- 1Institute of Tropical Forestry and Forest Products (INTROP), Universiti Putra Malaysia, Serdang, Malaysia
- 2Institute of Advanced Technology, Universiti Putra Malaysia, Serdang, Malaysia
- 3Department of Aerospace Engineering, Faculty of Engineering, Universiti Putra Malaysia, Serdang, Malaysia
The emergence of high-strength graphene marks a new milestone in the material science field. With only a small percentage inclusion into the matrix system, this organic nanoparticle could tremendously improve the strength in vast arrays of composites. At the same time, there is a growing interest in using the low-cost, lightweight, and high early strength geopolymer as the new binder for concrete. Compared to the traditional Ordinary Portland Cement (OPC), geopolymer emits 80% less CO2 during its production while exerting similar strength. Thus, the geopolymer has the potential to commercialize as new and green concrete. Geopolymer is a mixture of aluminosilicate powders and alkaline solutions. When incorporated with nano-sized graphene, the material forms a composite known as Graphene Reinforced Geopolymer Nanocomposite (GRGN). The addition of graphene enhances the strength of geopolymer, which can further improve its competitiveness. However, this depends on several factors, including the types of graphene, the surface modification of graphene, and the concentration of alkaline solutions. Generally, the presence of graphene alters the porous structure of geopolymer into a substantially filled porous structure, thus increasing compressive strength and flexural strength. On the other hand, Graphene Oxide (GO) undergoes a chemical reduction in the alkaline solution, producing epoxy functional groups. The chemical treatment results in two conditions which are weak interaction between graphene and geopolymer matrix, and better graphene dispersibility in geopolymer matrix. This review also highlights the analytical modelling aspect of GRGN. The dissolution of Si(OH)4 and Al(OH)4- from the aluminosilicate source was consistent with experimental work and analytical modeling, while the dissolution of Si–OH on the surface-modified graphene indicated otherwise. Therefore, this paper will provide an insightful review of the GRGN mechanical properties.
Introduction
The idea of geopolymer was first encountered by Joseph Davidovits in 1972 when he was initially searching for incombustible material due to the catastrophic fires in France at that time. The idea arose from the fact that a simple hydrothermal condition could govern the synthesis of plastics (polymer) in an alkaline medium (Davidovits, 2002). Davidovits then develop a three-dimensional, semi-crystalline mineral polymer called geopolymer. The first application of geopolymer is a fire-resistant coating for wooden chipboard panels produced by compression. In 1975, he made the first breakthrough of producing a geopolymeric liquid binder with the combination of metakaolin (MK) and soluble alkali silicates. Due to its fluidity, the liquid binder gathers attention from industry for its use as cement (Davidovits, 2015a). Since then, various types of new geopolymers materials have been produced, including adhesives (Bell et al., 2005; He et al., 2011; Quan et al., 2017), waste encapsulations (Frizon, and Desbats-le-Chequer, 2010; Kupwade-Patil et al., 2013a; Mohajerani et al., 2019) and resin for fiber composites (Ruzaidi et al., 2014; Hron et al., 2018).
Not limited to MK, geopolymer can be made from other kinds of abundant aluminosilicate as well. The aluminosilicate includes slag, fly ash, clays, waste glass powder, volcanic tuffs, and calcined natural fibers such as rice husks, bamboo leaves, and elephant grass (Kriven, 2012). Ultimately, these aluminosilicate sources contain silica and alumina (Léonard and Su, 2007; Tulyaganov et al., 2013). When mixed with alkaline solution (either at room temperature or elevated temperature), the silica and alumina will react, following several steps in becoming a geopolymer. These steps include alkalinization, depolymerization of silicates, gel formation of olio-sialates, polycondensation, reticulation (networking), and lastly, geopolymer solidification (Habert, 2014; Davidovits, 2018).
Presence of low silica to alumina content, Si:Al ratio, is preferred for the high rigidity of geopolymer. When Si:Al ratio is between 1:1 and 2:1, the geopolymer material possesses a 3D network. When the ratio increases to 35:1, the network becomes 2D. The silica and alumina content varies with the aluminosilicate source. Thus, to achieve the desired Si:Al ratio and the intended resultant properties, one can utilize more than one type of aluminosilicate material in the production of geopolymer. On the other hand, the concentration of an alkaline solution affects the stability of geopolymer in water. This is because alkaline solution contains positive ions, for example Na+ in NaOH solution and K+ in KOH solution. These positive ions are often labeled as K. During geopolymerization, only positive ions can attach themselves to Al− from the aluminosilicate source. When the content of positive ions exceeds alumina’s content (K:Al>1), it will cause the positive ions in the geopolymer to be exposed and migrate easily in water. Therefore, in this scenario, the geopolymer structure will be broken (Davidovits, 2018). Thus, geopolymer rigidity and stability depend on silica and alumina content from aluminosilicate sources and positive ions from alkaline solutions.
Even with low Si:Al ratio and balanced K:Al ratio, the geopolymer has one main flaw, which is brittle. Like ceramic, geopolymer is highly susceptible to cracking under loading (Chi et al., 2018). The unstable geopolymer structure is due to its highly cross-linked framework. Filler reinforcement in the geopolymer matrix can improve fracture toughness and resist crack propagation in geopolymer, thus combating the brittleness of geopolymer material (Wang et al., 2016; Dac Ho, 2020). Variety types of reinforcement have been added into the geopolymer matrix to improve the geopolymer properties. This paper discusses in detail the effects of adding two-dimensional graphene and its derivatives on enhancing the mechanical performance of geopolymer nanocomposite.
Graphene and its Derivatives
Graphene has been reinforced in polymer composite due to its attractive properties. Research on using graphene to modify the properties of the polymer (Carotenuto et al., 2012; Itapu and Jayatissa, 2018; Moharana et al., 2019), and ceramics (Porwal et al., 2013; Ikram and Farooq, 2019; Głuchowsk et al., 2020; Sun et al., 2020) have received much attention. The two-dimensional honeycomb structure of the graphene carbon network has multi functionally unique properties such as high thermal conductivity, which is in the range of 2000–5000 W/mK (Jauregui et al., 2010), large surface area which is more than 2,630 m2/g (Lambert et al., 2009; Moon et al., 2010a), high Young Modulus of about 1 TPa (Liu et al., 2013), and high electrical conductivity. Graphite is the simplest form of crystalline carbon allotropes arranged in hexagonal manners with a C-C bond distance of only 0.142 nm (Anderson et al., 2013; Tiwari et al., 2020). The planar structure of graphene sheets creates a very high contact area with the host material because the top and bottom surface of the sheet is in close contact with the matrix (Lambert et al., 2009). The graphene sheet consists of a one-atom-thick lattice with carbon atoms arranged in a hexagonal ring structure (Roberts et al., 2010). The development of different types of graphene structures is shown in Figure 1.
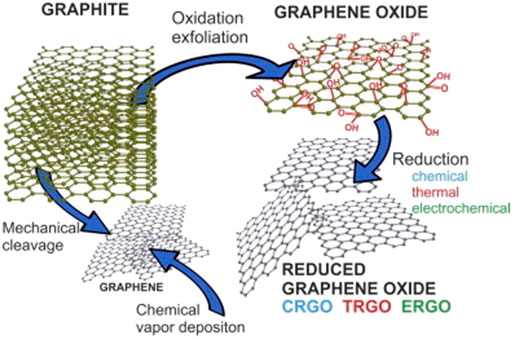
FIGURE 1. Schematic diagram on the preparation of graphene and Graphene Oxide (GO) (Filip and Tkac, 2014).
Apart from Chemical Vapor Deposition (CVD) shown in Figure 1, graphene can be synthesized through methods such as micromechanical exfoliation of graphite (Novoselov et al., 2004), epitaxial growth (Huang et al., 2008), chemical intercalation (Malik et al., 2010), and the reduction of graphene oxide (Moon et al., 2010b). Preparation of graphene from GO stands out because it is promising for mass production of graphene based materials (Mao et al., 2012). GO can be synthesized in large quantity by oxidizing inexpensive graphite powders using strong oxidants where graphite powders are exfoliated and broken into layers with increased interlayer distance. The landscape of the sp2 carbon network is modified with oxygen containing functional groups and defects (holes) (Mao et al., 2012).
These oxygen-based functional groups such as epoxy (-COC) and hydroxyl (-OH) are randomly attached across the carbon backbone and carbonyl (C=O) and carboxylic acid (-COOH) are distributed at the edges of the graphene sheets (Lambert et al., 2009; Mao et al., 2012; Smith et al., 2019; Zhang Q. et al., 2020). These oxygen-containing moieties make GO hydrophilic and readily exfoliated when immersed in water (Ku Muhammad et al., 2016). In other words, GO can quickly disperse in an alkaline solution (Kuilla et al., 2010; Pei and Cheng, 2012). Good GO dispersion is said mainly due to the strong hydrogen bonding between GO functional groups and solvent molecules (Neklyudov et al., 2017). However, it has also been reported that GO sheets are highly negatively charged when dispersed in water as a result of ionization of the carboxylic acid and phenolic hydroxyl groups that are known to exist on GO sheets. Formation of stable GO colloids should be attributed to electrostatic repulsion rather than just hydrophilicity of GO (Li et al., 2008).
Reduced graphene oxide (rGO) has similar properties to GO but with reduced oxygen content. The rGO is produced by a thermal or chemical reduction process on GO. A range of reducing agent through chemical method includes hydrazine, alcohol, sodium borohydride, hydriodic acid with acetic acid, sodium/potassium hydroxide and many others (Mao et al., 2012). The defects introduced onto graphene oxide sheet due to oxidation degrades its unique properties, so it is important to reduce a much less defective rGO. The rGO still has good dispersibility in an alkaline medium which is suitable to be processed with geopolymer (Fan et al., 2008). This can be attributed mainly due to the residual of oxygen functional group on rGO. A one-step approach to reducing and functionalizing GO under alkaline solution has been reported (Yuan et al., 2014). Functionalized GO sheets display enhanced compatibility with polymer matrix. Thus, simultaneously reduced, and functionalized GO sheets exhibit better dispersion in matrix (Li et al., 2011; Ma et al., 2012; Mao et al., 2012).
GO sheets show that they are highly negatively charged when dispersed in water as a result of ionization of the carboxylic acid and phenolic hydroxyl groups that are known to exist on GO sheets. Formation of stable GO colloids should be attributed to electrostatic repulsion rather than just hydrophilicity of GO. It is worth nothing that the defects in GO and rGO causes reduction in electrical conductivity but offers a new applications that cannot be achieved with pristine graphene. This includes surfactant free graphene colloids. Graphene oxide can dispersion can be directly converted to stable graphene colloids through hydrazine reduction under controlled condition. The use of polymeric or surfactant is not required. Complete removal of metal salts and acids which often remain in the starting graphite oxide is critical to stability. These residual electrolytes can neutralize the charges on the sheets, destabilizing the resulting dispersion. As synthesized GO was suspended in water to give brown suspension which was subjected to dialysis to completely remove residual salts and acids (Li et al., 2008).
Reduction level of rGO is precisely controlled by heating temperature, gas environment and duration. To achieve a high reduction level, combination of chemical reduction with thermal reduction is proposed. Missing atoms or holes in GO carbon network could not be fixed with chemical reduction but by thermal reduction with carbon containing gas source, it is possible to repair the carbon network in GO. Overall, the properties of this nanoparticle depends on the type and distribution of functional groups, defects and holes from missing carbon in the GO carbon lattice (Mao et al., 2012).
Graphene nanoplatelet (GNP) is another type of graphene used as an additive in the geopolymer nanocomposites. GNP structure consists of several layers of graphene and less affected by agglomeration due to its thickness which is approximately 100 nm (Dusza et al., 2012). Compared to the single layer of graphene, GNP possesses good interaction with the matrix and can transfer stress efficiently in the composite. The multi-flakes structure of GNP and its ultrahigh surface areas imparts good interfacial adhesion with matrix (Yavari et al., 2010). GNP’s presence aid in producing refine pores to prevent microcracks deflection and increased the geopolymer compactness (Wang et al., 2016). Owing to the excellent chemical bonding with the geopolymer matrix, the spatial arrangement of the GNP structure creates a tortuous path for water molecules in the geopolymer matrix (Du and Pang, 2018). It was reported that the high surface area of GNP arrests the initiation of microcracks in the geopolymer, which subsequently improves the compressive strength (Matalkah and Soroushian, 2020).
Graphene reinforced geopolymer nanocomposites
The improvement in mechanical properties brought by graphene in geopolymer composite is due to several factors such as high surface area of graphene structure, well disperse graphene in geopolymer matrix, and good bonding behavior of graphene in geopolymer. However, this is highly dependent on the type of graphene used. Dispersion of pristine graphene in geopolymer is complicated as the pristine graphene itself is hydrophobic. Graphene sheets are strongly attracted by van der Waals force and strong π-π stacking between graphene lamellae, leading to agglomeration (Fang et al., 2009; Liang et al., 2018). Moreover, the low surface activity of pristine graphene creates a weak bonding interaction with the matrix, making the dispersion challenging (Ma et al., 2013). Therefore, meticulous attention is needed to improve its dispersibility in the matrix.
Dispersion of graphene into the matrix can be carried out through physical, chemical, or thermal methods. Physical dispersion can be achieved through mechanical methods such as the use of a high-speed mechanical stirrer (Chun et al., 2017; Manigandan et al., 2017), ultrasonic dispersion (Liu et al., 2018; Zhang and Chen, 2019), and ball milling process (Kothiyal et al., 2016). High shear stress was applied onto the pristine graphene in the mechanical dispersion process to separate it into individual graphene sheets, allowing it to serve as a nanofiller instead of agglomerated micro filler. Graphene can be chemically dispersed within the matrix by altering its surface by covalent bonding or non-covalent bonding with various functional groups. Functionalization with covalent bonding forms functional oxygen-containing groups on the graphene surface, such as hydroxyl groups (-OH), carboxyl groups (-COOH), and epoxy groups (C-O-C). Meanwhile, non-covalent bonding occurs through the attachment of non-covalent functional groups by weak Van der Waals force, π-π interactions, ion interactions, and electrostatic interaction in the graphene basal plane (Naebe et al., 2014).
