- 1Key Laboratory of Eco-functional Polymer Materials of the Ministry of Education, Key Laboratory of Eco-environmental Polymer Materials of Gansu Province, College of Chemistry and Chemical Engineering, Northwest Normal University, Lanzhou, China
- 2College of Chemistry and Chemical Engineering, Central South University, Changsha, China
With unique advantages, such as high energy density, long lifespan and environmental friendliness, lithium-ion batteries (LIBs) have been widely used in various portable electronics, and placed great expectations on the application in electric vehicles. To meet the ever-increasing high-energy-density demand of the next-generation LIBs, silicon suboxide SiOx(0 < x < 2) has been considered as one of the most promising anode materials, due to its high mass specific capacity, good cycling performance, proper working potential, low cost, and environmental friendliness. However, there are still several drawbacks before the application of SiOx, such as low intrinsic electronic conductivity and high irreversible capacity in the first cycle, which lead to low electrochemical activity and low initial coulombic efficiency (ICE). To tackle these issues, extensive efforts have been made and remarkable progresses have achieved in recent years. Here, latest developments of SiOx-based anodes are briefly reviewed, especially on the subject of metal/metal oxide doping on SiOx-based electrode materials, and the future application of SiOx anodes in rechargeable LIBs is also prospected.
Introduction
With unique advantages of high operating voltage, large energy density and long lifespan, LIBs have been widely used in various portable electronics, and placed great expectations on the application in electric vehicles (Li et al., 2020b; Liu et al., 2021). Graphite, which is relatively cheap, reliable and easy to manufacture into large electrodes by slurry coating process, has been extensively used as an active anode material for commercial LIBs. However, due to its low theoretical capacity of 372 mAh·g−1, graphite cannot meet the increasing actual requirements of the high-quality and fast-paced life for energy density, safety and service life in energy storage and supply devices (Yu et al., 2020a; Yu et al., 2020b; Zhou et al., 2020; Li et al., 2021), it is urgent to develop new anode materials with higher specific capacity (Etacheri et al., 2011; Zhou et al., 2019; Qu et al., 2020). With a theoretical capacity of 4,200 mAh·g−1, Si is expected as an ideal candidate anode material for the next-generation LIBs with high energy density. However, the drastic volume fluctuation (∼300%) and the formation of unstable solid electrolyte interface (SEI) during lithiation/de-lithiation process always result in indisposed cycling efficiencies and capacity retention, which hinders the widespread application of Si-based electrodes (Bruce et al., 2008; An et al., 2019; Weng et al., 2020; Xiang et al., 2020). As a derivative of Si, SiOx (0 < x < 2) has attracted more and more attentions because of its abundant reserves, low cost, easy synthesis, ideal gravimetric capacity and relatively small volume expansion compared with pure Si (Pan et al., 2019).
However, several crucial issues still need to be solved before the practical application of SiOx-based anode materials. Its relatively low conductivity (6.7 × 10−4 S·cm−1) (Kim et al., 2007) and large volume change (∼200%) during cycling always results in poor rate performance and rapid capacity decline, respectively. Moreover, the large initial irreversible capacity of SiOx hinders its practical application (Temkin, 1975). To tackle these issues, significant efforts have been devoted. Composing SiOx with conductive carbonaceous materials, such as carbon layer, graphite and graphene sheets, can largely improve the charge transfer capability (Doh et al., 2008; Ren et al., 2011; Nguyen et al., 2013; Back et al., 2014; Yuan et al., 2015). Recently, researchers focused on creating nanostructures and limiting the size of the active material into the nanoscale range, which not only reduces the diffusion distance of Li+, but also effectively adapts to the accommodation of volume change during cycling, resulting in the improvement of the high-rate and long-term-stability performance (Zhang et al., 2017a). Designing and constructing rational structures of SiOx, such as porous structure, layered structure and so on, plays a very important role in the mitigation of the volume change during cycling and the improvement of the lithium-storage performance (Liu et al., 2009; Lee and Park, 2013; Chen et al., 2020). Up to now, there have been several reviews on SiOx-based anode materials (Chen et al., 2017; Liu et al., 2019; Jiao et al., 2020), which mainly focus on reviewing the compositing SiOx with carbon materials, and the selections and optimizations on electrolytes, additives or binders for the improvements of electrochemical properties for SiOx-based electrodes. However few reviews have been published specifically on the subject of metal/metal oxide doping on SiOx-based electrode materials, which is an easy industrialization of the research direction and of great significance for the development of SiOx-based anode materials. In this review, the recent advances of SiOx anode materials in lithium-storage mechanisms, modifications and electrochemical properties are also summarizes. At the end of this review, a general outlook is given for the application of SiOx-based electrodes in LIBs.
Lithiation Process of Silion Suboxide
SiOx (0 < x < 2) is a kind of amorphous material with relatively complex structure. Among the various silicon suboxide, SiO (x ≈ 1), owning a relatively simple structure, has attracted most attentions as one of the most promising anode material for commercial use in next-generation LIBs. The atomic structure of SiO has been a subject of controversy since its discovery, where two main models were preferred. Philipp proposed a random-bonding model, which described that the Si-Si and Si-O bonds were statistically and randomly distributed throughout a continuous random network of single-phase SiO, hence implying a single-phase material (Philipp, 1971). Temkin suggested a random-mixture model, which assumed that SiO contained mixtures of small domains of Si and SiO2, corresponding to a multi-phase mixture (Temkin, 1975).
