- Zernike Institute for Advanced Materials, University of Groningen, Groningen, Netherlands
In recent years, there has been a growing interest in spin-orbit torques (SOTs) for manipulating the magnetization in nonvolatile magnetic memory devices. SOTs rely on the spin-orbit coupling of a nonmagnetic material coupled to a ferromagnetic layer to convert an applied charge current into a torque on the magnetization of the ferromagnet (FM). Transition metal dichalcogenides (TMDs) are promising candidates for generating these torques with both high charge-to-spin conversion ratios, and symmetries and directions which are efficient for magnetization manipulation. Moreover, TMDs offer a wide range of attractive properties, such as large spin-orbit coupling, high crystalline quality and diverse crystalline symmetries. Although numerous studies were published on SOTs using TMD/FM heterostructures, we lack clear understanding of the observed SOT symmetries, directions, and strengths. In order to shine some light on the differences and similarities among the works in literature, in this mini-review we compare the results for various TMD/FM devices, highlighting the experimental techniques used to fabricate the devices and to quantify the SOTs, discussing their potential effect on the interface quality and resulting SOTs. This enables us to both identify the impact of particular fabrication steps on the observed SOT symmetries and directions, and give suggestions for their underlying microscopic mechanisms. Furthermore, we highlight recent progress of the theoretical work on SOTs using TMD heterostructures and propose future research directions.
Introduction
Spin-orbit torques (SOTs) are promising candidates for effective manipulation of magnetization through electric currents with applications in nonvolatile magnetic memory and logic devices. SOTs convert an electric current into a magnetic torque in non-magnetic/ferromagnetic heterostructure, i.e., an electric current through the stack can modulate the direction of the ferromagnet’s magnetization (Gambardella and Miron, 2011; Manchon et al., 2019). Devices showing large SOT efficiencies usually rely on a nonmagnetic material with large spin-orbit coupling in contact with a ferromagnet (FM). Transition metal dichalcogenides (TMDs), with chemical formula MX2, where M is a transition metal (e.g., Mo, and W) and X a chalcogen element (e.g., S and Se), can provide large spin-orbit coupling and pristine surfaces which can result in a more intimate contact between the TMD and the FM layer. Furthermore, this family of materials offers a wide range of electronic and crystalline properties and symmetries. Although numerous articles were published on SOTs in TMD/ferromagnetic heterostructures, a clear understanding of the different mechanisms underlying observed SOTs remain yet to be understood.
In this mini-review, we give an overview of the recent progress on SOTs in TMD/FM heterostructures. Materials with high charge-to-spin conversion efficiencies, such as WTe2 and TaTe2 (Safeer et al., 2019; Zhao et al., 2020; Hoque et al., 2020), are often considered as good candidates for large SOT efficiencies. However, large charge-to-spin conversion efficiencies are no guarantee for large SOT efficiencies, as SOTs are often an emergent phenomenon, depending on proximity effects (spin-orbit coupling and magnetic exchange), wavefunction overlap, and interface spin transparency (spin mixing conductance) as well. Indeed, the observed torques in TMD/FM heterostructures cannot always be explained by well-known effects such as the bulk spin Hall effect (SHE) (Dyakonov and Perel, 1971; Hirsch, 1999; Sinova et al., 2015) or the interfacial Rashba-Edelstein Effect (REE) (Edelstein, 1990; Ganichev et al., 2002; Kato et al., 2004; Mihai Miron et al., 2010; Ganichev et al., 2016) (Figure 1), indicating that other mechanisms involving material specific properties or interfacial effects are into play. This is supported by recent works suggesting that both the type of ferromagnetic layer (Dolui and Nikolic, 2020; Go and Lee, 2020) and the interface properties between the TMD and the ferromagnetic layer (Amin et al., 2020; Sousa et al., 2020; Go et al., 2020) (Sahoo et al., 2020; Kumar et al., 2020; Xue et al. 2020) are of paramount importance for the observed SOTs, allowing for enhanced and unconventional SOTs.