In the geopolymer synthesis process, the hydrophilic oxygen groups located on the basal plane of GO allowed it to be dispersed in water and mixed with the alkaline solution. The presence of a high concentration of functional groups on GO provides good interaction with geopolymer and improves matrix workability. In addition, the formation of a thick water layer around GO sheets aid in improved the rheology behavior for geopolymer workability (Zhong et al., 2017).
During geopolymer synthesis, the inclusion of GO into the alkaline solution will transform the GO to rGO by converting C=O to C-O bonds and eliminating some of the oxygen functional groups. X-ray photoelectron spectroscopy (XPS) analysis can be used to confirm the reduction process, where the C/O ratio was found to increase after GO is immersed in an alkaline solution. The reaction between GO and sodium hydroxide (NaOH) to produce rGO is as follows (Amri et al., 2021):
The reaction between GO and strong alkaline solution such as NaOH produces epoxy as shown in Figure 2. Besides that, the GO will undergo deoxygenation, which then reduced the number of hydroxyl groups (Fan et al., 2008). The presence of epoxy groups can also reduce the interaction between the functional groups and the geopolymer matrix. Poor interaction is due to the low binding energy of the epoxy groups that may lower the strength of the geopolymer nanocomposite with an excessive amount of functional groups.
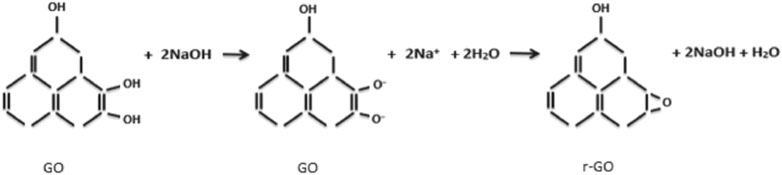
FIGURE 2. Formation of epoxy groups by reaction with NaOH in GO/geopolymer nanocomposite (Amri et al., 2021).
Figure 3A shows the thin GO sheets observed under the transmission electron microscope (TEM), which revealed the formation of single-layer GO (Yan et al., 2015). GO sheets contain electronegative characteristics that can react with cationic ions such as Na+, K+, and Ca+ in a geopolymer solution. These reactions will then repels the presence of electronegative ions such as Al (OH)4− tetrahedral to balance out the negative charge from the substitution of Si with Al (Provis and Van Deventer, 2009). The repellant action is also said to accelerate geopolymerization as the electron binding energy of Al (OH)4− tetrahedral increases (Liu et al., 2020). Two factors were affecting the repellant reaction rate, which are activation energy and mass transfer rate. GO adsorbs free ions in geopolymer slurry as a high electron active material during the reaction and accelerates the mass transfer rate. (Guo et al., 2010).
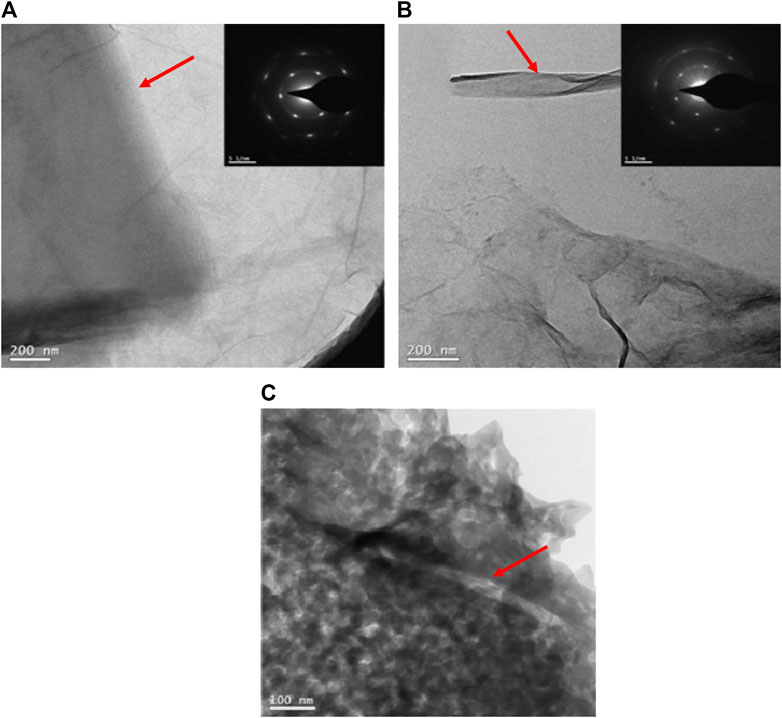
FIGURE 3. Morphology observation under TEM on (A) Graphene Oxide (GO) sheet; (B) reduced Graphene Oxide (rGO); (C) rGO in geopolymer (Yan et al., 2015).
When Al (OH)4− tetrahedral is repelled, it will reduce the Si/Al ratio in the geopolymer gel, while the Al element in the solution increased. Incomplete removal of negatively charged oxygen functional groups leads to repulsive effects between charged rGO sheets. Moreover, the van der Waals interaction between rGO sheets is weakened and leads to the electrostatic stabilization improvement of rGO, making it homogeneously dispersed in geopolymer solution (Yan et al., 2016b). Besides, the sheet edges of GO get scrolled up during the reduction process, as shown in Figure 3B (Yan et al., 2015). The intercalation of oxygen-containing groups indicates successful reduction process (Long et al., 2019b). The wrinkled and folded rGO has a higher surface area that can promote better interfacial adhesion with the geopolymer matrix (Kim et al., 2010; Liu et al., 2015). Good interfacial interaction between wrinkled rGO sheets and matrix improves mechanical strength. Besides, high concentrations of OH- ions attached to the RGO sheets can expedite the slag dissolution process (Sun and Vollpracht, 2018). The adhesion of rGO sheets onto geopolymer was observed, as shown in Figure 3C, where the arrow indicates the rGO is coated with a geopolymer matrix. Thus, it is expected that the addition of GO or rGO in the geopolymer system provides efficient load transfer from the matrix to the nano reinforcement.
Compression Analysis on the Graphene Reinforced Geopolymer NanocompositeS
Compressive analysis was done on the geopolymer to understand the ability of the materials to withstand the applied load. The compressive strength of the graphene reinforced geopolymer nanocomposite (GRGN) can be measured according to ASTM C293-10. The high elastic graphene cannot easily deform during compression of geopolymer nanocomposite due to its high rigidity structure. When a load is applied to the GRGN, the stress faces the geopolymer matrix. Then, cracks start to initiate from nano-scale and widen to micron-scale, which later the crack propagates to the graphene sheets. For the crack to move further, it must pass the high strength of graphene sheets. Therefore, graphene sheets can inhibit crack propagation by serving as a crack-bridging and crack blocking (Rehman et al., 2018). In addition, the well-distributed of high surface area of the graphene sheet in the matrix can facilitate the crack arrest effect in the nanocomposite (Zohhadi, 2014). The graphene thickness also may facilitate the stress transfer by inhibiting the crack propagation (Rehman et al., 2017). Thus, graphene sheets can interrupt the crack propagation, improve the fracture toughness, and make the composite more ductile than the unfilled geopolymer. Figure 4 shows that when a load is applied to the GRGN in compression, the graphene sheets absorb high energy due to strong interaction with the matrix. The stress is initially transferred to the graphene sheets and then uniformly to other parts in the matrix, represented by yellow and pink arrows that distributes them in vertical and horizontal direction respectively. Then, as the load is released, the nanocomposite failed in a brittle manner. The nanocomposite failed due to the sudden release as the energy has been accumulated when the graphene is pulled out from the matrix (Ranjbar et al., 2015). The uniform stress transfer occurred as the graphene was distributed evenly in the geopolymer matrix. However, defects or overlapping of the graphene sheet will lead to non-uniform stress formation (Danial et al., 2019). Geopolymer composite filled with GO is much denser and more compact due to the strengthening mechanism of GO in the geopolymer matrix.
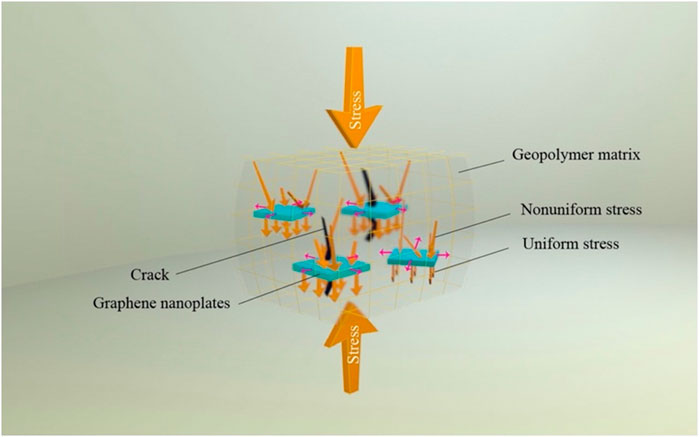
FIGURE 4. Schematic diagram on the compressive behavior of graphene/geopolymer nanocomposite (Ranjbar et al., 2015)
Geopolymer materials develop their strength properties with time. The voids within geopolymer structures will reduce with an increment in curing days (Yaghoubi et al., 2020). Reduction in voids number was contributed by the growth of the geopolymer network that consolidates and solidifies the material. This continuous cross-linking of geopolymerization occurs rapidly within the first few weeks of curing days, and the reaction slows down as it reaches months. Numerous studies reported the development of compressive strength on Day 1, Day 3, Day 7, Day 14, or Day 28 of curing for a more apparent result (Kim et al., 2014; Kupaei et al., 2014; Neupane et al., 2015).
Based on Table 1, the neat MK geopolymer (0% graphene) shows increasing compressive strength with longer curing days up to 28 days. Geopolymer filled with 1% of graphene shows enhancement of compressive strength at day 3 about 287%. The compressive strength increases due to well-distributed graphene in geopolymer and good interfacial adhesion with geopolymer matrix. Simultaneously, the geopolymer hydration products become highly compacted, reducing the internal porosity, which increases its compressive strength. A similar observation was found for the same graphene geopolymer composite on Day 7 and Day 28, indicating that graphene does not interfere with the geopolymerization cross-linking with time. Similarly, the trend is consistent geopolymer loaded with 2, 3, and 4% graphene (Zhang and Lu, 2018). However, it is reported elsewhere that agglomeration and overlapping of above 1% graphene nanoplatelet can occur in the geopolymer system (Ranjbar et al., 2015). This is because the surface of graphene is hydrophobic which indicates that graphene has the potential to agglomerate in aqueous solutions and geopolymer composites (Jishnu et al., 2020). This indifference in result may be because Zhang uses a readily graphene dispersant liquid while Ranjbar personally sonicate graphene in water for 5 min (Zhang and Lu, 20188; Ranjbar et al., 2015). Ranjbar admitted that his method may not be suitable for high loading of graphene but high water content may be added to alter the experimental design to solve the issue. However, water must be added with care as excessive water would lead to composite weakening due to high porosity (Ranjbar et al., 2015). At the same time, it must be kept in mind that water is an important medium for destruction of raw materials and transfer of effective ions (Sagoe-Crentsil and Weng, 2007). Thus, an optimum amount of water is crucial for a good geopolymer fabrication.
GO was introduced into fly ash only based geopolymer system and improvement in compressive strength was observed at Day 7, Day 14, and Day 28. Thanks to its oxygen functional groups, GO can disperse well in geopolymer slurry (Xu et al., 2018). Well dispersed graphene promises long-term stability in geopolymeric solutions (Yuan et al., 2014). This is because the solvent has provided the necessary force for the GO molecules to move around in the medium. This force is stronger than that which hold the GO together. Thus, deagglomeration and sedimentation of graphene is minimized (Lahir, 2019). Moreover, the mechanical interlocking adhesion between GO sheets and geopolymer binder contributes to the enhancement of the compressive strength. This is mainly due to the wrinkling effect on GO with respect to reduction in alkaline solution during fabrication. When wrinkled, GO provides a rough and higher surface area for adhesion with geopolymer binder (Bellum et al., 2020). In addition to this, it has been reported that reduction of GO induces hole defect onto the rGO. This is because the hole structures may allow polymer penetration through graphene sheets thus enhancing polymer-filler interaction (Lin et al., 2013).
Oxidation on parent graphene result in GO with 2% holes that form as CO and CO2 are released during aggressive oxidation and sheet exfoliation. The defect hole here is usually less than 5 nm. Reduced and annealed graphene oxide has an increased area of holes with 5% now as CO and CO2 formed during annealing. Even though all oxygen containing groups are completely removed from carbon network, the remaining functionalities and holes in rGO drastically differentiate from pristine graphene (Erickson et al., 2010).
From Table 1, Zhang uses MK while Xu uses FA in their geopolymer, which leads to the formation of different geopolymer material (Zhang and Lu, 2018; Xu et al., 2018). Frequently, MK contains low Si:Al ratio of about 1 (Constâncio Trindade et al., 2017 and Dousti et al., 2017) while FA contains higher Si:Al ratio of about 3 (Güneyisi et al., 2013 and Hariharan et al., 2015). According to Davidovits, a low Si:Al ratio of 1–3 leads to 3D geopolymer networking while higher Si:Al ratio results in 2D networking instead (Davidovits, 2015b). The problem incurs with 2D networking is that the linear structure can easily depolymerize, making the geopolymer weak (Davidovits, 2006; Davidovits, 2015c). Despite this, Table 1 shows that the compressive strength of FA geopolymer is greater than the MK geopolymer. The presence of GO causes the difference in compressive strength value. Aside from great dispersibility of GO in alkaline solution, GO content has direct effect onto concentration of Al. In mole ratio analysis, increasing the GO content will increase the relative concentration of Al. Al mainly exerts a negative charge (for example, Al(OH)4−. The oxygen group on GO also carries a negative charge (Skoda et al., 2014). When in the presence of both these groups, they repel each other, increasing Al concentration (Xu et al., 2018). On the other hand, positively charged ions like Ca2+, Na+, K+, and Mg2+ likely to attract the oxygen functional group on GO. Due to this, the cationic ions are most likely to be entrapped via the -OH functional group, thus decreasing the positively charged ions concentration in the geopolymer solution (Ramesha et al., 2011; Archanjo et al., 2014). The effect of these charges is not significant toward Si as Si mainly exists as neutral Si (OH)4 unit, and SiO (OH)3− and SiO2 (OH)22− anions. In other words, the effect of GO on the distribution of Si is relatively weak (Xu et al., 2018).
