- Institute of Materials, China Academy of Engineering Physics, Mianyang, China
TiO2 nanowire arrays in situ grown on Ti foil (TiO2/Ti) were prepared to remove uranium (VI) from aqueous solution. As the Ti foil serves as a carrier for TiO2, the TiO2/Ti adsorbent can be effortlessly retrieved from aqueous solutions by tweezers after adsorption. The presence of TiO2 nanowire arrays on Ti foil was verified by X-ray diffraction and scanning electron microscopy. Parameters in the adsorption process were fully evaluated, including solution pH, contact time, temperature, and uranium (VI) concentration. The adsorption was most efficient in the pH range of 5.0 to 9.0. The maximum uranium (VI) adsorption capacity of TiO2/Ti, based on the Langmuir model, was 354.5 mg g–1 at pH 5.0 and T = 323 K. Thermodynamic parameters showed that the adsorption of uranium (VI) on TiO2/Ti is endothermic and spontaneous. The adsorption capacity of TiO2/Ti remained essentially unchanged after three adsorption–desorption cycles in uranium (VI) solutions. Our results support the application of this adsorbent to removal of uranium (VI) from diversified aqueous samples.
Introduction
With the rapid development of nuclear power, environmental pollution by uranium has raised global concerns. For example, uranium mining and hydrometallurgical processes are known to cause serious water contamination. Uranium contamination is harmful and long-lasting because of its radiotoxicity and chemotoxicity (Basheer, 2018a,b). The potential radiation damage and chemotoxicity of uranium pose serious threats to human health (Kurttio et al., 2006; Prat et al., 2009; Schnug and Lottermoser, 2013; Manigandan and Chandar Shekar, 2014). Meanwhile, uranium resources for nuclear power face potential depletion in the near future and cannot meet the urgent industrial demands. Therefore, the removal and recovery of uranium from waste and natural water are critically important for environmental protection and conservation of energy resources (Xie et al., 2019). Conventional methods for the treatment of water containing uranium include chemical precipitation, adsorption, solvent extraction, electrocoagulation, membrane filtration, and biological methods (Shi et al., 2009; Santos and Ladeira, 2011; Stylo et al., 2013; Tripathi et al., 2013; Azari et al., 2014, 2017, 2019; Beltrami et al., 2014; Karami et al., 2017; Li et al., 2017; Zhu et al., 2019; Zhong et al., 2021). Among various methods, adsorption techniques are relatively efficient, cost-effective, and easy to implement, exhibiting great potential for removing uranium (VI) from aqueous solutions (Ali et al., 2019; Xie et al., 2019; Azari et al., 2020; Liu et al., 2021).
To date, assorted types of adsorbents have been developed for uranium, such as activated carbon, oxides, graphene, nanocomposites, and polymers (Nair et al., 2014; Xu et al., 2015; Zhang et al., 2015; Wang et al., 2016; El-Maghrabi et al., 2017). Among these adsorbents, metal oxides, especially titanium dioxide, have attracted considerable attention because of its high adsorptive capacity for uranium (VI), good radiochemical stability, and negligible solubility in both acidic and alkaline solutions (Comarmond et al., 2011; Tatarchuk et al., 2019; Wang et al., 2019). However, the separation of TiO2-based adsorbents from aqueous solutions after adsorption is usually complicated and time-consuming. To solve this problem, methods based on external magnetic field or coprecipitation have been explored (Tan et al., 2015; Wen et al., 2018).
In this study, we prepared TiO2 nanowire arrays (NWAs) on Ti foil (TiO2/Ti) via in situ growth. The TiO2 nanowires provide abundant active surface sites for uranium adsorption. The Ti foil serves as a carrier for TiO2 and can be removed from the solution with tweezers, achieving simple and effective separation of the adsorbent. The chemical components and structure of TiO2/Ti were characterized by X-ray diffraction (XRD) and scanning electron microscope (SEM), and the uranium (VI) adsorption process by TiO2/Ti was comprehensively evaluated. The results reveal that the TiO2/Ti adsorbent has superior uranium adsorption capacity (VI) and excellent reusability.
Materials and Methods
Ti foil, NaOH, HCl, ethanol, and sodium citrate were purchased from Alfa Aesar. The purity of Ti foil is > 99.9%, and other reagents are analytically pure. Uranyl nitrate was provided by Dingtian Chem. Corp. (Xi’an). All chemical reagents were used without further purification.
The fabrication method of TiO2 NWAs on Ti foil is as follows. A piece of Ti foil was ultrasonically cleaned in deionized (DI) water for 10 min and in ethanol for 10 min. It was placed in a 50-mL Teflon-lined stainless-steel autoclave with 30 mL 1 M NaOH and 0.01 M sodium citrate aqueous solution. The hydrothermal reaction was performed at 220°C for 24 h. The Ti foil covered with TiO2 NWA was immersed in 1 M HCl solution for 5 min to replace Na+ with H+. Then, the Ti foil with TiO2 NWAs was rinsed with DI water and ethanol. Finally, the obtained TiO2/Ti was sintered at 450°C for 30 min.
In a typical adsorption experiment, a given amount of TiO2/Ti was mixed with 20 mL UO2(NO3)2⋅6H2O solution in a conical flask. The conical flask was placed in a thermostatic water shaker at a speed of 150 revolutions/min. In this process, the experimental parameters were adjusted, including solution concentration, solution pH, adsorption time, and temperature. The solution pH was adjusted by adding 0.1 M HNO3 or NaOH solution. After the adsorption processes, the VIU-TiO2/Ti samples were collected with tweezers. The adsorption capacity (Qe) and adsorption removal efficiency were calculated according to the following equations (Tan et al., 2015):
where, C0 and Ce (mg L–1) are concentrations of uranium (VI) at the initial and equilibrium states, respectively; m (g) is the weight of TiO2/Ti adsorbent, and V (L) is the volume of the solution. The distribution coefficient (KD) was obtained according to Eq. (3) (Wang et al., 2019):
The phase constitution of TiO2/Ti was analyzed by XRD on a Tongda TD-3500 diffractometer at a scanning rate of 4°/min. The microstructure was studied by an SEM (Helios NanoLab 600i, ZEISS).
Desorption was carried out by soaking the VIU-TiO2/Ti samples in 20 mL 0.1 mol L–1 HCl solution for 60 min, followed by thorough washing with DI water. The desorbed TiO2/Ti adsorbents were then reused.
