain).

TABLE 3. Summary of main properties of mycelium fibers (Haneef et al., 2017).
In preparing this review paper, we perform our mechanical test on samples taken from the skin and middle parts of Pleurotus eryngii mushrooms (king oyster, as shown in Figures 7A, B) to better understand the mechanics of mycelium with different water content and thus material density. We use an Instron 5,966 machine (10 KN static load cell, 1 KN pneumatic grips with 90 psi holding pressure) to stretch all the material samples to get their stress-strain curves in tension. We measure the initial sample length as the distance between the edges of the two grips as L0, zero the force before clamping, and zero the displacement before the test. The lower grips during the test are fixed by a pin, and the upper grips move at a constant displacement speed of
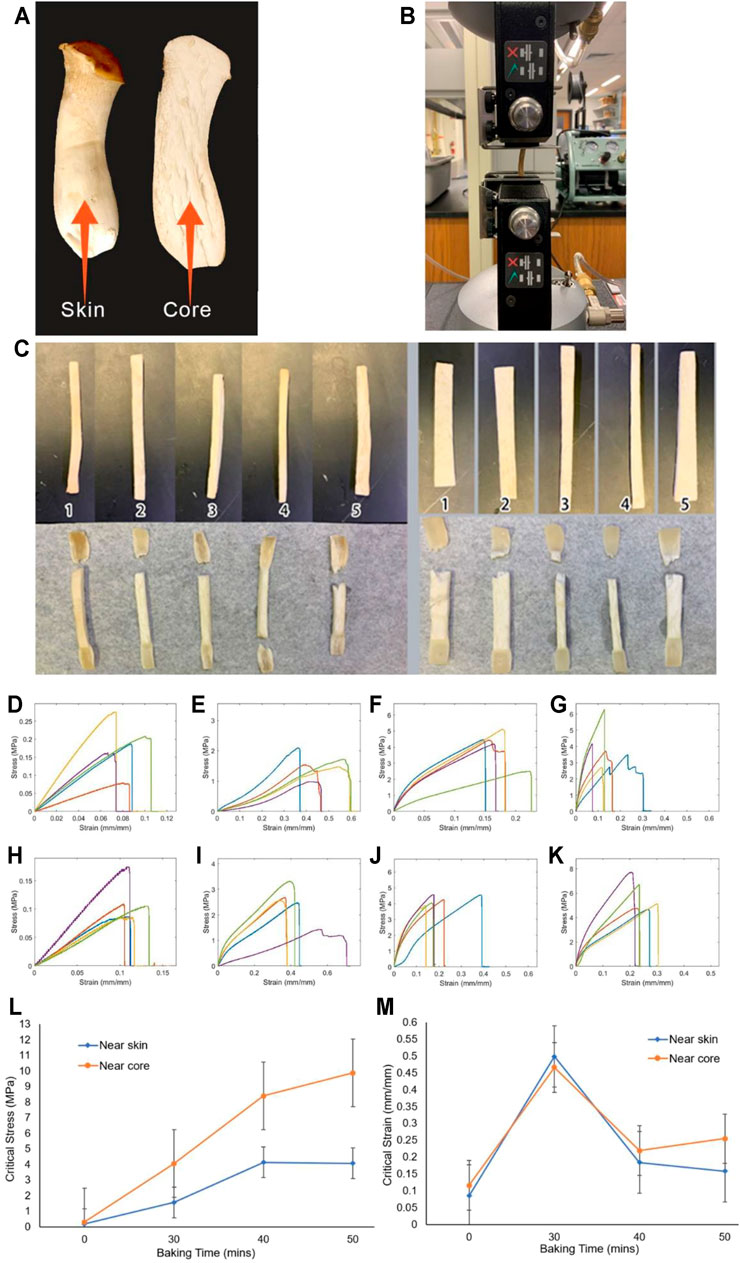
FIGURE 7. Tensile tests of Pleurotus eryngii samples after low-temperature baking (A). Snapshots of Pleurotus eryngii mushroom and the location where we obtain the skin and core samples (B). Snapshot of the Instron machine for tensile test (C). The natural Pleurotus eryngii (without baking and water loss) near the skin (left) and core (right) samples before (upper) and after (lower) the tensile test (D–G). Stress-strain curve of different baking time of samples near the skin, with baking time of 0, 30, 40, and 50 min, respectively (H–K). Stress–strain curve of different baking times of samples near the core, with baking time of 0, 30, 40, and 50 min, respectively (L). Critical stress of king oyster mushrooms as a function of baking time for samples at skin and core (M). Critical strain of king oyster mushrooms as a function of baking time for samples at skin and core.
Our mechanical testing results are shown in Figures 7C–M for the snapshot of the natural sample before and after tensile test, as well as the stress-strain curves of these samples after baking with different amounts of time that correspond to a certain amount of water loss (Figure 3). It is shown that the samples in tensile loading fail by generating zigzag surfaces at the breaking point after necking taking place, suggesting the ductile failure of the natural samples govern by the sliding failure between mycelium fibers (Figure 7C). The stress-strain curves of samples obtained after a certain amount of baking time are summarized in Figures 7D–K. We summarize all the key mechanical features that can be learned from the stress-strain curves in Figures 7L–M, and Table 4. It is shown in Figure 7L that while the critical stress monotonically increases with the bake time, as well as the water loss (Figure 3), of the skin and core samples, the critical strain of the mushroom sample after baking for 30 min with 31 and 35% of water loss is larger than the other samples (Figure 7M). It is unclear why the critical stress keeps increasing for drier samples, but the critical strain increases up to 30% of water loss and then decreases afterward. The interaction between chitin and water may strongly contribute to this phenomenon, as water can play a crucial role in turning a physical interface from ductile to brittle in mechanical loading, as observed in collagen and wood materials (Qin et al., 2012; Jin et al., 2015).
Biomedical Application
The biomedical properties of chitin and chitosan of their healing mechanisms and advanced wound-treatment methods have been proven through some research (Jones et al., 2020a).
Normally, the chitin can be obtained by the exoskeletons of crabs and prawns. However, the crustacean-derived chitin is limited by seasonal and regional variations and cannot be obtained anytime. In the meantime, the fungi-derived chitin academic and business interests are increasing. Even though the content of chitin is lower than the crustaceans, it provides a good alternative source. The fungi-derived chitin does not require strong acid to remove calcium carbonate and other minerals (Di Mario et al., 2008; Hassainia et al., 2018). Moreover, the fungi-derived chitin generates a natural nano-composite structure by branched β-glucan. It not only provided rigidity to the chitin but also can produce strong fiber networks when extracted (Di Mario et al., 2008; Fazli Wan Nawawi et al., 2019).