A geopolymer nanocomposite with the most negligible graphene content of about 0.005 wt% only has been produced. The result still shows an encouraging increment in compressive strength up to 46.86 MPa (Yeswanth Sai and Durga Prasad, 2020). The improvement could be due to two reasons; lower weight of GO and synergistic effect among aluminosilicate materials. Firstly, GO is reduced to rGO and holes are formed, the weight of rGO is lowered. Therefore, for the same weight, there were more rGO used compared to pristine graphene. Secondly, it is due to the synergistic effect of two different types of aluminosilicate mixed in their formulation, which are slag and fly ash. Combining more than one aluminosilicate powder is known to result in high compressive strength (Kumar and Revathi, 2016; Vogt et al., 2019). Individual aluminosilicate sources do not have well-balanced characteristics to be the only source used in geopolymer production. For example, kaolin is one commonly used aluminosilicate source due to its abundance (Akinyele et al., 2017). In addition to this, kaolin has a low Si:Al ratio that is beneficial for producing 3D network geopolymers for bricks, ceramic, and fire protection applications (Davidovits, 2015c). Joseph Davidovits reported using kaolin in their earliest invention of geopolymer (Davidovits, 2015a). Indeed, various works have been reported recently on fabrications and properties of kaolin or calcined kaolin (called metakaolin, MK) in producing geopolymer (Jaya et al., 2016a; Jaya et al., 2016b; Hájková, 2018; Merabtene et al., 2019). However, kaolin has one dominating flaw, which it has high water demand. Kaolin is likely to absorb water due to its plate-like structure, which is still retained even after calcined to become MK. Despite benefiting from high reactivity, the high surface area ferociously increases the viscosity of geopolymer slurry (Provis et al., 2010). This forces researchers to add higher water content for good geopolymer workability.
A hybrid geopolymer nanocomposite was produced by adding titanium treated reduced graphene oxide (TiO2-RGO) in the mixture of slag/fly ash geopolymer (Guo et al., 2020). The authors observed an increment of strength on the geopolymer with an increment of TiO2-RGO loading. With the presence of TiO2-RGO, the compressive strength jumped to 73.5 MPa. The unique spherical shape of titanium dioxide combined with rGO enhanced the geopolymer solution workability. Thus, it reduced the need to add extra during the processing of the geopolymer nanocomposite. Excessive water content is known to degrade the mechanical strength of geopolymer because water is not involved in geopolymerization and will be evaporated, which leaves behind empty spaces. Geopolymer material with less water content consistently exhibits high compressive strength. In addition to this, during evaporation, geopolymer tends to crack as it cannot sustain the previous shape held by water within. The failure will cause other problems like shrinkage (Asif et al., 2015; Raphaëlle et al., 2019). Aside from benefitting the lower water demand, TiO2 also serves as an anti-aggregation particle and it can inhibit the interlaminar recombination of RGO sheets (Guo et al., 2020). Morphological observation under the transmission electron microscope (TEM), displays that TiO2-RGO shows uniformly dispersed spherical morphology of TiO2 nanoparticles onto the RGO sheets (Nainani and Thakur, 2016). This evident the prevention of RGO aggregation through adhesion of TiO2 nanoparticles onto the RGO sheets. Interestingly, lamella graphene can also support zinc oxide in the geopolymer matrix, which subsequently improves the mechanical properties of the GRGN (Zhang L. W. et al., 2020).
Yeswanth Sai and Guo reported on selecting a similar aluminosilicate source which are slag and FA class F, in the fabrication of GRGN. They also reported a similar choice of alkaline solution, which are NaOH and Na2SiO3 used to fabricate the GRGN. The observation found that incorporation of 0.005 wt% GO increases the compressive strength at day 28, which is from 38.06 to 46.86 MPa. On the other hand, adding 0.02 wt% TiO2-RGO shows a similar trend on strength increment at day 3, which is from 38 to 47.6 MPa. The high early strength for TiO2-RGO geopolymer evident the advantage of using surface treated GO. Thanks to the low water demand, TiO2-RGO geopolymer produces lesser pores during curing and therefore higher early strength (Guo et al., 2020; Yeswanth Sai and Durga Prasad, 2020).
Even though all geopolymer is known to develop its strength over time, the GO geopolymer cannot surpass the compressive strength of the TiO2-RGO geopolymer. This is because it was reported that the compressive strength of slag/FA continues to develop over six months. However, the rate of strength development decreases each month. The compressive strength was enhanced by 10.4% from first month to second month of curing while from second month to the third, it was increased by 3.77%. Further reduction in percentage is observed in the following curing period. This is because slag is a highly reactive aluminosilicate source. Its presence in the slag/FA geopolymer slurry causes high reaction in the early period of curing. Thus, it achieves consolidation at a quicker rate (which in this case, is in the first month). Therefore, the strength development slows down as consolidation almost complete (Lloyd, 2009). Since GO geopolymer already achieved 46.86 MPa at day 28, its strength development is expected to slow down in the coming month and will not be able to overcome the strength of TiO2-RGO geopolymer. Aside from this, it is essential to note that other factors could affect the strength of GRGN, which includes curing temperature and stirring speed.
In Table 1, it is clear that all GRGN exhibit higher compressive strength than their counterparts with no graphene. This is a clear indication that the strength of geopolymer is improved with graphene, no matter the type of graphene or treatment on graphene. As water is evaporated from geopolymer upon reaction, the porous spaces left behind is the source of geopolymer weakening when load is exerted. Any small sized particles that is incorporated into geopolymer matrix will act as filler that fills in these pores, aside from serving other advantages mentioned in the above paragraphs (Saafi et al., 2015; Ashfaq Alvi et al., 2020).
Table 2 shows the compressive strength of MK reinforced with graphene using a combination of two types of alkaline solution: sodium silicate (Na2SiO3) and sodium hydroxide (NaOH) (Zhang and Lu, 2018). In their experiment, the amount of MK, graphene, Na2SiO3, and water content is consistent, while the percentage of NaOH solution is varied (8, 10, 12, 14, and 16%). The change in the compressive strength was due to increasing the concentration of sodium cations from NaOH. Noticeably, the increment in compressive strength with the addition of NaOH percentage shows the same pattern at Day 3, Day 7, and Day 28. This can be attributed to the ease of reaction between NaOH and MK due to the smaller alkali cations present in the NaOH compared to Na2SiO3. The alkali cation from NaOH reacts with MK in generating polymeric chains of geopolymer. Thus, the addition of a high amount of NaOH percentage directly increases the reaction of MK, which accelerates hydration reaction and causing sufficient reaction (Zhang and Lu, 2018).
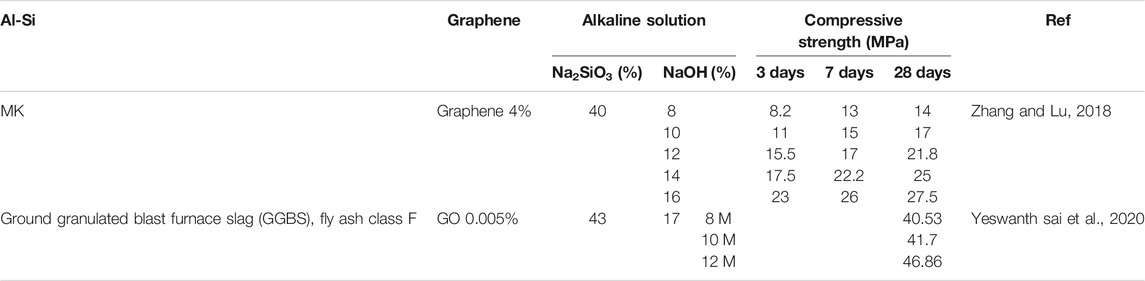
TABLE 2. Compressive strength of Graphene Reinforced Geopolymer Nanocomposites at different alkaline solution concentration.
Variation in NaOH concentration at 8, 10, and 12 M in producing GO reinforced slag and fly ash geopolymer composite was reported. The authors observed that 12 M of NaOH gives the highest compressive strength with 46.86 MPa (Yeswanth sai and Durga Prasad, 2020). This value is high for only 0.005% of GO used in the geopolymer system, unlike other researchers, which often incorporate graphene in the range of 0.02–1 wt% (Yan et al., 2017; Xu et al., 2018). The difference was due to the high reactivity of NaOH with the aluminosilicate source in the geopolymer slurry. In addition to this, GO reinforced slag and fly ash geopolymer composite displays 70% higher compressive strength than the graphene reinforced MK geopolymer composite. The increment in compressive strength reveals the benefit of designing an excellent geopolymer recipe with various constituents compared to using only one type of aluminosilicate source with an excellently low Si:Al ratio. Table 3 summarizes the compressive strength of all GRGN discussed.
Flexural analysis on the graphene reinforced geopolymer nanocomposites
A bending test on a composite is performed to identify the amount of stress under the bending load that the materials can resist without breaking. The improvement in the flexural strength can be due to the numerous toughening mechanisms of graphene in the geopolymer matrix. Figure 5 shows the toughening effect with the presence of graphene in geopolymer nanocomposite. Initially, when the load is applied onto the graphene/geopolymer nanocomposite, the crack will form at the sample center. The crack initiation at the center indicates that the highest bending stress was applied and that the crack begins to branch from the matrix to the graphene sheets. When the load is further applied, the graphene sheets in the geopolymer will form a bridge to slow down the crack formation, resulting in high stress transfer due to the greater energy absorption to break the nanocomposite sample. It was mentioned that the high stress exerted between the graphene sheets may break out the adjacent graphene sheets due to strong adhesion between graphene and the geopolymer matrix (Guo et al., 2020).
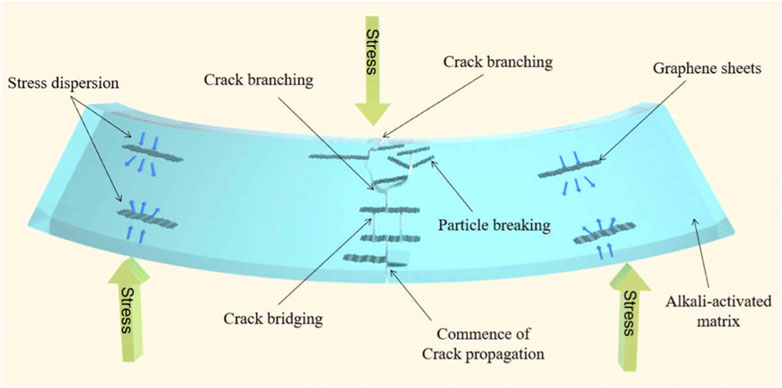
FIGURE 5. Schematic diagram of toughening mechanism due to bending load applied on the graphene/geopolymer nanocomposite (Guo et al., 2020).
Figure 6 shows microscopic images of graphene nanoplatelet (GNP) as a toughening agent to improve the strength of the geopolymer matrix. With a high elastic modulus of GNP, the stress is transfer uniformly throughout the geopolymer matrix, and the nanocomposite is not easy to deform under stress when the load is applied. As the crack starts to initiate, it will propagate parallel to the direction of the loading. Then, the crack will reach the GNP sheets, and the GNP resists the crack by forming a bridge which results in toughening mechanism (Figure 6A). As the crack formation is harsh and sudden, the GNPs are broken and pulled out from the geopolymer matrix, leading to the formation of crack branching (Figure 6B) (Ranjbar et al., 2015).
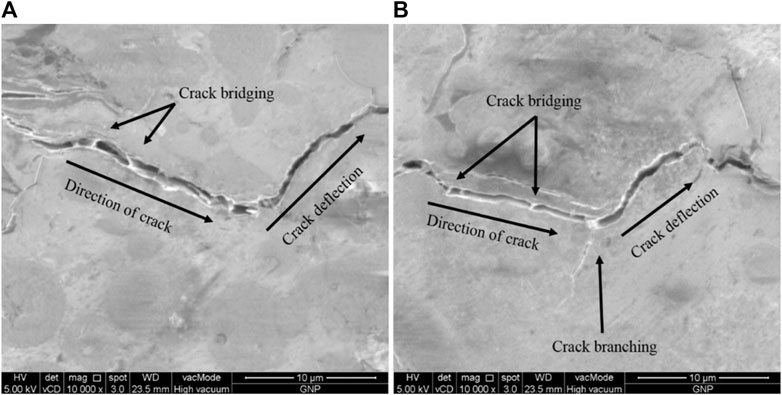
FIGURE 6. Toughening effects by graphene nanoplatelet (GNP) in geopolymer (Ranjbar et al., 2015)
The inclusion of rGO in the geopolymer matrix can affect the stiffness properties of the nanocomposite. As illustrated in Figure 7, a low amount of rGO sheets were separated by the geopolymer matrix and make the composite less brittle. By increasing the amount of rGO content to medium, some of the rGO sheets are overlapping and may restrict the movement of the geopolymer chain in the nanocomposite. However, as the rGO content is increased to a high amount, the geopolymer nanocomposite will become stiffer as the rGO constrains the geopolymer matrix’s movement. High van der Waals forces between rGO sheets form a rigid laminate structure, making the geopolymer nanocomposite fail in a brittle manner (Saafi et al., 2015).