Results and Discussion
Characterization of Samples
The XRD patterns of TiO2/Ti are shown in Figure 1. All characteristic peaks of TiO2 were observed (Anatase, JCPDS #71-1166). The peaks of Ti were marked with stars in Figure 1. This result indicated successful fabrication of crystalline TiO2 on the Ti foil. As shown in Figure 2, the peaks that appeared in 600 and 1,040 cm–1 were Ti-O bending vibration, which further proved the TiO2 has been successfully fabricated. The broad bands in the range of 3,000–3,600 cm–1 and 1,600–1,645 cm–1 were H–O–H bending vibration. It indicated that the fabricated TiO2 nanowires adsorbed water while they were exposed to the air. In Figure 3, homogenous TiO2 NWAs were observed on the Ti foil. The length of TiO2 NWs is greater than 10 μm, and the thickness of the TiO2 film on Ti foil substrate is approximately 15 μm. The large specific surface area can potentially provide superior adsorption performance.
Effect of Solution pH
Solution pH plays an important role in the adsorption process owing to its influences on the aqueous chemical properties of uranium and the adsorbent (Lamb et al., 2016; Song et al., 2017). Figure 4 depicts the effect of initial pH in aqueous solution on uranium (VI) removal efficiency. The removal of uranium (VI) by TiO2/Ti was tested by varying pH from 1.5 to 9.0. The removal efficiency sharply increased as pH increased from 3.0 to 5.0 and remained almost unchanged from 5.0 to 9.0. At pH < 3.0, the binding sites on TiO2/Ti surface become positively charged, and the adsorption of uranyl cations is negligible. At pH > 3.0, the surface of TiO2/Ti becomes deprotonated, resulting in sharp increase of uranium (VI) adsorption. Further increase in pH may lead to the formation of anionic uranyl complexes, which suppress the adsorption of uranium (VI) onto TiO2/Ti materials (Tatarchuk et al., 2019; Wang et al., 2019).
Effect of Contact Time and Adsorption Dynamics
Figure 5 shows the effect of contact time on uranium (VI) removal by TiO2/Ti. Contact times from 5 min to 24 h were evaluated.
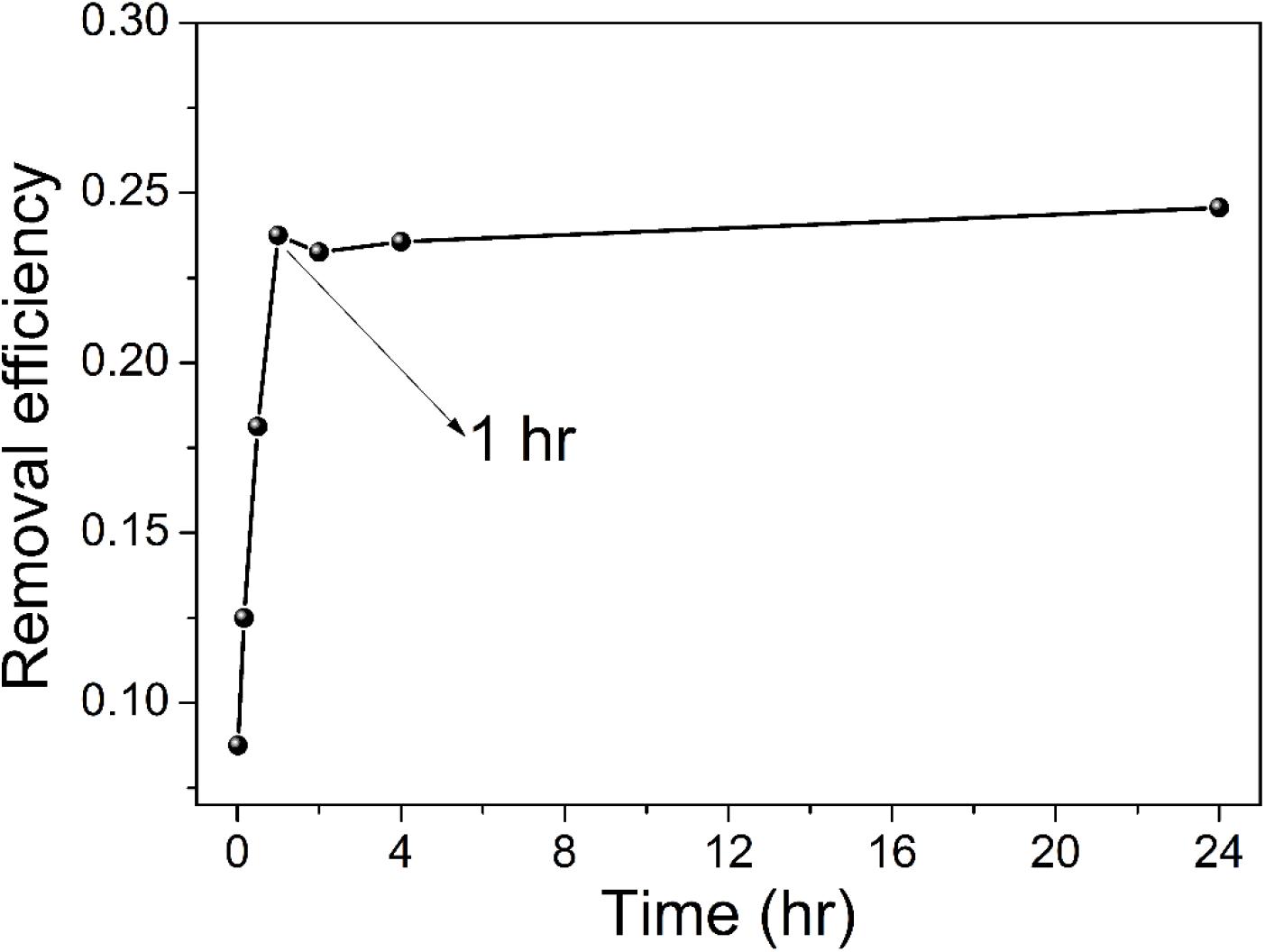
Figure 5. The relationship between uranium removal efficiency by TiO2/Ti and contact time (T = 298 K, pH 5.0, C0 = 40 mg L–1, mixing time 1–1,440 min, and amount of TiO2/Ti 0.025 g).
As shown in Figure 5, uranium (VI) removal increases with the increase of contact time, and the adsorption process reaches equilibrium after 1 h. To probe the kinetic mechanism of uranium adsorption on TiO2/Ti, the adsorption data were analyzed using pseudo–first-order and pseudo–second-order models. The pseudo–first-order and pseudo–second-order kinetic equations are (Ho and McKay, 1999; Ho, 2006):
where, k1 (min–1) represents the pseudo–first-order adsorption rate constant, t (min) is time. Qe and Qt (mg g–1) are adsorption capacity of uranium at equilibrium and at time t, respectively. k2 (g mg–1 min–1) is the pseudo–second-order adsorption rate constant. The pseudo-first-order and pseudo-second-order fitting curves are shown in Figures 6A,B, respectively. The relevant kinetics data are presented in Table 1. The adsorption of uranium (VI) on TiO2/Ti more likely follows the pseudo–second-order model (R2 = 0.99) than the pseudo–first-order model (R2 = 0.39). The pseudo–second-order kinetic model further indicates that the dominant mechanism for uranium (VI) adsorption on TiO2/Ti is chemical adsorption or strong surface complexation rather than mass transport (Ho and McKay, 2000; Kamari et al., 2009).