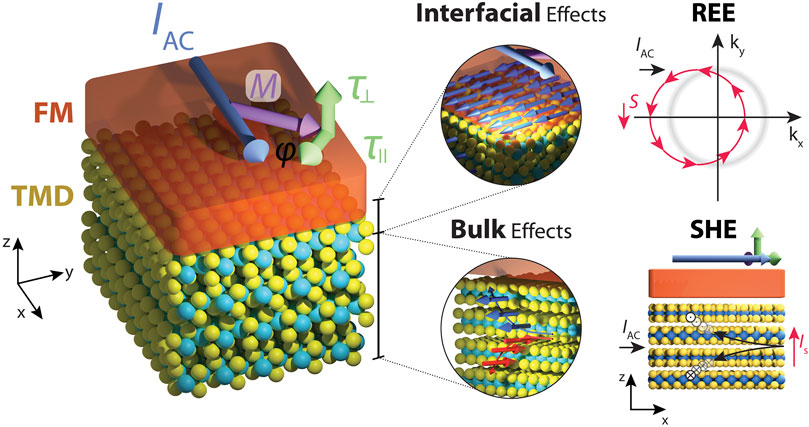
FIGURE 1. Schematics of SOTs in TMD/FM heterostructures. A charge current, usually oscillating at low (RF) frequencies for SHH (ST-FMR) measurements is applied along a device consisting of a TMD layer and a FM. The magnetization of the FM layer, oriented along an external magnetic field, observes a current-induced SOT in-plane (
To describe to different torques, we use the notation in terms of odd
Discussion on Recent Progress
The field of SOTs using TMD-based devices has been rapidly developed in the past 5 years. Experimental studies have used different TMD sources (e.g., mechanical exfoliation or chemical vapor deposition, CVD), FM materials, deposition methods (e.g., sputtering or electron-beam evaporation), and measurement techniques, namely second-harmonic Hall (SHH) (Garello et al., 2013; Hayashi et al., 2014; Avci et al., 2014; Ghosh et al., 2017) or spin-torque ferromagnetic resonance (ST-FMR) (Liu et al., 2011; Fang et al., 2011; Berger et al., 2018). So far, it is unclear how these different techniques and procedures affect the measured SOTs.
In this section, we discuss the results for semiconducting, semi-metallic and metallic TMDs, giving an overview of their fabrication and measurement techniques (Table 1). Comparing the TMDs in this way allows us to pinpoint important differences and similarities in the observed torques.
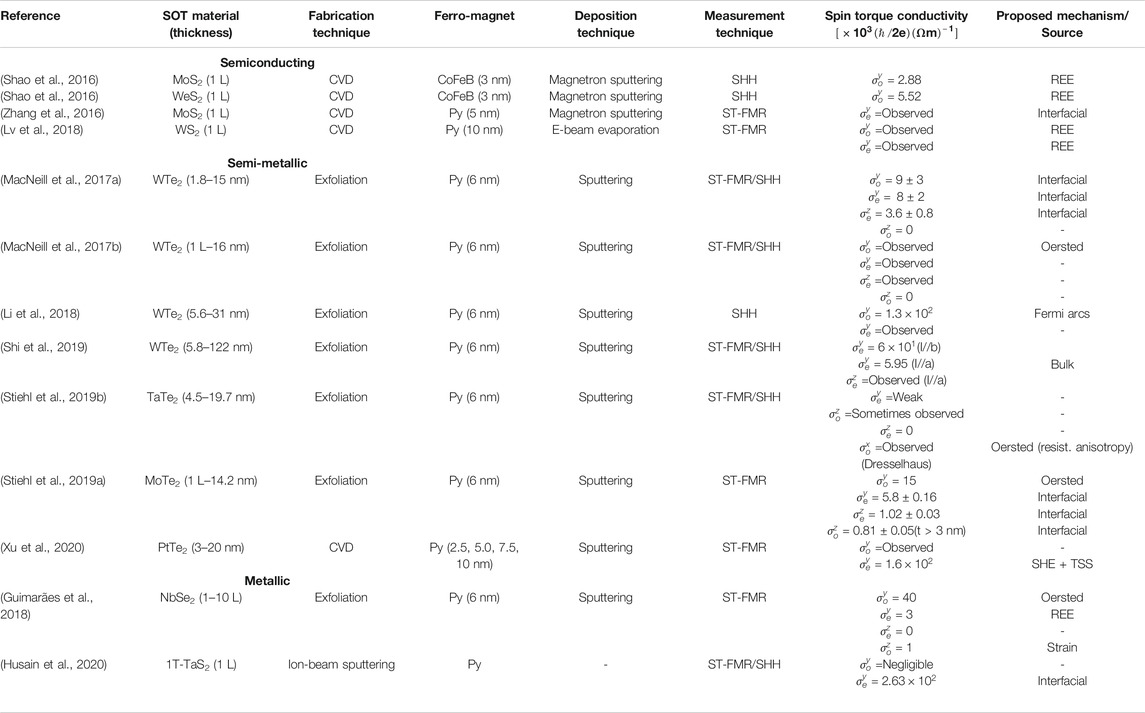
TABLE 1. Recent studies on TMD/FM heterostructures with their fabrication techniques and spin torque conductivities.