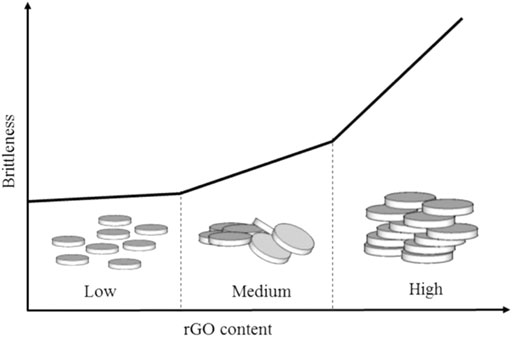
FIGURE 7. Relationship between the amount of rGO and brittle properties (Saafi et al., 2015).
Table 4 shows the graphene content ranges from 0 to 4%, while the molarity and weight of NaOH and Na2SiO3 are kept constant. Each composite shows higher flexural strength proportional to increasing curing days, except for 4% graphene. The strength of geopolymer reinforced with 4% graphene reduces by 1.5% on Day 28 when compared to Day 7. According to Zhang, the possible reason for this is the uneven dispersion of graphene in alkaline solution due to excessive graphene content. Thus, in turn, decreases the strength of GRGN. Aside from this, Zhang also studied the effect of increasing NaOH concentration on the flexural strength of GRGN. In this experiment, the amount of other constituents is kept constant, while NaOH concentration is varied from 8 to 16% with an increment of 2%. The flexural strength increases as NaOH concentration increases due to the accelerated reaction between NaOH and geopolymer solution. However, the reaction reaches a threshold point at which strength began to decrease with higher NaOH concentration. According to the author, at this point, an excessive reaction has occurred. This excessive reaction is called carbonation, and it can cause the alkali content to react with CO2 in the air (Zhang and Lu, 2018). At times, one can observe a blooming, whitish appearance on a geopolymer structure due to excessive alkalinity (Davidovits, 2016). Generally, carbonation occurred due to excess alkalinity when the K:Al ratio is very high. It can reduce the strength of geopolymer because it has a high destroying impact on geopolymer structure when exposed to moisture (Pandey, 2011).
It has been reported that the highest NaOH concentration gives the best strength of geopolymer (Yeswanth Sai and Durga Prasad, 2020). However, this is in contrast with the finding from Zhang explained earlier (Zhang and Lu, 2018). In Zhang’s reported work, there is a critical point in increasing NaOH concentration. For geopolymer without graphene, the oligomer chain and sodium silicate reacted with the soluble Al, which increase the reaction process and improve the geopolymer strength. However, the achievement in bending strength improvement is only at a certain threshold. Beyond the limit, the aggressive reaction occurred because an excessive amount of alkaline solution reacted with CO2 in the air and subsequently reduced the composite strength. With the presence of 4% graphene in the geopolymer synthesizes with highly concentrated NaOH, the strength becomes less significant. Agglomeration of graphene in a highly alkali NaOH solution and lack of dispersion are the reasons for declining in strength properties. A different trend in results may occur if a different combination of the alkaline solution is used in the production of GRGN. This is an excellent insight for geopolymer studies that involve varying concentrations of NaOH solution (Zhang and Lu, 2018).
To prevent cracking and maintain the structural integrity of the cured geopolymer, a proper curing condition by controlling the temperature and curing time is essential (Shi et al., 2017). Rapid drying during curing must be avoided (Perera et al., 2007). It is vital to retain a small amount of water in the cured geopolymer to avoid dehydration and excessive shrinkage due to long curing times at high temperatures (Van Jaarsveld et al., 2002). It was found that the flexural strength increases to about 29% for slag geopolymer composite filled with rGO steamed cured for 48 h at 60°C (Long et al., 2019a). There are three reasons for the geopolymer strength improvement. Firstly, due to the presence of–OH ions on the rGO sheets that promote better dissolution of slag. At the same time, rGO sheets repel the negatively charged Al and adhere well with the geopolymer matrix, which contributes to the improvement in flexural strength. Secondly, the processing temperature of GO sheets in NaOH solution may affect the GO structure integrity. The 60°C treated rGO shows a higher ID/IG value in Raman Spectroscopy analysis, indicating a greater degree of structural defects which is optimum for adhesion to the geopolymer matrix (Kani and Allahverdi, 2009). Thirdly, steam curing has been reported to reduce porosity within geopolymer matrix, resulting in higher mechanical strength (Fekoua et al., 2021). For the geopolymer composites cured under steam condition, the obtained Si:Al ratio and K:Al ratio is closer to the designed composition than the same geopolymer composites that are cured in room temperature, which indicates a higher formation of aluminosilicate gel from the material source (Kani and Allahverdi, 2009).
Graphene also is famously known for its unique thermal properties, as reported by many (Kolhatkar et al., 2018; Phiri et al., 2018; Liu et al., 2019). The thermal properties of graphene are related to its anisotropic bonding and effective heat transfer by lattice vibrations. In addition, the two-dimensional (2D) and sp2 covalent bonds between adjacent carbon atoms make graphene one of the strongest carbon allotrope. Thus, when geopolymer is sintered at an elevated temperature, the geopolymer structure becomes dense. Simultaneously, when GO is present during the sintering process, GO is reduced to rGO and forms a 3D network with a geopolymer matrix to create good interfacial bonding. The rGO restricts the crystal formation in geopolymer, and it may act as a barrier dislocation movement, which increases the composite’s dislocation density. A work has been published on sintering process of graphene/leucite nanocomposite to 1,000°C. It was found that the sintering process managed to improve the flexural strength by 12 times and reduce GO together with change transformation of leucite grain structure. With the reduction of GO at high temperature to rGO, the rGO scrolled, folded, attached with leucite particles, and helps in refining the leucite grain structure, making the composite denser, leading to improved mechanical properties. As the sintering process increase beyond 1,000°C, making the rGO becomes much smaller, scrolled, and folded, which may lead to the degradation of rGO and easily debonded from the matrix when the load is applied. In addition, the amorphous geopolymer is fully transformed into leucite which subsequently degrades the composite strength (Yan et al., 2016a).
The presence of GO in geopolymer has been shown to promote better flexural strength at certain stirring temperature. When the processing temperature of graphene/aluminosilicate in alkaline solution is increased from room temperature to 40 and 60°C, the flexural strength of the produced GRGN increases correspondingly. The holes that formed onto GO during reduction acts as microscopic nets that made them highly flexible (Lin et al., 2013). At the same time, The residual oxygen functional group helps in dispersing rGO in alkaline solution. However, when stirring temperature is further increased to 80°C, the flexural strength is observed to decline due to severe structural defects of RGO (Long et al., 2019a). Extreme stirring heat in alkaline solution not only causes reduction of graphene to GO, but also exhibits wrinkles on GO structure (Deng and Berry, 2016). This severe defect onto rGO weakens its strength.
In addition to this, the right technique on adding sand in geopolymer fabrication is essential to understand. The addition of sand can lead to water trapped within the geopolymer pore structure. Calcareous sand, for example, has a pore diameter of at least 1 mm, while the diameter of one water molecule is only 0.27 nm (National Nanotechnology Coordinated Infrastructure, 2012; Zhu et al., 2013). Thus, the small size of water molecules can easily penetrate the bigger sand pore size and trapped within the pores. When graphene is added into geopolymer, the graphene is likely to be dispersed in distilled water to ensure the dispersibility or exfoliation of the layered graphene structure in the geopolymer matrix. However, if the graphene solution is trapped within sand pores, the reinforcing effect becomes less efficient. Moreover, if the aluminosilicate source or alkaline solution is trapped within the sand pores, the geopolymer reaction might be affected, and there is a possibility that the materials are not hardened. Thus, the suitable time to add sand is during the final mixing stage, where aluminosilicate powder has been well stirred with an alkaline solution, forming a geopolymer slurry. Homogeneous mixing of aluminosilicate and alkaline solution creates a viscous solution and less trapped problem when sand is added into it the mixture.
Graphene can be doped with various elements such as Si, N, B, P, and many more. By doping, active sites are created on the graphene surface to alter the graphene properties and increase its catalytic activities. Doped graphene can foster better graphene dispersibility in a matrix medium. Cho investigated the properties of Si-doped graphene and found that the composite flexural strength increased from 11 to 12 MPa over pristine graphene (Cho, 2015). Meanwhile, Zhang studied the effects of pristine graphene, Si-doped graphene, and GO sheets in geopolymer composite. In water, the Si-doped graphene shows better dispersibility as water molecules easily penetrate the interlayer Si-doped graphene region and expand the graphene sheets. The hydrophilicity surface of Si-doped graphene was higher than GO as the hydrogen bonds per group are 16.9% higher than available in GO. While pristine graphene shows poor dispersion in water due to its hydrophobic behavior (Zhang L. W. et al., 2020).
Interestingly, Si-OH groups on the Si-doped graphene reacted well with the aluminate and silicate species in the geopolymer matrix by typical condensation reactions. Even though Si-doped graphene shows better dispersibility in the geopolymer matrix, the geopolymer composite strength decreased due to the transition of sp2 to sp3 bond on the Si-doped graphene layered structure deteriorates and affects its mechanical properties. However, the reinforcement effect of Si-doped graphene/geopolymer composite is still higher than unfilled geopolymer. It was mentioned that the Si-graphene is twice the weight of pristine graphene, which also makes sense, making Si-graphene geopolymer exhibiting higher density. The flexural strength of all GRGN is summarized in Table 5 (Cho, 2015).
The condition of graphene before being incorporated into geopolymer matrix is equally important in effort to produced enhanced flexural strength of GRGN. During annealing process, microgasbags are constructed in Graphene Nanosheets (GNS). These microgasbags are closely packed and usually in dimension of several micrometers. It is said that during compression of paper sheet filled with GNS, microgasbags disappear and microfolds are formed. These microfolds are the factor that determine superflexibility of the thin sheet paper (Ding et al., 2017). This hints the importance of graphene condition before incorporation in geopolymer. There has also been a reported work on improving the flexibility of GNS film with aramid nanofiber framework (Xie et al., 2021). In addition to this, conventional method of exfoliating GO through vigorous sonication can destroy the GO structure, resulting in smaller sized GO nanosheets. To minimize this destruction, a homogenizer can be used to apply shear force in the solution. When compared, the average lateral size (mm2) of sonicated GO was found to be 100 times smaller than homogenized GO (Han et al., 2018).
Analytical Modeling Aspects of Graphene Reinforced Geopolymer NanocompositeS
Material development has started from “materials by design” in the early 21st century, which involves in-depth knowledge of microscopic origins and later into processing that leads to new advanced materials. “Materials by design” or experimental work of geopolymers have been reported countless times (Zhang and Lu, 2018; Ren and Zhang, 2019; Yao et al., 2019; Cui et al., 2020; Yeddula and Karthiyaini, 2020). The optimum approach available to scientific research is to combine theory, experiment, and simulation (Landau, 2005). Generally, computer simulation can be used for prediction, qualitative or systemic predictions, giving us insight into materials system and behavior. This predictive capability is the catalyst to technological discovery and innovation in materials science and engineering (Murr, 2016). Several simulation techniques exist to understand the polymerization and gelation process in geopolymer materials. To date, the primary methods used to study geopolymer materials are density functional theory (DFT), molecular mechanics (MM), molecular dynamics (MD), and Monte Carlo (MC) simulations (Kupwade-Patil et al., 2013b).
Understanding the interfacial interaction mechanisms between Si-doped graphene and aluminosilicate source during geopolymerization is part of the comprehensive MD study done by Zhang (Zhang L. W. et al., 2020). The authors observe the dissolution of Si (OH)4 and Al (OH)4− monomers from aluminosilicate source and ≡Si–OH groups from Si-doped graphene during geopolymerization. The Al (OH)4− monomers are fully consumed by condensation reaction after 30 ps as observed from the MD simulation. While for Si (OH)4 monomers, there is almost no residual after 300 ps from simulation observation. This agrees with NMR analysis that proves almost entirely reacted Si (OH)4 monomers (Duxson et al., 2005). However, the dissolution of ≡Si–OH groups of Si-doped graphene does not correlate between the simulation and experimental analysis. According to Zhang, the simulation analysis displays only 80% of ≡Si–OH groups chemically bonded with geopolymer structure (Zhang L. W. et al., 2020). In other words, this means that only 80% of ≡Si–OH groups are polymerized. The reason to this is due to sequential attachment. Once active monomers are attached onto the 3D skeleton of geopolymer through condensation, they are unable to surface for reaction with ≡Si–OH groups. However, in reality, hydrolysis and polymerization processes occur simultaneously; thus ≡, Si–OH groups would attach to both the 3D skeleton of the geopolymer and the surface of Si-doped graphene. Besides, the ≡Si–OH monomers could dissolve in an alkaline solution in advance. The finding indicates that experimentally, ≡Si–OH groups can be fully polymerized, unlike simulation findings (Zuhua et al., 2009).
Various aluminosilicate sources were used to fabricate geopolymer composite, including different types of alkaline solution and varying concentrations of reinforcing fillers. A study investigated the physicochemical properties of rGO in geopolymer composite. The authors figure out that the higher strength in GO was due to the strong C-O bond with the electrostatic neutral carbon network in graphene. However, from their observation, inclusion of 0.5 wt% GO decreased the geopolymer compressive strength. Through inspection using fourier transform infrared (FTIR) analysis, a distinct peak occurs for 0.5 wt% GO geopolymer at 1,150 cm−1, which corresponds to an excess epoxy group produced due to GO reduction in alkaline solution. The presence of epoxy groups on GO weakens the interaction between the functional group and geopolymer matrix due to the lower binding energy of the epoxy-functional group. Nevertheless, when epoxy functional groups form onto the graphene surface, a negative charge is entailed. DFT is a theoretical modeling that can be used to calculated the electronic structure of geopolymer (Kurth et al., 2005). Through DFT, rGO is configured by inserting oxygen atoms into the carbon skeleton of graphene, and epoxy groups are added to the C-C bridges. The epoxy-type atom in GO entails a negative charge of ∼0.5 e, confirming the weakening of geopolymer composite with 0.5 wt% GO (Amri et al., 2021).