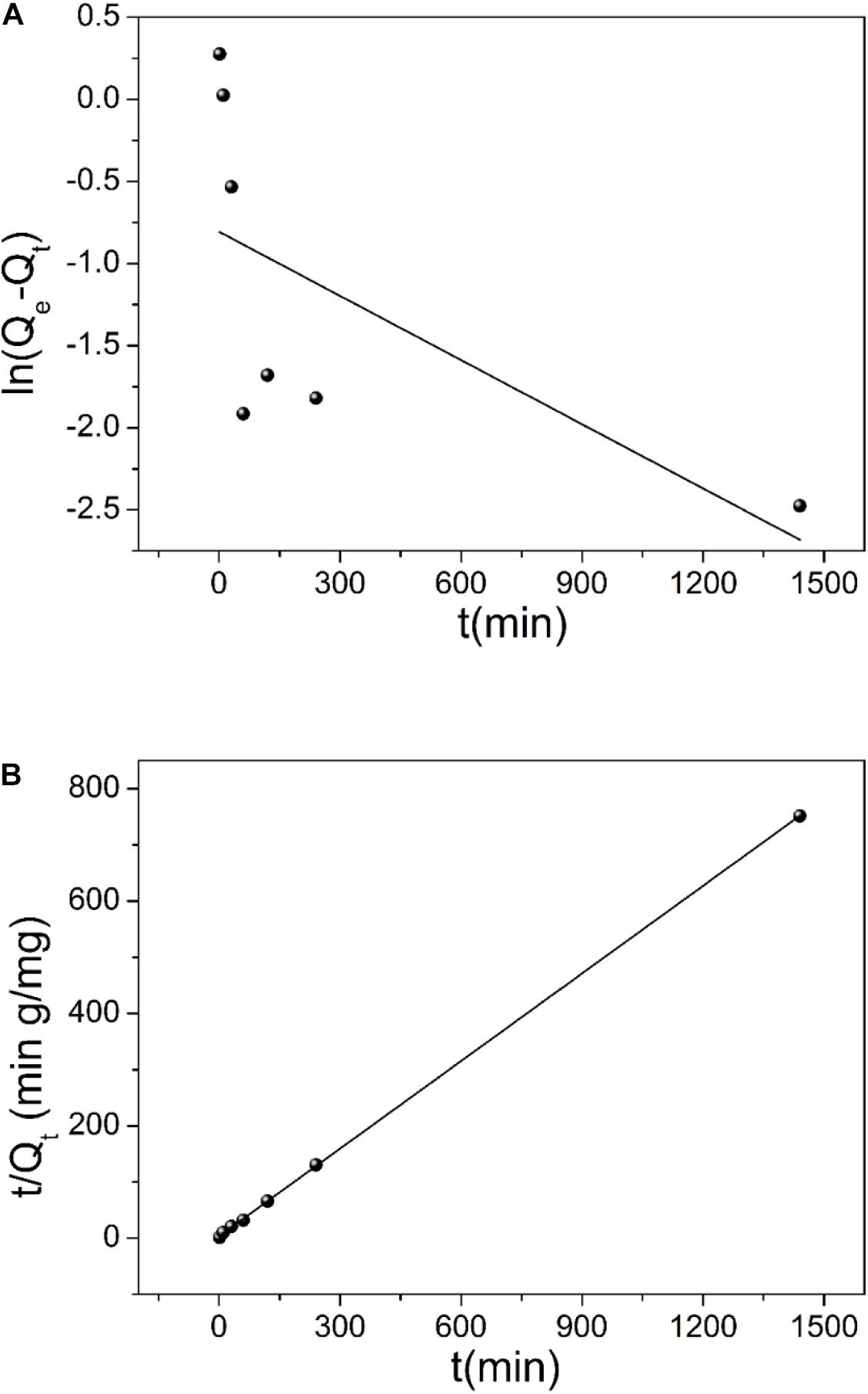
Figure 6. The fitting of pseudo–first-order (A) and pseudo–second-order (B) kinetic models for uranium (VI) adsorption on TiO2/Ti.
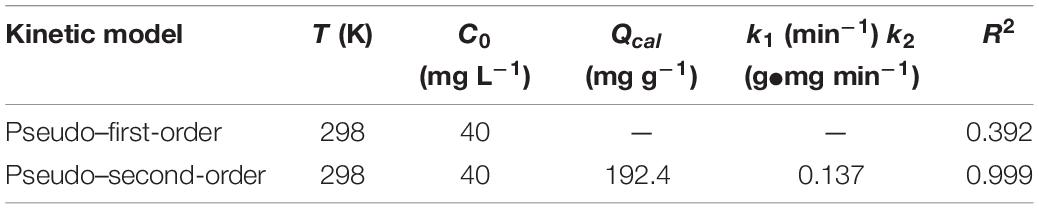
Table 1. Kinetic parameters of different models for uranium (VI) adsorption on TiO2/Ti (temperature 298 K, pH 5.0, mixing time 60 min, and amount of TiO2/Ti 0.025 g).