Even so, it is very important to understand the lithiation/de-lithiation process of SiOx materials in order to solve the inherent defects of materials in practical application. Considering the complex and indeterminate structure of SiOx, the lithium-storage mechanism of SiOx and the composition of its lithiated products are complex. For instance, the lithium-storage mechanism of SiO can be proposed as follows (Liu et al., 2019):
At the initial stage during lithiation process, Si, lithium silicates and Li2O are formed by the irreversible reaction of SiO with Li. And then, the lithiation/de-lithiation reactions between the formed Si and Li can proceed reversibly. When the formed Si from SiO is fully lithiated to form Li4.4Si, a theoretical reversible capacity of 2,680 mAh·g−1 can be delivered for SiO (Pan et al., 2019). In a similar way, Li2O and Li4SiO4 are produced during the lithiation of nonstoichiometric SiOx, which can act efficiently as volume buffer zones, which is beneficial to the full lithiation and de-lithiation of the formed Si. However, the formed Li2O and Li4SiO4 are both inert phases, which consume the Li irreversibly and further results in low initial coulombic efficiency (Kim et al., 2013).
Improvements in Lithium-Storage Electrochemical Performance of Silion Suboxide-Based Anodes
The low intrinsic conductivity of SiOx, large volume change during the lithiation/de-lithiation process, and the partially irreversible reaction of SiOx with Li are the major stumbling blocks of SiOx-based anodes for practical application. To tackle these issues, extensive efforts have been made. Reducing the particle sizes (Zhang et al., 2017a), constructing porous structures (Yu et al., 2014), combining with conductive carbonaceous materials, and/or composing with other heterogeneous metals or components can be taken to effectively improve lithium-storage electrochemical performance of SiOx-based anodes.
Silion Suboxide/C Composite Materials
To improve electrical conductivity of SiOx-based electrodes, combining SiOx with conductive carbonaceous materials is an effective strategy. Owing to their unique properties of high conductivity, good ductility, readily accessibility, and ability to form stable SEI layers, carbonaceous materials are ideal additives for electrode materials (Choi et al., 2012; McDowell et al., 2013). Guo et al. developed a graphite-like SiOx/C composite, in which the nano-sized SiOx particles uniformly anchored in the carbon matrix (Xu et al., 2018a). Benefiting from the unique structure, when used as anode for LIBs, the obtained graphite-like SiOx/C derived high reversible capacity of 645 mAh·g−1 with a high capacity retention of 90% for 500 cycles. Wu and his co-workers designed a two-step manufacturing process to prepare SiOx-C composite with a hierarchical structure (Wu et al., 2015). After 100 cycles, the SiOx-C anode delivered a reversible specific capacity of 674.8 mAh·g−1 and 83.5% capacity retention at 100 mA·g−1. Direct growth of vertical graphene nanosheets on SiO microparticles can remarkably improve the lithium-storage performance, which showed a high capacity retention up to 93% after 100 cycles (Shi et al., 2017). More recently, an integral interface containing Li polyacrylate (Li-PAA) and carbon nanotubes (CNTs) was constructed on the carbon-coated SiOx by Guo et al. (Li et al., 2020). The flexible Li-PAA protective layer can not only adjust the volume change of SiOx due to its high stretchability (up to 582%), but also provide uniform Li+ transmission interface during charging and discharging. The embedded CNTs can provide fast electronic pathways in the Li-PAA layer, which ensures the superior electronic conductivity. Attributing to the dynamically stable interface (Figure 1), the obtained C-SiOx/C anode showed a remarkably improved cycling ability for 500 cycles, and a high reversible specific capacity of 836 mAh·g−1 can be delivered. The pea-pod structure of SiOx/C composite via combining electrospinning and high-temperature carbonization also showed the enhanced cycling stability and rate performance, attributing to its unique structure with carbon conductive network (Zheng et al., 2020).
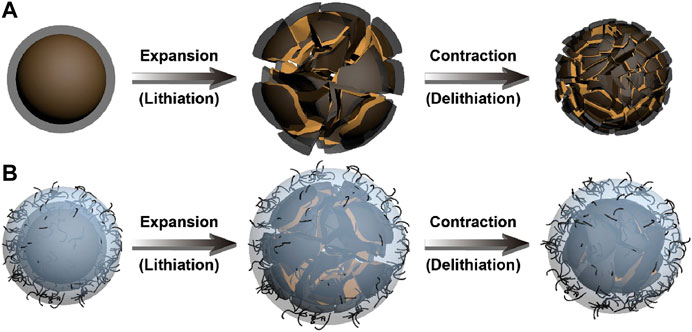
FIGURE 1. Schematic illustration of the lithiation and delithiation of (A) SiOx/C and (B) C-SiOx/C (Li et al., 2020). Reproduced with permission from ref. (Li et al., 2020). Copyright 2020, Elsevier.