The difference in Si:Al ratio will affect the geopolymer strength properties. Low Si:Al ratio in geopolymer would lead to an improvement in mechanical properties. However, reported work on kaolinite-based geopolymers reveals a different outcome when comparing experimental and simulation analysis. DFT was used to model the geometry of geopolymer and discover that the total energy of geopolymer decreases as the Si:Al ratio increases. This indicates that at Si:Al of 1:1 ratio, the geopolymer has the most stable molecular orientation, which is translated as the highest rigidity. However, during experimental work at Si:Al ratio of 1, the geopolymer displays the lowest compressive strength. Upon an inspection of the microscopic images, it is revealed that there is the presence of a highly crystalline phase of zeolitic nuclei in geopolymer with Si:Al ratio of 1. The nuclei are not dispersed in the geopolymer matrix and lead to macropores formation, weakening the geopolymer structure when a compressive load is applied (Wan et al., 2017). Aside from this, there are also other reported work on low Si:Al ratio geopolymer but with weaker strength compared to higher Si:Al ratio. This includes slag based geopolymer (Mustofa and Pintowantoro, 2017) and fly ash based geopolymer (Asif et al., 2014). Both reported to observe homogenous binder at Si:Al ratio of two while lower ratio reveals insoluble aluminosilicate particles. It was mentioned in the work that silica content is increased by adding more sodium silicate. Therefore, in this case, the higher the Si:Al ratio, the higher the workability which leads to better particles dissolution (Asif et al., 2014). Despite the analytical modeling proving otherwise, experimental work represents real life situation. It is undeniable that simulations is useful in producing new knowledge just like experiments do, but the information and knowledge needed in running a good simulation is way more than running a good experiment (Guala, 2002).
To date, there are not many simulations works done on geopolymer and its reaction. A great insight has been made through the work done despite the limited modeling on graphene geopolymer composites. It is anticipated that a polymerization modeling would be made soon on the distribution of positive ions of alkaline solution on the 3D network of geopolymer. This is deemed necessary as the attachment of positive ions on alumina would stabilize the geopolymer structure in water. Any excess of positive ions may react with the water molecules form and disrupts the formation of a 3D network of geopolymer. In return, this may overall prevent geopolymerization from occurring. Based on the gathered information, Table 6 is produced to summarize the pros and cons of the analytical modeling approach and Table 7 on the pros and cons of the experimental approach.
Recommendations
Graphene is a unique and multi-functional nanoparticle for geopolymer composite. However, as a hydrophobic nanofiller with platelet like structure, graphene has high tendency to overlap and agglomerate in geopolymer matrix. Therefore, several types of treatments can be done to overcome this issue. Firstly, graphene can be doped with spherical shaped particles to improve workability of geopolymer slurry and to prevent interlaminar recombination of graphene sheets. Graphene can also be functionalized with hydrophilic elements to improve dispersibility in alkaline solution. Without treatment, the use of graphene dispersant liquid is recommended to prevent agglomerated graphene that could be the weakening point of geopolymer composite.
Since pristine graphene is often highly priced, its derivative which is GO is less expensive, making it a great alternative. During oxidation of graphene to produce GO, hole defects (<5 nm) are induced onto its surface. These holes are great for interlocking mechanism between GO and geopolymer matrix for enhanced mechanical properties. It is important to note that oftentimes geopolymer gel are reported to be in the range of micrometers (Temuujin et al., 2009; Kupaei et al., 2014; Mohammed et al., 2019). No work has been reported on impregnation of geopolymer nanogel into GO. The authors feel that an elucidation on this mechanism would give a new insight on the synergistic interaction between GO and geopolymer matrix in nano scale.
With presence of oxygen functional groups on GO, its dispersibility is better than pristine graphene. However, there is still a limit as to how much nanofiller can be reinforced into a matrix system. A high content of GO would lead to uneven dispersion in alkaline solution. To overcome this, a more reactive alkaline solution such as NaOH can be used to promote high early strength and high reaction of aluminosilicate source. This is because the small sodium cation eases the reaction with aluminosilicate source which increases geopolymerization. Despite providing high reaction, cautious action must be taken as high concentration of NaOH would lead to geopolymer carbonation which lead to harsh structure rupture under water. Besides that, during fabrication of geopolymer, GO will be reduced to rGO when in contact with alkaline solution. This causes enlargement of the hole, which will be severed when not controlled, thus resulting in detrimental effect on the graphene unique properties.
Oftentimes geopolymers are cured in high temperature to evaporate the high-water content added for workability. This can incur two problems: further reduction of GO and cracking of geopolymer. Aside from holes enlargement during GO reduction, the amount of oxygen functional group on GO will also be lowered. This would reduce the hydrophilicity of GO that is needed for good dispersibility. Rapid drying under high temperature would cause geopolymer to crack. To avoid this, steam curing is recommended to control moisture evaporation.
Summary and Future Work
Since 2004, graphene research has been extensively conducted, accelerating its academic publication to almost 120,000 papers as of March 2021 (Publons, 2021). With such immense interest in graphene from the academic field, the industry must keep up with current graphene technology processing (Randviir et al., 2014). The advancement in graphene research and technology will increase demands in graphene production which in the future, the cost of graphene production at maximum production can be minimized. Table 8 shows the cost for several types of graphenes.
The most popular method in making graphene is through chemical vapor deposition (CVD), whereby the process involves a mixture of gases that reacts with a surface in producing a layer of graphene. This method produces high-quality graphene but detaching the graphene from its substrate often leads to damaged product (Pistilli, 2020). A cheaper way to produce graphene derivatives is from solution-based processes (Laurén, 2018). GO is created by attaching an oxygen-based functional group to increase the dispersion ability in the water. In addition, GO can be reduced to rGO in many ways, including thermal, chemical, and electrochemical techniques. In 2019, a group of researchers from RMIT University Australia discovered a new approach to produce graphene by using a Eucalyptus bark extract. In this way, more economical graphene sheets can be synthesized in which the price reduce almost 200 times cheaper than the conventional way (Taylor, 2019).
Graphene offers enhanced electrical properties for civil engineering applications, including bridges, buildings, and roads (Mohamed et al., 2014). However, the reduction of GO to rGO during geopolymerization is undesirable when an excess epoxy functional group is present on the rGO sheet due to its lower binding energy than hydroxyl groups. Interestingly, epoxy groups’ negative charges have positive effects on the electrical properties in geopolymer composite, producing the material called Intrinsic self-sensing concrete (ISSC). By measuring the electrical resistance of ISSC, the stress, strain, crack, and damage of concrete can be monitored without embedding or attaching remote sensors (Han et al., 2015; Han et al., 2018). When implemented, ISSC made of graphene geopolymer composite can be very cost-effective in the long run.
While various works have been reported using geopolymer as green construction material, geopolymer can also be used for outer space application, where geopolymer is fabricated on the Moon using lunar regolith. Since geopolymer can be cured in average daytime temperature (127°C) and lunar regolith contains aluminosilicate materials, attempts have been made for in-situ materials utilization. It has been observed that vacuum conditions affect the geopolymerization process. Under vacuum treatment, the geopolymer crumbles into smaller pieces, which is suspected to fill in the pores in the structure (Davis et al., 2017). It has been discussed that the addition of graphene into geopolymer results in substantially filled pores, which would be useful in this case. On the other hand, graphene has been discussed for its potential to be used as a viable material in interstellar solar sail (Santoli, 2010; Matloff, 2012). When tested, graphene is lighter than the conventional polyester film and still successfully accelerates when shone with a 1 W laser. Perhaps, the use of graphene geopolymer lunar regolith-based composite could catalyst the production of geopolymer on lunar.
The mechanical strength of graphene/geopolymer nanocomposite was discussed based on various factors such as types of graphene, effects of graphene in alkaline solution, reaction between graphene and aluminosilicate particles, effects of graphene concentration on the workability of geopolymer, and geopolymer nanocomposite sintering temperature. Most of the studies observed that the strength of geopolymer filled with treated graphene surpasses pristine graphene in both compressive and flexural strength due to the higher dispersion and better reinforcement effects in the geopolymer matrix. Analytical modeling on graphene geopolymer provides an insight into the interfacial reaction between graphene and geopolymer and its geopolymerization process that cannot be achieved experimentally. Nevertheless, current analytical modeling does not represent the simultaneous process in geopolymerization, which could not give an accurate and complete illustration. Therefore, it is expected to see a more commercialized application of graphene geopolymer composites with better detailed and thorough modeling.
Author Contributions
All authors listed have made a substantial, direct, and intellectual contribution to the work and approved it for publication.
Funding
The research work was funded by the Ministry of Higher Education (MOHE), Malaysia, under Fundamental Research Grant Scheme (FRGS) with grant number 5540317.
Conflict of Interest
The authors declare that the research was conducted in the absence of any commercial or financial relationships that could be construed as a potential conflict of interest.
Acknowledgments
The authors thank the Universiti Putra Malaysia (UPM),especially the Department of Aerospace Engineering, Institute of Advanced Technology (ITMA), and Institute of Tropical Forestry and Forest Products (INTROP) for the infrastructures lend, allowing this project to be completed.
References
Akinyele, J. O., Odunfa, S. O., Famoye, A. A., and Kuye, S. I. (2017). Structural Behaviour of Metakaolin Infused concrete Structure. Nig. J. Tech. 36 (2), 331–338. doi:10.4314/njt.v36i2.2
Amri, A., Najib, A. A., Olivia, M., Altarawneh, M., Aman Rahman, M. M., Saputro, S., et al. (2021). Physicochemical Properties of Geopolymer Composites with DFT Calculations of In-Situ Reduction of Graphene Oxide. Ceram. Int. 47 (10), 13440–13445. doi:10.1016/j.ceramint.2021.01.202
Anderson, M., Lloyd Spetz, A., and Pearce, R. (2013). “Chapter 4-Recent Trends in Silicon Carbine (SiC) and Graphene-Based Gas Sensors,” in Semiconductor Gas Sensors. Editors R. Jaaniso, and O. K. Tan (Cambridge: Woodhead Publishing Series).
Archanjo, B. S., Araujo, J. R., Silva, A. M., Capaz, R. B., Falcão, N. P. S., Jorio, A., et al. (2014). Chemical Analysis and Molecular Models for Calcium-Oxygen-Carbon Interactions in Black Carbon Found in fertile Amazonian Anthrosoils. Environ. Sci. Technol. 48, 7445–7452. doi:10.1021/es501046b
Ashfaq Alvi, M. A., Khalifeh, M., and Agonafir, M. B. (2020). Effect of Nanoparticles on Properties of Geopolymer Designed for Well Cementing Applications. J. Pet. Sci. Eng. 191, 1–16. doi:10.1016/j.petrol.2020.107128
Asif, A., Man, Z., Mohd Azizli, K. A., Nuruddin, M. F., and Ismail, L. (2014). The Effect of Si/Al Ratio and Sodium Silicate on the Mechanical Properties of Fly Ash Based Geopolymer for Coating. Msf 803, 355–361. doi:10.4028/www.scientific.net/msf.803.355
Asif, A., Man, Z., Mod Azizli, K. A., and Mohammed Hamidi, R. (2015). Effect of Alkali and Water Content on Setting Time and Strength of Fly Ash Based Geopolymer. Appl. Mech. Mater. 699, 93–98. doi:10.4028/www.scientific.net/amm.699.93
Bell, J. L., Gordon, M., and Kriven, W. M. (2005). Use of Geopolymeric Cements as a Refractory Adhesive for Metal and Ceramic Joins. Ceram. Eng. Sci. Proc. 26 (3), 407–413. doi:10.1002/9780470291238.ch46
Bellum, R. R., Muniraj, K., Indukuri, C. S. R., and Madduru, S. R. C. (2020). Investigation on Performance Enhancement of Fly Ash-GGBFS Based Graphene Geopolymer Concrete. J. Build. Eng. 32, 101659. doi:10.1016/j.jobe.2020.101659
Carotenuto, G., Romeo, V., Cannavaro, I., Roncato, D., Martorana, B., and Gosso, M. (2012). Graphene-polymer Conference Series: Materials Science and Engineering, 1-7. Bedforshire, UK: IOP Publishing Ltd.
Chi, H. L., Louda, P., Periyasamy, A. P., Bakalova, T., and Kovacic, V. (2018). Flexural Behaviour of Carbon Textile-Reinforced Geopolymer Composite Thin Plate. Fibers 6 (87), 1–14. doi:10.3390/fib6040087
Cho, S. (2015). Gepolymer Composites and Their Applications in Stress Wave Mitigation. Urbana-Champaign: University of Illinois. PhD Thesis.
Chun, W. W., Leng, T. P., Osman, A. F., and Keat, Y. C. (2017). The Properties of Epoxy/Graphene Conductive Materials Using High Speed Mechanical Stirrer and Bath Sonicator. Mater. Sci. Forum 888, 222–227. doi:10.4028/www.scientific.net/msf.888.222
Constâncio Trindade, A. C., Ahmed Alcamand, H., Ribeiro Borges, P. H., and Andrade Silva, F. d. (2017). “On the Durability Behaviour of Natural Fibre Reinforced Geopolymers,” in Conference: 41st International Conference and Expo on Advanced Ceramics and Composites, Daytona Beach, FL, January 22–27, 2017 (USA: ICACC).
Cui, Y., Zhang, P., and Bao, J. (2020). Bond Stress between Steel-Reinforced Bars and Fly Ash-Based Geopolymer Concrete. Adv. Mater. Sci. Eng. 2020 (7), 1–11. doi:10.1155/2020/9812526
Dac Ho, V. (2020). Development of Next-Generation Construction Materials with Graphene AdditivesPhD Thesis. Australia: The University of Adelaide.