Jones stated how chitin and chitosan can improve wound healing. There are four stages of wound healing: hemostasis, inflammation, proliferation, and remodeling (Guo and DiPietro, 2010). The first stage is called the blood clot. In this stage, chitosan forms a coagulum with red blood cells to improve the rate of clotting (Malette et al., 1986). The second stage is called inflammatory. In this stage, the macrophage will consume dead cells, attract fibroblasts, and support skin and blood vessel replacement and synthesis of the extracellular matrix. Chitin and chitosan can attract macrophages to help the reaction in this stage (Ueno et al., 1999; 2001b). The third stage is called proliferative, and in this stage, the function of fibroblasts is the reformation of the dermis and synthesis of the extracellular matrix. Chitosan increases IL-8 production in fibroblasts, the IL-8 is an essential regulator of keratinocyte migration and proliferation (Ueno et al., 2001a). The keratinocyte, an essential cell of the last stage for wound healing, can help the reformation of the epidermis (Jones et al., 2020a).
Other Engineering Applications
Pelletier et al. (2013) tested the mycelium-based foam with different substrates, and even under the worst-property samples, the acoustic absorption rate at 1,000 Hz exceeded 70–75%. The comparison between the audio frequency spectrum shows that the absorption rate is highest when the substrate is composed of 50% switchgrass and 50% sorghum (Pelletier et al., 2013).
According to Jones et al. (2018), when the surface layer of MBF becomes carbon, the mycelium-based foam passivation occurs. Charcoal delays the generation and diffusion of smoke and reduces thermal conductivity. Especially the composite that contains the glass fines shows the best fire resistance because of its much higher silica concentration, making it less combustible (Jones et al., 2018). Moreover, some authors discussed the thermal properties from the molecular scale. As a unique protein in fungi, hydrophobin associates with cell wall morphogenesis, hydrophobicity, and substrate adhesion in both water and air environments (Wösten, 2001; Zampieri et al., 2010). Despite their small amounts, these proteins represent an important driver of the interfacial function of mycelium. It has been reported that hydrophobic is beneficial to the production of thermally stable carbonaceous structures when applied to cotton fabrics and has been used as a natural flame retardant for textile coatings (Alongi et al., 2014). The protein works by reducing the release of volatile substances that would hinder complete combustion but favor carbonization (Alongi et al., 2014; Appels et al., 2018; Willsey et al., 2020).
For the materials applied to the construction industry, besides studying acoustic absorption and thermal insulation of mycelium bio-composite material, enhancing the resistance to pests in mycelium-based bio-composites is also crucial. The mycelium-based bio-composites are mainly used for substrate containing cellulose that is prone to termite attack. Bajwa et al. (2017) used four termiticides: vetiver oil, guayule resin, cedar oil, and borax. The results showed that natural oils have a strong potential to act as effective termiticides in cellulosic fiber-based composites bonded with mycelium. Vetiver oil, cedar oil, and guayule resin exhibited variable repellency toward termite attack. Commercial termiticide borax at 10% concentration was the least effective, resulting in the highest weight loss. Corn stover fiber as a base material was preferred by termites than kenaf and hemp pith. The termites did not show any preference for fungus types. Overall, the lowest weight loss was recorded for guayule resin-treated kenaf pith-based biocomposites (Bajwa et al., 2017).
Modeling and Simulation
Further development of the mycelium composite materials requires modeling work that helps us to quantitatively understand the relationships between the environmental factors, multiscale structures, and the material functions of the mycelium composite materials. From the simple mechanical aspect, by taking the mycelium composite as a cellular material and studying its constitutive law as well as its mechanics as a function of density can be useful to guide the design and application of mycelium composite. A multiscale model of the mycelium network is necessary. It is composed of specific chemical structures and microstructures of each fiber and the whole network that can predict the mechanical response of the mycelium composite in different loading conditions. Examples of mycelium models mainly focus on two scales: the mycelium network (microscale) and the stochastic continuum (macroscale).
Islam et al. state that the most appropriate model would be a random fiber network with stochastic fiber diameter and mechanical properties (Islam et al., 2017). However, such a model includes many fibers, which is a complex problem that requires massive molding and simulation effort. To solve this problem, the authors use a stochastic continuum with a finite element model to represent the macroscopic scale of the samples. The density and the mechanical behavior are allowed to change from sub-domain to sub-domain on this scale, with a characteristic length scale. Figure 8 shows the representative network configuration and the finite element model containing 8,000 sub-domains. The macroscopic mycelium mechanical behavior can be obtained by a 3D stochastic continuum model based on the representative network configuration. Each subdomain is assigned a network density sampled from the distribution (Islam et al., 2017). This finite element model only can represent the relationship between the change of density of the mycelium-based bio composite and the change of strain-stress curve. Since this model focuses on the macroscale, it lacks the discussion of connection to the mesoscale structure and the molecular structure of mycelium network. Shinde et al. use a different approach, which is intermediate-scale to modeling the mycelium growth (Shinde et al., 2020). They focus on the individual hypha modeled as a growing one-dimensional (1D) lattice, and a single source of nutrients to generate a single-colony mycelium as a growing two-dimensional morphology. They discussed a small driven lattice gas model. This model generates the morphological characteristics associated with single-colony mycelium arising from the growth and branching process of fungal hyphae, which is fed by a single source of nutrients. The 1D model defined the growth characteristics of the primary hypha and the 2D model describes the single fungal hyphae elongation and branching to generate an entire single-colony mycelium (Shinde et al., 2020).
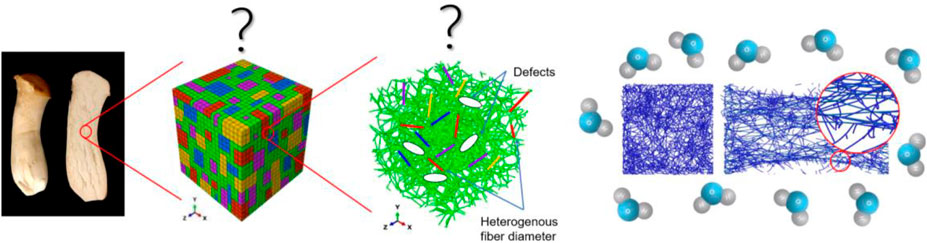
FIGURE 8. Multiscale model of mycelium network and its composite from the finite element model (Islam et al., 2017) (reproduced under a creative commons attribution license) to fibrous networks and its interface with water and other materials in the substrates.