Silion Suboxide/Metal or Silion Suboxide/Alloys Composite Materials
On account of favorable electrical conductivity and good flexibility, metals or alloys can be used to improve the lithium-storage electrochemical performance of SiOx anode. The lithium-inactive metal of Fe, Ni or Ti was doped into SiOx, respectively, via a co-deposition technique, which was conducive to the diffusion of Li+ (Miyachi et al., 2007). The obtained Ni-doped SiOx anode delivered a high ICE of 84% and a capacity retention rate of 82% after 400 cycles when matched with an manganese oxide cathode. Similarly, a mixture of SiO, Ni, and reduced graphene oxide was prepared by a hydrothermal mixing and sintering process, and showed an improved cycle performance (Liu et al., 2018). A SiO/Cu/expanded graphite composite was fabricated by a simple electroless plating combined with ultrasonication method, and delivered a markedly improved reversible capacity and cycling stability (Zhang et al., 2017b). In addition, Xu et al. developed a carbon-coated SiO/Cu composites via Cu deposition combined with carbon coating, which also demonstrated a good cycling performance with capacity retention of 88.3% (Xu et al., 2018b). More recently, by conducting a selective alcoholysis method, Kwon et al. synthesized a vanadium-doped SiOx composites, which exhibited an excellent reversible capacity of 1,305 mAh·g−1 at 100 mA·g−1, attributing that the sluggish kinetics of the electrochemical reactions between SiOx and lithium has been accelerated by metal doping (Kwon et al., 2020). The pre-lithiation strategy of SiOx can also greatly improve its ICE (Kim et al., 2016).
The SiO2 phase in SiO can be partially converted into other lithium inactive metal oxides and additional silicon through chemical reduction of SiO2 with elemental metals, which would reduce the irreversible lithiation and improve the reversible specific capacities and ICEs of the electrodes. Based on this, Jeong and his colleagues converted the SiO2 phases in SiO into lithium-inactive alumina and new formed nano-silicon through a mechanochemical process, during which the nanostructured SiAl0.2O material was in situ formed. The new formed silicon nanocrystallites in the matrix enhanced the ICE, while the lithium-inactive alumina improved the cycling performance (Jeong et al., 2010).
Beyond that, the electrochemically active metal can be also added into SiOx for enhancing lithium-storage performance. For example, nanoscale Sn particles were mixed with SiO through a mechanical milling process, and the obtained hybrid delivered a significant improvement of both specific capacity by 50% and ICE from 66.5 to 85.5% (Fu et al., 2019). During the lithiation/de-lithiation process, Sn nanoparticles not only can boost reaction kinetics due to their excellent conductivity, but also play as a reagent revive intermediate Li2O interphase by the reaction of Sn + xLi2O → SnOx + xLi+ + xe−, which benefits to the improvement of the lithium-storage performance. A submicron-sized hybrid SiOx/Sn/C anode was prepared by Li et al., in which the 0D-Sn nanoparticles were reduced in situ in 2D-SiOx inner layer and closely coated by carbon outer layer. With the introduction of Sn, the as-obtained SiOx/Sn/C anode demonstrated a remarkable tap density of 0.74 g·cm−3 and an excellent long-cycle performance of 654 mAh·g−1 at a current density of 1 A·g−1 even after 500 cycles (Li et al., 2019).
In addition, alloys are also used as boosters for the electrochemical properties of SiOx-based anodes. For instance, Abouimrane et al. prepared a SiO-SnxCoyCz composite by high-energy mechanical milling using 50 wt% SiO and 50wt% Sn30Co30C40 as raw materials. The as-obtained hybrid exhibited a remarkable improved specific capacity of 900 mAh·g−1 at 300 mA·g−1 (Liu et al., 2012) . A nanocrystal-FeSi-embedded Si/SiOx anode was synthesized using Fe-Si alloy as the raw material (He et al., 2017). Using the amorphous SiOx as a buffer layer and the self-conductive nanocrystal-FeSi as a robust skeleton, the as-prepared sample showed a reversible capacity of 616.6 mAh·g−1 even at a high current density of 500 mA·g−1. The retractable three-dimensional porous residual Al-doped Si/SiOx composite was also prepared from 6-μm Al-Si alloy particles (Wang et al., 2020), which displayed an attractive application prospects with specific capacity of 899.7 mAh·g−1 even after 300 cycles. Recently, MXene/Si@SiOx@C layer-by-layer superstructure with auto-adjustable function was successfully prepared by magnsiothermic reduction and pyrolytic carbon coating using two-dimensional MXene Ti3C2Tx (Zhang et al., 2019b). The as-obtained nanohybrids provided a reversible specific capacity of 1,674 mAh·g−1 at 0.2 C with an initial coulombic efficiency of 81.3%, and superior stable lithium storage with 76.4% capacity retention even after 1,000 cycles. The superior lithium-storage performance can be attributed to the advantages of mechanical stability by the synergistic effect of SiOx, MXene, and N-doped carbon coating, and excellent structural stability by the forming a strong Ti-N bond among the layers.