Semi-Conducting TMDs
Shao et al. were one of the first to examine SOTs in TMD/FM heterostructures (Shao et al., 2016). There, SOTs were quantified by the non-resonant SHH measurements on monolayer (1L) MoS2 and WSe2 coupled with CoFeB (3 nm). They observed a temperature independent out-of-plane FL torque
Interestingly, in a concurrent work, Zhang et al. obtained different results using a high-frequency technique, ST-FMR, on 1L-MoS2/Permalloy (Ni80Fe20 – Py) 5 nm (Zhang et al., 2016). There, they identified an in-plane DL
The discrepancy between results for MoS2/FM bilayers suggests that not only the spin-orbit material but also the type of ferromagnetic material (CoFeB vs Py) can play a significant role in the observed torques. This is theoretically substantiated in a recent work (Dolui and Nikolic, 2020), where calculations on MoSe2/Co, WSe2/Co and TaSe2/Co heterostructures were performed. They find that the hybridization of the Co wavefunctions with those of the TMDs leads to dramatic transmutation of the electronic and spin structure of the Co layers, even within eight layers away from the interface. This suggests that injecting unpolarized spin currents in these spin-orbit-proximitized layers of Co generates nonequilibrium spin densities, which in turn leads to a nonzero local torque on the magnetization. Both the spin polarization direction and magnitude were shown to differ between the different TMDs and complex spin textures were obtained for the spin-orbit-proximitized layers. These results indicate that the FM material can play an active role in the type of SOTs observed. Moreover, recent theoretical works (Sousa et al., 2020) pointed out that different scattering mechanisms lead to different torque symmetries, indicating that the sample quality, symmetry and nature of scatterers also plays a role here. Different FM materials in FM/TMD heterostructures might therefore exhibit different SOTs as was the case for Shao et al. and Zhang et al.
More recently, WS2 was studied by Lv et al. in a 1L-WS2/Py (10 nm) heterostructure (Lv et al., 2018) using CVD-grown WS2 and electron-beam evaporated Py layer. The authors observe both a DL torque
Semi-Metallic TMDs
In addition to semiconducting TMDs, a variety of semi-metallic TMDs have been studied, with special focus given to low-symmetry crystals. A particularly interesting candidate is WTe2, belonging to space group
MacNeill et al. were the first to examine SOTs using WTe2 (MacNeill et al., 2017a). Using ST-FMR, the authors observed
Subsequent studies indicated a strong temperature dependence (
More recently, WTe2/Py heterostructures have been shown to be very efficient for current-induced in-plane magnetization switching, with switching current densities in the order of 105 A/cm2 (Shi et al., 2019). In the same work, the authors also reported a thickness dependence on the spin Hall efficiency in WTe2, with larger values at higher thicknesses. However, the ST-FMR results show a significant frequency dependence and the role of artifacts such as skin-depth effects could not be ruled out. Nevertheless, the low threshold for current-induced magnetization switching indicates a promising direction for TMDs in future applications. Interestingly, these structures have also shown the presence of a Dzyaloshinskii-Moriya interaction, an essential ingredient for chiral magnetism.
The anisotropic in-plane conductivity in low-symmetry crystals can also impact SOTs. Results on TaTe2/Py heterostructures have shown SOTs with Dresselhaus-like symmetries (
Interestingly, both TaTe2 and WTe2 have shown to induce an in-plane magnetic anisotropy on Py, indicating a strong interaction between the semi-metallic TMDs and the FM layer. The anisotropy induced by WTe2 was shown to be about 10 s of mT and one order of magnitude larger than the one induced by TaTe2. Additionally, the two TMDs induced anisotropy in different directions with respect to their crystal orientations, hinting toward the dependence of the induced magnetic anisotropy and the electronic structure of the TMD.
