- 1Department of Chemistry, Materials and Chemical Engineering “G. Natta,” Politecnico di Milano, Milan, Italy
- 2INSTM – National Interuniversity Consortium of Materials Science and Technology, Firenze, Italy
- 3School of Pharmacy and Biomolecular Sciences, University of Brighton, Brighton, United Kingdom
Titanium oxide nanotube arrays are extremely promising materials for localized drug delivery in orthopedic implants due to their excellent properties and facile preparation. TiO2 nanotubes can act as an effective drug reservoir to prevent bacterial colonization and implant infection and, at the same time, could promote tissue regeneration and effective osseointegration. Here, highly ordered TiO2 nanotubes (NTs) were synthesized by electrochemical anodization of titanium foils and the process parameters were varied in order to obtain a large range of NT diameters to evaluate its influence. The effect of NTs’ diameter on gentamicin loading/release and on osteosarcoma cell and bacterial adhesion was assessed. Anodization was confirmed as an easy and effective method to prepare highly ordered, open top nanotubes with predictable diameter as a function of imposed voltage. A lower amount of bacteria Staphylococcus Aureus adhesion was found on unloaded NTs surfaces at 24 h. When gentamicin was loaded, protracted release and antibacterial action was observed and bacteria adhesion was prevented on all NTs dimension. However, higher cell proliferation and a more favorable cell morphology was observed on smaller nanotubes, to support the indication toward a reduction in NTs diameter for the preparation of effective implant surfaces.
Introduction
One of the main challenges in the orthopedic field is the development of implant surfaces that combine a fast and durable fixation with an effective limitation of bacterial adhesion, to prevent implant failure and the incidence of implant-associated osteomyelitis.
Among the large variety of methods aimed at improving the interfacial properties of titanium implants (Giavaresi et al., 2008; De Nardo et al., 2012; LeGeros et al., 2016; Jäger et al., 2017; Zhao et al., 2018; Stewart et al., 2019), the generation of TiO2 nanotubes (NTs) by anodization has recently attracted considerable attention with the objective to evolve from osteoconductive to osteoinductive implant performance (Farid, 2019).
TiO2 nanotubes were first described in the nineties as having a “columnar honeycomb-like lattice,” observed upon addition of fluoride ionic species to the electrolyte in the anodization of Ti and Ti6Al4V (Zwilling and Darque-Ceretti, 1997). More recently, titania nanotubes have gained interest as bone contact surfaces mainly for two characteristic properties: a unique topography, to support early osteoblast adhesion and proliferation (Iwata et al., 2017) and the possibility to incorporate different classes of biologically-active molecules (either with osteogenic or antimicrobic activity) (Liu et al., 2016; Tao et al., 2019; Ion et al., 2020).
In vitro experiments on nanotube surfaces have shown improved cell adhesion, proliferation, and differentiation, as well as enhanced bone-forming abilities (Xia et al., 2012). In vivo experiments with screw- and disk-shaped implants have shown that nanotubes’ surfaces increase direct bone/cell contact and improve osseointegration strength compared to their blasted counterpart (Bjursten et al., 2010; Sul, 2010; Li et al., 2019).
The effectiveness of different bioactive agents differently loaded into nanotubes, which includes the simple adsorption of growth factors (e.g., bone morphogenetic protein-2; Li et al., 2019) or antibiotic compounds, as well as coatings with biopolymers (Huang et al., 2014; Saha et al., 2019), was investigated. Gentamicin-loaded nanotubes, in particular, were used to deliver high doses of antibiotics locally and inhibit bacterial adhesion without systemic toxicity and with no significant effect on osteogenic differentiation (Lin et al., 2014).
Together with the potential benefits in terms of clinical response, the processes for the preparation of nanotubes is simple, scalable, reproducible (Maher et al., 2018), and applicable to relatively complex 3D geometries. TiO2 nanotubes can be effectively grown on any pure titanium surface, and, in principle, to different titanium alloys’ surfaces of implantable orthopedic devices. Sandblasted surfaces, as well as machined surfaces, are eligible substrates for their preparation by anodization. Furthermore, according to specific clinical needs, drug loading can be performed either during the implant production, or, technically, even during surgery.
The array of nanotubes obtained on titania through electrochemical anodization is highly controllable in dimensions by acting on applied voltage and processing time. To date, however, there appears to be no consensus on the optimal nanotube diameter to promote cellular adhesion and proliferation and effective drug delivery. Although nanotubes with an 100–150 nm diameter were shown to promote cell viability (Brammer et al., 2009; Zhang et al., 2015), apoptosis was also reported for diameters larger than 50 nm (Park et al., 2007, 2009; von Wilmowsky et al., 2009; Kulkarni et al., 2016). Similarly, different works (Popat et al., 2007; Lin et al., 2014) have studied the interaction between gentamicin-loaded nanotubes with bacteria, but little attention has been dedicated to the optimization of drug release in time.