Those two models help to understand the structure-function relationship of the mycelium network from two different scales. They provide valuable insights to the growth of the mycelium network and its mechanical properties. However, these models are limited to be applied to certain aspects of a mycelium study, while a comprehensive multiscale model should connect the molecular composition of mycelium fiber and its interaction to water and substrate particles to the mechanics of the mycelium network and its composite materials. It should also allow us to run simulations and see how the material responds to different external loading conditions and how the molecular interaction and environmental factors from one end may affect the material function at the other end. To achieve these purposes, the following points need to be considered. We built a model of mycelium-based composite based on both accurate geometry and mechanical properties, allowing us to analyze the influence of density and mechanics on the mycelium fiber mechanical response. By varying the number, type, and mechanical properties of mycelium fibers and performing tensile tests on the models, we determined the fiber failure and post-failure deformation for plastic deformation after yielding. Since the water content is also an important factor that can affect the mycelium-based bio-composite mechanical properties, the effect of water on the mechanics of fiber (viscoelastic) and network (drag force from water in deformation) also needs to be considered. Moreover, the coarse-grained models composed of actual mycelium fibers can be used to simulate the mechanical behavior of mycelium network. It provides a more accurate description of the network distortion in loading than a finite element model can do. The single fiber deformation may also connect to molecular simulation, which helps to understand the interfacial interaction between different material phases (e.g., chitin, glucan, protein, and water, etc., Figure 5).
Discussion and Conclusion
Unlike protein or protein-based biological materials (e.g., silk, collagen, cytoskeleton, etc.), less attention is paid to microorganisms and their multiscale structures. Studying mycelium and their composite materials can help to understand the mechanics of the fungus network, its biological function, and its application to produce green composite materials with both good mechanics and lightweight, in both simulation and corresponding experiments for synthesis (Holt et al., 2012; Haneef et al., 2017; Jiang et al., 2017; Girometta et al., 2019; Attias et al., 2020). A method to grow and process mycelium-based composites can lead to a promising and innovative way to produce building materials from using the agricultural method (Attias et al., 2020). The study of molecular composition and biological function in the mycelium network may facilitate the discovery of new drugs produced by a fungus with certain biological functions or inspire the design of the topology of the internet of things with low power consumption and the function of a fast-response to pests and diseases (Muzzarelli et al., 2007; Naseri et al., 2014; Morin-Crini et al., 2019; Wang et al., 2019; Jones et al., 2020a).
As an alternative environmentally friendly material over synthetic foams, mycelium composite shows its advantage in several engineering applications (e.g., packaging materials, acoustic and thermal insulation boards) and is receiving more attention. Producing such material is still a pioneering field and the standardized process to yield optimized material property has yet to be identified. This bio-composite material has the ability to be widely used in furniture, agriculture, civil engineering, and the biomedical field. In general, in terms of mechanical properties, the mycelium composites show properties different from synthetic polymer foams or natural cellular materials. Their mechanics are not simply defined by the processing method at the end of its production but as the collective result of the fungi species, their substrate, and related environments during the growth. The properties of the substrate define the mechanics of the matrix material within the composite. The mycelium network itself is affected by the composition and structure of the substrate. Moreover, since both the mycelium and substrate can absorb water, the water content of the final composite is also crucial. Usually, a hot press process can help to remove the water and inactivate the mycelium, effectively preventing it from growing during application. However, due to the wide range of available parameters, results are often incomparable among different studies. For example, compared with the most important competitor traditional material (EPS), the mycelium-based bio-composite has not shown a lot of advantages.
In addition to be used as bio-composites because of its mechanics, mycelium is rich in chitin, which provides reinforcement and strength to cell walls. The interfacial interaction between chitin to other components, and how water plays an intermediate role, needs molecular modeling and analysis at the fundamental length scale. Moreover, the chitin purified from crustacean shells has been widely used in biological applications, such as wound healing. Even though the mycelium cell wall contains a lot of chitin that can be gained without geographic and seasonal limitations, the applications of the chitin purified from mycelium is not as wildly used as that from crustacean shells, which requires more research and attention.
Even though the mycelium-based composites show advantages for their mechanics, lightweight, and many environmentally friendly features, they have limitations and challenges for their large-scale applications. For instance, as a biomaterial, its production is less standardized than conventional engineering materials such as steel, cement, and polymer, and it is not clear how to customize the types of substrates for the certain species of fungi to maximize the yield of mycelium and to optimize the composite mechanics. However, since there are over one million species (Blackwell, 2011), testing the microstructure and mechanics for each of them is extremely difficult and we may need to investigate the structure-mechanics relationship of different classes of fungi (by type of rot, type of hyphae, gene, etc.) to identify the most promising species of yielding composite with the best mechanics. Moreover, unlike polymer foams, mycelium-based biocomposites cannot be massively produced within a short time by machines, as growing the mycelium needs about 2 weeks or more time. It is important to automatically control the growing factors, including temperature, humidity, supplied nutrition, and light within an incubating environment without direct usage of human labor during its growth. It is also not clear how each of its constituting building blocks contributes to its interface to wood fibers and thus affects the integrity of the fibrous network of the composite. These limitations are crucial before supplying the material to the architect and there are broader industrial applications.
Studying mycelium can go broadly beyond material usage. As the vegetative part of a fungus, mycelium has the unique capability to utilize discrete agricultural wastes as substrates for the growth of its network, which integrates the wastes from pieces to continuous composites without energy input or generating extra waste (Jones et al., 2018; Appels et al., 2019; Girometta et al., 2019). Besides fixing pieces of the soil, mycelium in nature has a more important function as an information highway that speeds up interactions between a diverse population of plants (Simard et al., 2012; Gorzelak et al., 2015; Fricker et al., 2017). It allows individual plants that are widely separated to effectively defend themselves against pests and diseases by communication and exchange matters (Babikova et al., 2014). The study of mycelium-based composite, as to how it integrates different discrete blocks and achieves material functions that none of the building blocks can achieve by themselves, goes beyond the mechanics of material study, and becomes the main reason we want to understand more about the mycelium network and its biological functions. The current point, the functions of the mycelium network are of the interest to primary ecologists, while how exactly the chemical signals are conducted in the hierarchical structure of the mycelium network and how its effectiveness relates to the geometry and topology of the network are still unknown, as well as how such knowledge may contribute knowledge to the topology of the Internet and the internet of things, or innovative Internet media with low energy consumption. Most of these questions need to be addressed with interdisciplinary efforts and some of them may be answered by developing a multiscale model of the mycelium network and use it in related simulations. We will study its application to produce green composite materials but will also generate knowledge to design an information network system.
