- Beijing Smart-Chip Microelectronics Technology Co., Ltd., Beijing, China
Graphene-MoS2 composites were synthesized via one-step microwave-assisted chemical bath deposition and used as counter electrodes for dye-sensitized solar cells, instead of traditional Pt film which has high cost and poor stability. The effects of graphene additive amount on the performance of cells were studied. The results reveal that sheet porous structure graphene and MoS2 particles has been incorporated tightly, which is the benefit of electrical conductivity and catalysis ability. A maximum efficiency of 6.3% has been achieved under 100 mW cm−2 illumination when the Mo:C is 1:1.
Introduction
Dye-sensitized solar cell (DSSC) has always been a research hotspot in the materials and energy field, which is mainly due to its simple preparation process, low cost, diverse colors, and flexibility (Bajpai et al., 2011). A typical DSSC is composed of three parts, including photoanode, electrolyte, and counter electrode. The photoanode receives photons and emits electrons to the external circuit (Hong et al., 2008). After passing the load, electrons are sent to the electrolyte through counter electrode, then reducing the I3− in electrolyte (Zhu et al., 2017). As a precious metal, Pt is currently the mainstream choice for traditional counter electrode, due to its excellent conductivity and catalytic performance (Ghosh et al., 2020). However, Pt is scarce and expensive, which is not conducive to DSSC’s mass production (Hauch and Georg, 2001). In addition, iodine-based electrolyte and air are also corrosive to Pt, which reduces the life of cells (Olsen et al., 2000). Therefore, it is necessary to find cheap and corrosion-resistant alternative materials for the counter electrode (Sun et al., 2014).
As a two-dimensional carbon material, graphene has been widely used as counter electrode in DSSC research field, with excellent performance due to its electrical conductivity, porous structure, specific surface area, and corrosion resistance (Kavan et al., 2011; Battumur et al., 2012; Liu et al., 2020a; Liu et al., 2020b; Liu et al., 2020c). Roy-Mayhew observed that adjusting the ratio of carbon to oxygen in graphene can improve cell efficiency (Roy-Mayhew et al., 2010). Choi et al. processed graphene at high temperature and used it in DSSC to improve efficiency (Choi et al., 2011). In recent years, the combination of other materials with excellent properties and graphene has become a research hotspot (Peng et al., 2011; Wang et al., 2012). Dou et al. compounded Nil2P5 particles with graphene as counter electrode for DSSC and achieved an efficiency of 5.7% showing improved electrochemical performance (Dou et al., 2011). Wen et al. used TiN with nitrogen-doped graphene composites to improve electrocatalysis (Wen et al., 2011). The composite of graphene and other catalytic materials can not only increase the overall performance of the electrode, but also increase its stability and reduce production costs (Feldt et al., 2013; Zhou et al., 2014).
As a typical layered transition metal sulfide, the molecular structure of MoS2 is similar to that of graphene. MoS2 has been widely used in various fields due to its good electrochemical catalytic performance, including lithium ion batteries, sodium ion batteries, and photocatalytic hydrogen production (Yue et al., 2012; Zhu et al., 2016). In the previous work, we prepared carbon microspheres/MoS2 and used it as counter electrode in DSSC, but the spherical carbon microspheres cannot firmly combine with MoS2 (Zhu et al., 2017). Liu et al. used a physical method to mix MoS2 and graphite oxide and made a composite material through a reduction reaction as counter electrode (Liu et al., 2012). However, the physical mixing method cannot allow two components to grow tightly.
In this work, we use a one-step microwave method to prepare graphene and MoS2 composites, which greatly simplifies the preparation process (Zhou et al., 2020). The two components of the same sheet structure are more closely interacted with a synergetic effect to show an outstanding performance as counter electrode for DSSC.
Experimental
Graphite oxide was prepared by the Hummer method reported in our previous work. 100 ml phosphomolybdic acid solution (20 mM, PH = 6.8) was mixed with a certain amount of graphite oxide (0.16, 0.32, 0.64 g). Then stirred suspension was obtained after 100 ml thioacetamide aqueous solution (70 mM) was added. Subsequently, the above-mentioned precursor solution was heated in a microwave reactor at 160°C with irradiation power of 100 W for 10 min. After centrifugation, washing, and vacuum drying at 120°C for 12 h, the resultant precipitate was heated at 800°C for 2 h under N2/H2 atmosphere. In this work, the obtained composites material was named as MSG-2:1, MSG-1:1, and MSG-1:2 by component ratio of phosphomolybdic acid and graphite oxide.
