- 1Department of Civil, Environmental, and Architectural Engineering, University of Colorado Boulder, Boulder, CO, United States
- 2Materials Science and Engineering Program, University of Colorado Boulder, Boulder, CO, United States
Climate change, infrastructure resilience, and resource recovery from waste have emerged as grand challenges for civil engineers in the 21st century. Wicked problems associated with these global grand challenges are necessitating innovative, multidisciplinary thinking and multiscale, integrated solutions that are spurring the development of a new field—construction biotechnology. While the field of construction biotechnology spans multiple scales, this review highlights the promise and potential of nanoscale (<100 nm) biotechnological applications to civil engineering. While the field of nanotechnology has revolutionized other industries, applications of nanotechnology in civil engineering have remained limited due to techno-economic and environmental barriers. Biological production of functional nanoparticles (NPs), however, offers new economical routes to develop resilient, high-performance cementitious materials while simultaneously addressing critical needs related to wastewater treatment and resource recovery. Recent research has elucidated that biological production of NPs exhibit preferred—and genetically controllable—morphological characteristics that could tailor the structure-property relationships of civil engineering materials. The natural ability of microorganisms to immobilize heavy metals (eg, Hg, Cr, Zn, Cd, Cu, Ag)—and the programmability of microorganisms to do so via synthetic biology—as well as their ability to sequester greenhouse gases and neutralize volatile organic compounds affords civil engineers a grand opportunity to treat wastewater, recover rare earth elements, and minimize air pollution. In addition to featuring state-of-the-art research in the field, this review summarizes the opportunities and challenges of nanoscale biotechnology and proposes a roadmap of research for civil engineers of the 21st century.
1 Introduction
Among the grand societal challenges of our time, climate change, infrastructure resilience, and resource recovery from waste are of critical importance (DeJong et al., 2011). Given the ubiquity of concrete and its projected increase in utilization over the next few decades, the development of resilient and sustainable cementitious materials will be a key solution to these challenges. Resilience of cementitious materials refers to high performance (e.g., strength) and other capabilities (e.g., self-healing) that offer great resistance to—and recovery from—disasters. While sustainability has commonly been investigated in terms of reduced emissions associated with the manufacturing process, it could also refer to imparting additional functionalities with in situ environmental benefits (e.g., heavy metal immobilization).
To develop high-performance cementitious materials, nanotechnology has proven to be a highly effective tool. With addition of a variety of nanoparticles (e.g., SiO2, ZrO2, TiO2, CuO), the setting time and diffusivity of concrete are reduced, while the strength and high-temperature stability are increased (Sobolev et al., 2006; Rashad, 2013a, Rashad, 2013b; Reches, 2018). While compressive strength is the most critical performance criteria for cementitious materials, decreased diffusivity prevents/reduces the ingress of water and aggressive ions (e.g., sulfate and chloride) and, thus, greatly enhances concrete durability (Mindess, 2019).
Enhanced performance of concrete via nanotechnology minimizes needs for repair or replacement, thus reducing economic and environmental cost. However, the manufacturing of nanoparticles is quite energy-intensive (Colvin, 2003; Jayapalan et al., 2013). For example, the manufacture one ton of TiO2 NPs consumes ∼32–40 GJ during a conventional procedure (Osterwalder et al., 2006). In addition to high energy consumption, the use of toxic chemicals, such as non-polar solvents used during synthesis or chemicals used to functionalize NP surfaces, are safety concerns for environmental and human health (Li et al., 2011; Tiquia-Arashiro and Rodrigues, 2016). Such barriers have somewhat limited the applications of nanoparticles in cementitious materials.
Biological approaches offer an alternative to the conventional synthesis of nanoparticles. During biosynthesis, microorganisms (e.g., fungi and bacteria) take target metal ions from the environment and convert them into metal or metal oxide NPs (called bio-NPs hereafter) (Li et al., 2011; Hulkoti and Taranath, 2014; Tiquia-Arashiro and Rodrigues, 2016). Such processes eliminate or reduce the use of expensive and potentially toxic chemicals, a benefit that has significantly promoted research in the field of NP biosynthesis (Li et al., 2011; Faramarzi and Sadighi, 2013). Biological synthesis of NPs also eliminates high energy-consuming processes (e.g., grinding of ore, reacting with strong acid, and treating with steam). A bio-based approach is even more favorable considering the potential to carry out such biosynthesis under environments of varying temperature, pH and pressure, salt concentration, acidity and alkalinity, either by using extremophiles (i.e., microorganisms that survive under extreme environmental conditions) or through genetic modification (Stabnikov et al., 2015; Beeler and Singh, 2016; Tiquia-Arashiro and Rodrigues, 2016). As specific examples, genetic modification has been shown to control E. coli's capability for producing CaCO3 of tailored morphology and mechanical properties (Liang et al., 2018; Heveran et al., 2019) and its tolerance to harsh environment (e.g., high NaCl concentration) (Eslami et al., 2016).
While bio-based nanotechnologies have been applied to a number of fields, such as nanomedicine, environmental remediation, and energy generation (Li et al., 2011; Tiquia-Arashiro and Rodrigues, 2016), their applications in the field of construction is relatively new. Although other biotechnologies have been applied in construction (Pacheco-Torgal and Labrincha, 2013; Ivanov et al., 2015; Stabnikov et al., 2015), these technology’s nanoscale aspects, a scale that exhibits great potential for future civil engineering materials (Pacheco-Torgal and Jalali, 2011), has not been reviewed in detail.
This work summarizes for the first time the progress, promise, and potential of a new field—nanoscale construction biotechnology. First, we review nanoscale construction technology and biotechnology and identify links that bridge gaps between the two. Specifically, we focus on improving the performance of cementitious materials and the synergistic benefits related to environmental remediation capability. We begin with a current summary of NPs used to enhance engineering performance of cementitious materials, then examine the feasibility of bio-NP production (mainly via bacteria) for the same purpose and summarize possible approaches for such applications. Next, we focus on environmental remediation by cementitious materials or microorganisms alone, followed by a review of several ways to apply microorganisms (mainly bacteria, occasionally fungi and algae) to cementitious materials for enhanced remediation capability. Finally, the significance and future development of the field of nanoscale construction biotechnology are discussed in light of opportunities and challenges that civil engineers will face in the 21st century.
2 Engineering Performance
2.1 Nanotechnology in Cementitious Materials
Because of the ubiquity of cement paste and concrete, the study of the nanostructure of cementitious materials has attracted great interest. Among multiple phases of hydration products (eg, ettringite, monosulfate and calcium hydroxide) that are formed during portland cement hydration, calcium silicate hydrate (C-S-H) is the main phase in hydrated portland cements. A typical nanoscale TEM image of C-S-H clusters and its molecular structure are shown in Figure 1 (Pellenq et al., 2009). The C-S-H structure consists of calcium (green and gray spheres in Figure 1) layers sandwiched with chains composed of Si-O tetrahedra (yellow and red spheres), in addition to water molecules (blue and white spheres).
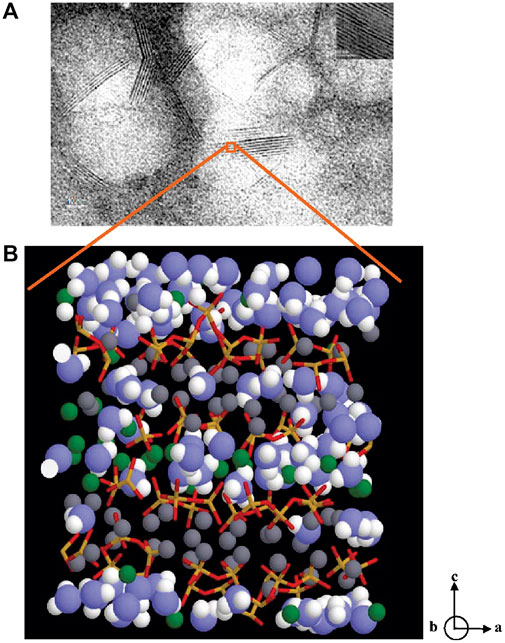
FIGURE 1. (A) TEM image of clusters of C-S-H, the inset is a TEM image of tobermorite (B) the molecular model of C-S-H: the blue and white spheres are oxygen and hydrogen atoms of water molecules, respectively; the green and gray spheres are inter and intra-layer calcium ions, respectively; yellow and red sticks are silicon and oxygen atoms in silica tetrahedra (Pellenq et al., 2009).
Nanoparticles (NPs, <100 nm) are highly efficient at tailoring the properties of cementitious materials at both early and late ages, even at low concentrations (<1%) (Reches, 2018; Mohajerani et al., 2019). In general, these effects are attributed to three mechanisms: 1) increased nucleation sites due to the NPs’ high surface area that facilitates formation of C-S-H; 2) formation of C-S-H via pozzolanic reaction of some silica-containing nanoparticles; and 3) densification of cementitious materials via the filler effect (Reches, 2018).
Increased nucleation sites substantially accelerate the setting time of C3S, the primary mineral in portland cement. The seminal work by Thomas (Thomas et al., 2009) demonstrated that C-S-H NP acts as nuclei for further growth of C-S-H, a change that eliminates the induction period of the cement hydration and substantially increases the total heat of hydration in the first 24 h. In addition to product-based seeds (i.e., C-S-H), many different types of NPs also exhibit accelerating effects on the setting of cementitious pastes (Figure 2) (Reches, 2018). Such nucleation promotes precipitation of C-S-H/C-A-S-H in alkali-activated fly ash/slag binders (Puligilla et al., 2019). This finding is in line with the concept of heterogeneous nucleation, in which the energy barrier is reduced, due to the presence of external surfaces that facilitate the reaction to proceed. Besides affecting the reaction kinetics, nucleation effects can also tailor the microstructures (Thomas et al., 2009; Puligilla et al., 2018; Puligilla et al., 2019). For example, the promoted nucleation of the C-S-H products in between the cement particles homogenizes the microstructure.
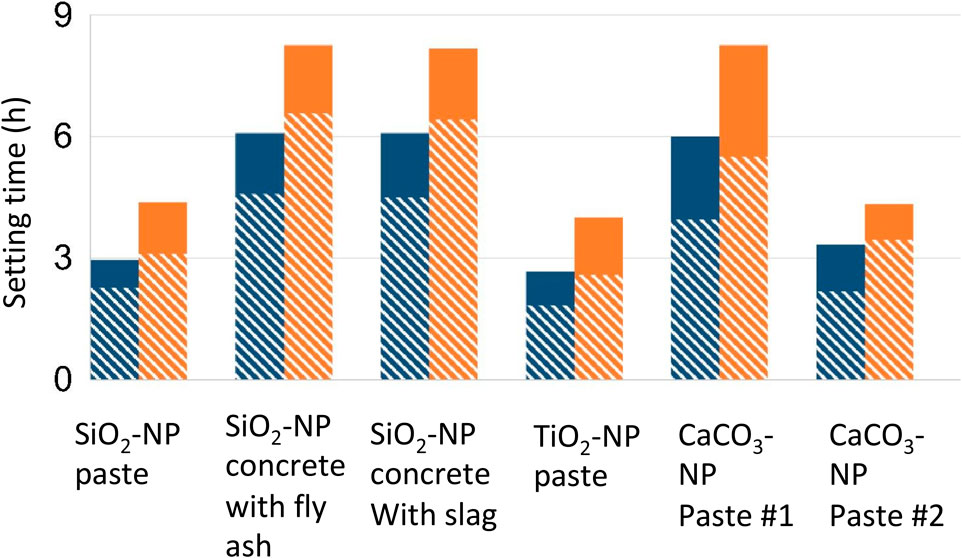
FIGURE 2. Initial (blue color) and final (yellow color) setting of cement products prepared with or without NPs in different cementitious mixtures, adapted from Reches, (2018). While solid represent control mixtures, the line-filled part represents seeded mixtures.
Consistent with the effects on setting time, many types of NPs, such as SiO2, TiO2, Fe2O3 and ZrO2, increase the strength of common cementitious materials (Table 1) (Khoshakhlagh et al., 2012; Li et al., 2017; Afzali Naniz and Mazloom, 2018; Trejo-Arroyo et al., 2019) and macroporous pervious concrete (Barnhouse and Srubar, 2016). The most substantial effects of NPs on strength occur at early ages (i.e., 1–7 days) (Reches, 2018). The effect is also pronounced when relatively low-reactive mineral admixtures (e.g., fly ash, rice husk ash, and other supplementary cementitious materials (SCMs)) are introduced as partial replacement for portland cement. While these mineral admixtures are a key solution to concrete’s sustainability (Juenger et al., 2019), the addition of NPs overcomes the slow reaction by accelerating the reaction kinetics.
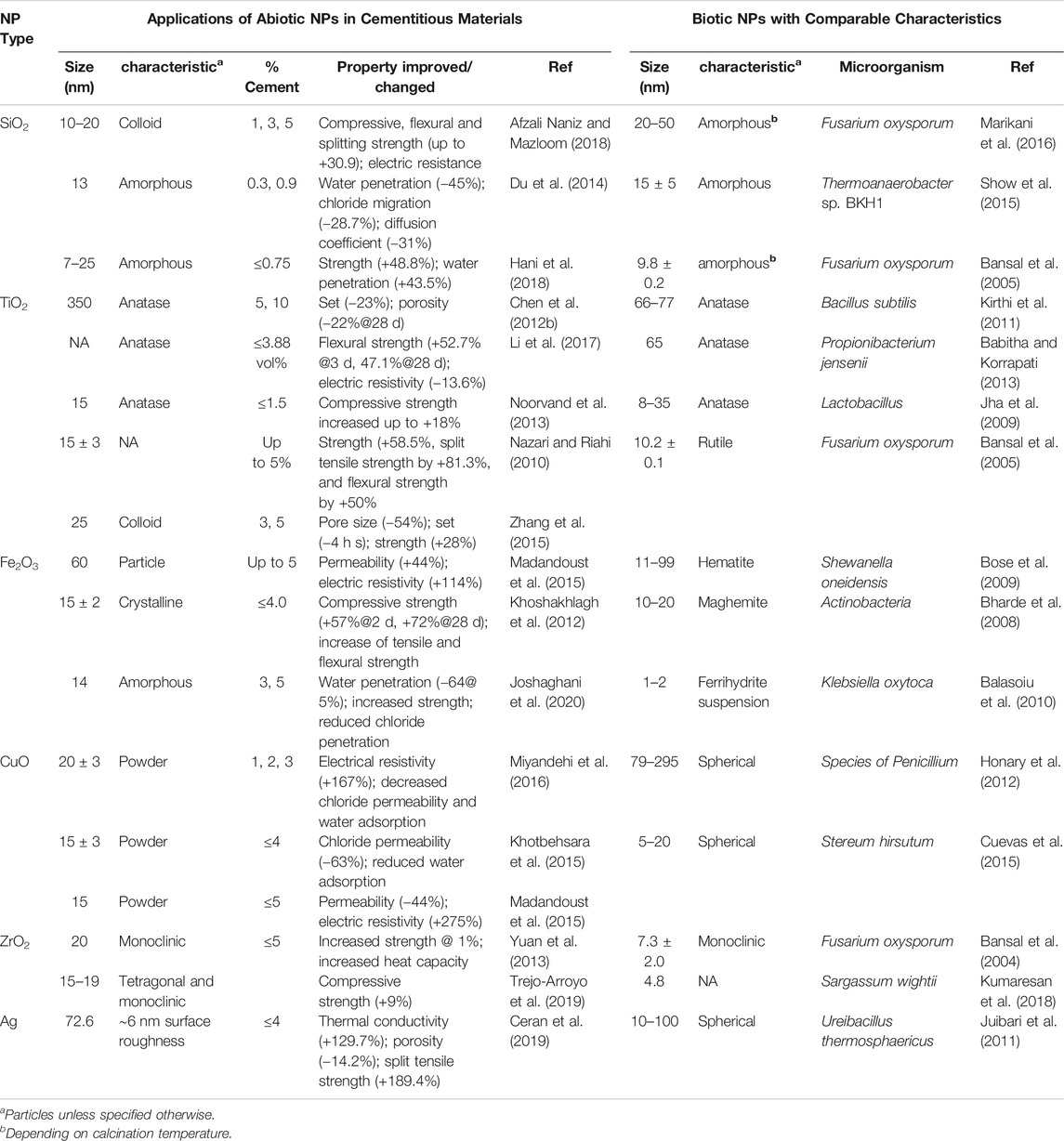
TABLE 1. Example applications of abiotic NPs in cementitious materials and biotic NPs with similar characteristics.