Author Contributions
ZQ had the conception and designed the structure of the work, LY performed the experiment, collect data and draft the article. ZQ and LY performed the data analysis and interpretation. All the authors contributed to the writing of this work.
Conflict of Interest
The authors declare that the research was conducted in the absence of any commercial or financial relationships that could be construed as a potential conflict of interest.
Publisher’s Note
All claims expressed in this article are solely those of the authors and do not necessarily represent those of their affiliated organizations, or those of the publisher, the editors and the reviewers. Any product that may be evaluated in this article, or claim that may be made by its manufacturer, is not guaranteed or endorsed by the publisher.
Acknowledgments
The authors acknowledge the Collaboration for Unprecedented Success and Excellence (CUSE) Grants (II-35–2020, II-45–2020) at Syracuse University for supporting the research work.
References
Alongi, J., Carletto, R. A., Bosco, F., Carosio, F., Di Blasio, A., Cuttica, F., et al. (2014). Caseins and Hydrophobins as Novel green Flame Retardants for Cotton Fabrics. Polym. Degrad. Stab. 99, 111–117. doi:10.1016/j.polymdegradstab.2013.11.016
Andlar, M., Rezić, T., Marđetko, N., Kracher, D., Ludwig, R., and Šantek, B. (2018). Lignocellulose Degradation: An Overview of Fungi and Fungal Enzymes Involved in Lignocellulose Degradation. Eng. Life Sci. 18, 768–778. doi:10.1002/elsc.201800039
Appels, F. V. W., Camere, S., Montalti, M., Karana, E., Jansen, K. M. B., Dijksterhuis, J., et al. (2019). Fabrication Factors Influencing Mechanical, Moisture- and Water-Related Properties of Mycelium-Based Composites. Mater. Des. 161, 64–71. doi:10.1016/j.matdes.2018.11.027
Appels, F. V. W., Dijksterhuis, J., Lukasiewicz, C. E., Jansen, K. M. B., Wösten, H. A. B., and Krijgsheld, P. (2018). Hydrophobin Gene Deletion and Environmental Growth Conditions Impact Mechanical Properties of Mycelium by Affecting the Density of the Material. Sci. Rep. 8, 4703. doi:10.1038/s41598-018-23171-2
Aranaz, I., Mengibar, M., Harris, R., Panos, I., Miralles, B., Acosta, N., et al. (2009). Functional Characterization of Chitin and Chitosan. Ccb 3, 203–230. doi:10.2174/2212796810903020203
Arantes, V., and Goodell, B. (2014). Current Understanding of Brown-Rot Fungal Biodegradation Mechanisms: A Review. ACS Symp. Ser. 1158, 3–21. doi:10.1021/bk-2014-1158.ch001
Arifin, Y. H., and Yusuf, Y. (2013). Mycelium Fibers as New Resource for Environmental Sustainability. in Proced. Eng. 53, 504–508. doi:10.1016/j.proeng.2013.02.065
Attias, N., Danai, O., Abitbol, T., Tarazi, E., Ezov, N., Pereman, I., et al. (2020). Mycelium Bio-Composites in Industrial Design and Architecture: Comparative Review and Experimental Analysis. J. Clean. Prod. 246, 119037. doi:10.1016/j.jclepro.2019.119037
Attwood, M. M., and Zola, H. (1967). The Association between Chitin and Protein in Some Chitinous Tissues. Comp. Biochem. Physiol. 20, 993–998. doi:10.1016/0010-406X(67)90069-2
Azimi, B., Ricci, C., Fusco, A., Zavagna, L., Linari, S., Donnarumma, G., et al. (2021). Electrosprayed Shrimp and Mushroom Nanochitins on Cellulose Tissue for Skin Contact Application. Molecules 26, 4374. doi:10.3390/molecules26144374
Azimi, B., Thomas, L., Fusco, A., Kalaoglu-Altan, O. I., Basnett, P., Cinelli, P., et al. (2020). Electrosprayed Chitin Nanofibril/electrospun Polyhydroxyalkanoate Fiber Mesh as Functional Nonwoven for Skin Application. Jfb 11, 62. doi:10.3390/jfb11030062
Babikova, Z., Johnson, D., Bruce, T., Pickett, J., and Gilbert, L. (2014). Underground Allies: How and Why Do Mycelial Networks Help Plants Defend Themselves? BioEssays 36, 21–26. doi:10.1002/bies.201300092
Bajwa, D. S., Holt, G. A., Bajwa, S. G., Duke, S. E., and McIntyre, G. (2017). Enhancement of Termite (Reticulitermes Flavipes L.) Resistance in Mycelium Reinforced Biofiber-Composites. Ind. Crops Prod. 107, 420–426. doi:10.1016/j.indcrop.2017.06.032
Baldrian, P., and Valášková, V. (2008). Degradation of Cellulose by Basidiomycetous Fungi. FEMS Microbiol. Rev. 32, 501–521. doi:10.1111/j.1574-6976.2008.00106.x
Bansal, V., Ahmad, A., and Sastry, M. (2006). Fungus-mediated Biotransformation of Amorphous Silica in rice Husk to Nanocrystalline Silica. J. Am. Chem. Soc. 128, 14059–14066. doi:10.1021/ja062113+
Bartnicki-Garcia, S. (1968). Cell wall Chemistry, Morphogenesis, and Taxonomy of Fungi. Annu. Rev. Microbiol. 22, 87–108. doi:10.1146/annurev.mi.22.100168.000511
Bartnicki-Garcia, S., and Nickerson, W. J. (1962). Isolation, Composition, and Structure of Cell walls of Filamentous and Yeast-like Forms of Mucor Rouxii. Biochim. Biophys. Acta 58, 102–119. doi:10.1016/0006-3002(62)90822-3
Bayer, E., and McIntyre, G. (2012). PATENT: Substrate Composition and Method for Growing Mycological Materials, 1, 2. (Ecovative+Design+Llc), Available at: https://patents.google.com/patent/US20120315687A1/en?assignee=Ecovative+Design+Llc&oq=assignee:
Bayer, E., and McIntyre, G. (2015). Method For Growing Mycological Materials, 4. Available at: https://patents.google.com/patent/US20150247115A1/en.
Bhuvaneshwari, S., Hettiarachchi, H., and Meegoda, J. (2019). Crop Residue Burning in India: Policy Challenges and Potential Solutions. Ijerph 16, 832. doi:10.3390/ijerph16050832
Blackwell, M. (2011). The Fungi: 1, 2, 3 … 5.1 Million Species? Am. J. Bot. 98, 426–438. doi:10.3732/ajb.1000298
Butterfield, B., Chapman, K., and Christie, L. (1992). Ultrastructural Characteristics of Failure Surfaces in Medium Density Fiberboard. For. Prod. J. 42, 55–60.