Danial, N. S., Che Halin, D. S., Ramli, M. M., Abdullah, M. M. A., Salleh, M. M. A., Mat Isa, S. S., et al. (2019). Graphene Geopolymer Hybrid: A Review on Mechanical Properties and Piezoelectric Effect. IOP Conf. Ser. Mater. Sci. Eng. 572, 1–10. doi:10.1088/1757-899x/572/1/012038
Davidovits, J. (2002). “30 Years in Successes and Failures in Geopolymer Applications. Market Trends and Potential Breakthroughs,” in Geopolymer 2002 Conference 2002, 1–16. Melbourne, Australia.
Davidovits, J. (2006). Difference between Geopolymer and Amorphous Zeolite. Retrieved from: https://www.geopolymer.org/faq/difference-between-geopolymer-and-amorphous-zeolite/ (Accessed October 28, 2020).
Davidovits, J. (2015a). “Chapter 1-Introduction,” in Geopolymer-Chemistry and Applications. Editor J. Davidovits 4th edition (France: Geopolymer Institute), 1–16.
Davidovits, J. (2015b). “Chapter 18-Quality Control,” in Geopolymer Chemistry and Applications. Editor J. Davidovits 4th edition (France: Geopolymer Institute).
Davidovits, J. (2015c). “Part IV: Applications,” in Geopolymer Chemistry and Applications. Editor J. Davidovits 4th edition (France: Geopolymer Institute).
Davidovits, J. (2016). Webinar Spring 2016 Special Focus on Geopolymer Cement. France: Geopolymer Insitute.
Davidovits, J. (2018). Why Alkali-Activated Materials (AAM) Are Not Geopolymers. Saint-Quentin: Geopolymer Institute. Technical Paper #25. 1–10.
Davis, G., Montes, C., and Eklund, S. (2017). Preparation of Lunar Regolith Based Geopolymer Cement under Heat and Vacuum. Adv. Space Res. 59 (7), 1–34. doi:10.1016/j.asr.2017.01.024
Deng, S., and Berry, V. (2016). Wrinkled, Rippled and Crumpled Graphene: an Overview of Formation Mechanism, Electronic Properties, and Applications. Mater. Today 19 (4), 197–212. doi:10.1016/j.mattod.2015.10.002
Ding, J., Zhao, H., Wang, Q., Dou, H., Chen, H., and Yu, H. (2017). An Ultrahigh thermal Conductive Graphene Flexible Paper. Nanoscale 9, 16871–16878. doi:10.1039/c7nr06667h
Dousti, A., Beaudoin, J. J., and Shekarchi, M. (2017). Chloride Binding in Hydrated MK, SF and Natural Zeolite-Lime Mixtures. Constr. Build. Mater. 154, 1035–1047. doi:10.1016/j.conbuildmat.2017.08.034
Du, H., and Pang, S. D. (2018). Dispersion and Stability of Graphene Nanoplatelet in Water and its Influence on Cement Composites. Constr. Build. Mater. 167, 403–413. doi:10.1016/j.conbuildmat.2018.02.046
Dusza, J., Morgiel, J., Duszová, A., Kvetková, L., Nosko, M., Kun, P., et al. (2012). Microstructure and Fracture Toughness of Si3N4+graphene Platelet Composites. J. Eur. Ceram. Soc. 32 (12), 3389–3397. doi:10.1016/j.jeurceramsoc.2012.04.022
Duxson, P., Provis, J. L., Lukey, G. C., Separovic, F., and van Deventer, J. S. J. (2005). 29Si NMR Study of Structural Ordering in Aluminosilicate Geopolymer Gels. Langmuir 21 (7), 3028–3036. doi:10.1021/la047336x
Erickson, K., Erni, R., Lee, Z., Alem, N., Gannett, W., and Zettl, A. (2010). Determination of the Local Chemical Structure of Graphene Oxide and Reduced Graphene Oxide. Adv. Mater. 22 (40), 4467–4472. doi:10.1002/adma.201000732
Fan, X., Peng, W., Li, Y., Li, X., Wang, S., Zhang, G., et al. (2008). Deoxygenation of Exfoliated Graphite Oxide under Alkaline Conditions: a green Route to Graphene Preparation. Adv. Mater. 20 (23), 4490–4493. doi:10.1002/adma.200801306
Fang, M., Wang, K., Lu, H., Yang, Y., and Nutt, S. (2009). Covalent Polymer Functionalization of Graphene Nanosheets and Mechanical Properties of Composites. J. Mater. Chem. 19, 7098–7105. doi:10.1039/b908220d
Fekoua, J. N. N., Kaze, C. R., Duna, L. L., Ghazouni, A., Ndassa, I. M., Kamseu, E., et al. (2021). Effect of Curing Cycles on Developing Strength and Microstructure of Goethite-Rich Aluminosilicate (Corroded Laterite) Based Geopolymer Composites. Mater. Chem. Phys. 270, 1–17. doi:10.1016/j.matchemphys.2021.124864
Filip, J., and Tkac, J. (2014). Is Graphene Worth Using in Biofuel Cells? Electrochim. Acta 136, 340–354. doi:10.1016/j.electacta.2014.05.119
Frizon, F., and Desbats-le-Chequer, C. (2010). Geopolymers as Waste Encapsulation Materials: Impact of Anions on the Materials Properties. Ast 69, 174–179. doi:10.4028/www.scientific.net/ast.69.174
Głuchowsk, P., Tomala, R., Jeżowski, A., Szeqwczyk, D., Macalik, B., Smolina, I., et al. (2020). Preparation and Physical Characteristics of Graphene Ceramics. Sci. Rep. 10, 11121. doi:10.1038/s41598-020-67977-5
Güneyisi, E., Gesoğlu, M., and İpek, S. (2013). Effect of Steel Fiber Addition and Aspect Ratio on Bond Strength of Cold-Bonded Fly Ash Lightweight Aggregate Concretes. Constr. Build. Mater. 47, 358–365. doi:10.1016/j.conbuildmat.2013.05.059
Guala, F. (2002). “Models, Simulations and Experiments,” in Model-based Reasoning. Editors L. Magnani, and N. J. Nersessian (Boston: Springer).
Guo, S., Qiao, X., Zhao, T., and Wang, Y. -S. (2020). Preparation of Highly Dispersed Graphene and its Effect on the Mechanical Properties and Microstructures of Geopolymer. J. Mater. Civil Eng. 32 (11), 1–10. doi:10.1061/(asce)mt.1943-5533.0003424
Guo, X., Shi, H., and Dick, W. A. (2010). Compressive Strength and Microstructural Characteristics of Class C Fly Ash Geopolymer. Cem. Concr. Compos. 32 (2), 142–147. doi:10.1016/j.cemconcomp.2009.11.003
Hájková, P. (2018). Kaolinite Claystone-Based Geopolymer Materials: Effect of Chemical Composition and Curing Conditions. Minerals 8 (10), 444. doi:10.3390/min8100444
Habert, G. (2014). “Chapter 10-Assessing the Environmental Impact of Conventional and 'Green' Cement Production,” in Eco-efficient Construction and Building Materials. Editors F. Pacheco-Torgal, L. F. Cabeza, J. Labrincha, and A. de Magalhães (United Kingdom: Woodhead Publishing).
Han, B., Ding, S., and Yu, X. (2015). Intrinsic Self-Sensing concrete and Structures: A Review. Measurement 59, 110–128. doi:10.1016/j.measurement.2014.09.048
Han, J. T., Jeong, S. Y., Jeong, H. J., and Lee, G.-W. (2018). Chemically Exfoliated Graphene Nanosheets for Flexible Electrode Applications. London, United Kingdom: Intech Open. doi:10.5772/intechopen.77284
Hariharan, R., Santhi, A. S., and Ganesh, N. (2015). Maximum Agreement Subtree (Of 2 Binary Trees). Int. J. Civil Eng. 13 (3), 1–5. doi:10.1007/978-3-642-27848-8_220-2
He, J., Zhang, G., Hou, S., and Cai, C. S. (2011). Geopolymer-based Smart Adhesives for Infrastructure Health Monitoring: Concept and Feasibility. J. Mater. Civ. Eng. 23 (2), 100–109. doi:10.1061/(asce)mt.1943-5533.0000140
Hron, R., Martaus, F., and Kadlec, M. (2018). “Compressive Properties of Geopolymer Matrix Composites,” in 2nd international conference on mechanical, material and aerospace engineering, Wuhan, China, May 10–13, 2018, 179, 1–8. doi:10.1051/matecconf/201817902003
Huang, H., Chen, W., Chen, S., and Wee, A. T. S. (2008). Bottom-up Growth of Epitaxial Graphene on 6H-SiC(0001). ACS Nano 2 (12), 2513–2518. doi:10.1021/nn800711v
Ikram, M., and Farooq, M. U. (2019). Ceramics (Si- and Al-Based Oxides)-Graphene Hybrids and Advanced Applications. London, United Kingdom: IntechOpen, 1–22.
Itapu, B., and Jayatissa, A. (2018). A Review in Graphene/Polymer Composites. Csij 23 (3), 1–16. doi:10.9734/csji/2018/41031
Jauregui, L. A., Yue, Y., Sidorov, A. N., Hu, J. N., Yu, Q., Lopez, J., et al. (2010). Thermal Transport in Graphene Nanostructures: Experiments and Simulations. Ecs Trans. 28 (5), 73. doi:10.1149/1.3367938
Jaya, N. A., Al Bakri Abdullah, M. M., Ruzaidi Ghazali, C. M., Hussain, M., Hussin, K., and Ahmad, R. (2016a). Kaolin Geopolymer as Precursor to Ceramic Formation. MATEC Web conf. 78, 01061. doi:10.1051/matecconf/20167801061
Jaya, N. A., Al Bakri Abdullah, M. M., Ghazali, C. M. R., Binhussain, M., Hussin, K., and Ahmad, R. (2016b). Characterization and Microstructure of Kaolin-Based Ceramic Using Geopolymerization. Kem 700, 3–11. doi:10.4028/www.scientific.net/kem.700.3
Jishnu, A., S Jayan, J., Saritha, A., A S, S., and Venu, G. (2020). Superhydrophobic Graphene-Based Materials with Self-Cleaning and Anticorrosion Performance: An Appraisal of Neoteric Advancement and Future Perspectives. Colloids Surf. A. Physicochem Eng. Asp 606, 125395–125414. doi:10.1016/j.colsurfa.2020.125395
Kani, N. E., and Allahverdi, A. (2009). Effects of Curing Time and Temperature on Strength Development of Inorganic Polymeric Binder Based on Natural Pozzolan. J. Mater. Sci. 44 (12), 3088–3097. doi:10.1007/s10853-009-3411-1
Kim, H., Abdala, A. A., and Macosko, C. W. (2010). Graphene/Polymer Nanocomposites. Macromolecules 43 (16), 6515–6530. doi:10.1021/ma100572e
Kim, Y. Y., Lee, B.-J., Saraswathy, V., and Kwon, S.-J. (2014). Strength and Durability Performance of Alkali-Activated rice Husk Ash Geopolymer Mortar. Scientific World J, 2014, 1–10. doi:10.1155/2014/209584
Kolhatkar, G., Boucherif, A., Boucherif, A. R., Dupuy, A., Fréchette, L. G., Arès, R., et al. (2018). Nanotechnology 29 (14), 1–7. doi:10.1088/1361-6528/aaac40
Kothiyal, N. C., Sharma, S., Mahajan, S., and Sethi, S. (2016). Characterization of Reactive Graphene Oxide Synthesized from ball - Milled Graphite: its Enhanced Reinforcing Effects on Cement Nanocomposites. J. Adhes. Sci. Technol. 30 (9), 915–933. doi:10.1080/01694243.2015.1129214
Kriven, W. M. (2012). The Geopolymer Route to High Tech Ceramics. Geopolymer Institute Technical Paper. 2012. France: Geopolymer Institute, 1–72.
Ku Muhammad, K. S. S., Mohamed, F., Radiman, S., Hamzah, A., Sarmani, S., Siong, K. K., et al. (2016). Synthesis and Characterization of Exfoliated Graphene Oxide. AIP Conf. Proc. 174 (1), 1–6. doi:10.1063/1.4966799
Kuilla, T., Bhadra, S., Yao, D., Kim, N. H., Bose, S., and Lee, J. H. (2010). Recent Advances in Graphene Based Polymer Composites. Prog. Polym. Sci. 35 (11), 1350–1375. doi:10.1016/j.progpolymsci.2010.07.005
Kumar, M. L., and Revathi, V. (2016). Metakaolin Bottom Ash Blend Geopolymer Mortar-A Feasibility Study. Constr. Build. Mater. 114, 1–5. doi:10.1016/j.conbuildmat.2016.03.149
Kupaei, R. H., Alengaram, U. J., and Jumaat, M. Z. (2014). The Effect of Different Parameters on the Development of Compressive Strength of Oil Palm Shell Geopolymer Concrete. Sci. World J. 2014, 1–16. doi:10.1155/2014/898536
Kupwade-Patil, K., Allouche, E. N., Islam, M., and Gunasekaran, A. (2013a). Encapsulation of Solid Waste Incinerator Ash in Geopolymer Concretes and its Applications. Aci Mater. J., 1–11.
Kupwade-Patil, K., Soto, F., Kunjumon, A., Allouche, E. N., and Mainardi, D. S. (2013b). Multi-scale Modeling and Experimental Investigations of Geopolymeric Gels at Elevated Temperatures. Comput. Struct. 122, 164–177. doi:10.1016/j.compstruc.2013.01.005
Kurth, S., Marques, M. A. L., and Gross, E. K. U. (2005). “Density-Functional Theory,” in Encyclopedia of Condensed Matter Physics. Editors F. Bassani, G. L. Liedl, and W. Peter (Cambridge: Academic Press).