The permeability of concrete is a key parameter that affects long-term durability. Permeability affects carbonation, sulfate attack, chloride intrusion, and alkali-aggregate reactions (i.e., alkali-silica reaction and alkali-carbonate reaction), among other degradation mechanisms (Mindess, 2019). NPs have shown to decrease the diffusivity to gases in dry conditions and ions in water-saturated conditions (Reches, 2018), likely due to the beneficial nucleation and densification effects previously discussed. The effect of NPs on permeability can be substantial. Depending on the mixture proportions, up to 75% decrease in permeability has been observed with addition of ∼1 wt% NPs (Reches, 2018). Relevantly, the reduced permeability (or porosity) is associated with concomitant increase in electrical resistivity and resistance to penetration of chloride and water (see Table 1).
2.2 Applications of Bio-NPs in Cementitious Materials
Abiotic NPs are fabricated via conventional (mainly physical and chemical) techniques. Although these techniques can produce large quantities of NPs in a short time with well-defined characteristics (e.g., size and shape), their procedures are complicated and cost/energy consuming (Li et al., 2011). These conventional techniques usually employ atomistic and molecular processing in an aqueous condition and commonly use chemicals that are considered toxic to the environment and, especially, human health (Li et al., 2011; Tiquia-Arashiro and Rodrigues, 2016).
Microorganism-based synthesis can serve as a non-toxic and environment-friendly alternative to NP production. A wide range of fungi and bacteria have been exploited for such synthesis (Li et al., 2011; Tiquia-Arashiro and Rodrigues, 2016). During synthesis, the microorganisms either uptake ions intracellularly or adsorb them onto surface structures, thereby resulting in passive NP precipitation or active conversion by enzymes. These processes, in lieu of expensive and oftentimes toxic chemicals, enable an environmentally friendly synthesis route. As a result, many researchers in the field of NP synthesis have turned to bio-synthesis (Li et al., 2011; Faramarzi and Sadighi, 2013). This bio-based approach is even more favorable, considering the potential to genetically engineer microorganisms for desirable characteristics, for example, engineering genetic control over NP morphology or harnessing extremophiles (Tiquia-Arashiro and Rodrigues, 2016) capable of biosynthesis and persistence under the extreme environments that may occur during and after construction.
2.2.1 Synthesis Mechanisms of Bio-NPs
While biosynthesis of NPs involve multiple complex processes, it typically starts by trapping the metal ions to the surface or inside of the cells, followed by conversion of these ions into NPs (Li et al., 2011). During the conversion process, microorganisms act as biocatalysts and alter the ionic concentration of the surrounding environment in a very localized manner, thus supersaturating the solution and facilitating precipitation. Alternatively, the conversion of metal ions can take place either through interaction with intracellular or extracellular proteins and organic molecules or by interacting with the cell wall components, as illustrated in Figure 3 with bacteria as an example (Fang et al., 2019). As an example, during the biosynthesis of Ag NPs by Cladosporium cladosporioides fungi, Ag ions are adsorbed on the cell surface and subsequently converted to silver nuclei through an enzyme-induced reduction reaction. These nuclei grow through further reduction and accumulation (Balaji et al., 2009). More fundamentally, this conversion process is related to the electron shuttling (or redox mediating) enzymes, for example, nitrate reductase (Kalishwaralal et al., 2008).
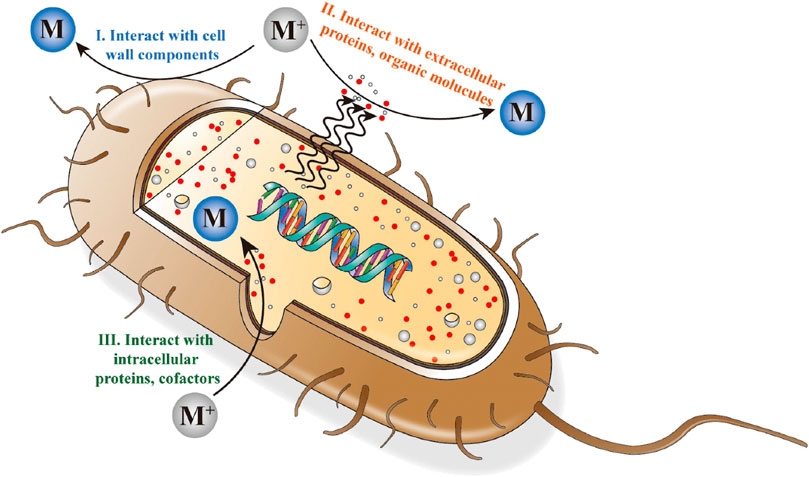
FIGURE 3. Schematic illustration of bacterial synthesis of NPs (intracellular and extracellular procedures) (Fang et al., 2019).
Similar procedures (i.e., entrapment followed by conversion) are seen in synthesis of metal oxide NPs (Li et al., 2011). In the synthesis of magnetic iron oxide particles by magnetotactic bacteria, including magnetite (Fe3O4) and maghemite (γ-Fe2O3), vesicles are first formed and serve as a scaffold for proteins involved in the production of the NPs. These vesicles then assemble into a line along with cytoskeletal filaments, and Fe ions accumulate in the vesicles. This accumulation is aided by membrane transporters (e.g., proteins and siderophores). In the last step, relevant proteins trigger and regulate the nucleation and growth process of the iron oxide NPs. Throughout this process, various proteins contribute to supersaturation of Fe and maintain conditions for reduction and oxidation reactions that induce NP production (Arakaki et al., 2008). An alternative process was observed for synthesis of magnetite with Shewanella oneidensis (Perez-Gonzalez et al., 2010). This mechanism initially involves active production of Fe2+ by using ferrihydrite as a terminal electron acceptor. Then, a passive process takes place, during which Fe2+ and Fe3+ accumulate at the negatively charged cell wall and extracellular structures leading to supersaturation and precipitation of NPs.
2.2.2 Direct Addition of Bio-NPs to Cementitious Materials
Bio-NPs exhibit great potential for applications in cementitious materials. As mentioned in section 2.1, NPs improve the performance of cementitious materials mainly by enhancing nucleation of the C-S-H product and by acting as a filler to densify the microstructures. Both mechanisms relate to the small particle sizes and large surface area of NPs. It follows that bio-NPs of sufficiently small size and large surface area would improve the performance of cementitious materials in a similar fashion.
In Table 1, we summarize biogenic NPs reported in literature that exhibit similar sizes and characteristics to conventional NPs added to cementitious materials to enhance engineering performance. SiO2 NP additives used in cementitious materials exhibit amorphous features with average sizes of 7–25 nm (Du et al., 2014; Hani et al., 2018; Afzali Naniz and Mazloom, 2018). Similarly, SiO2 particles produced via Fusarium oxysporum and Thermoanaerobacter sp. BKH1 bacteria exhibit amorphous features and sizes from 9.8 to 50 nm (Bansal et al., 2005; Show et al., 2015; Marikani et al., 2016). These nanostructural characteristics indicate feasibility of these bio-NPs to serve as additives to improve performance of cementitious materials, unlike the micro-sized particles that exhibit less pronounced effects (Sato and Diallo, 2010). Other common additives to cementitious materials (e.g., TiO2, Fe2O3, CuO, ZrO2 ang Ag) can also be produced with a variety of microorganisms in comparable sizes and characteristics.
Additionally, the size and morphology of the bio-NPs can be controlled by tuning the biosynthesis parameters. During the Corynebacterium glutamicum-mediated synthesis, the particle sizes of Ag NPs could be tuned by varying the media parameters including pH, temperature and AgNO3 concentration (Sneha et al., 2010). Besides the media-related parameters, biological factors also control the synthesis of bio-NPs. For example, The CuO NPs synthesized from Penicillium citrium, Penicillium waksmanii and Penicillium aurantiogriseum under the same pH and temperature conditions exhibit different values of polydispersity index (PDI) and average particles, indicating that strain selection has a significant impact on NP morphology (Honary et al., 2012).
Bio-NPs need to be purified after production to avoid any complications to hydration of cementitious materials (Basaran Bundur et al., 2015). Purification typically requires specialized equipment. For example, Ag NPs synthesized from Escherichia coli are preliminarily centrifuged and washed several times and then transferred to a dialysis tube to remove high molecular weight molecules, followed by an ultracentrifugation (200,000 rpm at 4°C for 16 h) (Gurunathan et al., 2009). In another example, the biosynthesized Au NPs from Bacillus cereus or Fusarium oxysporum were purified three times by centrifugation (14,700 rpm, 30 min) using double-distilled water. For comparison, to purify chemically synthesized NPs (again by taking Ag as an example), while centrifugation can be carried out (Guzman et al., 2009), simple filtration and washing can also be conducted (Sun et al., 2003), given that the purification process is relatively straightforward in most chemical synthesis processes (Tan and Cheong, 2013).
In summary, the bio-NPs, upon purification, exhibit great potential to be directly used in cementitious materials, because their size and characteristics are comparable to abiotic counterparts that have been used successfully to enhance the performance of cementitious materials. Additionally, the size and morphology of bio-NPs can be tuned by optimizing the biosynthesis conditions, which offers new pathways to optimizing biosynthesis to produce bio-NPs with the most desirable characteristics.
2.2.3 Addition of Bio-NPs via Surface Treatment of Aggregates
In addition to the direct addition of purified bio-NPs to cementitious materials during mixing, bio-NPs could potentially be precipitated/coated on the surface of aggregates before mixing with cements. A similar strategy has been proposed by Wong (Wong, 2015). Specifically, soil/sand, aggregates (Wang et al., 2017) or some solid industrial wastes (Achal et al., 2013; Cuzman et al., 2015) can be biomineralized and subsequently used as fillers and aggregates in concrete. Besides densifying the aggregates/fillers themselves, such biomineralization benefits the performance for the final concrete materials, especially when the resulting precipitation 1) exhibits preferred nanostructural morphology and 2) occurs on surfaces of fillers/aggregates. The treated fillers/aggregates, when possessing nanostructural morphology, would enhance the engineering performance similar to direct addition. Additionally, the presence of the NPs at the interface between aggregates and cement matrix (i.e., interfacial transition zone or ITZ) promotes localized nucleation of hydration products. Since the ITZ is usually the weakest zone in concrete (Mindess et al., 2003), the application of NP-modified aggregate offers great potential benefits to enhancing engineering performance.
Currently, microorganism-based precipitation on the surface of cementitious materials and aggregates has been studied mainly via calcium carbonate (CaCO3) biomineralization (Seifan et al., 2016). Biomineralization by living organisms has also been explored for biosynthesis of NPs (Achal et al., 2015; Salehizadeh et al., 2020), though it, unlike many other studies related to the biosynthesis of NPs, can also produce larger-sized minerals or structural features, including shells and bones. During biomineralization, metal ions are precipitated with ligands (e.g., sulfide, phosphate or organic functional groups) or with carbonates/hydroxides (Newsome et al., 2014; Bhattacharyya et al., 2019).
As a typical type of biomineralization, microbially induced carbonate precipitation (MICP) produces calcium carbonates as NPs that exhibit nanostructural morphology at initial stages of precipitation (Zhang, 2016). This observation suggests that with well-controlled environmental conditions the MICP-treated aggregates exhibit nanostructural feature that could promote the hydration upon mixing with cement pastes. Similar nanostructural morphology is also seen in other types of extracellular biogenic NPs, for example, on bacterial cell walls (Figure 4) (Selvakumar et al., 2014) or in biofilms (i.e., a group of microorganisms in which cells stick to each other and also to a surface) (Kalathil et al., 2011).
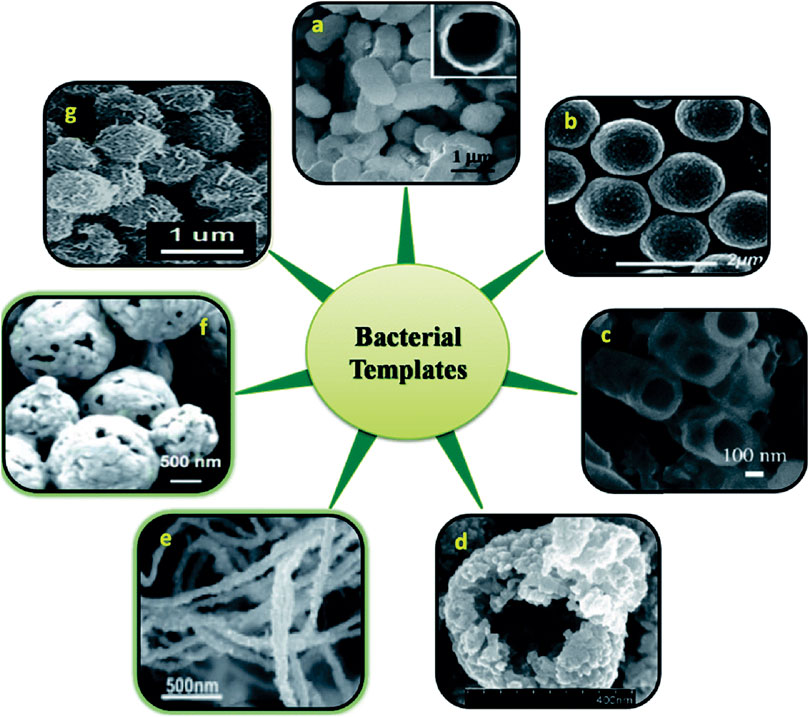
FIGURE 4. Various nanostructures derived using the entire cell of bacteria via extracellular biomineralization. (A) FESEM images of E. coli templated hollow silica microparticles (insert: cross sectional view of calcined particle); (B)S. thermophilus/ZnO core–shell spheres; (C) SEM images of crushed calcined zirconia particles using E. coli template; (D) Co3O4-decorated ZnO hollow spheres; (E) Au nanostructures synthesized by the reduction of aqueous HAuCl4; (F) SEM image of H2O2 etched Ag microspheres at a higher magnification using S. thermophilus template; (G) FE-SEM image of uniform, bacteria-supported, hierarchical Co3O4 superstructures produced without changing the spherical shape of the original micrococcus template (Selvakumar et al., 2014).