The as-synthesized composites material or pure MoS2, polyvinylidene fluoride (PVDF) and carbon black (Super-P) were mixed and ground into N-methylpyrrolidone solvent (NMP) at a mass ratio of 8:1:1 to form suspension and then were dropped onto the cleaned F-doped SnO2 (FTO) (resistivity: 14 Ω/□, Nippon Sheet Glass, Japan) glass with 100°C as counter electrodes. The Pt electrodes were also prepared for comparison.
A layer of about 14 μm thickness and 0.2 cm2 TiO2 film was pasted on FTO through the screen printing method as the photoanode. After being sintered at 450°C for 30 min, the photoanode was put into 0.5 mM N719 dye solution for 24 h when it was cooled to 70°C. The dye-treated photoanode and counter electrode were sealed together with a 60 μm spacer (Surlyn) to form a sandwich structure, and electrolyte was injected between the two electrodes. The redox electrolyte contained 0.6 M 1-butyl-3-methylimidazolium iodide (BMII), 30 mM I2, 0.5 M tert-butylpyridine, and 0.1 M guanidinium thiocyanate (GuNCS) in a solvent mixture of 85% acetonitrile with 15% valeronitrile by volume.
The surface morphology of samples was analyzed by field emission scanning electron microscope (FESEM, Hitachi, S4800, Chiyoda-ku, Japan). The structure was characterized by high resolution transmission electron microscope (HRTEM, JeOL-2010) and X-ray diffractometer (XRD, Holland Analytical PRO PW3040/60) using copper target K radiation (V = 30 kV, I = 25 mA, λ = 1.5418 Å). The electrochemical impedance spectra (EIS) and cyclic voltammetry curve (CV) were evaluated by using an electrochemical workstation (Autolab PGSTAT 302N) in a three electrode mode. The photocurrent density-photovoltage curves (J-V) were measured at one Sun (AM 1.5 G, 100 mWcm−2) by the Newport solar simulator system.
Results and Discussion
Figure 1A shows the morphology of pure MoS2. It can be observed from the FESEM that the MoS2 nanoparticles are in flake-shaped structure and aggregated into clusters. The surface morphologies of MSG-2:1, MSG-1:1, and MSG-1:2 are shown in Figures 1B–D, respectively. After being combined with graphene, the agglomeration of the flake-shaped MoS2 nanoparticles is greatly reduced, and MoS2 sheets were closely attached with graphene to form a network structure. According to previous literature, the molybdate ions in precursor solution are easily combined with the oxygen-containing functional groups on graphite oxide, so that MoS2 could grow tightly on the reduced graphene oxide. This compact structure facilitates the transmission of electrons, thereby enhancing the electrical conductivity of the composite (Lee et al., 2010). The FESEM of the three composites is very similar, so it is impossible to distinguish the doping amount of graphene in different samples, which will be discussed in the XRD pattern analysis.
Figure 2 is the HRTEM of MSG-1:1. It can be clearly seen from the figure that MoS2 and graphene are very tightly combined, showing a layered structure. Among them, the lattice fringe with interplanar spacing of 0.62 nm corresponds to the (002) crystal plane of hexagonal MoS2, and the interplanar spacing of 0.34 nm matches the (002) crystal plane of graphene (Wu et al., 2011).
XRD patterns were applied to further analyze the structure of MoS2 and the composites, as shown in Figure 3. Pure MoS2 presents a hexagonal crystal structure, which is consistent with the PDF card (JCPDS card no.73-1508), including (002), (100), (103), (006), (105), (110), and (112) crystal plane. The diffraction peaks of MoS2 appear in all the three composites, indicating that the composite of graphene did not affect the formation of MoS2. From the intensity of the C(002) diffraction peak, it can be seen that the graphene content in MSG-1:2 is the most and decreases in MSG-1:1 and MSG-2:1 sequentially. It indicates that graphene has been successfully incorporated with MoS2, which is consistent with the HRTEM results (Wu et al., 2011).