Lahir, Y. (2019). “Chapter 7-Graphene and Graphene-Based Nanomaterials Are Suitable Vehicles for Drug Delivery,” in Applications of Targeted Nano Drugs and Delivery Systems: Nanoscience and Nanotechnology in Drug Delivery. Editors S. S. Mohapatra, S. Ranjan, N. Dasgupta, R. K. Misra, and S. Thomas (Amsterdam: Elsevier).
Lambert, T., Chavez, C. A., Hernandez-Sanchez, B., Lu, P., Bell, N. S., Ambrosini, A., et al. (2009). Synthesis and Characterization of Titania-Graphene Nanocomposites. J. Phys. Chem. 113 (46), 18912–19823. doi:10.1021/jp905456f
Landau, D. P. (2005). “The Future of Simulations in Materials Science,” in Handbook of Materials Modelling. Editor S. Yip (Dordrecht: Springer).
Laurén, S. (2018). What Is Graphene Oxide? Retrieved from: https://www.biolinscientific.com/blog/what-is-graphene-oxide (Accessed November 28, 2020).
Léonard, A., and Su, B.-L. (2007). “A Mechanistic Study on the Degradation of Highly Ordered, Non-ionic Surfactant Templated Aluminosilicate Mesoporous Materials Al-CMI-1 in Boiling Water,” in Recent Progress in Mesostructured Materials. Editors D. Zhao, S. Qiu, Y. Tang, and C. Yu, 113–116. doi:10.1016/s0167-2991(07)80278-3
Li, D., Müller, M. B., Gilje, S., Kaner, R. B., and Wallace, G. G. (2008). Processable Aqueous Dispersions of Graphene Nanosheets. Nat. Nanotech 3 (2), 101–105. doi:10.1038/nnano.2007.451
Li, W., Tang, X.-Z., Zhang, H.-B., Jiang, Z.-G., Yu, Z.-Z., Du, X.-S., et al. (2011). Simultaneous Surface Functionalization and Reduction of Graphene Oxide with Octadecylamine for Electrically Conductive Polystyrene Composites. Carbon 49 (14), 4724–4730. doi:10.1016/j.carbon.2011.06.077
Liang, A., Jiang, X., Hong, X., Jiang, Y., Shao, Z., and Zhu, D. (2018). Recent Developments Concerning the Dispersion Methods and Mechanisms of Graphene. Coatings 8 (1), 33. doi:10.3390/coatings8010033
Lin, Y., Watson, K. A., Kim, J-W., Baggett, D. W., Working, D. C., and Connell, J. W. (2013). Bulk Preparation of Holey Graphene via Controlled Catalytic Oxidation. Nanoscale 17 (2013), 7814–7824. doi:10.1039/c3nr02135a
Liu, X., Suk, J. W., Boddeti, N. G., Cantley, L., Wang, L., Gray, J. M., et al. (2013). Large Arrays and Properties of 3-Terminal Graphene Nanoelectromechanical Switches. Adv. Mater. 26 (10), 1571–1576. doi:10.1002/adma.201304949
Liu, F., Hu, N., Ning, H., Liu, Y., Li, Y., and Wu, L. (2015). Molecular Dynamics Simulation on Interfacial Mechanical Properties of Polymer Nanocomposites with Wrinkled Graphene. Comput. Mater. Sci. 108, 160–167. doi:10.1016/j.commatsci.2015.06.023
Liu, X., Wang, L.-Y., Zhao, L.-F., He, H.-F., Shao, X.-Y., Fang, G.-B., et al. (2018). Research Progress of Graphene-Based Rubber Nanocomposites. Polym. Compos. 39 (4), 1006–1022. doi:10.1002/pc.24072
Liu, F., Wang, M., Chen, Y., and Gao, J. (2019). Thermal Stability of Graphene in Inert Atmosphere at High Temperature. J. Solid State Chem. 276, 100–103. doi:10.1016/j.jssc.2019.04.008
Liu, X., Wu, Y., Li, M., Jiang, J., Guo, L., Wang, W., et al. (2020). Effects of Graphene Oxide on Microstructure and Mechanical Properties of Graphene Oxide-Geopolymer Composites. Constr. Build. Mater. 247, 1–12. doi:10.1016/j.conbuildmat.2020.118544
Lloyd, R. R. (2009). “Chapter 8-Accelerated Ageing of Geopolymers,” in Geopolymers: Structures, Processing, Properties and Industrial Applications. Editors J. L. Provis, and J. S. J. van Deventer (United Kingdom: Woodhead Publishing).
Long, W., Ye, T., Luo, Q., Wang, Y., and Mei, L. (2019a). Reinforcing Mechanism of Reduced Graphene Oxide on Flexural Strength of Geopolymers: a Synergistic Analysis of Hydration and Chemical Composition. Nanomaterials 9, 1–15. doi:10.3390/nano9121723
Long, W.-J., Ye, T.-H., Li, L.-X., and Feng, G.-L. (2019b). Electrochemical Characterization and Inhibiting Mechanism on Calcium Leaching of Graphene Oxide Reinforced Cement Composites. Nanomaterials 9 (2), 288. doi:10.3390/nano9020288
Ma, H.-L., Zhang, H.-B., Hu, Q.-H., Li, W.-J., Jiang, Z.-G., Yu, Z.-Z., et al. (2012). Functionalization and Reduction of Graphene Oxide with P-Phenylene Diamine for Electrically Conductive and Thermally Stable Polystyrene Composites. ACS Appl. Mater. Inter. 4 (4), 1948–1953. doi:10.1021/am201654b
Ma, J., Meng, Q., Michelmore, A., Kawashima, N., Izzuddin, Z., Bengtsson, C., et al. (2013). Covalently Bonded Interfaces for Polymer/graphene Composites. J. Mater. Chem. A. 1 (13), 4255–4264. doi:10.1039/c3ta01277h
Malik, S., Vijayaraghavan, A., Erni, R., Ariga, K., Khalakhan, I., and Hill, J. P. (2010). High Purity Graphenes Prepared by a Chemical Intercalation Method. Nanoscale 2 (10), 2139–2143. doi:10.1039/c0nr00248h
Manigandan, S., Gunasekar, P., Nithya, S., Revanth, G. D., and Anudeep, A. V. S. C. (2017). Experimental Analysis of Graphene Nanocomposite on Kevlar. IOP Conf. Ser. Mater. Sci. Eng. 225, 1–6. doi:10.1088/1757-899x/225/1/012061
Mao, S., Pu, H., and Chen, J. (2012). Graphene Oxide and its Reduction: Modeling and Experimental Progress. RSC Adv. 2 (7), 2643–2662. doi:10.1039/c2ra00663d
Matalkah, F., and Soroushian, P. (2020). Graphene Nanoplatelet for Enhancement the Mechanical Properties and Durability Characteristics of Alkali Activated Binder. Constr. Build. Mater. 249, 118773. doi:10.1016/j.conbuildmat.2020.118773
Matloff, G. L. (2012). Graphene, the Ultimate Interstellar Solar Sail Material? J. Br. Interplanet. Soc. 65, 1–5.
Merabtene, M., Kacimi, L., and Clastres, P. (2019). Elaboration of Geopolymer Binders from Poor Kaolin and Dam Sludge Waste. 5(6), 1–12. doi:10.1016/j.heliyon.2019.e01938
Mohajerani, A., Suter, D., Jeffrey-Bailey, T., Song, T., Arulrajah, A., Horpibulsuk, S., et al. (2019). Recycling Waste Materials in Geopolymer concrete. Clean. Techn Environ. Pol. 21, 493–515. doi:10.1007/s10098-018-01660-2
Mohamed, S., Leung, T., Jason, F., Mahbubur, R., Fiona, S., John, L., et al. (2014). Graphene/fly Ash Geopolymeric Composites as Self-Sensing Structural Materials. Smart Mater. Struct. 23 (6), 222–231. doi:10.1088/0964-1726/23/6/065006
Mohammed, B. S., Haruna, S., Wahab, M. M. A., Liew, M. S., and Haruna, A. (2019). Mechanical and Microstructural Properties of High Calcium Fly Ash One-Part Geopolymer Cement Made with Granular Activator. Heliyon 5, 1–9. doi:10.1016/j.heliyon.2019.e02255
Moharana, S., Kar, S. K., Mishra, M. K., and Mahaling, R. N. (2019). “Synthesis and Properties of Graphene and Graphene Oxide-Based Polymer Composites,” in Surface Engineering of Graphene. Editors S. Sahoo, S. Tiwari, and G. Nayak (Cham, Switzerland: Springer).
Moon, K., Lee, J., Ruoff, R. S., and Lee, H. (2010a). Reduced Graphene Oxide by Chemical Graphitization. Nat. Commun. 1 (73), 1–6. doi:10.1038/ncomms1067
Moon, K., Li, Z., Yao, Y., Lim, Z., Liang, Q., Agar, J., Song, M., Liu, M., and Wong, C. P. (2010b). in Proceedings: 60th Electronic Components and Technology Conference (ECTC), Las Vegas, NV, June 1–4, 2010 (Las Vegas: IEEE). doi:10.1109/ectc.2010.5490644
Murr, L. E. (2016). “Computer Simulations in Materials Science and engineering,” in Handbook of Materials Structures, Properties, Processing and Performance (Switzerland: Springer International Publisher), 1–17. doi:10.1007/978-3-319-01905-5_61-2
Mustofa, M., and Pintowantoro, S. (2017). The Effect of Si/Al Ratio to Compressive Strength and Water Absorption of Ferronickel Slag-Based Geopolymer. Ijps, 167–172. doi:10.12962/j23546026.y2017i2.2334
Naebe, M., Wang, J., Amini, A., Khayyam, H., Hameed, N., Li, L. H., et al. (2014). Mechanical Property and Structure of Covalent Functionalised Graphene/epoxy Nanocomposites. Sci. Rep. 4 (1), 1–7. doi:10.1038/srep04375
Nainani, R. K., and Thakur, P. (2016). Facile Synthesis of TiO2-RGO Composite with Enhanced Performance for the Photocatalytic Mineralization of Organic Pollutants. Water Sci. Technol. 73 (8), 1927–1936. doi:10.2166/wst.2016.039
National Nanotechnology Coordinated Insfrastructure (2012). Molecules: Lots of Shapes and Sizes. Natl. Nanotechnol. Coord. Insfrastruct. (11), 1–8.
Neklyudov, V. V., Khafizov, N. R., Sedov, I. A., and Dimiev, A. M. (2017). New Insights into the Solubility of Graphene Oxide in Water and Alcohols. Phys. Chem. Chem. Phys. 19, 17000–17008. doi:10.1039/c7cp02303k
Neupane, K., Kidd, P., Chalmers, D., Baweja, D., and Shrestha, R. (2015). Investigation on Compressive Strength Development and Drying Shrinkage of Ambient Cured Powder-Activated Geopolymer Concretes. Aust. J. Civil Eng. 14 (1), 72–83. doi:10.1080/14488353.2016.1163765
Novoselov, K. S., Geim, A. K., Morozov, S. V., Jiang, D., Zhang, Y., Dubunos, S. V., et al. (2004). Electric Field Effect in Atomically Thin Carbon Films. Science 306 (5696), 666–669. doi:10.1126/science.1102896
Pandey, B. (2011). Effect of Carbonation on Leachability and Compressive Strength of Cement Solidified and Geopolymer Solidified Synthetic Metal Wastes. Canada: Lakehead University. Master Thesis.
Pei, S., and Cheng, H.-M. (2012). The Reduction of Graphene Oxide. Carbon 50 (9), 3210–3228. doi:10.1016/j.carbon.2011.11.010
Perera, D. S., Uchida, O., Vance, E. R., and Finnie, K. S. (2007). Influence of Curing Schedule on the Integrity of Geopolymers. J. Mater. Sci. 42 (9), 3099–3106. doi:10.1007/s10853-006-0533-6
Phiri, J., Johansson, L.-S., Gane, P., and Maloney, T. (2018). A Comparative Study of Mechanical, thermal and Electrical Properties of Graphene-, Graphene Oxide- and Reduced Graphene Oxide-Doped Microfibrillated Cellulose Nanocomposites. Composites B: Eng. 147, 104–113. doi:10.1016/j.compositesb.2018.04.018
Pistilli, M. (2020). What Factors Impact Graphene Cost? Retrieved from: https://investingnews.com/daily/tech-investing/nanoscience-investing/graphene-investing/graphene-cost/#:∼:text=Specific%20pricing%20data%20is%20hard,graphene%20has%20many%20exciting%20applications (Accessed November 28, 2020). doi:10.1109/icmew46912.2020.9105972
Porwal, H., Grasso, S., and Reece, M. J. (2013). Review of Graphene-Ceramic Matrix Composites. Adv. Appl. Ceramics 112 (8), 443–454. doi:10.1179/174367613x13764308970581
J. L. Provis, and J. S. J. Van Deventer in Geopolymers: Structures, Processing, Properties, and Industrial Applications (Amsterdam: Elsevier).
Provis, J. L., Duxson, P., and van Deventer, J. S. J. (2010). The Role of Particle Technology in Developing Sustainable Construction Materials. Adv. Powder Technol. 21 (1), 2–7. doi:10.1016/j.apt.2009.10.006
Publons (2021). Graphene Publications. Retrieved from: https://publons.com/publon/?title=graphene (Accessed November 28, 2020).