Silion Suboxide/Metal Oxides Composite Materials
In recent years, the strategy of combining SiOx with metal oxides has been adopted to improve the electrochemical lithium-storage performance of SiOx-based anodes. Among them, TiO2 is usually considered as one of the best composition of electrode materials due to the high reversibility and conductivity of the lithiated product LixTiO2 (TiO2 + nLi+ + ne− ↔ LinTiO2). Furthermore, the insertion/extraction process of lithium ions in TiO2 layer is very rapid along (001) plane. A nano-scale and thin TiO2 surface coating on SiO has been obtained by a facile sol–gel process (Jeong et al., 2012). According to the unique role of the TiO2 coating, the obtained anode delivered significantly improved specific capacity, coulombic efficiency, rate capability and cycling performance, which are much higher than these of bare SiO electrode. Li et al. embedded ultrafine TiO2 nanocrystals in SiOx particles to form SiOx-TiO2 dual-phase core and then coated them with carbon shells (Li et al., 2018). The initial lithiation process of the as-obtained watermelon-like SiOx-TiO2@C nanoparticle is shown in Figure 2. The incorporation of TiO2 effectively enhanced the lithium ionic and electrical conductivities, and released the structure stress during cycling. As a result, the as-obtained watermelon-like structured SiOx-TiO2@C nanocomposite could exhibit a high capacity of 910 mAh·g−1 for 200 cycles at a current density of 100 mA·g−1. Hu and his co-workers designed a nitrogen plasma-treated core-bishell structure, in which Si nanoparticles were encapsulated in SiOx shell and N-doped TiO2-δ shell (Hu et al., 2019). Both the SiOx shell and N-doped TiO2-δ shell acted as buffer components to adapt the volume change and stabilize the SEI films. In addition, increased oxygen vacancies and Ti3+ species can be obtained after the nitrogen plasma treatment, resulting in the improved diffusion kinetics and conductivity of Li+. After 300 cycles in the half-cell, the Si@SiOx@TiO2−δ electrode showed excellent cycling stability with a reversible specific capacity of 650 mAh·g−1 at 200 mA·g−1.
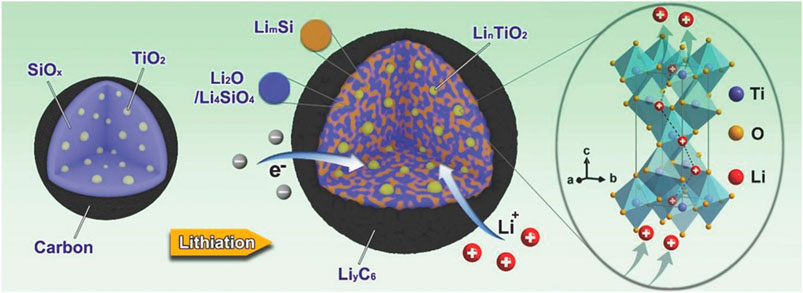
FIGURE 2. Schematic illustration for first lithiation process of watermelon-like structured SiOx-TiO2@C nanoparticle (Li et al., 2018). Reproduced with permission from ref. (Li et al., 2018). Copyright 2018, Wiley Online Library.
Meanwhile, during the charge/discharge processes, corresponding metal nanoparticles can be in situ transformed from the added transition metal oxides, which are helpful to increase the electrical conductivity of the composite materials. Zhou et al. successfully prepared a SiO/Fe2O3 composite via a mechanical grinding process (Zhou et al., 2013). The additional Fe2O3 phase can be lithiated to form metallic Fe during the discharge processes, which can improve the electrical conductivity, resulting to the enhanced reversible specific capacity and ICE from 59 to 68%. An egg-like few-layered graphene-wrapped and Fe3O4-pillared SiOx anodes was established by Liao et al., which delivered a reversible specific capacity of 833.4 mAh·g−1 with a high ICE of 84.9% and capacity retention ratio of 81.8% even after 500 cycles at a current density of 500 mA·g−1 (Liao and Wu, 2019). The excellent performance was benefited from the comprehensive effects of integrated structure, enhanced Li+-diffusion kinetics, and improved pseudocapacitance behavior.
Morover, metal oxides can react with SiOx to form metal silicate compound at high temperature, which can increase the crystallinity of SiOx, resulting in the improvement on the ICEs of SiOx-based anodes. For instance, SiO was coated with a olivine structure Fe2SiO4 layer by heating Fe2O3 and SiO at a molar ratio of 1:0.2, which significantly improved ICE from 70 to 90% (Yamamura et al., 2013). Zhang et al. prepared carbon-coated C-SiO-MgSiO3-Si composites by heating the mixture of SiO, MgO and Si (Zhang et al., 2019a). The in situ formation of the MgSiO3 phase can consume the deleterious SiO2 phase resulting from the disproportionation reaction of SiO at high temperatures, which helps to improve the ICE to 78.3% for lithium storage. Meanwhile, as an electrochemically inert phase, MgSiO3 can also effectively buffer the volume change during cycling, which is beneficial to enhanceing the cycling stability.