Adsorption Isotherms of Uranium
According to Figure 7, the adsorption capacity increases with elevating temperature. The adsorption data were analyzed using the Langmuir and Freundlich isotherm models (Freundlich, 1906; Langmuir, 1918). The linearized form of the Langmuir equation is:
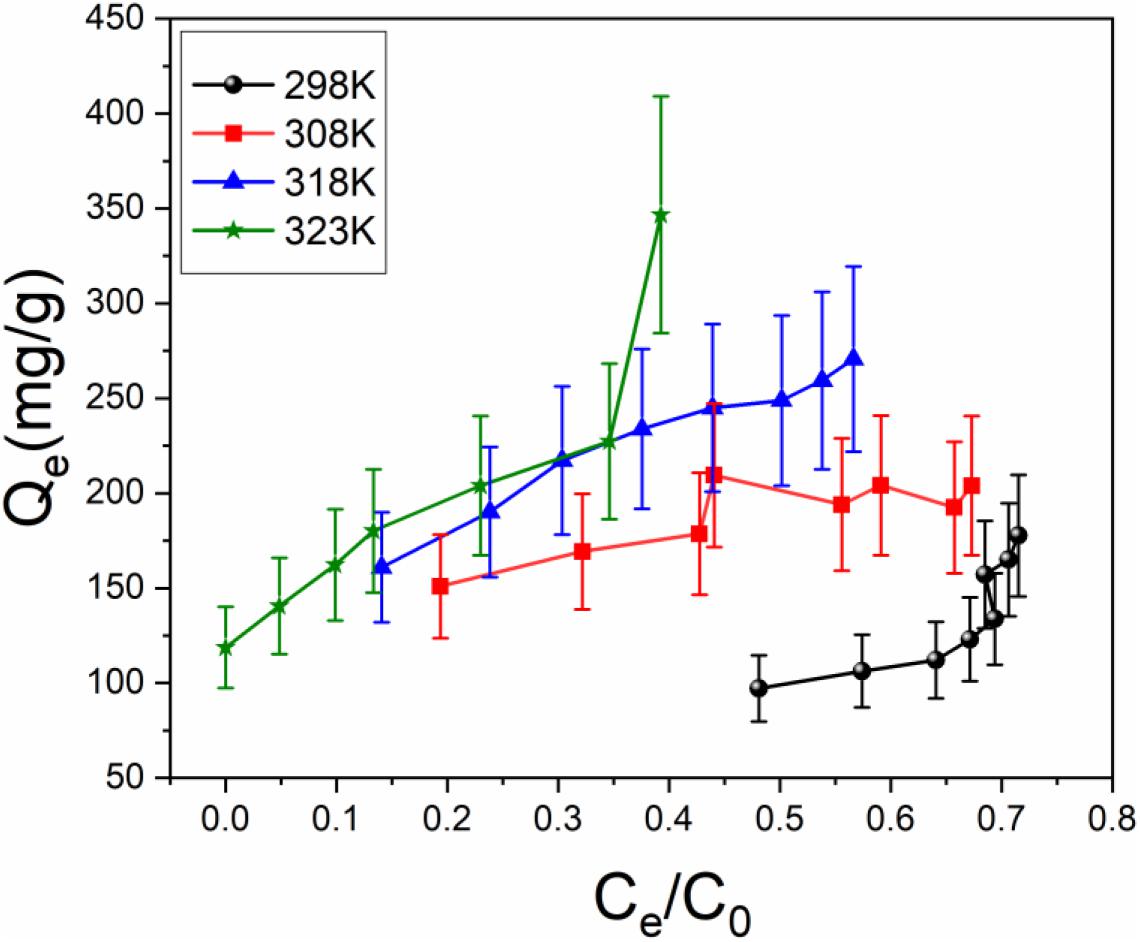
Figure 7. Adsorption isotherms of TiO2/Ti for uranium (VI) at different temperatures (temperature 298–323 K, pH 5.0, amount of TiO2/Ti 0.025 g, and mixing time 60 min).
where, KL (L mg–1) is the Langmuir constant; Qe and Qm (mg g–1) are the equilibrium adsorption capacity and maximum adsorption capacity, respectively. Qm and KL can be calculated from the linear regression of Ce/Qe against Ce (Figure 8A).
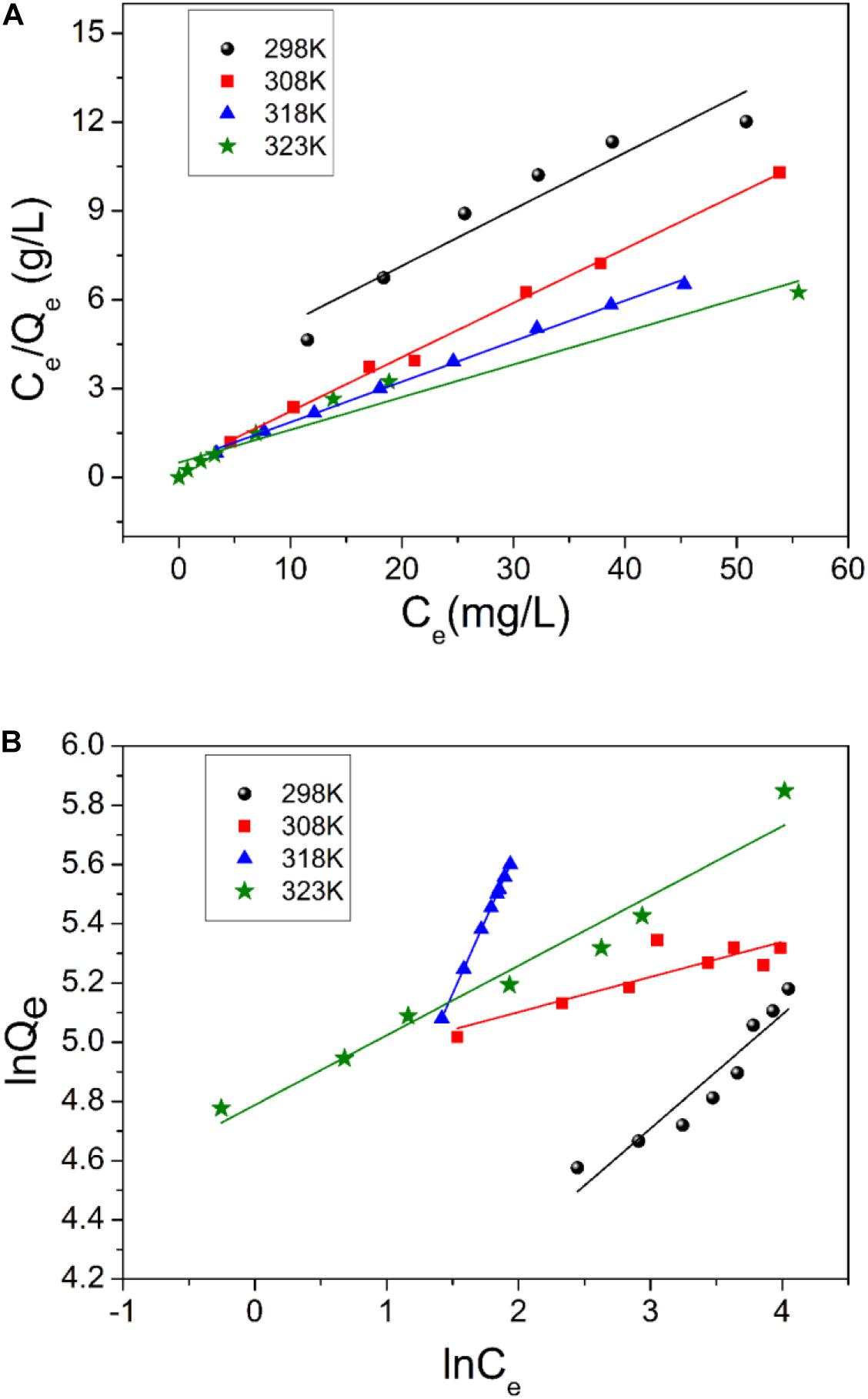
Figure 8. Langmuir (A) and Freundlich (B) plot for the adsorption of uranium (VI) on TiO2/Ti (temperature 298–323 K, pH 5.0, amount of TiO2/Ti 0.025 g, and mixing time 60 min).