MICP-produced CaCO3 have been applied in infrastructure usually through binding with cementitious materials. CaCO3 precipitation approaches have been adopted to fill or heal the external cracks to enhance durability (De Muynck et al., 2010). For example, a CaCO3-based bio-cementitious crust on the surface of sand has been developed that exhibits a flexural strength of 35.9 MPa and high water impermeability (Stabnikov et al., 2011). Besides surface treatment, such bio-based precipitation has been reported to form bulk bio-blocks, such as reinforced soil and sand (van Paassen et al., 2010; Gorospe et al., 2013). More recently, microorganism-induced mineralization has been utilized to engineer a living building material through binding and toughening a structural scaffold of sand and gelatin (Heveran et al., 2020).
Besides the above examples of CaCO3 precipitation, more direct evidence and explanation indicate that precipitation can occur on the surface of cementitious materials and aggregates. SEM images reveal that CaCO3 precipitates occur on the surface of microbial-treated sand (Porter et al., 2017) and recycled aggregates (Wang et al., 2017). Based on SEM and micro-CT characterizations of magnesia cement-based blends, microbial-induced formation of nesquehonite and hydromagnesite has been identified on the surface of cementitious materials and has been shown to exhibit healing effects (Ruan et al., 2019). The high cohesion of this precipitation was attributed to the incorporation of inorganic components and epitaxial growth of the crystals (Wang et al., 2016).
Taken together, the aforementioned studies indicate that microorganism-induced precipitation can produce NPs that exhibit beneficial nanostructural morphologies directly on aggregates surfaces. This potential type of nano-modified aggregates could enhance the engineering performance as common nano-additives and strengthen the ITZ of concrete.
3 Environmental Sustainability
In this section, we examine the feasibility of nanoscale biotechnologies to enhance the capability of cementitious materials to remediate heavy metals (section 3.1) and remove air pollutants (section 3.2). We then discuss application methods for these biotechnologies (section 3.3).
3.1 Heavy Metal Removal
Acceleration of some industrial activities, such as mining and battery production, has induced substantial discharge of heavy metals into the environment (Fu and Wang, 2011). Heavy metals with atomic weights from 63.5 to 200.6 and specific gravity >5.0, can accumulate in living microorganisms rather than being biodegraded. Some common heavy metals of great toxicity include cadmium, mercury, lead, zinc, copper, nickel and chromium. While these heavy metals possess a variety of chemical properties that correspond to multiple mechanisms of toxicity, in general, exposure results in cellular changes resulting in various health issues (Giovanella et al., 2020). For example, mercury causes dysfunction of proteins via complexing with the protein’s sulfhydryl groups that lead to long-lasting neurological and developmental deficits (Farina et al., 2011); chromium, when accumulated in the food chain especially in the form of Cr(VI), result in severe skin irritation or even lung carcinoma (Khezami and Capart, 2005).
Numerous techniques have been developed to remediate heavy metals, including chemical precipitation, membrane filtration, ion exchange and electrochemical technologies for treating wastewater, groundwater, and soil (Bradl, 2004; Fu and Wang, 2011; Hashim et al., 2011). The most common methods involve chemical precipitation (via hydroxide, carbonate and sulfide groups), lime coagulation, ion exchange (e.g., via resins), and chemical oxidation or reduction. These methods, however, are ineffective or costly (Suresh Kumar et al., 2015).
Microorganisms, including bacteria and algae, have proven useful candidates for remediation of heavy metals. Biological technologies exhibit high efficiency and low cost for removal of heavy metals from dilute solutions (Suresh Kumar et al., 2015). For example, the adsorption and elution of selected heavy metals were reported to be much higher by algae than by activated carbon and natural zeolites (Doshi et al., 2006). Here, we explore the feasibility of applying bioremediation in cementitious materials.
3.1.1 Immobilization in Cementitious Materials
Portland cement pastes have been shown to be a cost-efficient technique to immobilize heavy metals through three mechanisms: 1) sorption, 2) chemical incorporation (mainly precipitation and co-precipitation) and 3) encapsulation (Paria and Yuet, 2006; Chen et al., 2009). While sorption can be achieved physically via attraction to charged surfaces or chemically via covalent bonding especially at functional group sites of C-S-H phase, the latter two are more common mechanisms.
Chemical incorporation, mainly the formation of insoluble hydroxides, is a dominant mechanism for solidification and stabilization of heavy metals (Chen et al., 2009). As a result, metals including As(III), Cr(VI), and Hg that do not form hydroxides are not suitable for cement-based solidification. Meanwhile, pH is a critical influencing factor for the solidification of Cd, Cr, Cu, Pb Ni and Zn, because it affects solubility of their hydroxides. Besides hydroxide precipitation, carbonates, sulfates, and silicates of heavy metals are also precipitation strategies for solidifying heavy metals (Chen et al., 2009). Chemical incorporation in cement hydration products can also occur directly, since heavy metals, such as Zn and Pb, incorporate in the C-S-H in the Ca layer, Si chain or in the interlayer space (Guo et al., 2017a).
Heavy metals can be physically encapsulated by the C-S-H phase (Cartledge et al., 1990). Heavy metal wastes can be mixed with cementitious materials during their fresh state. Once materials are hardened, the heavy metals are isolated in a monolithic waste-like form that can exhibit long-term stability. Though physical encapsulation here is the main mechanism, chemical stabilization could also play a role (Guo et al., 2017a).
Geopolymers, as an alternative to ordinary portland cement (Chen et al., 2017b), also exhibit a propensity to adsorb heavy metals. In one study, the removal rate of Zn was shown to be 97.7% by the volcanic tuff based geopolymers but only 78.5% by the raw tuff, corroborating the potential of the geopolymerization techniques to remediate heavy metals (Al-Zboon et al., 2016). The mechanism often relates to the participation of heavy metals (e.g., Zn2+, Cu2+, Cd2+, and Cr3+) to charge-balance aluminates in the geopolymer framework (Rasaki et al., 2019), while other components (e.g., silicates) also react with heavy metals (e.g., Pb) to form Pb3Si5 precipitates (Guo et al., 2017a). Additionally, physical encapsulation and adsorption effects are also reported as primary immobilization mechanisms (Guo et al., 2017c; Ji and Pei, 2019).
The surface morphology and porosity of geopolymers affect their efficiency to remove heavy metals. For instance, the NaOH activated metakaolin geopolymers exhibit ∼90% removal capacity for Pb2+, Cu2+, Cr3+, and Cd2+, a phenomenon that is attributed to the mesoporous surface of aluminosilicate network (Cheng et al., 2012). A porous geopolymer (∼74.6% porosity) made from fly ash and iron ore tailing as the precursor and H2O2 as the foaming agent exhibited uptake percentage of Cu2+ at 90.7%, a high efficiency that was attributed to its well defined pore size distribution and high total porosity (Duan et al., 2016). Many other studies report that the adsorption of heavy metals (e.g., Pb2+, Cu2+, Sr2+, Co2+, Cs+, Cu2+, Cd2+, Zn2+, and Ni2+) follows the pseudo-second-order model (Rasaki et al., 2019). Fitting to this model, which involves intra-particle diffusion and kinetics parameters (Ho and McKay, 1999), suggests a heterogeneous surface of geopolymers for uptake of heavy metals. Considering the surface is a critical factor for removal capacity, several treatments that enhance the mesoporous nature by introducing chemical additives have shown to significantly increase the removal efficiency for heavy metals (Tang et al., 2015; Ge et al., 2017).
In summary, portland cements immobilize/solidify heavy metals mainly through the hardening process or precipitation (e.g., hydroxide). Furthermore, adsorption by geopolymers generally depends on their microstructural porosity and surface morphology. Both materials exhibit a potential to benefit from additional efficacy of heavy metal remediation enabled by biotechnology, for example via biomineralization on surfaces of aggregates or concrete materials, among other ways, as reviewed in detail in section 3.
3.1.2 Removal via Microorganisms
Many different types of microorganisms exhibit the capacity to remove heavy metals. A halophilic Haloferax strain was found to remove up to 21% of cadmium in a culture solution (Das et al., 2014). The thermophilic Geobacillus thermodentrificans cells removed multiple types of heavy metals (e.g., Fe, Cr, Cd, and Pb) via biosorption from an industrial effluent (Chatterjee et al., 2010). Mercury ions (Hg2+) from soil were reduced to Hg by the acidophile Acidithiobacillus ferrooxidans SUG 2–2 (Takeuchi et al., 2001). Many other acidophiles also exhibit great potency in removal of many types of heavy metals through various mechanisms (Dopson et al., 2003; Wu et al., 2012; Dopson and Holmes, 2014). Like bacteria, algae also exhibit high efficiency for heavy metal removal (Monteiro et al., 2012), even when used as non-living biomass (after acid-, heat- or other treatment of live cells) (Mehta and Gaur, 2005).
Most processes of bioremediation by cells are a resistance mechanism that allows for the survival in presence of heavy metals. These mechanisms are the foundation to develop biotechnologies for civil engineering that involve bioremediation. Using uranium as a general example, the bacterial mechanisms categorized as bioreduction (or biotransformation), biomineralization, biosorption, and bioaccumulation are illustrated in Figure 5 (Newsome et al., 2014).
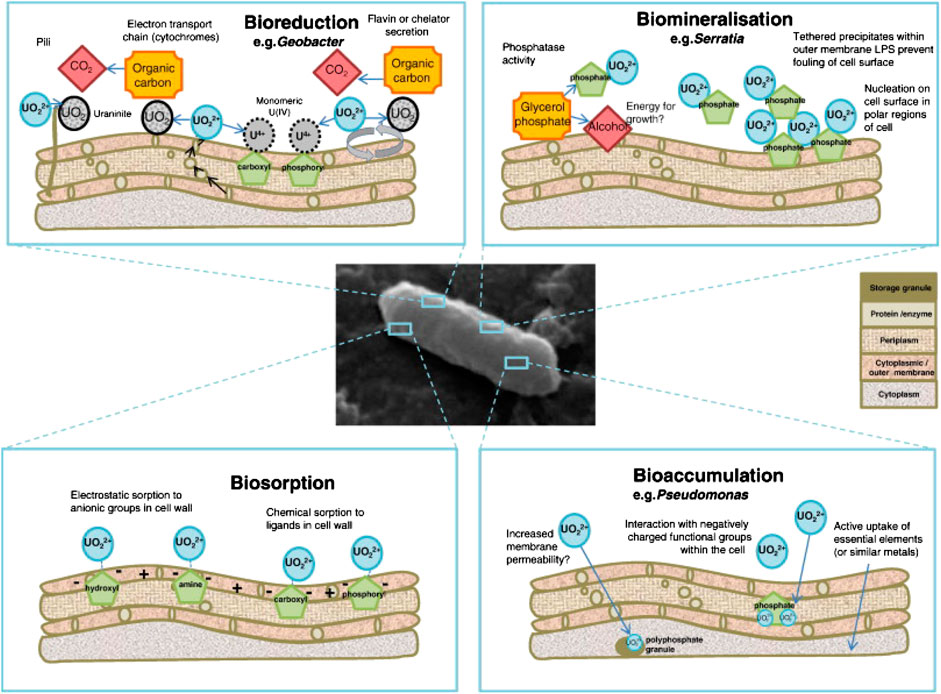
FIGURE 5. Schematic illustrating the mechanisms of interaction between a microbe and heavy metals (taking uranium as a general example): bioreduction, biomineralization, biosorption, and bioaccumulation (Newsome et al., 2014).
Bioreduction involves the conversion of oxidation states of metals, for example, by reducing U(VI) to U(IV) with Geobacter through respiration of metal ions as an electron acceptor to gain energy for metabolism (Figure 5) (Newsome et al., 2014). Aggregation of such bio-reduced metal ions would lead to the formation of NPs (as reviewed in section 2.2.1). For the transformation from Hg(II) to the least toxic form of Hg0 (Mathema et al., 2011), the mercury resistance genes relate to the key enzymes in the biotransformation process (Barkay et al., 2003). Specifically, the organomercury lyase (MerB) promotes the cleavage of organomercury compounds, and the released Hg2+ is reduced to Hg0 by mercuric reductase (MerA) (Benison et al., 2004).
Biomineralization, commonly seen as MICP in cementitious materials as reviewed in section 2.2.3, is also a critical mechanism to remediate heavy metals. To biomineralize uranium, the Serratia species convert the organic glycerol phosphate to inorganic phosphate that then precipitates U(VI) to form hydrogen uranyl phosphate (Figure 5) (Newsome et al., 2014).
Other resistance mechanisms involve removing heavy metals through biosorption or bioaccumulation. During biosorption, the heavy metals are bound to cell surface through a physio-chemical process, such as electrostatic and chemical sorption (Newsome et al., 2014). This process, however, is not effective in remediation for some metals, such as uranium, due to the high desorption rate. Usually, biosorption is among the first steps before bioreduction and biomineralization take place (Giovanella et al., 2020). To the contrary, bioaccumulation accumulates the heavy metals inside the cell (Chojnacka, 2010). During bioaccumulation, heavy metals are immobilized in cells through formation of metalloproteins, metal-binding peptides and polymers (Diep et al., 2018; Giovanella et al., 2020).
These processes/mechanisms of heavy-metal removal via microorganisms impart pathways for environmental remediation to civil engineers. Specifically, all the four mechanisms (i.e., bioreduction, biomineralization, biosorption, and bioaccumulation) could be exploited by directly adding microorganisms into polluted water/suspensions. Biomineralization could be promoted on surfaces of aggregates/fillers or bulk concrete materials in order to remove heavy metals. Bioreduction, biosorption and bioaccumulation can take place in biofilms that could grow on surfaces of cementitious materials. These applications are discussed in detail in section 3.3.
3.2 Air-Pollution Removal
3.2.1 Removal via Cementitious Materials
While heavy metal pollution mainly affects water and soil, air pollution is another major issue that has attracted much recent attention from academia and industry. Polluted air was found to be responsible for 6.4 million deaths worldwide in 2015 (Landrigan, 2017). CO2, NOx (e.g., nitrogen dioxide), and volatile organic compounds (VOCs) are major sources of pollution in urban regions (Petronella et al., 2017).
Cement paste has been shown to react with atmospheric CO2 (Šavija and Luković, 2016). Hempcrete, which consists of CH and CSH (i.e., the reaction products also seen in cement pastes), has been shown to sequester up to -16 kgCO2e/m2 over its lifecycle (Souto-Martinez et al., 2018b; Arehart et al., 2020). While the CO2-remediation capability of cement pastes is limited (Souto-Martinez et al., 2017; Souto-Martinez et al., 2018a), the remediation by calcium- and/or magnesium-containing minerals (usually present or being added to cements) are expensive. Sequestration is estimated at $50 to $300 per tCO(2) sequestered (Sanna et al., 2014).
The degradation of NOx has been explored by utilizing catalytic properties of TiO2 nanoparticles (Spasiano et al., 2015). As shown in Figure 6, upon illumination, TiO2 coated surfaces generate oxygen to oxidize NOx into nitric acid, which are subsequently neutralized by alkaline phases in the cementitious materials. This mechanism has been applied to treat NOx polluted air in research studies experimentally (Hüsken et al., 2009; Guo et al., 2017b), via modeling (Ballari et al., 2010), and by developing commercial products (Petronella et al., 2017).
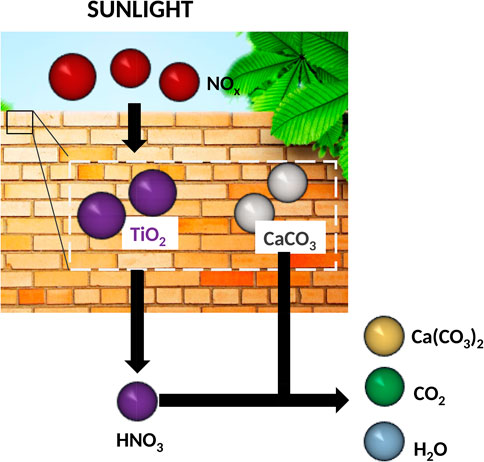
FIGURE 6. Schematic for neutralization of vehicle pollution (mainly NOx) via utilizing of energy captured from sunlight, adapted from Spasiano et al., (2015).