The cyclic voltammetry curve is used to test the electrochemical performance of each electrode. It can be seen from Figure 4 that the two pairs of redox peaks appearing in all five electrodes indicate that they all have electrochemical catalytic ability for iodine-based electrolyte. Among them, the peak current density of the Pt electrode is the highest and the pure MoS2 electrode is the lowest. When combined with graphene, the current densities of all the three composite electrodes are increased, which indicates that the incorporation of graphene can improve the electrochemical catalytic ability. The electrochemical performance of MSG-1:1 has the most obvious improvement, because the appropriate amount of graphene doping can exert the conductivity of graphene without inhibiting the catalytic activity of MoS2 (Boschloo and Hagfeldt, 2009; Li et al., 2009; Tai et al., 2012; Lin et al., 2013).
EIS can also be used to test the electrochemical performance of counter electrode. The Nyquist spectra of each electrode are shown in Figure 5, and the inset is an equivalent circuit diagram. All the curves have two semicircles in the high frequency and low frequency regions, respectively. The low frequency semicircle corresponds to the reaction at the photoanode/electrolyte interface, and the high frequency semicircle corresponds to the reaction at the counter electrode/electrolyte interface. Corresponding to the equivalent circuit, Rs is the series resistance of the cell. Rw is the charge transfer resistance at the interface between photoanode and electrolyte. Rct is the charge transfer resistance at the interface between counter electrode and electrolyte, which is an important parameter for the performance of counter electrode. Compared with the largest Rct (22.3 Ω) value of MoS2 counter electrode, the Rct of all the three composite electrodes are lower. Among them, the Rct of MSG-1:1 is the lowest (9.8 Ω), even lower than that of Pt counter electrode (10.2 Ω). EIS results demonstrate that the electrochemical performance of composite counter electrode is higher than pure MoS2 counter electrode, and the catalytic ability and conductivity of MSG-1:1 are comparable to Pt (Wang et al., 2011).
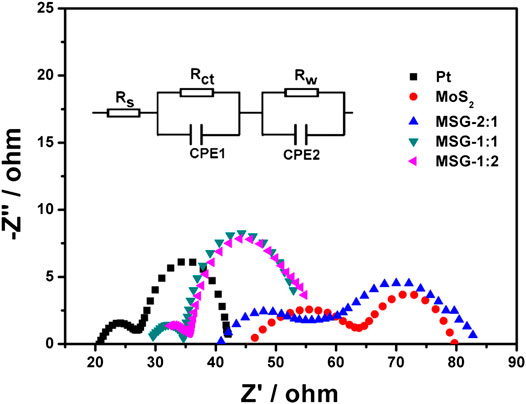
FIGURE 5. EIS of DSSCs with MSG-2:1, MSG-1:1, MSG-1:2, MoS2, and Pt counter electrodes. Inset displays the corresponding equivalent circuit.
The photovoltaic performance is characterized by J-V curve. All the counter electrodes were encapsulated with the photoanode to be prepared as DSSCs and measured under 100 mW cm−2 illumination, as shown in Figure 6. Combining the J-V curves and the current density (Jsc), open circuit voltage (Voc), fill factor (FF), and conversion efficiency (η) in Table 1, it can be found that when graphene is incorporated with MoS2, both FF and η of the cell are higher than that with single component graphene or MoS2 counter electrode. Among the three composite electrodes, MSG-1:1 has the highest FF and η, which are 62.1 and 6.3%, respectively. This is because the composite counter electrode can exploit both conductivity of graphene and catalytic activity of MoS2, and when the ratio of graphite oxide to MoS2 reaches 1:1, the conductivity of graphene and the catalytic activity of MoS2 are optimally fused (Li et al., 2010; Wang et al., 2011). Although the η based on the MSG-1:1 counter electrode is already impressive, it still fails to surpass the Pt counter electrode (7.0%) due to its poor reflectivity of sunlight.