Carvalho, L. M. H., and Costa, C. A. V. (1998). Modeling and Simulation of the Hot-Pressing Process in the Production of Medium Density Fiberboard (MDF). Chem. Eng. Commun. 170, 1–21. doi:10.1080/00986449808912732
Corner, E. J. H. (1953). The construction of polypores. 1. Introduction-polyporus-sulphureus, p-squamosus, p-betulinus and polystictus-microcyclus. Phytomorphology 3, 152–167.
Couturier, M., and Berrin, J.-G. (2013). “The Saccharification Step: The Main Enzymatic Components”. in Lignocellulose Conversion: Enzymatic and Microbial Tools for Bioethanol Production. Heidelberg, Germany: Springer. doi:10.1007/978-3-642-37861-4_5
Dahmen, J. (2017). Soft Futures: Mushrooms and Regenerative Design. J. Architectural Educ. 71, 57–64. doi:10.1080/10464883.2017.1260927
Dai, C., Yu, C., Xu, C., and He, G. (2007). Heat and Mass Transfer in wood Composite Panels during Hot Pressing: Part 4. Experimental Investigation and Model Validation. Wood Fiber Sci. 61, 83–88. doi:10.1515/HF.2007.013
Danti, S., Trombi, L., Fusco, A., Azimi, B., Lazzeri, A., Morganti, P., et al. (2019). Chitin Nanofibrils and Nanolignin as Functional Agents in Skin Regeneration. Ijms 20, 2669. doi:10.3390/ijms20112669
Defonseka, C. (2019). Introduction to Polymeric Composites with rice Hulls. Berlin, Germany: De Gruyter. doi:10.1515/9783110643206
Dhillon, G. S., Kaur, S., Brar, S. K., and Verma, M. (2013). Green Synthesis Approach: Extraction of Chitosan from Fungus Mycelia. Crit. Rev. Biotechnol. 33, 379–403. doi:10.3109/07388551.2012.717217
Di Mario, F., Rapanà, P., Tomati, U., and Galli, E. (2008). Chitin and Chitosan from Basidiomycetes. Int. J. Biol. Macromolecules 43, 8–12. doi:10.1016/j.ijbiomac.2007.10.005
Elieh-Ali-Komi, D., and Hamblin, M. R. (2016). Chitin and Chitosan: Production and Application of Versatile Biomedical Nanomaterials. Int. J. Adv. Res. (Indore) 4, 411–427. Available at: http://www.ncbi.nlm.nih.gov/pubmed/27819009%0Ahttp://www.pubmedcentral.nih.gov/articlerender.fcgi?artid=PMC5094803.
Elsacker, E., Vandelook, S., Brancart, J., Peeters, E., and De Laet, L. (2019). Mechanical, Physical and Chemical Characterisation of Mycelium-Based Composites with Different Types of Lignocellulosic Substrates. PLoS One 14, e0213954. doi:10.1371/journal.pone.0213954
Erjavec, J., Kos, J., Ravnikar, M., Dreo, T., and Sabotič, J. (2012). Proteins of Higher Fungi - from forest to Application. Trends Biotechnol. 30, 259–273. doi:10.1016/j.tibtech.2012.01.004
Fazli Wan Nawawi, W. M., Lee, K.-Y., Kontturi, E., Murphy, R. J., and Bismarck, A. (2019). Chitin Nanopaper from Mushroom Extract: Natural Composite of Nanofibers and Glucan from a Single Biobased Source. ACS Sustain. Chem. Eng. 7, 6492–6496. doi:10.1021/acssuschemeng.9b00721
Fricker, M., Boddy, L., and Bebber, D. (2007). “Network Organisation of Mycelial Fungi”. In Biology Of the Fungal Cell, 309–330. doi:10.1007/978-3-540-70618-2_13
Fricker, M. D., Heaton, L. L. M., Jones, N. S., and Boddy, L. (2017). The Mycelium as a Network. The Fungal Kingdom, 335–367. doi:10.1128/9781555819583.ch15
Gibson, L. J., and Ashby, M. F. (1982). The Mechanics of Three-Dimensional Cellular Materials. Proc. R. Soc. Lond. A. 382, 43–59. doi:10.1098/rspa.1982.0088
Gibson, L. J. (2012). The Hierarchical Structure and Mechanics of Plant Materials. J. R. Soc. Interf. 9, 2749–2766. doi:10.1098/rsif.2012.0341
Girometta, C., Picco, A. M., Baiguera, R. M., Dondi, D., Babbini, S., Cartabia, M., et al. (2019). Physico-mechanical and Thermodynamic Properties of Mycelium-Based Biocomposites: A Review. Sustainability 11, 281. doi:10.3390/su11010281
Girometta, C., Zeffiro, A., Malagodi, M., Savino, E., Doria, E., Nielsen, E., et al. (2017). Pretreatment of Alfalfa Stems by wood Decay Fungus Perenniporia Meridionalis Improves Cellulose Degradation and Minimizes the Use of Chemicals. Cellulose 24, 3803–3813. doi:10.1007/s10570-017-1395-6
Gorzelak, M. A., Asay, A. K., Pickles, B. J., and Simard, S. W. (2015). Inter-plant Communication through Mycorrhizal Networks Mediates Complex Adaptive Behaviour in Plant Communities. AoB Plants 7, plv050. doi:10.1093/aobpla/plv050
Guo, S., and DiPietro, L. A. (2010). Factors Affecting Wound Healing. J. Dent. Res. 89, 219–229. doi:10.1177/0022034509359125
Hackman, R. (1960). Studies on Chitin IV. The Occurrence of Complexes in Which Chitin and Protein Are Covalently Linked. Aust. Jnl. Bio. Sci. 13, 568. doi:10.1071/bi9600568
Haneef, M., Ceseracciu, L., Canale, C., Bayer, I. S., Heredia-Guerrero, J. A., and Athanassiou, A. (2017). Advanced Materials from Fungal Mycelium: Fabrication and Tuning of Physical Properties. Sci. Rep. 7, 41292. doi:10.1038/srep41292
Hassainia, A., Satha, H., and Boufi, S. (2018). Chitin from Agaricus Bisporus: Extraction and Characterization. Int. J. Biol. Macromolecules 117, 1334–1342. doi:10.1016/j.ijbiomac.2017.11.172
Hatakka, A., and Hammel, K. E. (2011). “Fungal Biodegradation of Lignocelluloses”. In Industrial Applications, 319–340. doi:10.1007/978-3-642-11458-8_15
Heux, L., Brugnerotto, J., Desbrières, J., Versali, M.-F., and Rinaudo, M. (2000). Solid State NMR for Determination of Degree of Acetylation of Chitin and Chitosan. Biomacromolecules 1, 746–751. doi:10.1021/bm000070y
Hoa, H. T., and Wang, C.-L. (2015). The Effects of Temperature and Nutritional Conditions on Mycelium Growth of Two Oyster Mushrooms (Pleurotus Ostreatus and Pleurotus Cystidiosus). Mycobiology 43, 14–23. doi:10.5941/MYCO.2015.43.1.14
Holt, G. A., McIntyre, G., Flagg, D., Bayer, E., Wanjura, J. D., and Pelletier, M. G. (2012). Fungal Mycelium and Cotton Plant Materials in the Manufacture of Biodegradable Molded Packaging Material: Evaluation Study of Select Blends of Cotton Byproducts. J. Biobased Mat Bioenergy 6, 431–439. doi:10.1166/jbmb.2012.1241
Islam, M. R., Omar, M., Pk, M. M. U., and Mia, R. (2015). Phytochemicals and Antibacterial Activity Screening of Three Edible Mushrooms Pleurotus Ostreatus, Ganoderma Lucidum and Lentinula Edodes Accessible in Bangladesh. Am. J. Biol. Life Sci. 3, 31–35.