Another interesting semi-metallic TMD is
In addition to the out-of-plane DL torque
More recently, PtTe2/Py devices (Xu et al., 2020) have shown a high spin-torque conductivity for the in-plane DL torque
Metallic TMDs
Despite offering stronger spin-orbit interaction and higher conductivity, metallic TMDs have received less attention than their semi-metallic and semiconducting counterparts. To date, only two experimental studies have been reported (Guimarães et al., 2018; Husain et al., 2020).
Thickness dependent ST-FMR measurements on NbSe2 (1–10 layers)/Py heterostructures revealed an in-plane DL torque
In addition to the SOTs with conventional symmetries, an in-plane FL torque
A large spin-torque conductivity of
Conclusion
In this review, we have given an overview of the current status of the field of SOTs in TMD/FM heterostructures. A multitude of SOT symmetries, magnitudes and directions were observed, which could not always be explained by well-known effects such as the SHE and REE. Different mechanisms that do not rely on a large spin-orbit coupling, such as anisotropic in-plane conductivity and uniaxial strain, can also play an important role. Additionally, interfacial effects such as spin-orbit filtering, spin-orbit precession and spin-momentum locking in topological surface states may affect the observed torques. In combination with the large torque conductivities obtained at clean interfaces, this suggests that the TMD/FM interface quality is of paramount importance for both the torque magnitude and direction. Lastly, the ferromagnetic layer, often considered to play a passive role, can have a significant effect on the observed SOTs due to changes of the electronic structure and intermixing at the interface. Dzyaloshinskii–Moriya interaction (DMI) has been shown to arise at TMD/FM interfaces demonstrating a strong interaction between these materials (Kumar et al., 2020; Shi et al., 2019; Wu et al., 2020). The large interfacial DMI in these heterostructures could be explored in future devices combining chiral magnetic structures and SOTs.
Although the crystal symmetry allows for a reasonable prediction of the allowed SOTs, a better understanding of the underlying microscopic mechanisms is key in qualitatively explaining the observed SOTs. In this regard, thickness dependent measurements provide a tool to better differentiate bulk effects from interfacial effects. However, as the contributions of different effects are measured all at once, it remains difficult to distinguish the numerous mechanisms underlying the torques with the current experimental techniques. To clarify the role of the ferromagnetic layer, a variety of devices with different FM materials should be fabricated.
Van der Waals heterostructures composed of TMDs, two-dimensional magnetic materials and graphene should allow for the study of SOTs at the ultimate thickness. Due to their small thickness, in addition to possibly reducing the device footprint, atomically-thin materials are more susceptible to external stimuli, such as gate-voltages, strain and illumination. Along these lines, interesting predictions point to the modulation of SOT and magnetization by gate-voltages in these structures (Dolui et al., 2019; Zollner et al., 2020). The exploration of gate-tunable SOTs in TMD/FM heterostructures could serve as a first step toward non-volatile data processing and storage as well as processing-in-memory applications. By giving an overview of the current status of the field, we hope to facilitate progress on elucidating the different underlying physical mechanisms for the SOTs.
Author Contributions
Both authors compiled the studies and wrote the manuscript.
Conflict of Interest
The authors declare that the research was conducted in the absence of any commercial or financial relationships that could be construed as a potential conflict of interest.
Acknowledgments
We acknowledge funding from the Dutch Research Council (NWO) Start-Up Grant (STU.019.014), the European Union Horizon 2020 research and innovation program under grant agreements No 696656 and 785219 (Graphene Flagship Core 2 and Core 3), and the Zernike Institute for Advanced Materials.
References
Amin, V. P., Haney, P. M., and Stiles, M. D. (2020). Interfacial spin-orbit torques. arXiv:2008.01182.