The photocatalytic process induced by TiO2 NPs have also been utilized to remove the toxic VOCs (e.g., ketones, halogenated hydrocarbons and aromatic compounds), leading to formation of benign products (e.g., H2O vapor and CO2) (Mo et al., 2009; Chen et al., 2012a; Petronella et al., 2017). An introduction of 1 wt% of TiO2 nanoparticles into portland cement was found to remove BTEX (benzene, toluene, ethylbenzene and o-xylene) up to 54% (Strini et al., 2005). Besides portland cement, geopolymers, when coated with a thin film of TiO2 NPs, exhibited degradation capability via photocatalytic process (Falah et al., 2016; Chen et al., 2017a).
Even though the applications of bio-based TiO2 NPs to cementitious have been limited so far, similar characteristics of abiotic TiO2 NPs with the bio-based NPs indicate such applications are promising. Microorganism produced TiO2 NPs can be anatase or rutile with particle sizes of ∼ 8–77 nm based on selected reports from literature (Table 1) (Bansal et al., 2005; Jha et al., 2009; Kirthi et al., 2011; Babitha and Korrapati, 2013). These characteristics are expected to exhibit identical photocatalytic properties and effects on the engineering performance of cement paste and concrete (Chen et al., 2012a).
3.2.2 Removal via Microorganisms
While TiO2 NPs produced via microorganisms (Table 1) can be applied to cementitious materials in the same manner as discussed in section 2.2.2, in this section we aim to review the remediation of air pollution directly by microorganisms. Some microorganisms exhibit great potential to sequester CO2. For example, growth of 1.0 kg microalgae is associated with fixation of 1.88 kg CO2 (Ho et al., 2011), much higher than carbon sequestration by type I cement based concrete over 25 years of exposure (i.e., <30 kg CO2 per 1 m3 of concrete column, or <0.0125 kg CO2 per kg of concrete if we assume a concrete density of 2,400 kg/m3) (Souto-Martinez et al., 2018a).
To remove CO2, MICP that converts CO2 into carbonate minerals has been shown to be an effective method (Anbu et al., 2016). As a safe and environment-friendly technique, carbonic-anhydrase-enzyme mediated MICP catalyzes reversible hydration of CO2 to formations of bicarbonates and calcite crystals (Liu et al., 2005; Kim et al., 2012). The carbonic anhydrase enzyme when purified deposits 15 times more carbonates than its crude counterpart (i.e., cells washed and resuspended in lysis buffer) (Ramanan et al., 2009).
While the CO2 remediation via carbonic-anhydrase based MICP could be costly (at least under lab scale where the extraction and purification of enzymes is complex), algae-based CO2 capture seems to be a more cost-efficient method. This CO2 capturing process is sustained under ambient temperature and daylight, and the resulting algal biomass can be used as biofuel, animal feed, and many other high-value products (Judd et al., 2015). Additionally, the algal photobioreactors can be as simple as a pond system that is robust to changes in CO2 concentration. Different algae have been reported to fix CO2 at different rates. While up to 56% of the total CO2 from a feed gas of 15% CO2 at a flow rate under 50 ml/min can be fixed by Chlorella vulgaris (Li et al., 2013), a fixation efficiency of 10% is seen for a gas containing 10% CO2 (flow rate not reported) by Dunaliella tertiolecta (Sydney et al., 2010). These efficiency values are generally dependent on many factors, including mass transfer of CO2 from gas to liquid phase, CO2 loading, biomass concentration and light intensity (Judd et al., 2015).
Microorganism-aided phytoremediation has been studied as one of the bio-approaches to remove air pollution. Endophytic microorganisms that colonize in the plant have shown to accelerate phytoremediation processes efficiently to decrease or remove VOCs and greenhouse gases (Stępniewska and Kuźniar, 2013). The bacterium Burkholderia cepacia L.S.2.4, a natural endophyte of yellow lupine, was genetically modified (commonly carried out to improve microorganisms’ target performance, such as the control of CaCO3 precipitation cited in the introduction section) by introducing a pTOM toluene-degradation plasmid of Burkholderia cepacian G4 and exhibited strong degradation capability for toluene (Barac et al., 2004). Some methanotropic bacteria (e.g., Methylocella palustris and Methylocapsa acidiphila) found in Sphagnum moss tissues were found to oxidize methane to carbon oxide that is then used by the moss plant during photosynthesis (Raghoebarsing et al., 2005; Stępniewska and Kuźniar, 2013). Such a cycle by oxidizing methane and utilizing carbon dioxide reduced the emission of these gases by up to 50% from peatlands (Kip et al., 2012).
More relevant to the removal of NxO gases by TiO2 as reviewed in section 3.2.1 above, several bacteria have been found to exhibit N2O-reducing capability (Hallin et al., 2018). The Bacillus vireti with the N2O reductase acts as the potential sink for N2O with the efficiency depending on the nitrate concentrations in the culture medium (Mania et al., 2016). While exploration in biochemistry and genetics of N2O respiration has made great progress (e.g., the discovery of the multicopper enzyme that catalyzes the transformation of N2O), further such mechanistic understanding at molecular level is needed before we can tailor the microorganisms for more efficient mitigation of N2O emissions (Zumft and Kroneck, 2006).
3.3 Applications of Nanoscale Biotechnology in Cementitious Materials
Currently, while very few applications of microorganisms have been reported to enhance the heavy metal removal in civil engineering, the reported cases as discussed above suggest great potential of bioremediation using civil engineering materials. Methods of application can be categorized into three types: 1) direct application of microorganisms in polluted water/suspensions; 2) surface treatment of cementitious materials and/or aggregates via biomineralization; and 3) surface treatment via formation of a biofilm. While biomineralization (2nd method) could directly relate to the nanoscale aspect, the direct application (1st method) and formation of biofilm (3rd method) could associate with formation of NPs and thus can also be regarded as “nanoscale” biotechnology.
Microorganisms have been directly mixed with polluted water or suspension and found to exhibit high efficiency for heavy metal removal (e.g., Fe, Zn, Cr) in soil, constructed wetland, and unclear waste disposal cells (Alquier et al., 2014; Singh et al., 2015; Bano et al., 2018; Wang et al., 2020). Below we focus on the two surface treatments that are more directly relevant to cementitious materials.
3.3.1 Surface Treatment of Cementitious Materials and Aggregates via Biomineralization
Surface precipitation on cementitious materials and aggregates can be achieved by biomineralization, as reviewed in section 2.2.3. Biomineralization has also been shown to remediate heavy metals (Anbu et al., 2016; Kumari et al., 2016). For example, the strain Kocuria flava CR1, an isolate from a mining area in China, removed up to 97% of copper through formation of carbonates (Achal et al., 2011). Biomineralization was seen to remove 99.95% of Cd at 2 g/L in 48 h in a culture media (Kang et al., 2014). Radionuclides (e.g., U, Sr, Np, and Cs) have also been removed through biomineralization of calcium carbonates and coprecipitation of radionuclide carbonates (Newsome et al., 2014).
With the capability to remove heavy metals, as well as to induce nanostructural surface precipitates (see section 2.2.3), the biomineralization process potentially brings additional functionality of heavy-metal removal to cementitious materials. More specifically, there are two possible approaches: 1) to promote biomineralization of heavy metals on the surface of concrete; and 2) to biomineralize aggregates prior to mixing with cementitious binders. The direct biomineralization or coating on the surface of concrete aided by microorganisms (De Muynck et al., 2008; Wang et al., 2016; Wong et al., 2020) potentially equips the concrete surfaces with the functionality of bioremediation. Alternatively, the aggregates or even recycled cementitious aggregates can be used to remove heavy metals in polluted media through biomineralization in a similar fashion to CaCO3 precipitation in soil and sand and on aggregate surfaces (Achal et al., 2013; Cuzman et al., 2015; Wang et al., 2017). Once precipitated as NPs on the aggregate surfaces, heavy metal pollutants can be encapsulated in the cementitious matrix as reviewed in section 3.1.1. Furthermore, nanostructural morphology on the aggregate surface could further enhance the engineering performance of concrete materials, as discussed in section 2.2.3. Such a pathway would not only allow bioremediation of heavy metals but also convert contaminated materials into high-performance concrete materials. Though more studies are needed to develop such multi-functional concrete with enhanced bioremediation capability and engineering performance, currently available information indicates this area of research and applications is promising.
3.3.2 Surface Treatment via Formation of Beneficial Biofilms
A biofilm is a group of microorganisms, including bacteria, fungi and protists, that commonly grow on living or non-living surfaces (Flemming and Wingender, 2010). In addition to the cells, biofilms are composed of a matrix of extracellular polysaccharides (EPS), proteins, and DNA, as well as absorbed nutrients, metabolites, ions/minerals, and some detritus from the surrounding environment.
Besides promoting precipitation through MICP (Decho, 2010) and protecting Ca-bearing materials by the precipitation of calcium oxalate at their surface (e.g., by lichen (Ariño et al., 1997)), biofilms exhibit remediation capability for heavy metals. For example, a sulphate-reducing bacterial biofilm was found to remediate a copper-contaminated medium through formation of copper sulfide (White and Gadd, 2000); natural films of sulfate-reducing bacteria of the Desulfobacteriaceae family were seen to concentrate Zn ions and promote precipitation of sphalerite (ZnS) particles of 2–5 nm (Labrenz et al., 2000). Other heavy metals (e.g., Cd, Ni, Co. Pd) can also be remediated (Singh et al., 2006). Such bioremediation process usually involves an immobilization of contaminants by microbial transformation that leads to detoxification and precipitation, sorption to cells or matrix, and biomineralization (Barkay and Schaefer, 2001). This remediation capability likely relates to biofilms’ high resistance to heavy metals (e.g., up to 600 times higher resistance for Pseudomonas eruginosa based biofilm than free-swimming cells (Teitzel and Parsek, 2003)), together with their wide chemical, physical, and structural diversity (Pal and Paul, 2008).
Apart from remediating heavy metals (usually accompanied by formation of NPs), biofilms can be applied on concrete surfaces. While biofilms have been more widely applied to protect the metal surfaces from corrosion (Zuo, 2007), several applications to concrete have been reported (Soleimani et al., 2013a; Soleimani et al., 2013b; Soleimani et al., 2013c). An E. coli DH5α biofilm can successfully cover mortar samples, continue to grow (almost doubling the thickness) despite application of sulfuric acid down to pH of 3, and reduce leaching of Ca2+ by 23–47% compared to samples without the biofilms (Soleimani et al., 2013a). This biofilm-covered mortar, when soaked in a culturing media with the undesirable sulfur-oxidizing bacteria (SOB) that induce the formation of sulfuric acid, exhibits a competing effect with growth of the SOB (Soleimani et al., 2016). Biofilms also help improve the durability through sealing the underlying microcracks and lowering the diffusion rates of chloride (Chlayon et al., 2018). In summary, the application of biofilms that are capable of both heavy metal bioremediation and formation of a barrier on concrete surface is a promising tool to tailor concrete materials with capability of bioremediation and high durability.
4 Research Significance and Future Developments
4.1 Significance: Opportunities and Challenges
This review highlights innovations in a new field of nanoscale construction biotechnology and applications in the design of resilient and sustainable civil engineering materials to benefit the construction industry. Nanotechnology has been shown to substantially improve the strength and durability of cementitious materials (see Table 1) (Sobolev et al., 2006; Rashad, 2013a; Rashad, 2013b; Reches, 2018; Mindess, 2019), while new biotechnologies offer an ideal solution to design environmentally friendly nano-additives by minimizing environmental and economic costs (Li et al., 2011; Tiquia-Arashiro and Rodrigues, 2016). Among biotechnology’s great environmental benefits to civil engineering materials as discussed in section 3.1 and 3.2, water treatment is one main application. As one example, yeast- and lactose-assisted bioremediation has successfully cleaned a large area (2–3 ha) of chlorine-contaminated area (town of Salem, New Hampshire) with an expenditure of just US $ 300,000 by saving an estimated US $ 2 million in remediation by conventional pump-and-treat approach (Schaffner, 2004). By taking the advantages of both nanotechnology and biotechnology, the engineering performance and environmental benefits of cementitious materials can be substantially improved (see Figure 7).
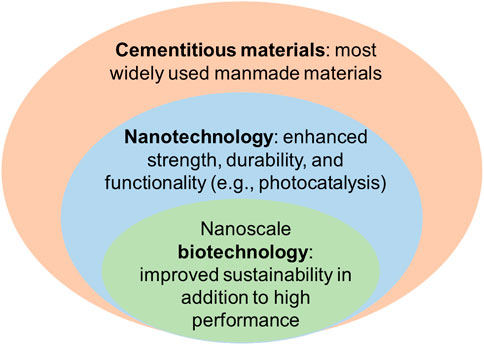
FIGURE 7. Schematic illustrating that nanoscale biotechnology leverages the advantages of two maturing fields—nanotechnology and biotechnology—and has applications in the design of high-performance, sustainable construction materials.
4.2 Opportunity: Enhancing Environmental Tolerance and Performance via Genetic Engineering
Recent advances in synthetic biology can further enhance the environmental tolerance and bioproduction of microorganisms. Synthetic biology is an engineering approach to biology that aims to construct new biological systems (e.g., living organisms) to have novel—or enhanced—abilities or useful purposes (e.g., material production, sense-and-respond functionality). Some of the methods used by synthetic biologists include DNA manipulation technologies, as well as omits approaches (i.e., genomics, transcriptomics, proxemics, and metabonomics).
The ability of microorganisms to survive (when necessary) while producing NPs and removing heavy metals largely depend on their adaptation to relevant environmental conditions. Under the extreme conditions in the cementitious materials (e.g., high ionic concentration, and high alkalinity), microorganisms’ survival and capability in NP production and metal removal could be of critical importance. To elucidate the genetic underpinnings of microorganism biosynthesis and persistence, omits approaches have been adopted to examine genomes, transcriptases, and proteomes (Giovanella et al., 2020) and their governance rules on microorganism behavior. For example, genome sequencing is often conducted on extremophiles that exhibit a strong adaptation to harsh pH, temperature, pressure, salinity and others. In doing so, genes relevant to the versatile environmental adaptability are revealed (Giovanella et al., 2020).
With this understanding, genetic modification can be achieved by utilizing recombinant DNA technology and selective pressure for rational design and directed evolution. While rational design involves construction of a single strain that possesses desirable metabolic characteristics or proteins from different organisms to allow specific reactions using recombinant DNA technology (Ang et al., 2005), directed evolution engineers the organism for improved characteristics through mutagenesis and environmental pressure based on Darwinian principles of mutation and selection (Hibbert and Dalby, 2005).
Based on this genetic engineering, genetically engineered microorganisms can be produced that exhibit boosted biosynthesis or efficiency in heavy metal removal. Heterologous biosynthesis pathways can also be engineered into non-native producer organisms (Kumar et al., 2013). For example, the size, shape and mechanical properties of biogenic CaCO3 precipitates can be tailored via genetically engineered E. coli strains (Liang et al., 2018; Heveran et al., 2019). Similarly, the abilities of sulfur-oxidizing and sulfate-reducing microorganisms have been engineered to increase extraction and capture of metals (Dopson and Johnson, 2012).