Islam, M. R., Tudryn, G., Bucinell, R., Schadler, L., and Picu, R. C. (2017). Morphology and Mechanics of Fungal Mycelium. Sci. Rep. 7, 13070. doi:10.1038/s41598-017-13295-2
Jayakumar, R., Prabaharan, M., Sudheesh Kumar, P. T., V., S., V., S., and Tamur, H. (2011). Novel Chitin and Chitosan Materials in Wound Dressing. Trends Mater. Sci. (Intechopen), 1–26. doi:10.5772/13509
Jiang, L., Walczyk, D., McIntyre, G., Bucinell, R., and Li, B. (2019). Bioresin Infused Then Cured Mycelium-Based sandwich-structure Biocomposites: Resin Transfer Molding (RTM) Process, Flexural Properties, and Simulation. J. Clean. Prod. 207, 123–135. doi:10.1016/j.jclepro.2018.09.255
Jiang, L., Walczyk, D., McIntyre, G., Bucinell, R., and Tudryn, G. (2017). Manufacturing of Biocomposite sandwich Structures Using Mycelium-Bound Cores and Preforms. J. Manufacturing Process. 28, 50–59. doi:10.1016/j.jmapro.2017.04.029
Jin, K., Qin, Z., and Buehler, M. J. (2015). Molecular Deformation Mechanisms of the wood Cell wall Material. J. Mech. Behav. Biomed. Mater. 42, 198–206. doi:10.1016/j.jmbbm.2014.11.010
Jones, M., Bhat, T., Huynh, T., Kandare, E., Yuen, R., Wang, C. H., et al. (2018). Waste-derived Low-Cost Mycelium Composite Construction Materials with Improved Fire Safety. Fire Mater. 42, 816–825. doi:10.1002/fam.2637
Jones, M., Huynh, T., Dekiwadia, C., Daver, F., and John, S. (2017). Mycelium Composites: A Review of Engineering Characteristics and Growth Kinetics. j bionanosci 11, 241–257. doi:10.1166/jbns.2017.1440
Jones, M., Kujundzic, M., John, S., and Bismarck, A. (2020a). Crab vs. Mushroom: A Review of Crustacean and Fungal Chitin in Wound Treatment. Mar. Drugs 18, 64. doi:10.3390/md18010064
Jones, M., Mautner, A., Luenco, S., Bismarck, A., and John, S. (2020b). Engineered Mycelium Composite Construction Materials from Fungal Biorefineries: A Critical Review. Mater. Des. 187, 108397. doi:10.1016/j.matdes.2019.108397
Karana, E., Blauwhoff, D., Hultink, E. J., and Camere, S. (2018). When the Material Grows: A Case Study on Designing (With) Mycelium-Based Materials. Int. J. Des. 12, 119–136.
Karimi, K., and Zamani, A. (2013). Mucor Indicus: Biology and Industrial Application Perspectives: A Review. Biotechnol. Adv. 31, 466–481. doi:10.1016/j.biotechadv.2013.01.009
Kilavan Packiam, K., George, T. S., Kulanthaivel, S., and Vasanthi, N. S. (2011). Extraction, Purification and Characterization of Chitosan from Endophytic Fungi Isolated from Medicinal Plants. World J. Sci. Technol. 1, 43–44.