Amin, V. P., and Stiles, M. D. (2016a). Spin transport at interfaces with spin-orbit coupling: Formalism. Phys. Rev. B 94, 104419. doi:10.1103/PhysRevB.94.104419
Amin, V. P., and Stiles, M. D. (2016b). Spin transport at interfaces with spin-orbit coupling: Phenomenology. Phys. Rev. B 94, 104420. doi:10.1103/PhysRevB.94.104420
Avci, C. O., Garello, K., Gabureac, M., Ghosh, A., Fuhrer, A., Alvarado, S. F., et al. (2014). Interplay of spin-orbit torque and thermoelectric effects in ferromagnet/normal-metal bilayers. Phys. Rev. B 90, 224427. doi:10.1103/PhysRevB.90.224427
Berger, A. J., Edwards, E. R. J., Nembach, H. T., Karenowska, A. D., Weiler, M., and Silva, T. J. (2018). Inductive detection of fieldlike and dampinglike ac inverse spin-orbit torques in ferromagnet/normal-metal bilayers. Phys. Rev. B 97, 094407. doi:10.1103/PhysRevB.97.094407
Bonell, F., Goto, M., Sauthier, G., Sierra, J. F., Figueroa, A. I., Costache, M. V., et al. (2020). Control of spin-orbit torques by interface engineering in topological insulator heterostructures. Nano Lett. 20, 5893–5899. doi:10.1021/acs.nanolett.0c01850
Clark, O. J., Neat, M. J., Okawa, K., Bawden, L., Marković, I., Mazzola, F., et al. (2018). Fermiology and superconductivity of topological surface states in PdTe2. Phys. Rev. Lett. 120, 1–7. doi:10.1103/PhysRevLett.120.156401
Dieny, B., Prejbeanu, I. L., Garello, K., Gambardella, P., Freitas, P., Lehndorff, R., et al. (2019). Opportunities and challenges for spintronics in the microelectronic industry. arXiv:1908.10584.
Dolui, K., and Nikolic, B. K. (2020). Spin-orbit-proximitized ferromagnetic metal by monolayer transition metal dichalcogenide: atlas of spectral functions, spin textures and spin-orbit torques in Co/MoSe2, Co/WSe2 and Co/TaSe2 heterostructures. Phys. Rev. Mater. 4, 104007.
Dolui, K., Petrovic, M. D., Zollner, K., Plechac, P., Fabian, J., and Nikolic, B. K. (2019). First-principles theory of proximity spin-orbit torque on a two-dimensional magnet: current-driven antiferromagnet-to-ferromagnet reversible transition in bilayer CrI3. Nano Lett. 20, 2288.
Dyakonov, M. I., and Perel, V. I. (1971). Current-induced spin orientation of electrons in semiconductors. Phys. Lett. 35, 459–460. doi:10.1016/0375-9601(71)90196-4
Edelstein, V. M. (1990). Spin polarization of conduction electrons induced by electric current in two-dimensional asymmetric electron systems. Solid State Commun. 73, 233–235. doi:10.1016/0038-1098(90)90963-C
Fan, Y., Kou, X., Upadhyaya, P., Shao, Q., Pan, L., Lang, M., et al. (2016). Electric-field control of spin-orbit torque in a magnetically doped topological insulator. Nat. Nanotechnol. 11, 352–359. doi:10.1038/nnano.2015.294
Fang, D., Kurebayashi, H., Wunderlich, J., Výborný, K., Zârbo, L. P., Campion, R. P., et al. (2011). Spin-orbit-driven ferromagnetic resonance. Nat. Nanotechnol. 6, 413–417. doi:10.1038/nnano.2011.68
Filianina, M., Hanke, J.-P., Lee, K., Han, D.-S., Jaiswal, S., Rajan, A., et al. (2020). Electric-field control of spin-orbit torques in perpendicularly magnetized W/CoFeB/MgO films. Phys. Rev. Lett. 124, 217701. doi:10.1103/PhysRevLett.124.217701
Gambardella, P., and Miron, I. M. (2011). Current-induced spin-orbit torques. Phil. Trans. R. Soc. A. 369, 3175–3197. doi:10.1098/rsta.2010.0336
Ganichev, S. D., Ivchenko, E. L., Bel'kov, V. V., Tarasenko, S. A., Sollinger, M., Weiss, D., et al. (2002). Spin-galvanic effect. Nature 417, 153–156. doi:10.1038/417153a
Garello, K., Miron, I. M., Avci, C. O., Freimuth, F., Mokrousov, Y., Blügel, S., et al. (2013). Symmetry and magnitude of spin-orbit torques in ferromagnetic heterostructures. Nat. Nanotechnol. 8, 587–593. doi:10.1038/nnano.2013.145
Ghosh, A., Garello, K., Avci, C. O., Gabureac, M., and Gambardella, P. (2017). Interface-enhanced spin-orbit torques and current-induced magnetization switching of Pd/Co/AlOx layers. Phys. Rev. Applied. 7, 014004. doi:10.1103/PhysRevApplied.7.014004
Go, D., Freimuth, F., Hanke, J.-P., Xue, F., Gomonay, O., Lee, K.-J., et al. (2020). Theory of current-induced angular momentum transfer dynamics in spin-orbit coupled systems. Phys. Rev. Res. 2, 033401.