The Freundlich isotherm is applicable to the adsorption on heterogeneous surfaces and multilayer adsorption. The linear form of Freundlich isotherm is:
where, KF is the Freundlich constant, and n is adsorption intensity. KF and n constants can be calculated from the linear regression of lnQe versus lnCe (Figure 8B).
The parameters of Langmuir and Freundlich equations are summarized in Table 2. The correlation coefficients (R2) indicate that the Langmuir model better accounts for the behavior of uranium (VI) adsorption by TiO2/Ti. According to the Langmuir isotherm, the monolayer saturation capacity of TiO2/Ti is about 354.5 mg g–1 for uranium (VI) at 323 K. The comparison of uranium (VI) adsorption capacity onto different TiO2 and its composites at 298 K is listed in Table 3.
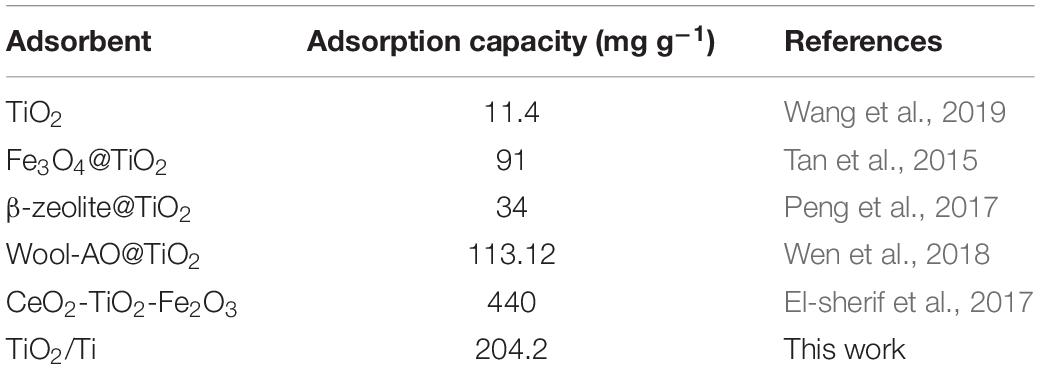
Table 3. The comparison of uranium (I) adsorption capacity onto different TiO2 and its composites at 298 K.
Effect of Temperature and Adsorption Thermodynamics
The effect of temperature on the uranium (VI) adsorption by TiO2/Ti was investigated at 298, 308, 318, and 323 K. Based on Figure 7 and Table 2, the adsorption capacity of uranium (VI) increases from 204.2 to 354.5 mg g–1 as the temperature rises from 298 to 323 K, suggesting that higher temperature is advantageous for uranium (VI) adsorption.
To understand the thermodynamic aspect of the adsorption process, the standard free energy change (ΔG°), standard enthalpy change (ΔH°), and standard entropy change (ΔS°) are calculated from the adsorption data at different temperatures via the following equations:
where, KD is the distribution coefficient (mL g–1), R is the ideal gas constant (8.314 J mol–1 K–1), and T is the temperature (K). The ΔH° and ΔS° values are determined from the slope and intercept of the linear regression of lnKD versus 1/T, respectively (Figure 9). The thermodynamic parameters are in Table 4. The positive ΔH° indicates an endothermic adsorption process of uranium on TiO2/Ti. The negative ΔG° indicates a spontaneous adsorption process. Moreover, the absolute value of ΔG° increases with elevating temperature, suggesting higher temperature is conducive to the adsorption of metal ions. The positive ΔS° indicates an increase of randomness on the adsorbent surface during the adsorption process. Therefore, the adsorption of uranium (VI) on TiO2/Ti is endothermic and spontaneous.
Desorption and Reusability Study
We performed two desorption experiments to assess the reusability of TiO2/Ti adsorbent. First, as depicted in Scheme 1, 20 mL uranium (VI) solution was used as the target solution. A piece of TiO2/Ti was immersed in the target solution for 60 min. After adsorption, the adsorbents were removed from the solution with tweezers and treated with 0.1 mol L–1 HCl solution for 60 min. Then, the desorbed TiO2/Ti adsorbents were reused in an identical target solution. The above adsorption–desorption cycle was repeated nine times. The initial uranium (VI) amount exceeded the maximum adsorption capacity of TiO2/Ti. The adsorption capacity of uranium (VI) and the residual uranium (VI) in target solution after every cycle were recorded. As shown in Figure 10, the adsorption capacity (Qe) was approximately 355 mg g–1 in the first cycle and decreased to 45 mg g–1 in the ninth time. This could be attributed to the continuous decrease of uranium (VI) concentration after each adsorption cycle. The residual uranium (VI) in the target solution was approximately 8% after nine cycles.
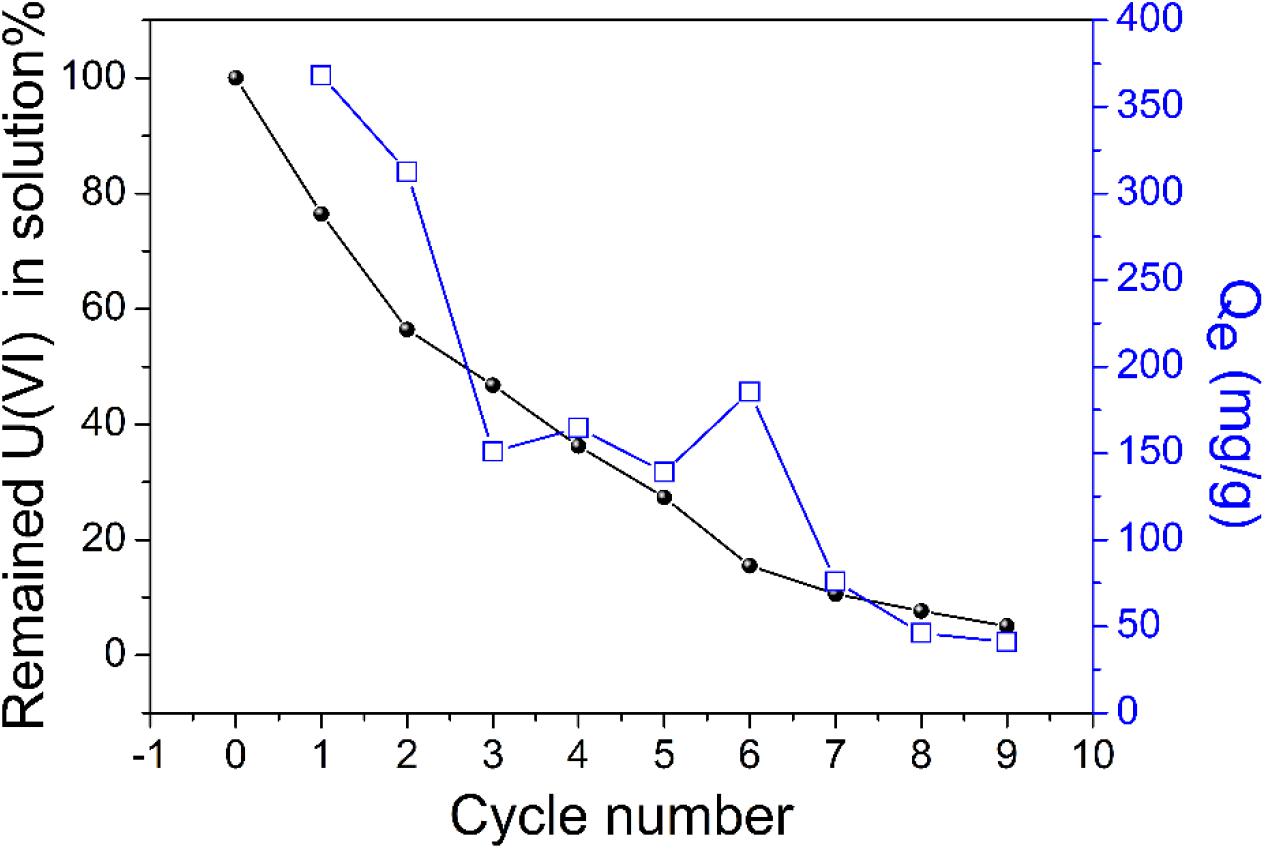
Figure 10. The reusability of TiO2/Ti adsorbents in the target solution (temperature 298 K, pH 5.0, amount of TiO2/Ti 0.025 g, mixing time 60 min, and C0 = 200 mg L–1).