Discussion
Because of its high capacity, good cycling stability and appropriate operating voltage, silicon suboxide (SiOx) has been regarded as one of the most promising anode materials for the next-generation LIBs. It is accepted that Li2O and a series of lithium silicates are formed in situ during the initial lithiation of SiOx, which not only insulates the inner active material from electrolyte, but also acts as a buffer for volume expansion. Therefore, SiOx possesses high capacity and excellent cycling performance, but it suffers from unsatisfied initial coulombic efficiency and low intrinsic conductivity, which limit the practical application of SiOx-based materials. In this mini review, the lithium-storage mechanism, modifications and electrochemical properties of SiOx-based anode materials are reviewed briefly. Only by fully understanding the lithiation/de-lithiation mechanisms of materials, can they be targeted to solve their own drawbacks. In addition, this review emphasizes that metal/metal oxide doping into SiOx electrode materials is effective to solve the issues before practical applications. Other strategies for improving electrochemical performance, such as pre-lithium technology through the in-situ formation of silicates, are also covered. Although some strategies have been proposed, large-scale practical applications of SiOx-based anode still have a long way to go. As for future’s research, more consideration should be given to the feasibility and cost of the batch production process, we look forward to seeing a breakthrough of SiOx-based anode materials in the field of LIBs.
Author Contributions
XZ: Conceptualization, Investigation, Formal analysis, Investigation, Validation, Visualization, Writing—original draft. ZQ: Writing—review and editing. QL: Supervision, Project administration. JT: Investigation, Validation. ML: Visualization. KD: Editing and Review. ZL: Review.
Funding
This work is supported by the National Natural Science Foundation of China (Grant numbers 52064045, 51462032), the China Postdoctoral Science Foundation funded project (Grant number 2018M643002), and the Foundation for Distinguished Young Scholars of Gansu Province (Grant number 17JR5RA066).
Conflict of Interest
The authors declare that the research was conducted in the absence of any commercial or financial relationships that could be construed as a potential conflict of interest.
References
An, W., Gao, B., Mei, S., Xiang, B., Fu, J., Wang, L., et al. (2019). Scalable synthesis of ant-nest-like bulk porous silicon for high-performance lithium-ion battery anodes. Nat. Commun. 10 (1), 1447. doi:10.1038/s41467-019-09510-5
Back, C.-K., Kim, T.-J., and Choi, N.-S. (2014). Activated natural porous silicate for a highly promising SiOx nanostructure finely impregnated with carbon nanofibers as a high performance anode material for lithium-ion batteries. J. Mater. Chem. 2 (33), 13648–13654. doi:10.1039/c4ta02706j
Bruce, P. G., Scrosati, B., and Tarascon, J. M. (2008). Nanomaterials for rechargeable lithium batteries. Angew Chem. Int. Ed. Engl. 47 (16), 2930–2946. doi:10.1002/anie.200702505
Chen, T., Wu, J., Zhang, Q., and Su, X. (2017). Recent advancement of SiOx based anodes for lithium-ion batteries. J. Power Sources 363, 126-144. doi:10.1016/j.jpowsour.2017.07.073
Chen, L., Zheng, J., Lin, S., Khan, S., Huang, J., Liu, S., et al. (2020). Synthesis of SiOx/C composite nanosheets as high-rate and stable anode materials for lithium-ion batteries. ACS Appl. Energy Mater. 3 (4), 3562–3568. doi:10.1021/acsaem.0c00084
Choi, I., Lee, M. J., Oh, S. M., and Kim, J. J. (2012). Fading mechanisms of carbon-coated and disproportionated Si/SiOx negative electrode (Si/SiOx/C) in Li-ion secondary batteries: dynamics and component analysis by TEM. Electrochim. Acta. 85, 369–376. doi:10.1016/j.electacta.2012.08.098
Doh, C.-H., Park, C.-W., Shin, H.-M., Kim, D.-H., Chung, Y.-D., Moon, S.-I., et al. (2008). A new SiO/C anode composition for lithium-ion battery. J. Power Sources 179 (1), 367–370. doi:10.1016/j.jpowsour.2007.12.074
Etacheri, V., Marom, R., Elazari, R., Salitra, G., and Aurbach, D. (2011). Challenges in the development of advanced Li-ion batteries: a review. Energy Environ. Sci. 4 (9), 3243–3252. doi:10.1039/c1ee01598b