Go, D., and Lee, H.-W. (2020). Orbital torque: torque generation by orbital current injection. Phys. Rev. Research. 2, 013177. doi:10.1103/PhysRevResearch.2.013177
Guimarães, M. H. D., Stiehl, G. M., Macneill, D., Reynolds, N. D., Ralph, D. C., Guimaraes, M. H. D., et al. (2018). Spin-orbit torques in NbSe2/permalloy bilayers. Nano Lett. 18, 1311–1316. doi:10.1021/acs.nanolett.7b04993
Haney, P. M., Lee, H.-W., Lee, K.-J., Manchon, A., and Stiles, M. D. (2013). Current induced torques and interfacial spin-orbit coupling: semiclassical modeling. Phys. Rev. B 87, 174411. doi:10.1103/PhysRevB.87.174411
Hayashi, M., Kim, J., Yamanouchi, M., and Ohno, H. (2014). Quantitative characterization of the spin-orbit torque using harmonic Hall voltage measurements. Phys. Rev. B 89, 144425. doi:10.1103/PhysRevB.89.144425
Hirsch, J. E. (1999). Spin Hall effect. Phys. Rev. Lett. 83, 1834–1837. doi:10.1103/PhysRevLett.83.1834
Hoque, A. M., Khokhriakov, D., Karpiak, B., and Dash, S. P. (2020). Charge-spin conversion in layered semimetal TaTe2 and spin injection in van der Waals heterostructures. Phys. Rev. Research. 2, 033204. doi:10.1103/PhysRevResearch.2.033204
Husain, S., Chen, X., Gupta, R., Behera, N., Kumar, P., Edvinsson, T., et al. (2020). Damping-like torque in monolayer 1T-TaS2. aarXiv:2004.02649.
Kato, Y. K., Myers, R. C., Gossard, A. C., and Awschalom, D. D. (2004). Current-induced spin polarization in strained semiconductors. Phys. Rev. Lett. 93, 176601. doi:10.1103/PhysRevLett.93.176601
Kumar, A., Chaurasiya, A. K., Chowdhury, N., Mondal, A. K., Bansal, R., Barvat, A., et al. (2020). Direct measurement of interfacial Dzyaloshinskii-Moriya interaction at the MoS2/Ni80Fe20 interface. Appl. Phys. Lett. 116, 232405. doi:10.1063/5.0009828
Kurebayashi, H., Sinova, J., Fang, D., Irvine, A. C., Skinner, T. D., Wunderlich, J., et al. (2014). An antidamping spin-orbit torque originating from the Berry curvature. Nat. Nanotechnol. 9, 211–217. doi:10.1038/nnano.2014.15
Li, P., Wen, Y., He, X., Zhang, Q., Xia, C., Yu, Z.-M., et al. (2017). Evidence for topological type-II Weyl semimetal WTe2. Nat. Commun. 8, 2150. doi:10.1038/s41467-017-02237-1
Li, P., Wu, W., Wen, Y., Zhang, C., Zhang, J., Zhang, S., et al. (2018). Spin-momentum locking and spin-orbit torques in magnetic nano-heterojunctions composed of Weyl semimetal WTe2. Nat. Commun. 9, 1–10. doi:10.1038/s41467-018-06518-1
Li, X., Casamento, J., Dang, P., Zhang, Z., Afuye, O., Mei, A. B., et al. (2020). Spin-orbit torque field-effect transistor (SOTFET): proposal for a magnetoelectric memory. Appl. Phys. Lett. 116, 242405. doi:10.1063/5.0002909
Liu, L., Moriyama, T., Ralph, D. C., and Buhrman, R. A. (2011). Spin-torque ferromagnetic resonance induced by the spin Hall effect. Phys. Rev. Lett. 106, 036601. doi:10.1103/PhysRevLett.106.036601
Lv, W., Jia, Z., Wang, B., Lu, Y., Luo, X., Zhang, B., et al. (2018). Electric-field control of spin-orbit torques in WS2/permalloy bilayers. ACS Appl. Mater. Interfaces. 10, 2843–2849. doi:10.1021/acsami.7b16919