Here, highly ordered and open top nanotubes with different diameters were prepared and loaded with gentamicin to evaluate the effect of dimensions on their “reservoir effect,” loading capacity, and release profiles together with the effectiveness in inhibiting bacterial adhesion and growth and on cellular interaction.
Materials and Methods
If not otherwise specified, all reagents were purchased from Sigma-Aldrich and used without further purification.
TiO2 Nanotubes Synthesis
Titanium disks (12 mm Φ, 0.07 mm thick) were punched from cold-rolled grade 2 titanium foils (Titalia S.p.A.) with Ra = 0.22 ± 0.05 μm and Rmax = 1.98 ± 0.7 μm. TiO2 nanotubes (Roy et al., 2011; Regonini et al., 2013; Apolinario et al., 2014) were generated on the surface of titanium disks in a conventional two-electrode cell at room temperature. A platinum electrode was used as the cathode. Before anodization, Ti samples were sonicated for 5 min in acetone and then in Milli-Q water. After a preliminary evaluation on different ethylene glycol- (EG) based solutions and anodization times, a solution of NH4F 0.5% and 5.5 M Milli-Q water in EG and 3 h were, respectively chosen to generate nanotubes with different diameters by changing the anodization voltage.
After anodization, the samples were washed in ethanol for 1 h on a shaking platform to remove organic residuals, sonicated for 20 s in Milli-Q water to remove the nanograss structures generated during the process, and finally oven-dried at 37°C.
The morphology of TiO2 nanotubes was observed using a scanning electron microscope (SEM Stereoscan 360, Cambridge Instruments). ImageJ image processing software (Schneider et al., 2012) was used to calculate the external and internal diameter of nanotubes on the acquired images.
Antibiotic Loading
To load samples with antibiotic (Kulkarni et al., 2016; Stewart et al., 2019), 100 μl of gentamicin solution 5 mg/mL in PBS were pipetted on the surface of titanium disks to cover their entire surface and samples were allowed to dry in an orbital shaker at 50 rpm and 40°C. Deposition and evaporation steps were repeated four times in order to load each disk with a total of 2 mg of gentamicin. After the final drying, sample surfaces were rinsed by pipetting 1 mL of PBS to remove weakly bounded antibiotics.
Drug Loading and Release
Gentamicin loading efficiency was calculated as:
Where η is the loading efficiency, mo is the amount of gentamicin in the loading solution, and mr is the antibiotic found in the rinsing solution.
Release kinetics of gentamicin was investigated at 37°C with loaded samples individually soaked in 500 μl of PBS solution, collected, and replaced at selected time-points up to 48 h.
The presence of gentamicin in the eluates was analyzed using a colorimetric assay (Schneider Frutos et al., 2000). Briefly, 2.5 g of o-phthaldialdehyde, 62.5 mL of methanol, and 3 mL of 2-mercaptoethanol were mixed in 560 mL 0.04 M sodium borate solution in deionized water and refrigerated for at least 24 h. Eluates from gentamicin loaded samples were mixed 1:1 with phthaldialdehyde solution and incubated for 30 min at room temperature, protected from light, to allow the formation of gentamicin o-phthaldialdehyde complexes that were spectrophotometrically read at 332 nm.
A calibration curve using six known gentamicin concentrations was used to calculate the actual amount of gentamicin released by nanotubes.
Agar Diffusion Test
An agar diffusion test (Hudzicki, 2009) was performed to test the ability of nanotubular surfaces to inhibit bacterial growth using Staphylococcus aureus (NCTC 10788). Antibiotic-loaded samples were placed on the agar plate seeded with bacteria and incubated overnight at 37°C; the growth inhibition zone was then measured.
Microbial Adhesion and Proliferation
A quantitative measure of antibacterial activity of gentamicin-loaded nanotubes samples were obtained by Colonies Forming Units (CFU) counting (Mathur et al., 2006) of Staphylococcus aureus. Ti samples were soaked in a suspension of 107 bacteria in 1 mL of trypticase soy broth (TSB) and incubated at 37°C, then both planktonic and adhered cells were evaluated. Bacterial suspension incubated with no material was used as control.
To count planktonic bacteria, 50 μl of supernatant was collected after 7 and 24 h, plated on agar, and incubated at 37°C for 24 h. To count adherent bacteria, titanium disks were washed in PBS to eliminate non-adherent bacteria, inserted in a centrifuge tube with 2 mL of fresh PBS and 3 small glass spheres (∼ 3 mm diameter), and vortexed for 30 sec to ensure complete detachment of cells.
Osteosarcoma Cells Adhesion and Proliferation
Cells’ adhesion and proliferation on both loaded and unloaded NTs was evaluated using a human osteosarcoma cell model (Saos 2) and by evaluating cell adhesion and proliferation on samples after 24 and 72 h (von der Mark et al., 2009; Demetrescu et al., 2010; Pérez-Jorge et al., 2012).