Second, three identical uranium (VI) solutions (I, II, and III) were tested with the same piece of TiO2/Ti. It was first immersed in solution I for 60 min. After adsorption, it was retrieved from the solution with tweezers and treated with 0.1 mol L–1 HCl solution for 60 min. Next, the recovered TiO2/Ti piece underwent the same adsorption–desorption procedure in solution II and then in solution III. According to Figure 11, the adsorption capacity (Qe) of TiO2/Ti remained essentially unchanged during three successive adsorption cycles.
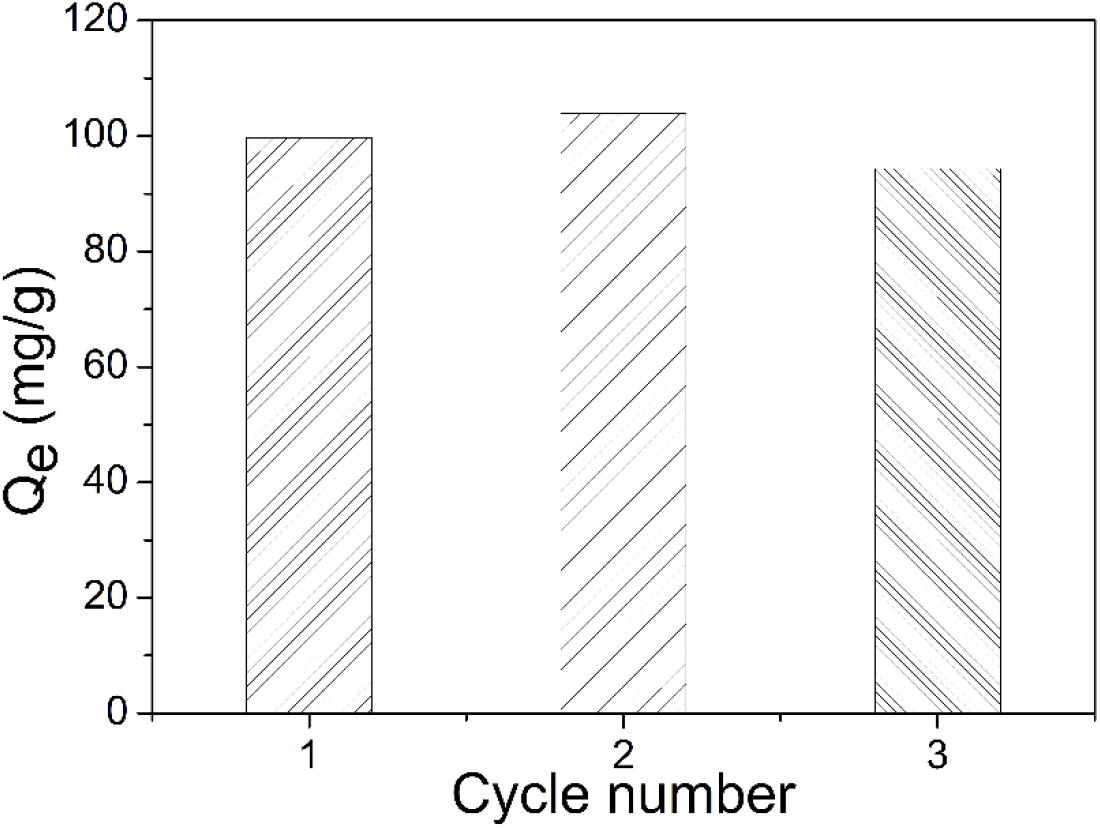
Figure 11. The reusability of TiO2/Ti adsorbents evaluated in three identical uranium solutions (temperature 303 K, pH 5.0, amount of TiO2/Ti 0.025 g, mixing time 60 min, and C0 = 100 mg L–1).
Conclusion
TiO2 NWAs in situ grown on Ti foil were constructed to recover uranium (VI) from aqueous solutions. The uranium (VI) adsorption capacity was affected by solution pH, contact time, temperature, and uranium (VI) concentration. The adsorption was most efficient in the pH range of 5.0 to 9.0. The adsorption process was found to follow pseudo–second-order kinetics and the Langmuir model, and the maximum uranium (VI) adsorption capacity was 354.5 mg g–1. Thermodynamic parameters suggest that the adsorption of uranium (VI) on TiO2/Ti is endothermic and spontaneous. Meanwhile, the TiO2/Ti material showed remarkable reusability, effectively removing 92% uranium (VI) after nine adsorption cycles. In addition, the TiO2/Ti adsorbents can be rapidly retrieved from aqueous solutions by tweezers. The ease of operation, superior adsorption performance, and excellent reusability indicate that the TiO2/Ti adsorbent could be potentially applied to the removal of uranium (VI) from water in diverse practical applications.
Data Availability Statement
The original contributions presented in the study are included in the article/supplementary material, further inquiries can be directed to the corresponding author/s.
Author Contributions
CC contributed to data curation, writing—original draft preparation, and funding acquisition. YZ and XuL contributed to investigation. XiL, JC, LY, ZY, BL, and WT contributed to conceptualization and investigation. ZX contributed to supervision and conceptualization. RG contributed to validation, writing, reviewing, and editing. All authors contributed to the article and approved the submitted version.