Fu, R., Wu, Y., Fan, C., Long, Z., Shao, G., and Liu, Z. (2019). Reactivating Li2O with nano-Sn to achieve ultrahigh initial coulombic efficiency SiO anodes for Li-ion batteries. ChemSusChem. 12 (14), 3377–3382. doi:10.1002/cssc.201900541
He, W., Liang, Y., Tian, H., Zhang, S., Meng, Z., and Han, W.-Q. (2017). A facile in situ synthesis of nanocrystal-FeSi-embedded Si/SiOx anode for long-cycle-life lithium ion batteries. Energy Storage Mater. 8, 119–126. doi:10.1016/j.ensm.2017.05.003
Hu, J., Fu, L., Rajagopalan, R., Zhang, Q., Luan, J., Zhang, H., et al. (2019). Nitrogen plasma-treated core-bishell Si@SiOx@TiO2-delta: nanoparticles with significantly improved lithium storage performance. ACS Appl. Mater. Interfaces. 11 (31), 27658–27666. doi:10.1021/acsami.9b04415
Jeong, G., Kim, Y.-U., Krachkovskiy, S. A., and Lee, C. K. (2010). A nanostructured SiAl0.2O anode material for lithium batteries. Chem. Mater. 22, 5570–5579. doi:10.1021/cm101747w
Jeong, G., Kim, J.-H., Kim, Y.-U., and Kim, Y.-J. (2012). Multifunctional TiO2 coating for a SiO anode in Li-ion batteries. J. Mater. Chem. 22 (16), 7999–8004. doi:10.1039/c2jm15677f
Jiao, M., Wang, Y., Ye, C., Wang, C., Zhang, W., and Liang, C. (2020). High-capacity SiOx (0≤x≤2) as promising anode materials for next-generation lithium-ion batteries. J. Alloys Compd. 842, 155774. doi:10.1016/j.jallcom.2020.155774
Kim, T., Park, S., and Oh, S. M. (2007). Solid-state NMR and electrochemical dilatometry study on Li+uptake/extraction mechanism in SiO electrode. J. Electrochem. Soc. 154 (12), A1112–A1117. doi:10.1149/1.2790282
Kim, M. K., Jang, B. Y., Lee, J. S., Kim, J. S., and Nahm, S. (2013). Microstructures and electrochemical performances of nano-sized SiOx (1.18≤x≤1.83) as an anode material for a lithium(Li)-ion battery. J. Power Sources 244, 115–121. doi:10.1016/j.jpowsour.2013.03.041
Kim, H. J., Choi, S., Lee, S. J., Seo, M. W., Lee, J. G., Deniz, E., et al. (2016). Controlled prelithiation of silicon monoxide for high performance lithium-ion rechargeable full cells. Nano Lett. 16 (1), 282–288. doi:10.1021/acs.nanolett.5b03776
Kwon, M.-J., Maniyazagan, M., Yang, H.-W., Kang, W. S., and Kim, S.-J. (2020). A facile synthesis of vanadium-doped SiOx composites for high-performance Li-ion battery anodes. J. Alloys Compd. 842, 155900. doi:10.1016/j.jallcom.2020.155900
Lee, J.-I., and Park, S. (2013). High-performance porous silicon monoxide anodes synthesized via metal-assisted chemical etching. Nanomater. Energy 2 (1), 146–152. doi:10.1016/j.nanoen.2012.08.009
Li, Z., Zhao, H., Lv, P., Zhang, Z., Zhang, Y., Du, Z., et al. (2018). Watermelon-like structured SiOx-TiO2@C nanocomposite as a high-performance lithium-ion battery anode. Adv. Funct. Mater. 28 (31), 1605711. doi:10.1002/adfm.201605711
Li, M., Qiu, J., Ming, H., Zhao, P., Jin, Z., Zhang, S., et al. (2019). A SiOx/Sn/C hybrid anode for lithium-ion batteries with high volumetric capacity and long cyclability. J. Alloys Compd. 809, 151659. doi:10.1016/j.jallcom.2019.151659
Li, G., Huang, L.-B., Yan, M.-Y., Li, J.-Y., Jiang, K.-C., Yin, Y.-X., et al. (2020). An integral interface with dynamically stable evolution on micron-sized SiOx particle anode. Nanomater. Energy 74, 104890. doi:10.1016/j.nanoen.2020.104890
Li, L., Xia, L., Yang, H., Zhan, X., Chen, J., Chen, Z., et al. (2020b). Solid-state synthesis of lanthanum-based oxides Co-coated LiNi0.5Co0.2Mn0.3O2 for advanced lithium ion batteries. J. Alloys Compd. 832, 154959. doi:10.1016/j.jallcom.2020.154959
Li, M., Su, A., Qin, Q., Qin, Y., Dou, A., Zhou, Y., et al. (2021). High-rate capability of columbite CuNb2O6 anode materials for lithium-ion batteries. Mater. Lett. 284, 128915. doi:10.1016/j.matlet.2020.128915
Liao, C., and Wu, S. (2019). Pseudocapacitance behavior on Fe3O4-pillared SiOx microsphere wrapped by graphene as high performance anodes for lithium-ion batteries. Chem. Eng. J. 355, 805–814. doi:10.1016/j.cej.2018.08.141
Liu, W.-R., Yen, Y.-C., Wu, H.-C., Winter, M., and Wu, N.-L. (2009). Nano-porous SiO/carbon composite anode for lithium-ion batteries. J. Appl. Electrochem. 39 (9), 1643–1649. doi:10.1007/s10800-009-9854-x