MacNeill, D., Stiehl, G. M., Guimaraes, M. H. D., Buhrman, R. A., Park, J., and Ralph, D. C. (2017a). Control of spin-orbit torques through crystal symmetry in WTe2/ferromagnet bilayers. Nat. Phys. 13, 300–305. doi:10.1038/nphys3933
MacNeill, D., Stiehl, G. M., Guimarães, M. H. D., Reynolds, N. D., Buhrman, R. A., and Ralph, D. C. (2017b). Thickness dependence of spin-orbit torques generated by WTe2. Phys. Rev. B 96, 1–8. doi:10.1103/PhysRevB.96.054450
Manchon, A., Železný, J., Miron, I. M., Jungwirth, T., Sinova, J., Thiaville, A., et al. (2019). Current-induced spin-orbit torques in ferromagnetic and antiferromagnetic systems. Rev. Mod. Phys. 91, 035004. doi:10.1103/RevModPhys.91.035004
Mellnik, A. R., Lee, J. S., Richardella, A., Grab, J. L., Mintun, P. J., Fischer, M. H., et al. (2014). Spin-transfer torque generated by a topological insulator. Nature 511, 449–451. doi:10.1038/nature13534
Mihai Miron, I., Gaudin, G., Auffret, S., Rodmacq, B., Schuhl, A., Pizzini, S., et al. (2010). Current-driven spin torque induced by the Rashba effect in a ferromagnetic metal layer. Nat. Mater. 9, 230–234. doi:10.1038/nmat2613
Miron, I. M., Garello, K., Gaudin, G., Zermatten, P.-J., Costache, M. V., Auffret, S., et al. (2011). Perpendicular switching of a single ferromagnetic layer induced by in-plane current injection. Nature 476, 189–193. doi:10.1038/nature10309
Nguyen, M.-H., Ralph, D. C., and Buhrman, R. A. (2016). Spin torque study of the spin Hall conductivity and spin diffusion length in platinum thin films with varying resistivity. Phys. Rev. Lett. 116, 126601. doi:10.1103/PhysRevLett.116.126601
Ramaswamy, R., Lee, J. M., Cai, K., and Yang, H. (2018). Recent advances in spin-orbit torques: moving towards device applications. Appl. Phys. Rev. 5, 031107. doi:10.1063/1.5041793
Safeer, C. K., Ontoso, N., Ingla-Aynés, J., Herling, F., Pham, V. T., Kurzmann, A., et al. (2019). Large multidirectional spin-to-charge conversion in low-symmetry semimetal MoTe2 at room temperature. Nano Lett. 19, 8758–8766. doi:10.1021/acs.nanolett.9b03485
Sahoo, K. R., Chakravarthy, T. P., Sharma, R., Bawari, S., Mundlia, S., Sasmal, S., et al. (2020). Probing proximity‐tailored high spin-orbit coupling in 2D materials. Adv Quantum Tech. 3, 2000042. doi:10.1002/qute.202000042
Shao, Q., Yu, G., Lan, Y.-W., Shi, Y., Li, M.-Y., Zheng, C., et al. (2016). Strong rashba-edelstein effect-induced spin-orbit torques in monolayer transition metal dichalcogenide/ferromagnet bilayers. Nano Lett. 16, 7514–7520. doi:10.1021/acs.nanolett.6b03300
Shi, S., Liang, S., Zhu, Z., Cai, K., Pollard, S. D., Wang, Y., et al. (2019). All-electric magnetization switching and Dzyaloshinskii-Moriya interaction in WTe2/ferromagnet heterostructures. Nat. Nanotechnol. 14, 945–949. doi:10.1038/s41565-019-0525-8
Sinova, J., Valenzuela, S. O., Wunderlich, J., Back, C. H., and Jungwirth, T. (2015). Spin Hall effects. Rev. Mod. Phys. 87, 1213–1260. doi:10.1103/RevModPhys.87.1213
Sousa, F. J., Tatara, G., and Ferreira, A. (2020). Emergent spin-orbit torques in two-dimensional material/ferromagnet interfaces. arXiv:2005.09670.