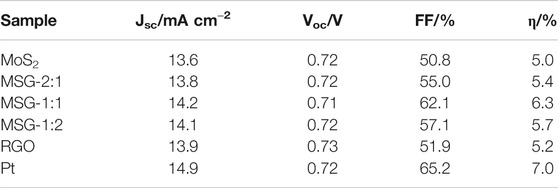
TABLE 1. Photovoltaic parameters of the cells with MSG-2:1, MSG-1:1, MSG-1:2, RGO, MoS2, and Pt counter electrodes.
Conclusion
Graphene and MoS2 with same sheet structure were combined and used as counter electrode in DSSCs. One-step microwave method can greatly simplify the preparation process of the composite electrode, and, through FESEM and HRTEM characterization, it can be found that the materials of the two sheet structures are very tightly combined, which is conducive to the advantages of the two materials. After optimization, MSG-1:1 has the best performance and the efficiency can reach 6.3%.
Data Availability Statement
The original contributions presented in the study are included in the article/Supplementary Material; further inquiries can be directed to the corresponding author.
Author Contributions
LY and HS proposed ideas and wrote the manuscript. Experiment was done by WY, WC, JL, JW, and XK. DL and BM modified the article. YW, SS, and ZW provided financial and technical support.
Conflict of Interest
LY, HS, WY, WC, JL, JW, XK, DL, BM, YW, SS, and ZW was employed by Beijing Smart-Chip Microelectronics Technology Co., Ltd.
References
Bajpai, R., Roy, S., Kumar, P., Bajpai, P., Kulshrestha, N., Rafiee, J., et al. (2011). Graphene supported platinum nanoparticle counter-electrode for enhanced performance of dye-sensitized solar cells. ACS Appl. Mater. Interfaces 3 (10), 3884–3889. doi:10.1021/am200721x
Battumur, T., Mujawar, S. H., Truong, Q. T., Ambade, S. B., Lee, D. S., Lee, W., et al. (2012). Graphene/carbon nanotubes composites as a counter electrode for dye-sensitized solar cells. Curr. Appl. Phys. 12 (Suppl. S1), e49–e53. doi:10.1016/j.cap.2011.04.028
Boschloo, G., and Hagfeldt, A. (2009). Characteristics of the iodide/triiodide redox mediator in dye-sensitized solar cells. Acc. Chem. Res. 42 (11), 1819–1826. doi:10.1021/ar900138m
Choi, H., Kim, H., Hwang, S., Han, Y., and Jeon, M. (2011). Graphene counter electrodes for dye-sensitized solar cells prepared by electrophoretic deposition. J. Mater. Chem. 21 (21), 7548–7551. doi:10.1039/c1jm11145k
Dou, Y. Y., Li, G. R., Song, J., and Gao, X. P. (2011). Nickel phosphide-embedded graphene as counter electrode for dye-sensitized solar cells. Phys. Chem. Chem. Phys. 14 (4), 1339–1342. doi:10.1039/c2cp23775j
Feldt, S. M., Lohse, P. W., Kessler, F., Nazeeruddin, M. K., Grätzel, M., Boschloo, G., et al. (2013). Regeneration and recombination kinetics in cobalt polypyridine based dye-sensitized solar cells, explained using Marcus theory. Phys. Chem. Chem. Phys. 15 (19), 7087–7097. doi:10.1039/c3cp50997d
Ghosh, S., Sartape, R., and Chakraborty, J. (2020). Role of dye-induced corrosion in determining the efficiency of ZnO-based DSSC: the case of ZnO nanoforest in N719. J. Mater. Sci. Mater. Electron. 31 (7), 2202. doi:10.1007/s10854-019-02752-5
Hauch, A., and Georg, A. (2001). Diffusion in the electrolyte and charge-transfer reaction at the platinum electrode in dye-sensitized solar cells. Electrochimica Acta 46 (22), 3457–3466. doi:10.1016/S0013-4686(01)00540-0
Hong, W., Xu, Y., Lu, G., Li, C., and Shi, G. (2008). Transparent graphene/PEDOT-PSS composite films as counter electrodes of dye-sensitized solar cells. Electrochemistry Commun. 10 (10), 1555–1558. doi:10.1016/j.elecom.2008.08.007