In addition to improvement in biosynthesis and heavy-metal removal, enhanced survivability of microorganisms can be achieved via genetic modification. For example, a new strain of E. coli was engineered to express a hypersaline azoreductase gene originally from Halomonas elongata allowing it to tolerate higher NaCl concentrations (Eslami et al., 2016). In another instance, Pichia pastoris expressed the laccase gene from Thermus thermophiles inducing greater tolerance to high temperature (∼90°C) (Liu et al., 2015). More relevant to the high alkaline environment in concrete materials, mutations to the Sporosarcina pasteurii (a strain capable of MICP) genome were induced via ultraviolet irradiation, and the mutants were capable of biomineralization under pH of 11–12 (Achal et al., 2009b). Such improved biosynthesis and tolerance to the harsh environments exhibits potential to enhance microorganism’s resilience for NP synthesis and removal of heavy metals.
In short, with the advent of new, rapid throughput DNA sequencing techniques, data analysis, and recombinant DNA technology, the fundamental understanding and modification of genes at a molecular level provide great opportunities to impart boosted functionalities to microorganisms that are of specific interest to civil engineers (e.g., biosynthesis of NPs and heavy metal remediation).
4.3 Challenge: Ensuring the Energy- and Cost-Efficiency of Biotechnology
Improved sustainability related to biological vs. physical or chemical synthesis has been claimed in almost all relevant studies (Li et al., 2011; Hulkoti and Taranath, 2014; Tiquia-Arashiro and Rodrigues, 2016; Iravani and Varma, 2020). The primary argument relates to the potential elimination of expensive and sometimes toxic solvents and by replacing energy intensive processes with biological ones. Improved sustainability, however, is not always guaranteed. For example, the biological process for producing TiO2 NPs demands cumulative energy only lower than the RF thermal plasma method but higher than all the other conventional methods, as shown in Figure 8 (Wu et al., 2019). As an example of potentially lower energy consumption, parameter optimization for biosynthesis of magnetite NPs (e.g., reducing the amount of sodium hydroxide and replacing iron sources) offers a six-fold reduction in environmental impacts (Sadhukhan et al., 2017). Furthermore, a systematic comparison between the biosynthesis of NPs and their conventional counterparts is rarely reported (Sadhukhan et al., 2017). Even for conventional synthesis, life cycle assessments using standardized methodologies that quantify environmental impacts face a number of challenges, especially at the industrial scale (Charitidis et al., 2014). These challenges are partially due to different equipment and methods required between lab and industrial scales (Hetherington et al., 2014). Therefore, in addition to research that leverages the advancements in nanotechnology and biotechnology, systematic quantification of environmental and economic impacts is essential prior to full-scale production and widespread application.
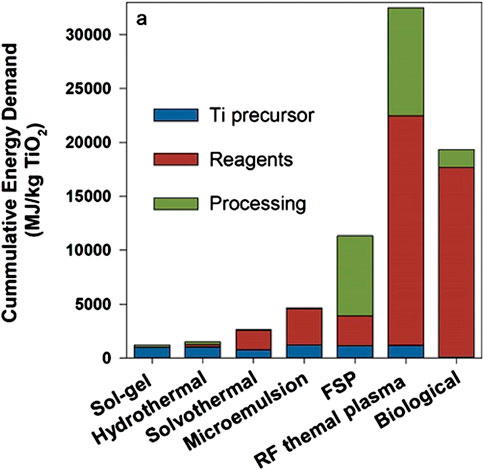
FIGURE 8. Cumulative energy demand (MJ/kg TiO2) of different conventional methods vs. the biological counterpart (Wu et al., 2019). FSP = flame spray pyrolysis; FR = radio frequency.
In addition to energy consumption associated with biotechnology, cost is an important consideration. The majority of biotechnology cost is claimed to come from the nutrients used to grow microorganisms (Achal et al., 2015). In the case of biocementation via MICP, yeast extract, meat extract, glucose, peptone and salts are commonly added to the nutrient media. The cost of these nutrients is estimated to be around $250 per kilogram, which could be used to produce 5.4E6 unit urease (i.e., enzyme that could hydrolyze 5.4E6 micromoles of urea in 1 min) (Achal et al., 2015). Fortunately, some nutrient-rich industrial effluents, such as lactose mother liquor (Achal et al., 2009a) and corn steep liquor (Achal et al., 2010; Fahmi et al., 2018), could serve as replacements and end with comparable or even enhanced precipitation of carbonates. In a similar fashion of using wastes, pumpkin peel is used as a carbon and energy source for fungi to produce Cu/Zn NPs (Noman et al., 2019). Additionally, careful selection of microorganism species offers additional benefits, for example, by using cyanobacteria to promote MICP via photosynthesis that does not require as much exogenous nutrient addition (Kamennaya et al., 2012). Future research in this area is of great importance to advance applications of biotechnology in civil engineering at scale.
5 Conclusion
In this review, we summarize the promise and potential of a new field of nanoscale construction biotechnology. By combining the advantages of nanotechnology with advances in biotechnology, this new field offers multiple opportunities to revolutionize the field of construction.
As a green alternative to the conventional nanotechnology, biologically produced NPs can exhibit comparable characteristics to those that have been proven to enhance the performance of cementitious materials. While direct addition of bio-NPs is proven feasible, surface treatment of NPs on the surfaces of aggregates prior to mixing with cementitious binders is an alternate, yet unexplored, strategy to enhance concrete performance.
While nanotechnological enhancements of materials can reduce economic and environmental costs, the application microorganisms potentially equip cementitious materials with additional functionality to remove heavy metals (e.g., Hg, U, Cr) from water and soil and clean pollutants from the air. Three possible approaches to achieve this multifunctionality have been reviewed: 1) direct application of microorganisms, 2) surface treatment in cementitious (and other) materials via biomineralization, and 3) formation of active biofilm surfaces. Together, these opportunities afford civil engineers a materials-centric approach to water treatment and recover rare earth elements.
This review also summarizes the challenges and opportunities of nanoscale biotechnology and proposes a roadmap of research for civil engineers of the 21st century. There exist great opportunities to enhance the environmental tolerance and performance of biotechnological systems, thanks in large part to the rapid development of genetic engineering. While concerns are present with regards to the biotechnology’s energy- and cost-efficiency, corresponding opportunities exist in parallel, as evidenced by initial proofs of concept that have been successfully reported. By taking advantage of both nanotechnology and advances in biotechnology, critical aspects of resilience and sustainability could be addressed within (and beyond) the modern civil engineering discipline.
Author Contributions
WS conceived of the study and provided writing and editing. XC conducted the study and wrote the first draft of the manuscript. MC contributed to the study and provided editing and revision of the manuscript.
Funding
The work depicted was sponsored by the Advanced Research Projects Agency-Energy (Award Number: DE-AR0001145) and the Defense Advanced Research Projects Agency (Agreement HR0011-17-2-0039). Publication of this article was funded by the University of Colorado Boulder Libraries Open Access Fund
Conflict of Interest
The authors declare that the research was conducted in the absence of any commercial or financial relationships that could be construed as a potential conflict of interest.
Acknowledgments
The content does not necessarily reflect the position or the policy of the Government, and no official endorsement should be inferred.
References
Achal, V., Mukherjee, A., Basu, P. C., and Reddy, M. S. (2009a). Lactose mother liquor as an alternative nutrient source for microbial concrete production by Sporosarcina pasteurii. J. Ind. Microbiol. Biotechnol. 36, 433–438. doi:10.1007/s10295-008-0514-7
Achal, V., Mukherjee, A., Basu, P. C., and Reddy, M. S. (2009b). Strain improvement of Sporosarcina pasteurii for enhanced urease and calcite production. J. Ind. Microbiol. Biotechnol. 36, 981–988. doi:10.1007/s10295-009-0578-z
Achal, V., Pan, X., Lee, D. J., Kumari, D., and Zhang, D. (2013). Remediation of Cr(VI) from chromium slag by biocementation. Chemosphere. 93, 1352–1358. doi:10.1016/j.chemosphere.2013.08.008
Achal, V., Mukherjee, A., Kumari, D., and Zhang, Q. (2015). Biomineralization for sustainable construction–a review of processes and applications. Earth Sci. Rev. 148, 1–17. doi:10.1016/j.earscirev.2015.05.008
Achal, V., Mukherjee, A., and Reddy, M. S. (2010). Original research: biocalcification by Sporosarcina pasteurii using corn steep liquor as the nutrient source. Ind. Biotechnol. 6, 170–174. doi:10.1089/ind.2010.6.170
Achal, V., Pan, X., and Zhang, D. (2011). Remediation of copper-contaminated soil by Kocuria flava CR1, based on microbially induced calcite precipitation. Ecol. Eng. 37, 1601–1605. doi:10.1016/j.ecoleng.2011.06.008
Afzali Naniz, O., and Mazloom, M. (2018). Effects of colloidal nano-silica on fresh and hardened properties of self-compacting lightweight concrete. J. Build. Eng. 20, 400–410. doi:10.1016/j.jobe.2018.08.014
Al-Zboon, K. K., Al-Smadi, B. M., and Al-Khawaldh, S. (2016). Natural volcanic tuff-based geopolymer for Zn removal: adsorption isotherm, kinetic, and thermodynamic study. Water, Air, Soil Pollut. 227, 248. doi:10.1007/s11270-016-2937-5
Alquier, M., Kassim, C., Bertron, A., Sablayrolles, C., Rafrafi, Y., Albrecht, A., et al. (2014). Halomonas desiderata as a bacterial model to predict the possible biological nitrate reduction in concrete cells of nuclear waste disposals. J. Environ. Manag. 132, 32–41. doi:10.1016/j.jenvman.2013.10.013
Anbu, P., Kang, C. H., Shin, Y. J., and So, J. S. (2016). Formations of calcium carbonate minerals by bacteria and its multiple applications. SpringerPlus. 5, 250. doi:10.1186/s40064-016-1869-2
Ang, E. L., Zhao, H., and Obbard, J. P. (2005). Recent advances in the bioremediation of persistent organic pollutants via biomolecular engineering. Enzym. Microb. Technol. 37, 487–496. doi:10.1016/j.enzmictec.2004.07.024
Arakaki, A., Nakazawa, H., Nemoto, M., Mori, T., and Matsunaga, T. (2008). Formation of magnetite by bacteria and its application. J. R. Soc. Interface. 5, 977–999. doi:10.1098/rsif.2008.0170
Arehart, J. H., Nelson, W. S., and Srubar, W. V. (2020). On the theoretical carbon storage and carbon sequestration potential of hempcrete. J. Clean. Prod. 266, 121846. doi:10.1016/j.jclepro.2020.121846
Ariño, X., Gomez-Bolea, A., and Saiz-Jimenez, C. (1997). Lichens on ancient mortars. Int. Biodeter. Bioder. 40, 217–224. doi:10.1016/S0964-8305(97)00036-X
Babitha, S., and Korrapati, P. S. (2013). Biosynthesis of titanium dioxide nanoparticles using a probiotic from coal fly ash effluent. Mater. Res. Bull. 48, 4738–4742. doi:10.1016/j.materresbull.2013.08.016
Balaji, D. S., Basavaraja, S., Deshpande, R., Mahesh, D. B., Prabhakar, B. K., and Venkataraman, A. (2009). Extracellular biosynthesis of functionalized silver nanoparticles by strains of Cladosporium cladosporioides fungus. Colloids Surf. B. Biointerfaces. 68, 88–92. doi:10.1016/j.colsurfb.2008.09.022
Balasoiu, M., Ischenko, L. A., Stolyar, S. V., Iskhakov, R. S., Raikher, Y. L., Kuklin, A. I., et al. (2010). Structural investigation of biogenic ferrihydrite nanoparticles dispersion. Optoelectron Adv. Mat. 4, 2136–2139.