Liu, B., Abouimrane, A., Ren, Y., Balasubramanian, M., Wang, D., Fang, Z. Z., et al. (2012). New anode material based on SiO–SnxCoyCz for lithium batteries. Chem. Mater. 24 (24), 4653–4661. doi:10.1021/cm3017853
Liu, Y., Huang, J., Zhang, X., Wu, J., Baker, A., Zhang, H., et al. (2018). A bm-SiO/Ni/rGO composite as an anode material for lithium-ion batteries. J. Alloys Compd. 749, 236–243. doi:10.1016/j.jallcom.2018.03.229
Liu, Z., Yu, Q., Zhao, Y., He, R., Xu, M., Feng, S., et al. (2019). Silicon oxides: a promising family of anode materials for lithium-ion batteries. Chem. Soc. Rev. 48 (1), 285–309. doi:10.1039/c8cs00441b
Liu, Z., Li, L., Chen, J., Yang, H., Xia, L., Chen, J., et al. (2021). Effects of chelating agents on electrochemical properties of Na0.9Ni0.45Mn0.55O2 cathode materials. J. Alloys Compd. 855, 157485. doi:10.1016/j.jallcom.2020.157485
McDowell, M. T., Lee, S. W., Nix, W. D., and Cui, Y. (2013). Understanding the lithiation of silicon and other alloying anodes for lithium-ion batteries. Adv. Mater. 25 (36), 4966–4985. doi:10.1002/adma.201301795
Miyachi, M. Y., Yamamoto, H., and Kawai, H. (2007). Electrochemical properties and chemical structures of metal-doped SiO anodes for Li-ion rechargeable batteries. J. Electrochem. Soc. 154 (4), A376–A380. doi:10.1149/1.2455963
Nguyen, D. T., Nguyen, C. C., Kim, J. S., Kim, J. Y., and Song, S. W. (2013). Facile synthesis and high anode performance of carbon fiber-interwoven amorphous nano-SiOx/graphene for rechargeable lithium batteries. ACS Appl. Mater. Interfaces 5 (21), 11234–11239. doi:10.1021/am4034763
Pan, K., Zou, F., Canova, M., Zhu, Y., and Kim, J.-H. (2019). Systematic electrochemical characterizations of Si and SiO anodes for high-capacity Li-Ion batteries. J. Power Sources 413 (8), 20–28. doi:10.1016/j.jpowsour.2018.12.010
Philipp, H. R. (1971). Optical properties of non-crystalline Si, SiO, SiOx and SiO2. J. Phys. Chem. Solid 32, 1935–1945. doi:10.1016/S0022-3697(71)80159-2
Qu, X., Yu, Z., Ruan, D., Dou, A., Su, M., Zhou, Y., et al. (2020). Enhanced electrochemical performance of Ni-rich cathode materials with Li1.3Al0.3Ti1.7(PO4)3 coating. ACS Sustain. Chem. Eng. 8 (15), 5819–5830. doi:10.1021/acssuschemeng.9b05539
Ren, Y., Ding, J., Yuan, N., Jia, S., Qu, M., and Yu, Z. (2011). Preparation and characterization of silicon monoxide/graphite/carbon nanotubes composite as anode for lithium-ion batteries. J. Solid State Electrochem. 16 (4), 1453–1460. doi:10.1007/s10008-011-1525-2
Shi, L., Pang, C., Chen, S., Wang, M., Wang, K., Tan, Z., et al. (2017). Vertical graphene growth on SiO microparticles for stable lithium ion battery anodes. Nano Lett. 17 (6), 3681–3687. doi:10.1021/acs.nanolett.7b00906
Temkin, R. J. (1975). An analysis of the radial distribution function of SiOx. J. Non-cryst. Solids 17, 215–230. doi:10.1016/0022-3093(75)90052-6
Wang, K., Tan, Y., Li, P., and Sun, J. (2020). Scalable 3D porous residual Al-doped Si/SiOx composites for high performance anodes: coupling effects of porosity, conductive sites and oxide layer. Electrochim. Acta 353, 136538. doi:10.1016/j.electacta.2020.136538
Weng, W., Yang, J., Zhou, J., Gu, D., and Xiao, W. (2020). Template-free electrochemical formation of silicon nanotubes from silica. Adv. Sci. 7 (17), 2001492. doi:10.1002/advs.202001492
Wu, W., Shi, J., Liang, Y., Liu, F., Peng, Y., and Yang, H. (2015). A low-cost and advanced SiOx-C composite with hierarchical structure as an anode material for lithium-ion batteries. Phys. Chem. Chem. Phys. 17 (20), 13451–13456. doi:10.1039/c5cp01212k
Xiang, Ben., Wei-Li, An., Fu, Ji-Jiang., Shi-Xiong, Mei., Guo, Si-Guang., Zhang, Xu-Ming., et al. (2020). Graphene-encapsulated blackberry-like porous silicon nanospheres prepared by modest magnesiothermic reduction for high-performance lithium-ion battery anode. Rare Met. doi:10.1007/s12598-020-01528-9
Xu, Q., Sun, J.-K., Yin, Y.-X., and Guo, Y.-G. (2018a). Facile synthesis of blocky SiOx/C with graphite-like structure for high-performance lithium-ion battery anodes. Adv. Funct. Mater. 28 (8), 1705235. doi:10.1002/adfm.201705235