Kavan, L., Yum, J. H., and Grätzel, M. (2011). Optically transparent cathode for dye-sensitized solar cells based on graphene nanoplatelets. ACS Nano 5 (1), 165–172. doi:10.1021/nn102353h
Lee, D. H., Kim, J. E., Han, T. H., Hwang, J. W., Jeon, S., Choi, S.-Y., et al. (2010). Versatile carbon hybrid films composed of vertical carbon nanotubes grown on mechanically compliant graphene films. Adv. Mater. 22 (11), 1247–1252. doi:10.1002/adma.200903063
Li, G.-r., Wang, F., Jiang, Q.-w., Gao, X.-p., and Shen, P.-w. (2010). Carbon nanotubes with titanium nitride as a low-cost counter-electrode material for dye-sensitized solar cells. Angew. Chem. Int. Ed. Engl. 49 (21), 3653–3656. doi:10.1002/anie.201000659
Li, P., Wu, J., Lin, J., Huang, M., Huang, Y., and Li, Q. (2009). High-performance and low platinum loading Pt/Carbon black counter electrode for dye-sensitized solar cells. Solar Energy 83 (6), 845–849. doi:10.1016/j.solener.2008.11.012
Lin, J.-Y., Chan, C.-Y., and Chou, S.-W. (2013). Electrophoretic deposition of transparent MoS2-graphene nanosheet composite films as counter electrodes in dye-sensitized solar cells. Chem. Commun. 49 (14), 1440–1442. doi:10.1039/c2cc38658e
Liu, C.-J., Tai, S.-Y., Chou, S.-W., Yu, Y.-C., Chang, K.-D., Wang, S., et al. (2012). Facile synthesis of MoS2/graphene nanocomposite with high catalytic activity toward triiodide reduction in dye-sensitized solar cells. J. Mater. Chem. 22 (39), 21057–21064. doi:10.1039/c2jm33679k
Liu, Y., Gao, X., Wang, K., Dou, X., Zhu, H., Yuan, X., et al. (2020a). Rocking-chair capacitive deionization with flow-through electrodes. J. Mater. Chem. A 8 (17), 8476–8484. doi:10.1039/C9TA14112J
Liu, Y., Gao, X., Wang, Z., Wang, K., Dou, X., Zhu, H., et al. (2020b). Controlled synthesis of bismuth oxychloride-carbon nanofiber hybrid materials as highly efficient electrodes for rocking-chair capacitive deionization. Chem. Eng. J. 403, 126326. doi:10.1016/j.cej.2020.126326
Liu, Y., Zhang, Y., Zhang, Y., Zhang, Q., Gao, X., Dou, X., et al. (2020c). MoC nanoparticle-embedded carbon nanofiber aerogels as flow-through electrodes for highly efficient pseudocapacitive deionization. J. Mater. Chem. A 8 (3), 1443–1450. doi:10.1039/C9TA11537D
Olsen, E., Hagen, G., and Lindquist, S.-E. (2000). Dissolution of platinum in methoxy propionitrile containing LiI/I2. Solar Energy Mater. Solar Cells 63 (3), 267–273. doi:10.1016/S0927-0248(00)00033-7
Peng, S., Wu, Y., Zhu, P., Thavasi, V., Mhaisalkar, S. G., and Ramakrishna, S. (2011). Facile fabrication of polypyrrole/functionalized multiwalled carbon nanotubes composite as counter electrodes in low-cost dye-sensitized solar cells. J. Photochem. Photobiol. A: Chem. 223 (2), 97–102. doi:10.1016/j.jphotochem.2011.08.004
Roy-Mayhew, J. D., Bozym, D. J., Punckt, C., and Aksay, I. A. (2010). Functionalized graphene as a catalytic counter electrode in dye-sensitized solar cells. Acs Nano 4 (10), 6203–6211. doi:10.1021/nn1016428
Sun, H., Pan, L., Piao, X., and Sun, Z. (2014). Enhanced performance of cadmium selenide quantum dot-sensitized solar cells by incorporating long afterglow europium, dysprosium co-doped strontium aluminate phosphors. J. Colloid Interface Sci. 416, 81–85. doi:10.1016/j.jcis.2013.10.050