Ballari, M. M., Hunger, M., Hüsken, G., and Brouwers, H. J. H. (2010). NOx photocatalytic degradation employing concrete pavement containing titanium dioxide. Appl. Catal. B Environ. 95, 245–254. doi:10.1016/j.apcatb.2010.01.002
Bano, A., Hussain, J., Akbar, A., Mehmood, K., Anwar, M., Hasni, M. S., et al. (2018). Biosorption of heavy metals by obligate halophilic fungi. Chemosphere. 199, 218–222. doi:10.1016/j.chemosphere.2018.02.043
Bansal, V., Rautaray, D., Ahmad, A., and Sastry, M. (2004). Biosynthesis of zirconia nanoparticles using the fungus Fusarium oxysporum. J. Mater. Chem. 14, 3303–3305. doi:10.1039/B407904C
Bansal, V., Rautaray, D., Bharde, A., Ahire, K., Sanyal, A., Ahmad, A., et al. (2005). Fungus-mediated biosynthesis of silica and titania particles. J. Mater. Chem. 15, 2583–2589. 10.1039/b503008k
Barac, T., Taghavi, S., Borremans, B., Provoost, A., Oeyen, L., Colpaert, J. V., et al. (2004). Engineered endophytic bacteria improve phytoremediation of water-soluble, volatile, organic pollutants. Nat. Biotechnol. 22, 583–588. doi:10.1038/nbt960
Barkay, T., Miller, S. M., and Summers, A. O. (2003). Bacterial mercury resistance from atoms to ecosystems. FEMS Microbiol. Rev. 27, 355–384. doi:10.1016/S0168-6445(03)00046-9
Barkay, T., and Schaefer, J. (2001). Metal and radionuclide bioremediation: issues, considerations and potentials. Curr. Opin. Microbiol. 4, 318–323. doi:10.1016/s1369-5274(00)00210-1
Barnhouse, P. W., and Srubar, W. V. (2016). Material characterization and hydraulic conductivity modeling of macroporous recycled-aggregate pervious concrete. Conster. Build. Mater. 110, 89–97. doi:10.1016/j.conbuildmat.2016.02.014
Basaran Bundur, Z., Kirisits, M. J., and Ferron, R. D. (2015). Biomineralized cement-based materials: impact of inoculating vegetative bacterial cells on hydration and strength. Cement Concr. Res. 67, 237–245. doi:10.1016/j.cemconres.2014.10.002
Beeler, E., and Singh, O. V. (2016). Extremophiles as sources of inorganic bio-nanoparticles. World J. Microbiol. Biotechnol. 32, 156. doi:10.1007/s11274-016-2111-7
Benison, G. C., Di Lello, P., Shokes, J. E., Cosper, N. J., Scott, R. A., Legault, P., et al. (2004). A stable mercury-containing complex of the organomercurial lyase MerB: catalysis, product release, and direct transfer to MerA. Biochemistry. 43, 8333–8345. doi:10.1021/bi049662h
Bharde, A. A., Parikh, R. Y., Baidakova, M., Jouen, S., Hannoyer, B., Enoki, T., et al. (2008). Bacteria-mediated precursor-dependent biosynthesis of superparamagnetic iron oxide and iron sulfide nanoparticles. Langmuir. 24, 5787–5794. doi:10.1021/la704019p
Bhattacharyya, A., Schmidt, M. P., Stavitski, E., Azimzadeh, B., and Martínez, C. E. (2019). Ligands representing important functional groups of natural organic matter facilitate Fe redox transformations and resulting binding environments. Geochem. Cosmochim. Acta. 251, 157–175. doi:10.1016/j.gca.2019.02.027
Bose, S., Hochella, M. F., Gorby, Y. A., Kennedy, D. W., Mccready, D. E., Madden, A. S., et al. (2009). Bioreduction of hematite nanoparticles by the dissimilatory iron reducing bacterium Shewanella oneidensis MR-1. Geochem. Cosmochim. Acta. 73, 962–976. doi:10.1016/j.gca.2008.11.031
Bradl, H. B. (2004). Adsorption of heavy metal ions on soils and soils constituents. J. Colloid Interface Sci. 277, 1–18. doi:10.1016/j.jcis.2004.04.005
Cartledge, F. K., Butler, L. G., Chalasani, D., Eaton, H. C., Frey, F. P., Herrera, E., et al. (1990). Immobilization mechanisms in solidification/stabilization of cadmium and lead salts using portland cement fixing agents. Environ. Sci. Tech. 24, 867–873. doi:10.1021/es00076a012
Ceran, Ö. B., Şimşek, B., Doruk, S., Uygunoğlu, T., and Şara, O. N. (2019). Effects of dispersed and powdered silver nanoparticles on the mechanical, thermal, electrical and durability properties of cementitious composites. Conster. Build. Mater. 222, 152–167. doi:10.1016/j.conbuildmat.2019.06.138
Charitidis, C. A., Georgiou, P., Koklioti, M. A., Trompeta, A.-F., and Markakis, V. (2014). Manufacturing nanomaterials: from research to industry. Manuf. Rev. 1, 11. doi:10.1051/mfreview/2014009
Chatterjee, S. K., Bhattacharjee, I., and Chandra, G. (2010). Biosorption of heavy metals from industrial waste water by Geobacillus thermodenitrificans. J. Hazard Mater. 175, 117–125. doi:10.1016/j.jhazmat.2009.09.136
Chen, H., Nanayakkara, C. E., and Grassian, V. H. (2012a). Titanium dioxide photocatalysis in atmospheric chemistry. Chem. Rev. 112, 5919–5948. doi:10.1021/cr3002092
Chen, J., Kou, S.-C., and Poon, C.-S. (2012b). Hydration and properties of nano-TiO2 blended cement composites. Cement Concr. Compos. 34, 642–649. doi:10.1016/j.cemconcomp.2012.02.009
Chen, L., Zheng, K., and Liu, Y. (2017a). Geopolymer-supported photocatalytic TiO2 film: preparation and characterization. Conster. Build. Mater. 151, 63–70. doi:10.1016/j.conbuildmat.2017.06.097
Chen, Q. Y., Tyrer, M., Hills, C. D., Yang, X. M., and Carey, P. (2009). Immobilisation of heavy metal in cement-based solidification/stabilisation: a review. Waste Manag. 29, 390–403. doi:10.1016/j.wasman.2008.01.019
Chen, X., Sutrisno, A., Zhu, L., and Struble, L. J. (2017b). Setting and nanostructural evolution of metakaolin geopolymer. J. Am. Ceram. Soc. 100, 2285–2295. doi:10.1111/jace.14641
Cheng, T. W., Lee, M. L., Ko, M. S., Ueng, T. H., and Yang, S. F. (2012). The heavy metal adsorption characteristics on metakaolin-based geopolymer. Appl. Clay Sci. 56, 90–96. doi:10.1016/j.clay.2011.11.027
Chlayon, T., Iwanami, M., and Chijiwa, N. (2018). Combined protective action of barnacles and biofilm on concrete surface in intertidal areas. Conster. Build. Mater. 179, 477–487. doi:10.1016/j.conbuildmat.2018.05.223
Chojnacka, K. (2010). Biosorption and bioaccumulation--the prospects for practical applications. Environ. Int. 36, 299–307. doi:10.1016/j.envint.2009.12.001
Colvin, V. L. (2003). The potential environmental impact of engineered nanomaterials. Nat. Biotechnol. 21, 1166–1170. doi:10.1038/nbt875
Cuevas, R., Durán, N., Diez, M. C., Tortella, G. R., and Rubilar, O. (2015). Extracellular biosynthesis of copper and copper oxide nanoparticles by Stereum hirsutum, a native white-rot fungus from Chilean forests. J. Nanomater. 2015, 789089. doi:10.1155/2015/789089
Cuzman, O. A., Rescic, S., Richter, K., Wittig, L., and Tiano, P. (2015). Sporosarcina pasteurii use in extreme alkaline conditions for recycling solid industrial wastes. J. Biotechnol. 214, 49–56. doi:10.1016/j.jbiotec.2015.09.011
Das, D., Salgaonkar, B. B., Mani, K., and Braganca, J. M. (2014). Cadmium resistance in extremely halophilic archaeon Haloferax strain BBK2. Chemosphere. 112, 385–392. doi:10.1016/j.chemosphere.2014.04.058
De Muynck, W., De Belie, N., and Verstraete, W. (2010). Microbial carbonate precipitation in construction materials: a review. Ecol. Eng. 36, 118–136. doi:10.1061/(ASCE)MT.1943-5533.0003141
De Muynck, W., Debrouwer, D., De Belie, N., and Verstraete, W. (2008). Bacterial carbonate precipitation improves the durability of cementitious materials. Cement Concr. Res. 38, 1005–1014. doi:10.1016/j.cemconres.2008.03.005
Decho, A. W. (2010). Overview of biopolymer-induced mineralization: what goes on in biofilms? Ecol. Eng. 36, 137–144. doi:10.1016/j.ecoleng.2009.01.003
Dejong, J. T., Soga, K., Banwart, S. A., Whalley, W. R., Ginn, T. R., Nelson, D. C., et al. (2011). Soil engineering in vivo: harnessing natural biogeochemical systems for sustainable, multi-functional engineering solutions. J. R. Soc. Interface. 8, 1–15. doi:10.1098/rsif.2010.0270
Diep, P., Mahadevan, R., and Yakunin, A. F. (2018). Heavy metal removal by bioaccumulation using genetically engineered microorganisms. Front. Bioeng. Biotech. 6, 157. 10.3389/fbioe.2018.00157
Dopson, M., Baker-Austin, C., Koppineedi, P. R., and Bond, P. L. (2003). Growth in sulfitic mineral environments: metal resistance mechanisms in acidophilic micro-organisms. Microbiology (Reading, Engl). 149, 1959–1970. doi:10.1099/mic.0.26296-0
Dopson, M., and Holmes, D. S. (2014). Metal resistance in acidophilic microorganisms and its significance for biotechnologies. Appl. Microbiol. Biotechnol. 98, 8133–8144. doi:10.1007/s00253-014-5982-2
Dopson, M., and Johnson, D. B. (2012). Biodiversity, metabolism and applications of acidophilic sulfur-metabolizing microorganisms. Environ. Microbiol. 14, 2620–2631. doi:10.1111/j.1462-2920.2012.02749.x
Doshi, H., Ray, A., Kothari, I. L., and Gami, B. (2006). Spectroscopic and scanning electron microscopy studies of bioaccumulation of pollutants by algae. Curr. Microbiol. 53, 148–157. doi:10.1007/s00284-005-0401-7
Du, H., Du, S., and Liu, X. (2014). Durability performances of concrete with nano-silica. Conster. Build. Mater. 73, 705–712. doi:10.1016/j.conbuildmat.2014.10.014
Duan, P., Yan, C., Zhou, W., and Ren, D. (2016). Development of fly ash and iron ore tailing based porous geopolymer for removal of Cu(II) from wastewater. Ceram. Int. 42, 13507–13518. doi:10.1016/j.ceramint.2016.05.143
Eslami, M., Amoozegar, M. A., and Asad, S. (2016). Isolation, cloning and characterization of an azoreductase from the halophilic bacterium Halomonas elongata. Int. J. Biol. Macromol. 85, 111–116. doi:10.1016/j.ijbiomac.2015.12.065
Fahmi, A., Katebi, H., Hajialilue Bonab, M., and Samadi Kafil, H. (2018). Microbial sand stabilization using corn steep liquor culture media and industrial calcium reagents in cementation solutions. Ind. Biotechnol. 14, 270–275. doi:10.1089/ind.2018.0016
Falah, M., Mackenzie, K. J. D., Knibbe, R., Page, S. J., and Hanna, J. V. (2016). New composites of nanoparticle Cu (I) oxide and titania in a novel inorganic polymer (geopolymer) matrix for destruction of dyes and hazardous organic pollutants. J. Hazard Mater. 318, 772–782. doi:10.1016/j.jhazmat.2016.06.016
Fang, X. J., Wang, Y., Wang, Z. G., Jiang, Z. X., and Dong, M. D. (2019). Microorganism assisted synthesized nanoparticles for catalytic applications. Energies. 12, 21. doi:10.3390/en12010190
Faramarzi, M. A., and Sadighi, A. (2013). Insights into biogenic and chemical production of inorganic nanomaterials and nanostructures. Adv. Colloid Interface Sci. 189–190, 1–20. doi:10.1016/j.cis.2012.12.001
Farina, M., Aschner, M., and Rocha, J. B. (2011). Oxidative stress in MeHg-induced neurotoxicity. Toxicol. Appl. Pharmacol. 256, 405–417. doi:10.1016/j.taap.2011.05.001
Flemming, H. C., and Wingender, J. (2010). The biofilm matrix. Nat. Rev. Microbiol. 8, 623–633. doi:10.1038/nrmicro2415
Fu, F., and Wang, Q. (2011). Removal of heavy metal ions from wastewaters: a review. J. Environ. Manag. 92, 407–418. doi:10.1016/j.jenvman.2010.11.011
Ge, Y., Cui, X., Liao, C., and Li, Z. (2017). Facile fabrication of green geopolymer/alginate hybrid spheres for efficient removal of Cu(II) in water: batch and column studies. Chem. Eng. 311, 126–134. doi:10.1016/j.cej.2016.11.079
Giovanella, P., Vieira, G. A. L., Otero, I. V. R., Pellizzer, E. P., Fontes, B. D., and Sette, L. D. (2020). Metal and organic pollutants bioremediation by extremophile microorganisms. J. Hazard Mater. 382, 14. doi:10.1016/j.cej.2016.11.079
Gorospe, C. M., Han, S.-H., Kim, S.-G., Park, J.-Y., Kang, C.-H., Jeong, J.-H., et al. (2013). Effects of different calcium salts on calcium carbonate crystal formation by Sporosarcina pasteurii KCTC 3558. Biotechnol. Bioproc. Eng. 18, 903–908. doi:10.1007/s12257-013-0030-0
Guo, B., Liu, B., Yang, J., and Zhang, S. (2017a). The mechanisms of heavy metal immobilization by cementitious material treatments and thermal treatments: a review. J. Environ. Manag. 193, 410–422. doi:10.1016/j.jenvman.2017.02.026
Guo, M.-Z., Ling, T.-C., and Poon, C. S. (2017b). Photocatalytic NOx degradation of concrete surface layers intermixed and spray-coated with nano-TiO2: influence of experimental factors. Cement Concr. Compos. 83, 279–289. doi:10.1016/j.cemconcomp.2017.07.022
Guo, X., Zhang, L., Huang, J., and Shi, H. (2017c). Detoxification and solidification of heavy metal of chromium using fly ash-based geopolymer with chemical agents. Conster. Build. Mater. 151, 394–404. doi:10.1016/j.conbuildmat.2017.05.199
Gurunathan, S., Kalishwaralal, K., Vaidyanathan, R., Venkataraman, D., Pandian, S. R., Muniyandi, J., et al. (2009). Biosynthesis, purification and characterization of silver nanoparticles using Escherichia coli. Colloids Surf. B. Biointerfaces. 74, 328–335. doi:10.1016/j.colsurfb.2009.07.048
Guzman, M., Dille, J., and Stephan, G. (2009). Synthesis of silver nanoparticles by chemical reduction method and their antibacterial activity. Int. J. Chem. Biomol. Eng. 2, 104–111. doi:10.7897/2230-8407.041024
Hallin, S., Philippot, L., Löffler, F. E., Sanford, R. A., and Jones, C. M. (2018). Genomics and ecology of novel N2O-reducing microorganisms. Trends Microbiol. 26, 43–55. doi:10.1016/j.tim.2017.07.003
Hani, N., Nawawy, O., Ragab, K. S., and Kohail, M. (2018). The effect of different water/binder ratio and nano-silica dosage on the fresh and hardened properties of self-compacting concrete. Conster. Build. Mater. 165, 504–513. doi:10.1016/j.conbuildmat.2018.01.045
Hashim, M. A., Mukhopadhyay, S., Sahu, J. N., and Sengupta, B. (2011). Remediation technologies for heavy metal contaminated groundwater. J. Environ. Manag. 92, 2355–2388. doi:10.1016/j.jenvman.2011.06.009
Hetherington, A. C., Borrion, A. L., Griffiths, O. G., and Mcmanus, M. C. (2014). Use of LCA as a development tool within early research: challenges and issues across different sectors. Int. J. Life Cycle Assess. 19, 130–143. doi:10.1007/s11367-013-0627-8
Heveran, C. M., Liang, L., Nagarajan, A., Hubler, M. H., Gill, R., Cameron, J. C., Cook, S. M., and Srubar, W. V. (2019). Engineered ureolytic microorganisms can tailor the morphology and nanomechanical properties of microbial-precipitated calcium carbonate. Sci. Rep. 9, 14721. doi:10.1038/s41598-019-51133-9
Heveran, C. M., Williams, S. L., Qiu, J., Artier, J., Hubler, M. H., Cook, S. M., et al. (2020). Biomineralization and successive regeneration of engineered living building materials. Matter. 2, 481–494. doi:10.1002/adma.201605903
Hibbert, E. G., and Dalby, P. A. (2005). Directed evolution strategies for improved enzymatic performance. Microb. Cell Factories. 4, 29. doi:10.1186/1475-2859-4-29
Ho, S. H., Chen, C. Y., Lee, D. J., and Chang, J. S. (2011). Perspectives on microalgal CO₂-emission mitigation systems--a review. Biotechnol. Adv. 29, 189–198. doi:10.1016/j.biotechadv.2010.11.001
Ho, Y. S., and Mckay, G. (1999). Pseudo-second order model for sorption processes. Process Biochem. 34, 451–465. doi:10.1016/S0032-9592(98)00112-5
Honary, S., Barabadi, H., Gharaei-Fathabad, E., and Naghibi, F. (2012). Green synthesis OF copper oxide nanoparticles using penicillium aurantiogriseum, penicillium citrinum and penicillium waksmanii. Dig. J. Nanomater. Bios. 7, 999–1005.
Hulkoti, N. I., and Taranath, T. C. (2014). Biosynthesis of nanoparticles using microbes- a review. Colloids Surf. B. Biointerfaces. 121, 474–483. doi:10.1016/j.colsurfb.2014.05.027
Hüsken, G., Hunger, M., and Brouwers, H. J. H. (2009). Experimental study of photocatalytic concrete products for air purification. Build. Environ. 44, 2463–2474. doi:10.1016/j.buildenv.2009.04.010
Iravani, S., and Varma, R. S. (2020). Bacteria in heavy metal remediation and nanoparticle biosynthesis. ACS Sustain. Chem. Eng. 8, 5395–5409. doi:10.1021/acssuschemeng.0c00292
Ivanov, V., Chu, J., and Stabnikov, V. (2015). “Basics of construction microbial biotechnology,” in Biotechnologies and biomimetics for civil engineering. Editors F. Pacheco Torgal, J.A. Labrincha, M.V. Diamanti, C.P. Yu, and H.K. Lee (Cham, Germany: Springer International Publishing), 21–56.