Ko, K. S., and Jung, H. S. (1999). Molecular Phylogeny of Trametes and Related Genera. Antonie Van Leeuwenhoek 75, 191–199. doi:10.1023/a:1001732532122
Kollár, R., Reinhold, B. B., Petráková, E., Yeh, H. J. C., Ashwell, G., Drgonová, J., et al. (1997). Architecture of the Yeast Cell Wall. J. Biol. Chem. 272, 17762–17775. doi:10.1074/jbc.272.28.17762
Kramer, K. J., Hopkins, T. L., and Schaefer, J. (1995). Applications of Solids NMR to the Analysis of Insect Sclerotized Structures. Insect Biochem. Mol. Biol. 25, 1067–1080. doi:10.1016/0965-1748(95)00053-4
Latgé, J. P. (2007). The Cell wall: A Carbohydrate armour for the Fungal Cell. Mol. Microbiol. 66, 279–290. doi:10.1111/j.1365-2958.2007.05872.x
Linder, M. B., Szilvay, G. R., Nakari-Setälä, T., and Penttilä, M. E. (2005). Hydrophobins: The Protein-Amphiphiles of Filamentous Fungi. FEMS Microbiol. Rev. 29, 877–896. doi:10.1016/j.femsre.2005.01.004
Liu, J., Lin, S., Liu, X., Qin, Z., Yang, Y., Zang, J., et al. (2020). Fatigue-resistant Adhesion of Hydrogels. Nat. Commun. 11, 1071. doi:10.1038/s41467-020-14871-3
López Nava, J. A., Méndez González, J., Ruelas Chacón, X., and Nájera Luna, J. A. (2016). Assessment of Edible Fungi and Films Bio-Based Material Simulating Expanded Polystyrene. Mater. Manufacturing Process. 31, 1085–1090. doi:10.1080/10426914.2015.1070420
Madurwar, M. V., Ralegaonkar, R. V., and Mandavgane, S. A. (2013). Application of Agro-Waste for Sustainable Construction Materials: A Review. Construction Building Mater. 38, 872–878. doi:10.1016/j.conbuildmat.2012.09.011
Malette, W. G., Quigley, H. J., and Adickes, E. D. (1986). “Chitosan Effect in Vascular Surgery, Tissue Culture and Tissue Regeneration”. In Chitin In Nature and Technology. Springer, 435–442. doi:10.1007/978-1-4613-2167-5_51
Maraveas, C. (2020). Production of Sustainable Construction Materials Using Agro-Wastes. Materials 13, 262. doi:10.3390/ma13020262
Morganti, P., and Morganti, G. (2008). Chitin Nanofibrils for Advanced Cosmeceuticals. Clin. Dermatol. 26, 334–340. doi:10.1016/j.clindermatol.2008.01.003
Morganti, P., Morganti, G., and Coltelli, M. B. (2019). “Chitin Nanomaterials and Nanocomposites for Tissue Repair,” in Marine-derived Biomaterials for Tissue Engineering Applications. Editors A. H. Choi, and B. Ben-Nissan (Singapore: Springer), 523–544. doi:10.1007/978-981-13-8855-2_21
Morin-Crini, N., Lichtfouse, E., Torri, G., and Crini, G. (2019). Applications of Chitosan in Food, Pharmaceuticals, Medicine, Cosmetics, Agriculture, Textiles, Pulp and Paper, Biotechnology, and Environmental Chemistry. Environ. Chem. Lett. 17, 1667–1692. doi:10.1007/s10311-019-00904-x
Muzzarelli, R. A. A. (2011). “Chitin Nanostructures in Living Organisms,” in “Chitin Nanostructures in Living Organisms,” in Chitin. Editor N. S. Gupta (Dordrecht: Springer), 1–34. doi:10.1007/978-90-481-9684-5_1
Muzzarelli, R. A. A., Morganti, P., Morganti, G., Palombo, P., Palombo, M., Biagini, G., et al. (2007). Chitin Nanofibrils/chitosan Glycolate Composites as Wound Medicaments. Carbohydr. Polym. 70, 274–284. doi:10.1016/j.carbpol.2007.04.008
Muzzarelli, R. A. A. (2012). Nanochitins and Nanochitosans, Paving the Way to Eco-Friendly and Energy-Saving Exploitation of Marine Resources. A Compr. Reference 10, 153–164. doi:10.1016/B978-0-444-53349-4.00257-0
Naseri, N., Algan, C., Jacobs, V., John, M., Oksman, K., and Mathew, A. P. (2014). Electrospun Chitosan-Based Nanocomposite Mats Reinforced with Chitin Nanocrystals for Wound Dressing. Carbohydr. Polym. 109, 7–15. doi:10.1016/j.carbpol.2014.03.031
Nawawi, W. M. F. B. W., Jones, M., Murphy, R. J., Lee, K.-Y., Kontturi, E., and Bismarck, A. (2020). Nanomaterials Derived from Fungal Sources-Is it the New Hype? Biomacromolecules 21, 30–55. doi:10.1021/acs.biomac.9b01141
Parisi, S., Rognoli, V., and Ayala-Garci, C. (2016). Designing Materials Experiences through Passing of Time - Material Driven Design Method Applied to Mycelium-Based Composite. in 10th International Conference on Design and Emotion - Celebration and Contemplation, 27-30, September, 2016, Amsterdam. doi:10.1115/POWER2020-16619
Pegler, D. N. (1996). Hyphal Analysis of Basidiomata. Mycol. Res. 100, 129–142. doi:10.1016/s0953-7562(96)80111-0
Pelletier, M. G., Holt, G. A., Wanjura, J. D., Bayer, E., and McIntyre, G. (2013). An Evaluation Study of Mycelium Based Acoustic Absorbers Grown on Agricultural By-Product Substrates. Ind. Crops Prod. 51, 480–485. doi:10.1016/j.indcrop.2013.09.008
Percot, A., Viton, C., and Domard, A. (2003). Characterization of Shrimp Shell Deproteinization. Biomacromolecules 4, 1380–1385. doi:10.1021/bm034115h
Pheng, L. S., and Hou, L. S. (2019). “The Economy and the Construction Industry,” in Construction Quality and the Economy. Editors L. Sui Pheng, and L. Shing Hou (Springer Singapore), 21–54. doi:10.1007/978-981-13-5847-0_2
Pochanavanich, P., and Suntornsuk, W. (2002). Fungal Chitosan Production and its Characterization. Lett. Appl. Microbiol. 35, 17–21. doi:10.1046/j.1472-765X.2002.01118.x
Qin, Z., Gautieri, A., Nair, A. K., Inbar, H., and Buehler, M. J. (2012). Thickness of Hydroxyapatite Nanocrystal Controls Mechanical Properties of the Collagen-Hydroxyapatite Interface. Langmuir 28, 1982–1992. doi:10.1021/la204052a
Qin, Z., Jung, G. S., Kang, M. J., and Buehler, M. J. (2017). The Mechanics and Design of a Lightweight Three-Dimensional Graphene Assembly. Sci. Adv. 3, e1601536. doi:10.1126/sciadv.1601536
Rinaudo, M. (2006). Chitin and Chitosan: Properties and Applications. Prog. Polym. Sci. 31, 603–632. doi:10.1016/j.progpolymsci.2006.06.001
Ruiz-Herrera, J., and Ortiz-Castellanos, L. (2019). Cell wall Glucans of Fungi. A Review. Cel Surf. 5, 100022. doi:10.1016/j.tcsw.2019.100022
Sánchez, C. (2009). Lignocellulosic Residues: Biodegradation and Bioconversion by Fungi. Biotechnol. Adv. 27, 185–194. doi:10.1016/j.biotechadv.2008.11.001
Sejian, V., Gaughan, J., Baumgard, L., and Prasad, C. (2015). “Climate Change Impact on Livestock: Adaptation and Mitigation,” in Climate Change Impact on Livestock: Adaptation and Mitigation. Editors V. Sejian, G. John, B. Lance, and P. Cadaba (New Delhi: Cadaba Springer). doi:10.1007/978-81-322-2265-1
Shinde, B., Khan, S., and Muhuri, S. (2020). Model for Growth and Morphology of Fungal Mycelium. Phys. Rev. Res. 2, 23111. doi:10.1103/PhysRevResearch.2.023111
Sietsma, J. H., and Wessels, J. G. H. (1979). Evidence for Covalent Linkages between Chitin and -Glucan in a Fungal Wall. J. Gen. Microbiol. 114, 99–108. doi:10.1099/00221287-114-1-99
Silverman, J. (2018). Development and Testing of Mycelium-Based Composite Materials for Shoe Sole Applications. Univ. Del. ProQuest Diss. Publ. Available at: https://www.proquest.com/docview/2088133051/abstract/B35964F1EEFB447APQ/1?accountid=14214.