Xu, T., Zhang, J., Yang, C., Luo, H., Xia, B., and Xie, X. (2018b). Facile synthesis of carbon-coated SiO/Cu composite as superior anode for lithium-ion batteries. J. Alloys Compd. 738, 323–330. doi:10.1016/j.jallcom.2017.12.162
Yamamura, H., Nakanishi, S., and Iba, H. (2013). Reduction effect of irreversible capacity on SiO anode material heat-reacted with Fe2O3. J. Power Sources 232, 264–269. doi:10.1016/j.jpowsour.2013.01.038
Yu, B.-C., Hwa, Y., Kim, J.-H., and Sohn, H.-J. (2014). A new approach to synthesis of porous SiOx anode for Li-ion batteries via chemical etching of Si crystallites. Electrochim. Acta. 117, 426–430. doi:10.1016/j.electacta.2013.11.158
Yu, Z., Qu, X., Dou, A., Zhou, Y., Su, M., and Liu, Y. (2020a). Carbon-coated cation-disordered rocksalt-type transition metal oxide composites for high energy Li-ion batteries. Ceram. Int. 47, 1758–1765. doi:10.1016/j.ceramint.2020.09.001
Yu, Z., Qu, X., Wan, T., Dou, A., Zhou, Y., Peng, X., et al. (2020b). Synthesis and mechanism of high structural stability of nickel-rich cathode materials by adjusting Li-excess. ACS Appl. Mater. Interfaces 36, 40393–40403. doi:10.1021/acsami.0c12541
Yuan, X., Xin, H., Qin, X., Li, X., Liu, Y., and Guo, H. (2015). Self-assembly of SiO/Reduced Graphene Oxide composite as high-performance anode materials for Li-ion batteries. Electrochim. Acta 155, 251–256. doi:10.1016/j.electacta.2014.12.124
Zhang, J., Zhang, C., Liu, Z., Zheng, J., Zuo, Y., Xue, C., et al. (2017a). High-performance ball-milled SiOx anodes for lithium ion batteries. J. Power Sources 339, 86–92. doi:10.1016/j.jpowsour.2016.11.044
Zhang, J., Zhang, J., Bao, T., Xie, X., and Xia, B. (2017b). Electrochemical and stress characteristics of SiO/Cu/expanded graphite composite as anodes for lithium ion batteries. J. Power Sources 348, 16–20. doi:10.1016/j.jpowsour.2017.02.076
Zhang, Y., Guo, G., Chen, C., Jiao, Y., Li, T., Chen, X., et al. (2019a). An affordable manufacturing method to boost the initial Coulombic efficiency of disproportionated SiO lithium-ion battery anodes. J. Power Sources 426, 116–123. doi:10.1016/j.jpowsour.2019.04.032
Zhang, Y., Mu, Z., Lai, J., Chao, Y., Yang, Y., Zhou, P., et al. (2019b). MXene/Si@SiOx@C layer-by-layer superstructure with autoadjustable function for superior stable lithium storage. ACS Nano. 13 (2), 2167–2175. doi:10.1021/acsnano.8b08821
Zheng, Y., Kong, X., Usman, I., Xie, X., Liang, S., Cao, G., et al. (2020). Rational design of the pea-pod structure of SiOx/C nanofibers as a high-performance anode for lithium ion batteries. Inorg. Chem. Front. 7 (8), 1762–1769. doi:10.1039/d0qi00069h
Zhou, M., Gordin, M. L., Chen, S., Xu, T., Song, J., Lv, D., et al. (2013). Enhanced performance of SiO/Fe2O3 composite as an anode for rechargeable Li-ion batteries. Electrochem. Commun. 28, 79–82. doi:10.1016/j.elecom.2012.12.013
Zhou, X., Lu, H., Tang, X., Zeng, Y., and Yu, X. (2019). Facile synthesis of Sb@Sb2O3/reduced graphene oxide composite with superior lithium-storage performance. J. Cent. South Univ. 26 (6), 1493–1502. doi:10.1007/s11771-019-4105-8
Keywords: silion suboxide, anode, composite, electrochemical performance, lithium-ion batteries
Citation: Zhou X, Qi Z, Liu Q, Tian J, Liu M, Dong K and Lei Z (2021) Research Progress of Silicon Suboxide-Based Anodes for Lithium-Ion Batteries. Front. Mater. 7:628233. doi: 10.3389/fmats.2020.628233
Received: 11 November 2020; Accepted: 18 December 2020;
Published: 22 January 2021.
Edited by:
Yunjian Liu, Jiangsu University, ChinaReviewed by:
Ling Wu, Soochow University, ChinaHailong Wang, Ningxia University, China
Lingjun Li, Changsha University of Science and Technology, China
Biao Gao, Wuhan University of Science and Technology, China
Copyright © 2021 Zhou, Qi, Liu, Tian, Liu, Dong and Lei. This is an open-access article distributed under the terms of the Creative Commons Attribution License (CC BY). The use, distribution or reproduction in other forums is permitted, provided the original author(s) and the copyright owner(s) are credited and that the original publication in this journal is cited, in accordance with accepted academic practice. No use, distribution or reproduction is permitted which does not comply with these terms.
*Correspondence: Xiaozhong Zhou, enh6MjAwMDRAMTYzLmNvbQ==