Stiehl, G. M., Li, R., Gupta, V., Baggari, I. E., Jiang, S., Xie, H., et al. (2019a). Layer-dependent spin-orbit torques generated by the centrosymmetric transition metal dichalcogenide β−MoTe2. Phys. Rev. B 100, 184402. doi:10.1103/PhysRevB.100.184402
Stiehl, G. M., MacNeill, D., Sivadas, N., El Baggari, I., Guimarães, M. H. D., Reynolds, N. D., et al. (2019b). Current-induced torques with dresselhaus symmetry due to resistance anisotropy in 2D materials. ACS Nano. 13, 2599–2605. doi:10.1021/acsnano.8b09663
Wang, Y., Deorani, P., Banerjee, K., Koirala, N., Brahlek, M., Oh, S., et al. (2015). Topological surface states originated spin-orbit torques inBi2Se3. Phys. Rev. Lett. 114, 257202. doi:10.1103/PhysRevLett.114.257202
Wang, Y., Zhu, D., Wu, Y., Yang, Y., Yu, J., Ramaswamy, R., et al. (2017). Room temperature magnetization switching in topological insulator-ferromagnet heterostructures by spin-orbit torques. Nat. Commun. 8, 1364. doi:10.1038/s41467-017-01583-4
Wu, Y., Zhang, S., Zhang, J., Wang, W., Zhu, Y. L., Hu, J., et al. (2020). Néel-type skyrmion in WTe2/Fe3GeTe2 van der Waals heterostructure. Nat. Commun. 11, 3–8. doi:10.1038/s41467-020-17566-x
Xu, H., Wei, J., Zhou, H., Feng, J., Xu, T., Du, H., et al. (2020). High spin Hall conductivity in large‐area type‐II Dirac semimetal PtTe 2. Adv. Mater. 32, 2000513. doi:10.1002/adma.202000513
Xue, F., Rohmann, C., Li, J., Amin, V., and Haney, P. (2020). Unconventional spin-orbit torque in transition metal dichalcogenide -ferromagnet bilayers from first-principles calculations. Phys. Rev. B 102, 014401.
Zhang, W., Sklenar, J., Hsu, B., Jiang, W., Jungfleisch, M. B., Xiao, J., et al. (2016). Research Update: spin transfer torques in permalloy on monolayer MoS2. Apl. Mater. 4, 032302. doi:10.1063/1.4943076
Zhang, X., Liu, Q., Luo, J.-W., Freeman, A. J., and Zunger, A. (2014). Hidden spin polarization in inversion-symmetric bulk crystals. Nat. Phys. 10, 387–393. doi:10.1038/nphys2933
Zhao, B., Khokhriakov, D., Zhang, Y., Fu, H., Karpiak, B., Hoque, A. M., et al. (2020). Observation of charge to spin conversion in Weyl semimetal WTe2 at room temperature. Phys. Rev. Research. 2, 1–8. doi:10.1103/physrevresearch.2.013286
Keywords: spin-orbit torques (SOT), transition metal dichalcogenides (TMD), van der Waals materials, spin-orbitronics, Two-dimensional materials (2D materials)
Citation: Hidding J and Guimarães MHD (2020) Spin-Orbit Torques in Transition Metal Dichalcogenide/Ferromagnet Heterostructures. Front. Mater. 7:594771. doi: 10.3389/fmats.2020.594771
Received: 14 August 2020; Accepted: 12 October 2020;
Published: 16 November 2020.
Edited by:
Myung Gwan Hahm, Inha University, South KoreaReviewed by:
Bipin Kumar Gupta, National Physical Laboratory (CSIR), IndiaNarayanan Tharangattu Narayanan, Tata Institute of Fundamental Research, India
Copyright © Hidding and Guimaraes. This is an open-access article distributed under the terms of the Creative Commons Attribution License (CC BY). The use, distribution or reproduction in other forums is permitted, provided the original author(s) and the copyright owner(s) are credited and that the original publication in this journal is cited, in accordance with accepted academic practice. No use, distribution or reproduction is permitted which does not comply with these terms.
*Correspondence: Marcos H. D. Guimarães, bS5oLmd1aW1hcmFlc0BydWcubmw=; Jan Hidding, amFuLmhpZGRpbmdAcnVnLm5s