Tai, S.-Y., Liu, C.-J., Chou, S.-W., Chien, F. S.-S., Lin, J.-Y., and Lin, T.-W. (2012). Few-layer MoS2 nanosheets coated onto multi-walled carbon nanotubes as a low-cost and highly electrocatalytic counter electrode for dye-sensitized solar cells. J. Mater. Chem. 22 (47), 24753–24759. doi:10.1039/c2jm35447k
Wang, G., Xing, W., and Zhuo, S. (2012). The production of polyaniline/graphene hybrids for use as a counter electrode in dye-sensitized solar cells. Electrochimica Acta 66 (4), 151–157. doi:10.1016/j.electacta.2012.01.088
Wang, H.-Y., Wang, F.-M., Wang, Y.-Y., Wan, C.-C., Hwang, B.-J., Santhanam, R., et al. (2011). Electrochemical formation of Pt nanoparticles on multiwalled carbon nanotubes: useful for fabricating electrodes for use in dye-sensitized solar cells. J. Phys. Chem. C 115 (16), 8439–8446. doi:10.1021/jp201220t
Wen, Z., Cui, S., Pu, H., Mao, S., Yu, K., Feng, X., et al. (2011). Metal nitride/graphene nanohybrids: general synthesis and multifunctional titanium nitride/graphene electrocatalyst. Adv. Mater. 23 (45), 5445–5450. doi:10.1002/adma.201102772
Wu, M., Wang, Y., Lin, X., Yu, N., Wang, L., Wang, L., et al. (2011). Economical and effective sulfide catalysts for dye-sensitized solar cells as counter electrodes. Phys. Chem. Chem. Phys. 13 (43), 19298–19301. doi:10.1039/c1cp22819f
Yue, G., Lin, J.-Y., Tai, S.-Y., Xiao, Y., and Wu, J. (2012). A catalytic composite film of MoS2/graphene flake as a counter electrode for Pt-free dye-sensitized solar cells. Electrochimica Acta 85, 162–168. doi:10.1016/j.electacta.2012.08.040
Zhou, N., Lee, B., Timalsina, A., Guo, P., Yu, X., Marks, T. J., et al. (2014). Cross-linkable molecular hole-transporting semiconductor for solid-state dye-sensitized solar cells. J. Phys. Chem. C 118 (30), 16967–16975. doi:10.1021/jp500489f
Zhou, N., Qin, W., Wu, C., and Jia, C. (2020). Graphene-attached vanadium sulfide composite prepared via microwave-assisted hydrothermal method for high performance lithium ion batteries. J. Alloys Compd. 834, 155073. doi:10.1016/j.jallcom.2020.155073
Zhu, G., Wang, H., Xu, H., Zhang, Q., Sun, H., and Zhang, L. (2016). Nitrogen-doped carbon microspheres counter electrodes for dye-sensitized solar cells by microwave assisted method. RSC Adv. 6, 58064–58068. doi:10.1039/C6RA09440F
Keywords: dye-sensitized solar cell, counter electrode, MoS2, graphene, microwave-assisted
Citation: Yi L, Sun H, Yang W, Chen W, Luo J, Wang J, Kang X, Luo D, Ma B, Wang Y, Su S and Wang Z (2021) Study of Microwave-Assisted MoS2 and Graphene Composite Counter Electrode for Dye-Sensitized Solar Cells. Front. Mater. 8:644432. doi: 10.3389/fmats.2021.644432
Received: 21 December 2020; Accepted: 12 January 2021;
Published: 30 March 2021.
Edited by:
Wei Qin, Changsha University of Science and Technology, ChinaCopyright © 2021 Yi, Sun, Yang, Chen, Luo, Wang, Kang, Luo, Ma, Wang, Su and Wang. This is an open-access article distributed under the terms of the Creative Commons Attribution License (CC BY). The use, distribution or reproduction in other forums is permitted, provided the original author(s) and the copyright owner(s) are credited and that the original publication in this journal is cited, in accordance with accepted academic practice. No use, distribution or reproduction is permitted which does not comply with these terms.
*Correspondence: Hengchao Sun, c2RwbHN1bmhjQDEyNi5jb20=