Jayapalan, A. R., Lee, B. Y., and Kurtis, K. E. (2013). Can nanotechnology be ‘green’? Comparing efficacy of nano and microparticles in cementitious materials. Cement Concr. Compos. 36, 16–24. doi:10.1016/j.cemconcomp.2012.11.002
Jha, A. K., Prasad, K., and Kulkarni, A. R. (2009). Synthesis of TiO2 nanoparticles using microorganisms. Colloids Surf. B Biointerfaces. 71, 226–229. doi:10.1016/j.colsurfb.2009.02.007
Ji, Z., and Pei, Y. (2019). Bibliographic and visualized analysis of geopolymer research and its application in heavy metal immobilization: a review. J. Environ. Manag. 231, 256–267. doi:10.1016/j.jenvman.2018.10.041
Joshaghani, A., Balapour, M., Mashhadian, M., and Ozbakkaloglu, T. (2020). Effects of nano-TiO2, nano-Al2O3, and nano-Fe2O3 on rheology, mechanical and durability properties of self-consolidating concrete (SCC): an experimental study. Conster. Build. Mater. 245, 118444. doi:10.3390/ma12213608
Judd, S., Van Den Broeke, L. J., Shurair, M., Kuti, Y., and Znad, H. (2015). Algal remediation of CO₂ and nutrient discharges: a review. Water Res. 87, 356–366. doi:10.1016/j.watres.2015.08.021
Juenger, M. C. G., Snellings, R., and Bernal, S. A. (2019). Supplementary cementitious materials: new sources, characterization, and performance insights. Cement Concrete Res. 122, 257–273. doi:10.1016/j.cemconres.2019.05.008
Juibari, M. M., Abbasalizadeh, S., Jouzani, G. S., and Noruzi, M. (2011). Intensified biosynthesis of silver nanoparticles using a native extremophilic Ureibacillus thermosphaericus strain. Mater. Lett. 65, 1014–1017. doi:10.1016/j.matlet.2010.12.056
Kalathil, S., Lee, J., and Cho, M. H. (2011). Electrochemically active biofilm-mediated synthesis of silver nanoparticles in water. Green Chem. 13, 1482–1485. doi:10.1039/C1GC15309A
Kalishwaralal, K., Deepak, V., Ramkumarpandian, S., Nellaiah, H., and Sangiliyandi, G. (2008). Extracellular biosynthesis of silver nanoparticles by the culture supernatant of Bacillus licheniformis. Mater. Lett. 62, 4411–4413. doi:10.1016/j.matlet.2008.06.051
Kamennaya, N. A., Ajo-Franklin, C. M., Northen, T., and Jansson, C. (2012). Cyanobacteria as biocatalysts for carbonate mineralization. Minerals. 2, 338–364. 10.3390/min2040338
Kang, C. H., Han, S. H., Shin, Y., Oh, S. J., and So, J. S. (2014). Bioremediation of Cd by microbially induced calcite precipitation. Appl. Biochem. Biotechnol. 172, 2907–2915. doi:10.1007/s12010-014-0737-1
Khezami, L., and Capart, R. (2005). Removal of chromium(VI) from aqueous solution by activated carbons: kinetic and equilibrium studies. J. Hazard Mater. 123, 223–231. doi:10.1016/j.jhazmat.2005.04.012
Khoshakhlagh, A., Nazari, A., and Khalaj, G. (2012). Effects of Fe2O3 nanoparticles on water permeability and strength assessments of high strength self-compacting concrete. J. Mater. Sci. Technol. 28, 73–82. 10.3389/fpls.2015.01263
Khotbehsara, M. M., Mohseni, E., Yazdi, M. A., Sarker, P., and Ranjbar, M. M. (2015). Effect of nano-CuO and fly ash on the properties of self-compacting mortar. Conster. Build. Mater. 94, 758–766. doi:10.1016/j.conbuildmat.2015.07.063
Kim, I. G., Jo, B. H., Kang, D. G., Kim, C. S., Choi, Y. S., and Cha, H. J. (2012). Biomineralization-based conversion of carbon dioxide to calcium carbonate using recombinant carbonic anhydrase. Chemosphere. 87, 1091–1096. doi:10.1016/j.chemosphere.2012.02.003
Kip, N., Fritz, C., Langelaan, E. S., Pan, Y., Bodrossy, L., Pancotto, V., et al. (2012). Methanotrophic activity and diversity in different Sphagnum magellanicum dominated habitats in the southernmost peat bogs of Patagonia. Biogeosciences. 9, 47–55. doi:10.5194/bg-9-47-2012
Kirthi, A. V., Rahuman, A. A., Rajakumar, G., Marimuthu, S., Santhoshkumar, T., Jayaseelan, C., et al. (2011). Biosynthesis of titanium dioxide nanoparticles using bacterium Bacillus subtilis. Mater. Lett. 65, 2745–2747. doi:10.1016/j.matlet.2011.05.077
Kumar, S., Dagar, V. K., Khasa, Y. P., and Kuhad, R. C. (2013). “Genetically modified microorganisms (GMOs) for bioremediation,” in Biotechnology for environmental management and resource recovery. Editors R.C. Kuhad, and A. Singh (India, SA: Springer India), 191–218.
Kumaresan, M., Vijai Anand, K., Govindaraju, K., Tamilselvan, S., and Ganesh Kumar, V. (2018). Seaweed Sargassum wightii mediated preparation of zirconia (ZrO. Microb. Pathog. 124, 311–315. doi:10.1016/j.micpath.2018.08.060
Kumari, D., Qian, X.-Y., Pan, X., Achal, V., Li, Q., and Gadd, G. M. (2016). “Chapter two - microbially-induced carbonate precipitation for immobilization of toxic metals,” in Advances in applied microbiology. Editors S. Sariaslani, and G.M. Gadd (Cambridge, MA, Academic Press), 79–108.
Labrenz, M., Druschel, G. K., Thomsen-Ebert, T., Gilbert, B., Welch, S. A., Kemner, K. M., et al. (2000). formation of sphalerite (ZnS) deposits in natural biofilms of sulfate-reducing bacteria. Science. 290, 1744–1747. doi:10.1126/science.290.5497.1744
Landrigan, P. J. (2017). Air pollution and health. The Lancet Public Health. 2, e4–e5. doi:10.1016/S0140-6736(17)32345-0
Li, S., Luo, S., and Guo, R. (2013). Efficiency of CO2 fixation by microalgae in a closed raceway pond. Bioresour. Technol. 136, 267–272. doi:10.1016/j.biortech.2013.03.025
Li, X., Xu, H., Chen, Z.-S., and Chen, G. (2011). Biosynthesis of nanoparticles by microorganisms and their applications. J. Nanomater. 2011, 270974. doi:10.1155/2011/270974
Li, Z., Han, B., Yu, X., Dong, S., Zhang, L., Dong, X., et al. (2017). Effect of nano-titanium dioxide on mechanical and electrical properties and microstructure of reactive powder concrete. Mater. Res. Express. 4, 095008. doi:10.1088/2053-1591/aa87db
Liang, L., Heveran, C., Liu, R., Gill, R. T., Nagarajan, A., Cameron, J., et al. (2018). Rational control of calcium carbonate precipitation by engineered Escherichia coli. ACS Synth. Biol. 7, 2497–2506. doi:10.1021/acssynbio.8b00194
Liu, H., Cheng, Y., Du, B., Tong, C., Liang, S., Han, S., et al. (2015). Overexpression of a novel thermostable and chloride-tolerant laccase from Thermus thermophilus sg0.5jp17-16 in Pichia pastoris and its application in synthetic dye decolorization. PloS One. 10, e0119833. doi:10.1371/journal.pone.0119833
Liu, N., Bond, G. M., Abel, A., Mcpherson, B. J., and Stringer, J. (2005). Biomimetic sequestration of CO2 in carbonate form: role of produced waters and other brines. Fuel Process. Technol. 86, 1615–1625. doi:10.17311/sciintl.2015.48.57
Madandoust, R., Mohseni, E., Mousavi, S. Y., and Namnevis, M. (2015). An experimental investigation on the durability of self-compacting mortar containing nano-SiO2, nano-Fe2O3 and nano-CuO. Conster. Build. Mater. 86, 44–50. doi:10.1016/j.conbuildmat.2015.03.100
Mania, D., Heylen, K., Van Spanning, R. J., and Frostegård, Å. (2016). Regulation of nitrogen metabolism in the nitrate-ammonifying soil bacterium Bacillus vireti and evidence for its ability to grow using N2 O as electron acceptor. Environ. Microbiol. 18, 2937–2950. doi:10.1111/1462-2920.13124
Marikani, D. K., Sangareswari, K., Suganya, P., Ganesan, R., and Rajarathinam, K. (2016). Biobased approach for the synthesis, characterization, optimization and application of silica nanoparticles by fungus Fusarium oxysporum. Pharm. Biol. Eval. 2, 223–233.
Mathema, V. B., Thakuri, B. C., and Sillanpää, M. (2011). Bacterial mer operon-mediated detoxification of mercurial compounds: a short review. Arch. Microbiol. 193, 837–844. doi:10.1007/s00203-011-0751-4
Mehta, S. K., and Gaur, J. P. (2005). Use of algae for removing heavy metal ions from wastewater: progress and prospects. Crit. Rev. Biotechnol. 25, 113–152. doi:10.1080/07388550500248571
Mindess, S. (2019). “6–resistance of concrete to destructive agencies,” in Lea's chemistry of cement and concrete. Editors P.C. Hewlett, and M. Liska. 5th Edn. (Cambridge, MA, Butterworth-Heinemann), 251–283.
Mindess, S., Young, J. F., and Darwin, D. (2003). Concrete. 2nd Edn. Upper Saddle River, NJ: Prentice Hall, 372.
Miyandehi, B. M., Feizbakhsh, A., Yazdi, M. A., Liu, Q.-F., Yang, J., and Alipour, P. (2016). Performance and properties of mortar mixed with nano-CuO and rice husk ash. Cement Concr. Compos. 74, 225–235. doi:10.1016/j.cemconcomp.2016.10.006
Mo, J., Zhang, Y., Xu, Q., Lamson, J. J., and Zhao, R. (2009). Photocatalytic purification of volatile organic compounds in indoor air: a literature review. Atmos. Environ. 43, 2229–2246. doi:10.1016/J.ATMOSENV.2009.01.034
Mohajerani, A., Burnett, L., Smith, J. V., Kurmus, H., Milas, J., Arulrajah, A., et al. (2019). Nanoparticles in construction materials and other applications, and implications of nanoparticle use. Materials. 12, 3052.
Monteiro, C. M., Castro, P. M., and Malcata, F. X. (2012). Metal uptake by microalgae: underlying mechanisms and practical applications. Biotechnol. Prog. 28, 299–311. doi:10.1002/btpr.1504
Nazari, A., and Riahi, S. (2010). The effect of TiO2 nanoparticles on water permeability and thermal and mechanical properties of high strength self-compacting concrete. Mater. Sci. Eng. 528, 756–763. doi:10.1016/j.msea.2010.09.074
Newsome, L., Morris, K., and Lloyd, J. R. (2014). The biogeochemistry and bioremediation of uranium and other priority radionuclides. Chem. Geol. 363, 164–184. doi:10.1016/j.chemgeo.2013.10.034
Noman, E., Al-Gheethi, A., Talip, B. A., Mohamed, R., and Kassim, A. H. (2019). Inactivating pathogenic bacteria in greywater by biosynthesized Cu/Zn nanoparticles from secondary metabolite of Aspergillus iizukae; optimization, mechanism and techno economic analysis. PloS One. 14, e0221522. doi:10.1371/journal.pone.0221522
Noorvand, H., Abang Ali, A. A., Demirboga, R., Farzadnia, N., and Noorvand, H. (2013). Incorporation of nano TiO2 in black rice husk ash mortars. Conster. Build. Mater. 47, 1350–1361. doi:10.1016/j.conbuildmat.2013.06.066
Osterwalder, N., Capello, C., Hungerbühler, K., and Stark, W. J. (2006). Energy consumption during nanoparticle production: how economic is dry synthesis? J. Nanopart. Res. 8, 1.