Conflict of Interest
The authors declare that the research was conducted in the absence of any commercial or financial relationships that could be construed as a potential conflict of interest.
Acknowledgments
This work was supported by the National Natural Science Foundation of China [Nos. 21703214 and 22006140].
References
Ali, I., Burakov, A. E., Melezhik, A. V., Babkin, A. V., Burakova, I. V., Neskomornaya, E. A., et al. (2019). Removal of Copper(II) and Zinc(II) Ions in Water on a Newly Synthesized Polyhydroquinone/Graphene Nanocomposite Material: Kinetics, Thermodynamics and Mechanism. ChemistrySelect 4, 12708–12718. doi: 10.1002/slct.201902657
Azari, A., Babaie, A. A., Rezaei-Kalantary, R., Esrafili, A., and Kakavandi, B. (2014). Nitrate removal from aqueous solution by carbon nanotubes magnetized with nano zero-valent iron. J. Mazandaran Univ. Med. Sci. 23, 15–27.
Azari, A., Nabizadeh, R., Nasseri, S., Mahvi, A. H., and Mesdaghinia, A. R. (2020). Comprehensive systematic review and meta-analysis of dyes adsorption by carbon-based adsorbent materials: Classification and analysis of lastdecade studies. Chemosphere 250, 126238. doi: 10.1016/j.chemosphere.2020.126238
Azari, A., Noorisepehr, M., Dehghanifard, E., Karimyan, K., Hashemi, S. Y., Kalhori, E. M., et al. (2019). Experimental design, modeling and mechanism of cationic dyes biosorption on to magnetic chitosan-lutaraldehyde composite. Int. J. Biol. Macromol 131, 633–645. doi: 10.1016/j.ijbiomac.2019.03.058
Azari, A., Salari, M., Dehghani, M. H., Alimohammadi, M., Ghaffari, H., Sharafi, K., et al. (2017). Efficiency of Magnitized Graphene Oxide Nanoparticles in Removal of 2,4-Dichlorophenol from Aqueous Solution. J. Mazandaran Univ. Med. Sci. 26, 265–281.
Basheer, A. A. (2018a). Chemical chiral pollution: Impact on the society and science and need of the regulations in the 21stcentury. Chirality 30, 402–406. doi: 10.1002/chir.22808
Basheer, A. A. (2018b). New generation nano-adsorbents for the removal of emerging contaminants in water. Journal of Molecular Liquids 261, 583–593. doi: 10.1016/j.molliq.2018.04.021
Beltrami, D., Cote, G., Mokhtari, H., Courtaud, B., Moyer, B. A., and Chagnes, A. (2014). Recovery of uranium from wet phosphoric acid by solvent extraction processes. Chem. Rev. 114, 12002–12023. doi: 10.1021/cr5001546
Comarmond, M. J., Payne, T. E., Harrison, J. J., Thiruvoth, S., Wong, H. K., Aughterson, R. D., et al. (2011). Uranium Sorption on Various Forms of Titanium Dioxide – Influence of Surface Area, Surface Charge, and Impurities. Environ. Sci. Technol. 45, 5536–5542. doi: 10.1021/es201046x
El-Maghrabi, H. H., Abdelmaged, S. M., Nada, A. A., Zahran, F., El-Wahab, S. A., Yahea, D., et al. (2017). Magnetic graphene based nanocomposite for uranium scavenging. J. Hazard. Mater 322, 370–379. doi: 10.1016/j.jhazmat.2016.10.007
El-sherif, R. M., Lasheen, T. A., and Jebril, E. A. (2017). Fabrication and characterization of CeO2-TiO2-Fe2O3 magnetic nanoparticles for rapid removal of uranium ions from industrial waste solutions. J. Mol. Liq 241, 260–269. doi: 10.1016/j.molliq.2017.05.119
Ho, Y.-S. (2006). Review of second-order models for adsorption systems. J. Hazard. Mater 136, 681–689. doi: 10.1016/j.jhazmat.2005.12.043
Ho, Y. S., and McKay, G. (1999). Pseudo-second order model for sorption processes. Process Biochem 34, 451–465. doi: 10.1016/S0032-9592(98)00112-5
Ho, Y. S., and McKay, G. (2000). The kinetics of sorption of divalent metal ions onto sphagnum moss peat. Water Res 34, 735–742. doi: 10.1016/S0043-1354(99)00232-8
Kamari, A., Ngah, W. S. W., Chong, M. Y., and Cheah, M. L. (2009). Sorption of acid dyes onto GLA and H2SO4 cross-linked chitosan beads. Desalination 249, 1180–1189. doi: 10.1016/j.desal.2009.04.010
Karami, A., Karimyan, K., Davoodi, R., Karimaei, M., Sharafie, K., Rahimi, S., et al. (2017). Application of response surface methodology for statistical analysis, modeling, and optimization of malachite green removal from aqueous solutions by manganese-modified pumice adsorbent. Desalin. Water Treat. 89, 150–161. doi: 10.5004/dwt.2017.21366
Kurttio, P., Harmoinen, A., Saha, H., Salonen, L., Karpas, Z., Komulainen, H., et al. (2006). Kidney Toxicity of Ingested Uranium From Drinking Water. Am. J. Kidney. Dis 47, 972–982. doi: 10.1053/j.ajkd.2006.03.002
Lamb, A. C. M., Grieser, F., and Healy, T. W. (2016). The adsorption of uranium (VI) onto colloidal TiO2. SiO2 and carbon black. Colloid Surf. A-Physicochem. Eng. Asp. 499, 156–162. doi: 10.1016/j.colsurfa.2016.04.003
Langmuir, I. (1918). The Adsorption of Gases on Plane Surfaces of Glass, Mica and Platinum. J. Am. Chem. Soc. 40, 1361–1403. doi: 10.1021/ja02242a004
Li, P., Zhun, B., Wang, X., Liao, P., Wang, G., Wang, L., et al. (2017). Highly Efficient Interception and Precipitation of Uranium(VI) from Aqueous Solution by Iron-Electrocoagulation Combined with Cooperative Chelation by Organic Ligands. Environ. Sci. Technol. 51, 14368–14378. doi: 10.1021/acs.est.7b05288
Liu, X., Pang, H., Liu, X., Li, Q., Zhang, N., Mao, L., et al. (2021). Orderly Porous Covalent Organic Frameworks-based Materials: Superior Adsorbents for Pollutants Removal from Aqueous Solutions. The Innovation 2, 100076. doi: 10.1016/j.xinn.2021.100076
Manigandan, P. K., and Chandar Shekar, B. (2014). Uptake of some radionuclides by woody plants growing in the rainforest of Western Ghats in India. J. Environ. Radioact 130, 63–67. doi: 10.1016/j.jenvrad.2013.12.023