Quan, J.-X., Zhang, H.-Y., Zhou, L.-Y., Zhou, Y., and Su, J.-W. (2017). Experimental Study of Geopolymer Used as Adhesive in anchorage of Steel Bars. Proced. Eng. 210, 45–52. doi:10.1016/j.proeng.2017.11.047
Ramesha, G. K., Vijaya Kumara, A., Muralidhara, H. B., and Sampath, S. (2011). Graphene and Graphene Oxide as Effective Adsorbents toward Anionic and Cationic Dyes. J. Colloid Interf. Sci. 361, 270–277. doi:10.1016/j.jcis.2011.05.050
Randviir, E. P., Brownson, D. A. C., and Banks, C. E. (2014). A Decade of Graphene Research: Production, Applications and Outlook. Mater. Today 17 (9), 426–432. doi:10.1016/j.mattod.2014.06.001
Ranjbar, N., Mehrali, M., Mehrali, M., Alengaram, U. J., and Jumaat, M. Z. (2015). Graphene Nanoplatelet-Fly Ash Based Geopolymer Composites. Cem. Concr. Res. 76, 222–231. doi:10.1016/j.cemconres.2015.06.003
Raphaëlle, P., Martin, C., and Raphaël, B. (2019). Influence of the Initial Water Content in Flash Calcined Metakaolin-Based Geopolymer. Constr. Build. Mater. 201, 421–429. doi:10.1016/j.conbuildmat.2018.12.201
Rehman, S., Ibrahim, Z., Memon, S., Javed, M., and Khushnood, R. (2017). A Sustainable Graphene Based Cement Composite. Sustainability 9 (7), 1229. doi:10.3390/su9071229
Rehman, S. K. U., Ibrahim, Z., Jameel, M., Memon, S. A., Javed, M. F., Aslam, M., et al. (2018). Assessment of Rheological and Piezoresistive Properties of Graphene-Based Cement Composites. Int. J. Concr. Struct. Mater. 12 (1), 1–23. doi:10.1186/s40069-018-0293-0
Ren, X., and Zhang, L. (2019). Experimental Study of Geopolymer concrete Produced from Waste concrete. J. Mater. Civil Eng. 31 (7), 1–14. doi:10.1061/(asce)mt.1943-5533.0002750
Roberts, M. W., Clemons, C. B., Wilber, J. P., Young, G. W., Buldum, A., and Quinn, D. D. (2010). Continuum Plate Theory and Atomistic Modeling to Find the Flexural Rigidity of a Graphene Sheet Interacting with a Substrate. J. Nanotechnol. 2010, 1–8. doi:10.1155/2010/868492
Ruzaidi, C. M., Abdullah, A., Mohd Mustafa, A. B. A., Kamarudin, H., and Ismail, A. N. (2014). Compressive Strength of Fly Ash Based Geopolymer/glass Fiber Composite via Filament Winding. Key Eng. Mater. 594-595, 78–82. doi:10.4028/www.scientific.net/kem.594-595.78
Saafi, M., Tang, L., Fung, J., Rahman, M., and Liggat, J. (2015). Enhanced Properties of Graphene/fly Ash Geopolymeric Composite Cement. Cem. Concr. Res. 67, 292–299. doi:10.1016/j.cemconres.2014.08.011
Sagoe-Crentsil, K., and Weng, L. (2007). Dissolution Processes, Hydrolysis and Condensation Reactions during Geopolymer Synthesis: Part II. High Si/Al Ratio Systems. J. Mater. Sci. 42 (9), 3007–3014. doi:10.1007/s10853-006-0818-9
Santoli, S. (2010). “Chapter: Carbon Nanotube Membrane Solar Sails: A challenge for Extremely Fast Space Flight,” in Carbon Nanotubes. Editor J. M. Marulanda (Rijeka: InTech Open), 591–610.
Shi, C., Tu, Z., Guo, M.-Z., and Wang, D. (2017). “Accelerated Carbonation as a Fast Curing Technology for concrete Blocks,” in Sustainable and Nonconventional Construction Materials Using Inorganic Bonded Fiber Composites. Editors H. S. Junior, J. Fiorelli, and S. F. dos Santos, 313–341. doi:10.1016/b978-0-08-102001-2.00015-2
Skoda, M., Dudek, I., Jarosz, A., and Szukiewicz, D. (2014). Graphene: One Material, many Possibilities-Application Difficulties in Biological Systems. J. Nanomater. 2014, 1–11. doi:10.1155/2014/890246
Smith, A. T., LaChance, A. M., Zeng, S., Liu, B., and Sun, L. (2019). Synthesis, Properties, and Applications of Graphene Oxide/reduced Graphene Oxide and Their Nanocomposites. Nano Mater. Sci. 1 (1), 31–47. doi:10.1016/j.nanoms.2019.02.004
Spasenovic, M. (2020). The price of Graphene. Retrieved from: https://www.graphenea.com/pages/graphene-price#.YGZZHrDitki (Accessed November 28, 2020).
Sun, Z., and Vollpracht, A. (2018). Isothermal Calorimetry and In-Situ XRD Study of the NaOH Activated Fly Ash, Metakaolin and Slag. Cem. Concr. Res. 103, 110–122. doi:10.1016/j.cemconres.2017.10.004
Sun, C., Huang, Y., Shen, Q., Wang, W., Pan, W., Zhong, P., et al. (2020). Embedding Two-Dimensional Graphene Array in Ceramic Matrix. Sci. Adv. 6 (39), 1–12. doi:10.1126/sciadv.abb1338
Taylor, G. (2019). Branching Out: Making Graphene from Gum Trees. Retrieved from: https://www.rmit.edu.au/news/all-news/2019/jun/graphene-from-gum-trees (Accessed November 28, 2020). doi:10.1007/978-3-030-30988-6
Temuujin, J., van Riessen, A., and Williams, R. (2009). Influence of Calcium Compounds on the Mechanical Properties of Fly Ash Geopolymer Pastes. J. Hazard. Mater. 167, 82–88. doi:10.1016/j.jhazmat.2008.12.1212009
Tiwari, S. K., Sahoo, S., Wang, N., and Huczko, A. (2020). Graphene Research and Their Outputs: Status and prospect. J. Sci. Adv. Mater. Devices 5 (1), 10–29. doi:10.1016/j.jsamd.2020.01.006
Tulyaganov, D. U., Reddy, A. A., Kharton, V. V., and Ferreira, J. M. F. (2013). Aluminosilicate-based Sealants for SOFCs and Other Electrochemical Applications − A Brief Review. J. Power Sourc. 242, 486–502. doi:10.1016/j.jpowsour.2013.05.099
Van Jaarsveld, J. G. S., van Deventer, J. S. J., and Lukey, G. C. (2002). The Effect of Composition and Temperature on the Properties of Fly Ash- and Kaolinite- Based Geopolymers. Chem. Eng. J. 89 (1-3), 63–73. doi:10.1016/s1385-8947(02)00025-6
Vogt, O., Ukrainczyk, N., Ballschmiede, C., and Koenders, E. (2019). Reactivity and Microstructure of Metakaolin Based Geopolymers: Effect of Fly Ash and Liquid/solid Contents. Materials 12 (3485), 1–21. doi:10.3390/ma12213485
Wan, Q., Rao, F., Song, S., García, R. E., Estrella, R. M., Patiño, C. L., et al. (2017). Geopolymerization Reaction, Microstructure and Simulation of Metakaolin-Based Geopolymers at Extended Si/Al Ratios. Cem. Concr. Compos. 79, 45–52. doi:10.1016/j.cemconcomp.2017.01.014
Wang, B., Jiang, R., and Wu, Z. (2016). Investigation of the Mechanical Properties and Microstructure of Graphene Nanoplatelet-Cement Composite. Nanomaterials 6 (11), 200. doi:10.3390/nano6110200
Xie, K., Liu, Y., Tian, Y., Wu, X., Wu, L., Mo, Y., et al. (2021). Improving the Flexibility of Graphene Nanosheets Films by Using Aramid Nanofiber Framework. Compos. A: Appl. Sci. Manuf. 142, 1–10. doi:10.1016/j.compositesa.2020.106265
Xu, G., Zhong, J., and Shi, X. (2018). Influence of Graphene Oxide in a Chemically Activated Fly Ash. Fuel 226, 644–657. doi:10.1016/j.fuel.2018.04.033
Yaghoubi, M., Arulrajah, A., Miri Disfani, M., Horpibulsuk, S., and Leong, M. (2020). Compressibility and Strength Development of Geopolymer Stabilized Columns Cured under Stress. Soils Found. 60 (5), 1241–1250. doi:10.1016/j.sandf.2020.07.005
Yan, S., He, P., Jia, D., Yang, Z., Duan, X., Wang, S., et al. (2015). In Situ fabrication and Characterization of Graphene/geopolymer Composites. Ceram. Int. 41 (9), 11242–11250. doi:10.1016/j.ceramint.2015.05.075
Yan, S., He, P., Jia, D., Duan, X., Yang, Z., Wang, S., et al. (2016a). In Situ Processing of Graphene/Leucite Nanocomposite Through Graphene Oxide/Geopolymer. J. Am. Ceram. Soc. 99 (4), 1164–1173. doi:10.1111/jace.14089
Yan, S., He, P., Jia, D., Yang, Z., Duan, X., Wang, S., et al. (2016b). Effect of Reduced Graphene Oxide Content on the Microstructure and Mechanical Properties of Graphene-Geopolymer Nanocomposites. Ceram. Int. 42 (1), 752–758. doi:10.1016/j.ceramint.2015.08.176
Yan, S., He, P., Jia, D., Duan, X., Yang, Z., Wang, S., et al. (2017). Effects of Graphene Oxide on the Geopolymerization Mechanism Determined by Quenching the Reaction at Intermediate States. RSC Adv. 7, 13498–13508. doi:10.1039/c6ra26340b
Yao, J., Qiu, H., He, H., Chen, X., and Hao, G. (2019). “Experimental Research and Application of Geopolymer in Soft Soil Foundation Treatment,” in Innovative Infrastructure Solutions Using Geosynthetics (New York, NY: Springer), 82–94. doi:10.1007/978-3-030-34242-5_8
Yavari, F., Rafiee, M. A., Rafiee, J., Yu, Z.-Z., and Koratkar, N. (2010). Dramatic Increase in Fatigue Life in Hierarchical Graphene Composites. ACS Appl. Mater. Inter. 2 (10), 2738–2743. doi:10.1021/am100728r
Yeddula, B. S. R., and Karthiyaini, S. (2020). Experimental Investigations and Prediction of Thermal Behaviour of Ferrosialate-Based Geopolymer Mortars. Arab J. Sci. Eng. 45, 3937–3958. doi:10.1007/s13369-019-04314-7
Yeswanth sai, T., and Durga Prasad, P. N. V. (2020). Comparative Study on Flyash GGBS Based Geopolymer concrete Using Graphene. J. Emerging Tech. Innovative Res. 7 (4), 2094–2099.
Yuan, F.-Y., Zhang, H.-B., Li, X., Ma, H.-L., Li, X.-Z., and Yu, Z.-Z. (2014). In Situ chemical Reduction and Functionalization of Graphene Oxide for Electrically Conductive Phenol Formaldehyde Composites. Carbon 68, 653–661. doi:10.1016/j.carbon.2013.11.046
Zhang, B., and Chen, T. (2019). Study of Ultrasonic Dispersion of Graphene Nanoplatelets. Materials 12 (11), 1–20. doi:10.3390/ma12111757
Zhang, G., and Lu, J. (2018). Experimental Research on the Mechanical Properties of Graphene Geopolymer. AIP Adv. 8 (6), 1–10. doi:10.1063/1.5020547
Zhang, L. W., Kai, M. F., and Chen, X. H. (2020). Si-doped Graphene in Geopolymer: Its Interfacial Chemical Bonding, Structure Evolution and Ultrastrong Reinforcing Ability. Cem. Concr. Compos. 109, 1–12. doi:10.1016/j.cemconcomp.2020.103522
Zhang, Q., Gao, Y., Xu, Z., Wang, S., Kobayashi, H., and Wang, J. (2020). The Effects of Oxygen Functional Groups on Graphene Oxide on the Efficient Adsorption of Radioactive Iodine. JMaterials 13, 1–13. doi:10.3390/ma13245770
Zhong, J., Zhou, G.-X., He, P.-G., Yang, Z.-H., and Jia, D.-C. (2017). 3D Printing strong and Conductive Geo-Polymer Nanocomposite Structures Modified by Graphene Oxide. Carbon 117, 421–426. doi:10.1016/j.carbon.2017.02.102
Zhu, C-Q., Wang, X-Z., Wang, R., Chen, H-Y., and Meng, Q.-S. (2013). Experimental Microscopic Study of Inner Pores of Calcareous Sand. J. Mater. Res. Innov. 18 (2), 207–214. doi:10.1179/1432891714Z.000000000408
Zohhadi, N. (2014). Functionalized Graphitic Nanoreinforcement for Cement Composites. Columbia: University of South Carolina. PhD Thesis.
Keywords: graphene, geopolymer, nanocomposite, flexural, compressive
Citation: Tay CH and Norkhairunnisa M (2021) Mechanical Strength of Graphene Reinforced Geopolymer Nanocomposites: A Review. Front. Mater. 8:661013. doi: 10.3389/fmats.2021.661013
Received: 30 January 2021; Accepted: 05 July 2021;
Published: 20 July 2021.
Edited by:
Mohd Mustafa Al Bakri Abdullah, Universiti Malaysia Perlis, MalaysiaReviewed by:
Neven Ukrainczyk, Darmstadt University of Technology, GermanyRomisuhani Binti Ahmad, Universiti Malaysia Perlis, Malaysia
Zarina Yahya, Universiti Malaysia Perlis, Malaysia
Yun Ming Liew, Universiti Malaysia Perlis, Malaysia
Copyright © 2021 Tay and Norkhairunnisa. This is an open-access article distributed under the terms of the Creative Commons Attribution License (CC BY). The use, distribution or reproduction in other forums is permitted, provided the original author(s) and the copyright owner(s) are credited and that the original publication in this journal is cited, in accordance with accepted academic practice. No use, distribution or reproduction is permitted which does not comply with these terms.
*Correspondence: Mazlan Norkhairunnisa, bm9ya2hhaXJ1bm5pc2FAdXBtLmVkdS5teQ==
†Materials Processing and Technology Laboratory, Institute of Advanced Technology, Universiti Putra Malaysia, Selangor, Malaysia