Pacheco-Torgal, F., and Jalali, S. (2011). Nanotechnology: advantages and drawbacks in the field of conster. Build. Mater. Conster. Build. Mater. 25, 582–590. doi:10.1016/j.conbuildmat.2010.07.009
Pacheco-Torgal, F., and Labrincha, J. A. (2013). The future of construction materials research and the seventh UN Millennium Development Goal: a few insights. Conster. Build. Mater. 40, 729–737. doi:10.1016/J.CONBUILDMAT.2012.11.007
Pal, A., and Paul, A. K. (2008). Microbial extracellular polymeric substances: central elements in heavy metal bioremediation. Indian J. Microbiol. 48, 49–64. doi:10.1007/s12088-008-0006-5
Paria, S., and Yuet, P. K. (2006). Solidification–stabilization of organic and inorganic contaminants using portland cement: a literature review. Environ. Rev. 14, 217–255. doi:10.1139/A06-004
Pellenq, R. J., Kushima, A., Shahsavari, R., Van Vliet, K. J., Buehler, M. J., Yip, S., et al. (2009). A realistic molecular model of cement hydrates. Proc. Natl. Acad. Sci. U.S.A. 106, 16102–16107. doi:10.1073/pnas.0902180106
Perez-Gonzalez, T., Jimenez-Lopez, C., Neal, A. L., Rull-Perez, F., Rodriguez-Navarro, A., Fernandez-Vivas, A., et al. (2010). Magnetite biomineralization induced by Shewanella oneidensis. Geochem. Cosmochim. Acta. 74, 967–979. doi:10.1016/j.gca.2009.10.035
Petronella, F., Truppi, A., Ingrosso, C., Placido, T., Striccoli, M., Curri, M. L., et al. (2017). Nanocomposite materials for photocatalytic degradation of pollutants. Catal. Today. 281, 85–100. doi:10.1016/j.cattod.2016.05.048
Porter, H., Dhami, N. K., and Mukherjee, A. (2017). Synergistic chemical and microbial cementation for stabilization of aggregates. Cement Concr. Compos. 83, 160–170. doi:10.1016/j.cemconcomp.2017.07.015
Puligilla, S., Chen, X., and Mondal, P. (2019). Does synthesized C-S-H seed promote nucleation in alkali activated fly ash-slag geopolymer binder? Mater. Struct. 52, 65. doi:10.1617/s11527-019-1368-3
Puligilla, S., Chen, X., and Mondal, P. (2018). Understanding the role of silicate concentration on the early-age reaction kinetics of a calcium containing geopolymeric binder. Conster. Build. Mater. 191, 206–215. doi:10.1016/j.conbuildmat.2018.09.184
Raghoebarsing, A. A., Smolders, A. J., Schmid, M. C., Rijpstra, W. I., Wolters-Arts, M., Derksen, J., et al. (2005). Methanotrophic symbionts provide carbon for photosynthesis in peat bogs. Nature. 436, 1153–1156. doi:10.1038/nature03802
Ramanan, R., Kannan, K., Sivanesan, S. D., Mudliar, S., Kaur, S., Tripathi, A. K., et al. (2009). Bio-sequestration of carbon dioxide using carbonic anhydrase enzyme purified from Citrobacter freundii. World J. Microbiol. Biotechnol. 25, 981–987. doi:10.1007/s11274-009-9975-8
Rasaki, S. A., Bingxue, Z., Guarecuco, R., Thomas, T., and Minghui, Y. (2019). Geopolymer for use in heavy metals adsorption, and advanced oxidative processes: a critical review. J. Clean. Prod. 213, 42–58. doi:10.1016/j.jclepro.2018.12.145
Rashad, A. M. (2013b). A synopsis about the effect of nano-Al2O3, nano-Fe2O3, nano-Fe3O4 and nano-clay on some properties of cementitious materials – a short guide for Civil Engineer. Mater. Des. 52, 143–157. doi:10.1016/j.matdes.2013.05.035
Rashad, A. M. (2013a). Effects of ZnO2, ZrO2, Cu2O3, CuO, CaCO3, SF, FA, cement and geothermal silica waste nanoparticles on properties of cementitious materials – a short guide for Civil Engineer. Conster. Build. Mater. 48, 1120–1133. doi:10.1016/j.conbuildmat.2013.06.083
Reches, Y. (2018). Nanoparticles as concrete additives: review and perspectives. Conster. Build. Mater. 175, 483–495. doi:10.1016/j.conbuildmat.2018.04.214
Ruan, S., Qiu, J., Weng, Y., Yang, Y., Yang, E.-H., Chu, J., and Unluer, C. (2019). The use of microbial induced carbonate precipitation in healing cracks within reactive magnesia cement-based blends. Cement Concrete Res. 115, 176–188. doi:10.1016/j.cemconres.2018.10.018
Sadhukhan, J., Joshi, N., Shemfe, M., and Lloyd, J. R. (2017). Life cycle assessment of sustainable raw material acquisition for functional magnetite bionanoparticle production. J. Environ. Manag. 199, 116–125. doi:10.1016/j.jenvman.2017.05.048
Salehizadeh, H., Yan, N., and Farnood, R. (2020). Recent advances in microbial CO2 fixation and conversion to value-added products. Chem. Eng. J. 390, 124584. doi:10.1016/j.cej.2020.124584
Sanna, A., Uibu, M., Caramanna, G., Kuusik, R., and Maroto-Valer, M. M. (2014). A review of mineral carbonation technologies to sequester CO2. Chem. Soc. Rev. 43, 8049–8080. doi:10.1039/c4cs00035h
Sato, T., and Diallo, F. (2010). Seeding effect of nano-CaCO3 on the hydration of tricalcium silicate. Transport. Res. Rec. 2141, 61–67. 10.3141/2141-11
Šavija, B., and Luković, M. (2016). Carbonation of cement paste: understanding, challenges, and opportunities. Conster. Build. Mater. 117, 285–301. doi:10.1016/j.conbuildmat.2016.04.138
Schaffner, R. (2004). Bioremediation offers an effective, ethical, and economical alternative to standard cleanups. Am. Water Works Assoc. J. 96, 22. doi:10.1002/j.1551-8833.2004.tb10519.x
Seifan, M., Samani, A. K., and Berenjian, A. (2016). Bioconcrete: next generation of self-healing concrete. Appl. Microbiol. Biotechnol. 100, 2591–2602. doi:10.1007/s00253-016-7316-z
Selvakumar, R., Seethalakshmi, N., Thavamani, P., Naidu, R., and Megharaj, M. (2014). Recent advances in the synthesis of inorganic nano/microstructures using microbial biotemplates and their applications. RSC Adv. 4, 52156–52169. doi:10.1039/C4RA07903E
Show, S., Tamang, A., Chowdhury, T., Mandal, D., and Chattopadhyay, B. (2015). Bacterial (BKH1) assisted silica nanoparticles from silica rich substrates: a facile and green approach for biotechnological applications. Colloids Surf. B Biointerfaces. 126, 245–250. doi:10.1016/j.colsurfb.2014.12.039
Singh, R., Paul, D., and Jain, R. K. (2006). Biofilms: implications in bioremediation. Trends Microbiol. 14, 389–397. doi:10.1016/j.tim.2006.07.001
Singh, R., Dong, H., Liu, D., Zhao, L., Marts, A. R., Farquhar, E., et al. (2015). Reduction of hexavalent chromium by the thermophilic methanogen Methanothermobacter thermautotrophicus. Geochem. Cosmochim. Acta. 148, 442–456. doi:10.1016/j.gca.2014.10.012
Sneha, K., Sathishkumar, M., Mao, J., Kwak, I. S., and Yun, Y. S. (2010). Corynebacterium glutamicum-mediated crystallization of silver ions through sorption and reduction processes. Chem.l Eng. J. 162, 989–996. doi:10.1016/j.cej.2010.07.006
Sobolev, K., Flores, I., Hermosillo, R., and Torres-Martinez, L. M. (2006). “Nanomaterials and nanotechnology for high-performance cement composites,” in Proceedings of ACI Session on “Nanotechnology of concrete: recent Developments and future perspectives”, Denver, USA, November 7, 2006 [abstract].
Soleimani, S., Ormeci, B., and Isgor, O. B. (2013b). Evaluation of E. coli biofilm as a protective barrier against microbiologically influenced deterioration of concrete (MICD) under mesophilic temperatures. Water Sci. Technol. 68, 303–310. doi:10.2166/wst.2013.252
Soleimani, S., Ormeci, B., and Isgor, O. B. (2013c). Growth and characterization of Escherichia coli DH5α biofilm on concrete surfaces as a protective layer against microbiologically influenced concrete deterioration (MICD). Appl. Microbiol. Biotechnol. 97, 1093–1102. doi:10.1007/s00253-012-4379-3
Soleimani, S., Isgor, O. B., and Ormeci, B. (2016). Effectiveness of E. coli biofilm on mortar to inhibit biodegradation by biogenic acidification. J. Mater. Civ. Eng. 28, 04015167. doi:10.1061/(ASCE)MT.1943-5533.0001440
Soleimani, S., Isgor, O. B., and Ormeci, B. (2013a). Resistance of biofilm-covered mortars to microbiologically influenced deterioration simulated by sulfuric acid exposure. Cement Concr. Res, 229–238. doi:10.1016/j.cemconres.2013.06.016
Souto-Martinez, A., Arehart, J. H., and Srubar, W. V. (2018a). Cradle-to-gate CO2e emissions vs. in situ CO2 sequestration of structural concrete elements. Energy Build. 167, 301–311. doi:10.1016/j.enbuild.2018.02.042
Souto-Martinez, A., Delesky, E. A., Foster, K. E. O., and Srubar, W. V. (2017). A mathematical model for predicting the carbon sequestration potential of ordinary portland cement (OPC) concrete. Conster. Build. Mater. 147, 417–427. doi:10.1016/j.conbuildmat.2017.04.133
Souto-Martinez, A., Sutley, E. J., Liel, A. B., and Srubar, W. V. (2018b). “Embodied carbon of wood and reinforced concrete structures under chronic and acute hazards,” in Embodied carbon in buildings: measurement, management, and mitigation. Editors F. Pomponi, C. De Wolf, and A. Moncaster (Cham, Germany: Springer International Publishing), 77–103.
Spasiano, D., Marotta, R., Malato, S., Fernandez-Ibañez, P., and Di Somma, I. (2015). Solar photocatalysis: materials, reactors, some commercial, and pre-industrialized applications. A comprehensive approach. Appl. Catal. B Environ. 170–171, 90–123. doi:10.1016/j.apcatb.2014.12.050
Stabnikov, V., Ivanov, V., and Chu, J. (2015). Construction Biotechnology: a new area of biotechnological research and applications. World J. Microbiol. Biotechnol. 31, 1303–1314. doi:10.1007/s11274-015-1881-7
Stabnikov, V., Naeimi, M., Ivanov, V., and Chu, J. (2011). Formation of water-impermeable crust on sand surface using biocement. Cement Concrete Res. 41, 1143–1149. doi:10.1016/j.cemconres.2011.06.017
Stępniewska, Z., and Kuźniar, A. (2013). Endophytic microorganisms—promising applications in bioremediation of greenhouse gases. Appl. Microbiol. Biotechnol. 97, 9589–9596. doi:10.1007/s00253-013-5235-9
Strini, A., Cassese, S., and Schiavi, L. (2005). Measurement of benzene, toluene, ethylbenzene and o-xylene gas phase photodegradation by titanium dioxide dispersed in cementitious materials using a mixed flow reactor. Appl. Catal. B Environ. 61, 90–97. doi:10.1016/j.apcatb.2005.04.009
Sun, L., Zhang, Z., and Dang, H. (2003). A novel method for preparation of silver nanoparticles. Mater. Lett. 57, 3874–3879. doi:10.1016/S0167-577X(03)00232-5
Suresh Kumar, K., Dahms, H. U., Won, E. J., Lee, J. S., and Shin, K. H. (2015). Microalgae - a promising tool for heavy metal remediation. Ecotoxicol. Environ. Saf. 113, 329–352. doi:10.1016/j.ecoenv.2014.12.019
Sydney, E. B., Sturm, W., De Carvalho, J. C., Thomaz-Soccol, V., Larroche, C., Pandey, A., et al. (2010). Potential carbon dioxide fixation by industrially important microalgae. Bioresour. Technol. 101, 5892–5896. doi:10.1016/j.biortech.2010.02.088
Takeuchi, F., Iwahori, K., Kamimura, K., Negishi, A., Maeda, T., and Sugio, T. (2001). Volatilization of mercury under acidic conditions from mercury-polluted soil by a mercury-resistant Acidithiobacillus ferrooxidans SUG 2-2. Biosci. Biotechnol. Biochem. 65, 1981–1986. doi:10.1271/bbb.65.1981
Tan, K. S., and Cheong, K. Y. (2013). Advances of Ag, Cu, and Ag–Cu alloy nanoparticles synthesized via chemical reduction route. J. Nanopart. Res. 15, 1537. doi:10.1007/s11051-013-1537-1
Tang, Q., Ge, Y.-Y., Wang, K.-T., He, Y., and Cui, X.-M. (2015). Preparation and characterization of porous metakaolin-based inorganic polymer spheres as an adsorbent. Mater. Des. 88, 1244–1249. doi:10.1016/j.matdes.2015.09.126
Teitzel, G. M., and Parsek, M. R. (2003). Heavy metal resistance of biofilm and planktonic Pseudomonas aeruginosa. Appl. Environ. Microbiol. 69, 2313–2320. doi:10.1128/AEM.69.4.2313-2320.2003
Thomas, J. J., Jennings, H. M., and Chen, J. J. (2009). Influence of nucleation seeding on the hydration mechanisms of tricalcium silicate and cement. J. Phys. Chem. C. 113, 4327–4334. doi:10.1021/jp809811w
Tiquia-Arashiro, S., and Rodrigues, D. F. (2016). Extremophiles: applications in nanotechnology, Cham, Switzerland, Springer International Publishing, 193.
Trejo-Arroyo, D. L., Acosta, K. E., Cruz, J. C., Valenzuela-Muniz, A. M., Vega-Azamar, R. E., and Jimenez, L. F. (2019). Influence of ZrO2 nanoparticles on the microstructural development of cement mortars with limestone aggregates. App. Sci.-Basel. 9, 12. doi:10.3390/app9030598
Van Paassen, L. A., Daza, C. M., Staal, M., Sorokin, D. Y., Van Der Zon, W., and Van Loosdrecht, M. C. M. (2010). Potential soil reinforcement by biological denitrification. Ecol. Eng. 36, 168–175. doi:10.1016/j.ecoleng.2009.03.026
Wang, J., Ersan, Y. C., Boon, N., and De Belie, N. (2016). Application of microorganisms in concrete: a promising sustainable strategy to improve concrete durability. Appl. Microbiol. Biotechnol. 100, 2993–3007. doi:10.1007/s00253-016-7370-6
Wang, J., Vandevyvere, B., Vanhessche, S., Schoon, J., Boon, N., and De Belie, N. (2017). Microbial carbonate precipitation for the improvement of quality of recycled aggregates. J. Clean. Prod. 156, 355–366. doi:10.1016/j.jclepro.2017.04.051
Wang, Y., Cai, Z., Sheng, S., Pan, F., Chen, F., and Fu, J. (2020). Comprehensive evaluation of substrate materials for contaminants removal in constructed wetlands. Sci. Total Environ. 701, 134736. doi:10.1016/j.scitotenv.2019.134736
White, C., and Gadd, G. M. (2000). Copper accumulation by sulfate-reducing bacterial biofilms. FEMS Microbiol. Lett. 183, 313–318. doi:10.1111/j.1574-6968.2000.tb08977.x
Wong, L. S. (2015). Microbial cementation of ureolytic bacteria from the genus Bacillus: a review of the bacterial application on cement-based materials for cleaner production. J. Clean. Prod. 93, 5–17. doi:10.1016/j.jclepro.2015.01.019
Wong, L. S., Oweida, A. F. M., Kong, S. Y., Iqbal, D. M., and Regunathan, P. (2020). The surface coating mechanism of polluted concrete by Candida ethanolica induced calcium carbonate mineralization. Conster. Build. Mater. 257, 119482. doi:10.1016/j.conbuildmat.2020.119482
Wu, F., Zhou, Z., and Hicks, A. L. (2019). Life cycle impact of titanium dioxide nanoparticle synthesis through physical, chemical, and biological routes. Environ. Sci. Tech. 53, 4078–4087. doi:10.1021/acs.est.8b06800
Wu, Y., Li, T., and Yang, L. (2012). Mechanisms of removing pollutants from aqueous solutions by microorganisms and their aggregates: a review. Bioresour. Technol. 107, 10–18. doi:10.1016/j.biortech.2011.12.088
Yuan, H., Shi, Y., Xu, Z., Lu, C., Ni, Y., and Lan, X. (2013). Influence of nano-ZrO2 on the mechanical and thermal properties of high temperature cementitious thermal energy storage materials. Conster. Build. Mater. 48, 6–10. doi:10.1016/j.conbuildmat.2013.06.088
Zhang, G. (2016). Biomineralization on the wavy substrate: shape transition of nacreous tablets from pyramids of amorphous nanoparticles to dome-capped prisms of single crystals. Acta Biomater. 36, 277–285. doi:10.1016/j.actbio.2016.03.018
Zhang, R., Cheng, X., Hou, P., and Ye, Z. (2015). Influences of nano-TiO2 on the properties of cement-based materials: hydration and drying shrinkage. Conster. Build. Mater. 81, 35–41. doi:10.1016/J.CONBUILDMAT.2015.02.003
Zumft, W. G., and Kroneck, P. M. (2006). Respiratory transformation of nitrous oxide (N2O) to dinitrogen by bacteria and archaea. Adv. Microb. Physiol. 52, 107–227. doi:10.1016/S0065-2911(06)52003-X
Keywords: nanotechnology, heavy metal remediation, Sustainability, cementitious materials, construction biotechnology
Citation: Chen X, Charrier M and Srubar WV (2021) Nanoscale Construction Biotechnology for Cementitious Materials: A Prospectus. Front. Mater. 7:594989. doi: 10.3389/fmats.2020.594989
Received: 14 August 2020; Accepted: 04 November 2020;
Published: 29 January 2021.
Edited by:
Chang-Mou Wu, National Taiwan University of Science and Technology, TaiwanReviewed by:
Masoud Mozafari, University of Toronto, CanadaUmapathi Reddicherla, Inha University, South Korea
Copyright © 2021 Chen, Charrier and Srubar III. This is an open-access article distributed under the terms of the Creative Commons Attribution License (CC BY). The use, distribution or reproduction in other forums is permitted, provided the original author(s) and the copyright owner(s) are credited and that the original publication in this journal is cited, in accordance with accepted academic practice. No use, distribution or reproduction is permitted which does not comply with these terms.
*Correspondence: Wil V. Srubar III, d3NydWJhckBjb2xvcmFkby5lZHU=