Simard, S. W., Beiler, K. J., Bingham, M. A., Deslippe, J. R., Philip, L. J., and Teste, F. P. (2012). Mycorrhizal Networks: Mechanisms, Ecology and Modelling. Fungal Biol. Rev. 26, 39–60. doi:10.1016/j.fbr.2012.01.001
Steigerwald, B. (2014). NASA Goddard Instrument Makes First Detection of Organic Matter on Mars. NASA Available at: https://www.nasa.gov/content/goddard/mars-organic-matter.
Streit, F., Koch, F., Laranjeira, M. C. M., and Ninow, J. L. (2009). Production of Fungal Chitosan in Liquid Cultivation Using Apple Pomace as Substrate. Braz. J. Microbiol. 40, 20–25. doi:10.1590/S1517-83822009000100003
Swift, R. S. (2018). “Organic Matter Characterization”. In Methods Of Soil Analysis. Madison, WI: the Soil Science Society of America, Inc.), 1011–1069. doi:10.2136/sssabookser5.3.c35
Ueno, H., Mori, T., and Fujinaga, T. (2001a). Topical Formulations and Wound Healing Applications of Chitosan. Adv. Drug Deliv. Rev. 52, 105–115. doi:10.1016/S0169-409X(01)00189-2
Ueno, H., Nakamura, F., Murakami, M., Okumura, M., Kadosawa, T., and Fujinaga, T. (2001b). Evaluation Effects of Chitosan for the Extracellular Matrix Production by Fibroblasts and the Growth Factors Production by Macrophages. Biomaterials 22, 2125–2130. doi:10.1016/S0142-9612(00)00401-4
Ueno, H., Yamada, H., Tanaka, I., Kaba, N., Matsuura, M., Okumura, M., et al. (1999). Accelerating Effects of Chitosan for Healing at Early Phase of Experimental Open Wound in Dogs. Biomaterials 20, 1407–1414. doi:10.1016/S0142-9612(99)00046-0
Velasco, P. M., Ortiz, M. P. M., Giro, M. A. M., Castelló, M. C. J., and Velasco, L. M. (2014). Development of Better Insulation Bricks by Adding Mushroom Compost Wastes. Energy and Buildings 80, 17–22. doi:10.1016/j.enbuild.2014.05.005
Volk, T. J., and Ryvarden, L. (1992). Genera of Polypores: Nomenclature and Taxonomy: Synopsis Fungorum 5. Mycologia 84, 950. doi:10.2307/3760304
Wang, C., Wang, J., Zeng, L., Qiao, Z., Liu, X., Liu, H., et al. (2019). Fabrication of Electrospun Polymer Nanofibers with Diverse Morphologies. Molecules 24, 834. doi:10.3390/molecules24050834
Webster, J., and Weber, R. (2007). Introduction to Fungi. Cambridge, MA: Cambridge University Press.
Whiteford, J. R., and Spanu, P. D. (2002). Hydrophobins and the Interactions between Fungi and Plants. Mol. Plant Pathol. 3, 391–400. doi:10.1046/j.1364-3703.2002.00129.x
Willsey, A. M., Hartwell, A. R., Welles, T. S., Park, D., Ronney, P. D., and Ahn, J. (2020). Investigation of Mycelium Growth Network as a thermal Transpiration Membrane for thermal Transpiration Based Pumping and Power Generation. in Proceedings of the ASME 2020 Power Conference collocated with the 2020 International Conference on Nuclear Engineering. ASME 2020 Power Conference. Virtual, Online. August 4-5, 2020. V001T03A014. ASME. doi:10.1115/POWER2020-16619
Woiciechowski, A. L., De Souza Vandenberghe, L. P., Karp, S. G., Letti, L. A. J., De Carvalho, J. C., Medeiros, A. B. P., et al. (2013). “The Pretreatment Step in Lignocellulosic Biomass Conversion: Current Systems and New Biological Systems”. Lignocellulose Convers. Enzymatic Microb. Tools Bioethanol Prod., 39–64. doi:10.1007/978-3-642-37861-4_3
Wösten, H. A. B. (2001). Hydrophobins: Multipurpose Proteins. Annu. Rev. Microbiol. 55, 625–646. doi:10.1146/annurev.micro.55.1.625
Wu, J., Qin, Z., Qu, L., Zhang, H., Deng, F., and Guo, M. (2019). Natural Hydrogel in American Lobster: A Soft Armor with High Toughness and Strength. Acta Biomater. 88, 102–110. doi:10.1016/j.actbio.2019.01.067
Xing, Y., Brewer, M., El-Gharabawy, H., Griffith, G., and Jones, P. (2018). Growing and Testing Mycelium Bricks as Building Insulation Materials. IOP Conf. Ser. Earth Environ. Sci., 121, 022032. doi:10.1088/1755-1315/121/2/022032
Zampieri, F., Wösten, H. A. B., and Scholtmeijer, K. (2010). Creating Surface Properties Using a Palette of Hydrophobins. Materials 3, 4607–4625. doi:10.3390/ma3094607
Zhou, S., Jin, K., and Buehler, M. J. (2021). Understanding Plant Biomass via Computational Modeling. Adv. Mater. 33, 2003206. doi:10.1002/adma.202003206
Keywords: mycelium, natural composite, micro-structure-mechancis relationship, infrastructure, multiscale modeling
Citation: Yang L, Park D and Qin Z (2021) Material Function of Mycelium-Based Bio-Composite: A Review. Front. Mater. 8:737377. doi: 10.3389/fmats.2021.737377
Received: 12 July 2021; Accepted: 24 August 2021;
Published: 30 September 2021.
Edited by:
Francisco Martin-Martinez, Swansea University, United KingdomReviewed by:
Serena Danti, University of Pisa, ItalyPeiman Brouki Milan, Iran University of Medical Sciences, Iran
Copyright © 2021 Yang, Park and Qin. This is an open-access article distributed under the terms of the Creative Commons Attribution License (CC BY). The use, distribution or reproduction in other forums is permitted, provided the original author(s) and the copyright owner(s) are credited and that the original publication in this journal is cited, in accordance with accepted academic practice. No use, distribution or reproduction is permitted which does not comply with these terms.
*Correspondence: Zhao Qin, enFpbjAyQHN5ci5lZHU=