Nair, S., Karimzadeh, L., and Merkel, B. J. (2014). Sorption of uranyl and arsenate on SiO2, Al2O3, TiO2 and FeOOH. Environ. Earth Sci. 72, 3507–3512. doi: 10.1007/s12665-014-3258-x
Peng, L., Ni, Y., Wei, X., Hanyu, W., Duoqiang, P., and Wangsuo, W. (2017). Removal of U(VI) from aqueous solution using TiO2modified β-zeolite. Radiochim. Acta 105, 1005–1013. doi: 10.1515/ract-2017-2765
Prat, O., Vercouter, T., Ansoborlo, E., Fichet, P., Perret, P., Kurttio, P., et al. (2009). Uranium Speciation in Drinking Water from Drilled Wells in Southern Finland and Its Potential Links to Health Effects. Environ. Sci. Technol. 43, 3941–3946. doi: 10.1021/es803658e
Santos, E. A., and Ladeira, A. C. Q. (2011). Recovery of Uranium from Mine Waste by Leaching with Carbonate-Based Reagents. Environ. Sci. Technol. 45, 3591–3597. doi: 10.1021/es2002056
Schnug, E., and Lottermoser, B. G. (2013). Fertilizer-Derived Uranium and its Threat to Human Health. Environ. Sci. Technol. 47, 2433–2434. doi: 10.1021/es4002357
Shi, Z., Liu, C., Zachara, J. M., Wang, Z., and Deng, B. (2009). Inhibition Effect of Secondary Phosphate Mineral Precipitation on Uranium Release from Contaminated Sediments. Environ. Sci. Technol. 43, 8344–8349. doi: 10.1021/es9021359
Song, S., Huang, S., Zhang, R., Chen, Z., Wen, T., Wang, S., et al. (2017). Simultaneous removal of U(VI) and humic acid on defective TiO2−x investigated by batch and spectroscopy techniques. Chem. Eng. J 325, 576–587. doi: 10.1016/j.cej.2017.05.125
Stylo, M., Alessi, D. S., Shao, P. P., Lezama-Pacheco, J. S., Bargar, J. R., and Bernier-Latmani, R. (2013). Biogeochemical Controls on the Product of Microbial U(VI) Reduction. Environ. Sci. Technol. 47, 12351–12358. doi: 10.1021/es402631w
Tan, L., Zhang, X., Liu, Q., Jing, X., Liu, J., Song, D., et al. (2015). Synthesis of Fe3O4@TiO2 core–shell magnetic composites for highly efficient sorption of uranium (VI). Colloid Surf. A-Physicochem. Eng. Asp. 469, 279–286. doi: 10.1016/j.colsurfa.2015.01.040
Tatarchuk, T., Shyichuk, A., Mironyuk, I., and Naushad, M. (2019). A review on removal of uranium(VI) ions using titanium dioxide based sorbents. J. Mol. Liq. 293, 111563. doi: 10.1016/j.molliq.2019.111563
Tripathi, A., Melo, J. S., and D’souza, S. F. (2013). Uranium (VI) recovery from aqueous medium using novel floating macroporous alginate-agarose-magnetite cryobeads. J. Hazard. Mater 246-247, 87–95. doi: 10.1016/j.jhazmat.2012.12.002
Wang, J., He, B., Wei, X., Li, P., Liang, J., Qiang, S., et al. (2019). Sorption of uranyl ions on TiO2: Effects of pH, contact time, ionic strength, temperature and HA. J. Environ. Sci. 75, 115–123. doi: 10.1016/j.jes.2018.03.010
Wang, X., Fan, Q., Yu, S., Chen, Z., Ai, Y., Sun, Y., et al. (2016). RETRACTED: High sorption of U(VI) on graphene oxides studied by batch experimental and theoretical calculations. Chem. Eng. J 287, 448–455. doi: 10.1016/j.cej.2015.11.066
Wen, J., Li, Q., Li, H., Chen, M., Hu, S., and Cheng, H. (2018). Nano-TiO2 Imparts Amidoximated Wool Fibers with Good Antibacterial Activity and Adsorption Capacity for Uranium(VI) Recovery. Ind. Eng. Chem. Res. 57, 1826–1833. doi: 10.1021/acs.iecr.7b04380
Xie, Y., Chen, C., Ren, X., Wang, X., Wang, H., and Wang, X. (2019). Emerging natural and tailored materials for uranium-contaminated water treatment and environmental remediation. Prog. Mater Sci 103, 180–234. doi: 10.1016/j.pmatsci.2019.01.005
Xu, C., Wang, J., Yang, T., Chen, X., Liu, X., and Ding, X. (2015). Adsorption of uranium by amidoximated chitosan-grafted polyacrylonitrile, using response surface methodology. Carbohydr. Polym 121, 79–85. doi: 10.1016/j.carbpol.2014.12.024
Zhang, L., Zhang, L., Wu, T., Jing, X., Li, R., Liu, J., et al. (2015). In situ growth of ZnO nanorod arrays on cotton cloth for the removal of uranium(vi). RSC Adv. 5, 53433–53440. doi: 10.1039/c5ra08489j
Zhong, X., Liang, W., Wang, H., Xue, C., and Hu, B. (2021). Aluminum-based metal-organic frameworks (CAU-1) highly efficient UO22+ and TcO4−ions immobilization from aqueous solution. J. Hazard. Mater 407, 124729. doi: 10.1016/j.jhazmat.2020.124729
Keywords: uranyl, TiO2, nanowire, Ti foil, adsorption, in situ growth, reusability
Citation: Chen C, Zhong Y, Liu X, Li X, Chu J, Yu L, Yang Z, Li B, Tang W, Xiong Z and Gao R (2021) TiO2 Nanowire Arrays in situ Grown on Ti Foil Exhibiting Superior Uranyl-Adsorption Properties. Front. Mater. 8:649998. doi: 10.3389/fmats.2021.649998
Received: 07 January 2021; Accepted: 01 March 2021;
Published: 30 April 2021.
Edited by:
P. Davide Cozzoli, University of Salento, ItalyReviewed by:
Ali Azari, Kashan University of Medical Sciences, IranXiangke Wang, North China Electric Power University, China
Imran Ali, Jamia Millia Islamia, India
Copyright © 2021 Chen, Zhong, Liu, Li, Chu, Yu, Yang, Li, Tang, Xiong and Gao. This is an open-access article distributed under the terms of the Creative Commons Attribution License (CC BY). The use, distribution or reproduction in other forums is permitted, provided the original author(s) and the copyright owner(s) are credited and that the original publication in this journal is cited, in accordance with accepted academic practice. No use, distribution or reproduction is permitted which does not comply with these terms.
*Correspondence: Zhonghua Xiong, eGlvbmd6aG9uZ2h1YUBjYWVwLmNu; Rui Gao, Z2FvcjA0QDEyNi5jb20=