To determine the number of viable and non−viable cells, 50 μl of Hoechst dye and 50 μl propidium iodide were diluted in 900 μl of medium and 60 μl of the resulting solution was added to each culture well. Images of stained samples were acquired under a fluorescence microscope to count live (blue), apoptotic (brighter blue), and dead (red) cells.
Phalloidin staining was used to evaluate cell morphology. Briefly, 80 μl of 3.7% paraformaldehyde solution in PBS was added in each well to fix the samples. After soaking for 5 min in 1% Triton X solution in PBS to permeabilize the cell membrane, 100 μl phalloidin solution (1:100 in PBS) was added and incubated for 1 h at room temperature.
Statistical Analysis
All statistical analyses were performed using GraphPad Prism 7. Groups were compared using one-way ANOVA and post-hoc tests after verification of data normality. P < 0.05 were considered statistically significant.
Results
Production and Characterization of TiO2 Nanotubes
The relation between anodization voltage and nanotube outer (O.D) and inner diameter (I.D.) are illustrated in Figure 1 and were consistent with previous findings (Lin et al., 2014). However, in the chosen conditions, a voltage threshold of 10 V was found, as no formation of nanotubes could be observed below this value.
As can be seen in Figure 2, an array of highly ordered nanotubes with a well-defined and open top morphology is found on the titanium surface.

Figure 2. Surface morphology of nanotubes’ array obtained at different voltages (A–C) and section view (D). Scale bar = 1 μm.
To build an experimental group on a representatively extended range of diameters, the voltage values in Table 1 were chosen for experimental samples of NTs’ preparation. For each voltage, the corresponding NTs’ diameters, lengths, and the designations used hereafter are indicated. If the length of nanotubes is considered, a reduction in nanotube depth is observed at lower voltages.
Drug Loading and Release
The loading efficiency of gentamicin calculated based on the quantity of antibiotic in the rinsing solution is reported in Table 2. As can be observed, similar values were generally found, but bigger nanotubes (NT160) appeared to have a slightly higher loading efficiency compared to smaller ones.
When the in vitro release was considered (Figure 3), a significant fraction of loaded gentamicin was found to be released from NTs after 1 h (about 30%). After this initial burst, the antibiotic release decreased slowly to become undetectable after 48 h.
A comparatively higher amount of gentamicin was released from larger nanotubes, but this is only partially attributable to the higher loading efficiency. Release from larger nanotubes was, in fact, notably faster between 1 and 2 h.
Agar Diffusion Test
After incubation at 37°C, an inhibition area was observed for all loaded NT samples on agar plates (Figure 4). All gentamicin-loaded disks were characterized by an inhibition zone with a diameter of about 30 mm, surrounding samples, with no statistically significant difference between the three different NTs’ dimensions. As reasonably expected, untreated Ti samples did not show any detectable inhibition zone.
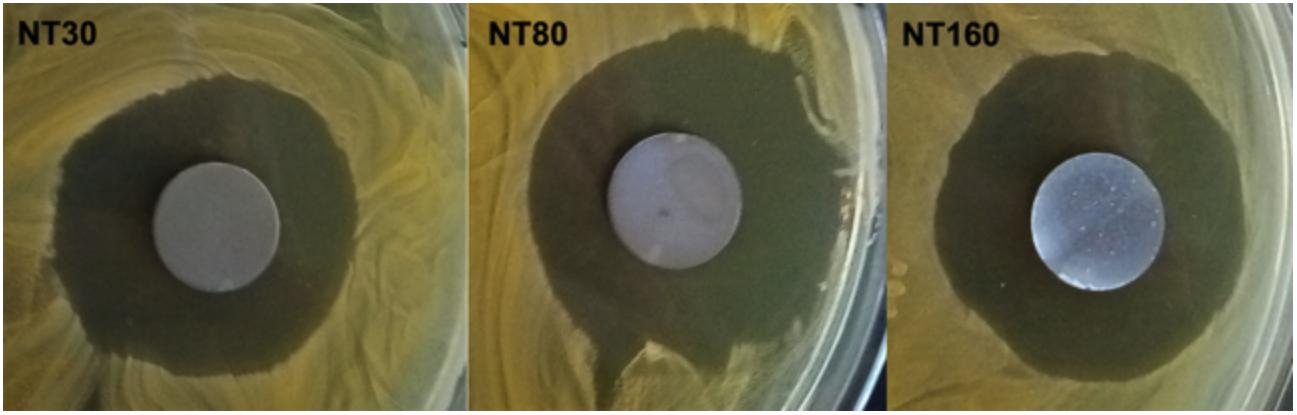
Figure 4. Representative images of inhibition zones in Agar diffusion test for gentamicin-loaded samples.
Bacterial Adhesion and Proliferation
The number of adhered bacteria for gentamicin loaded and unloaded Ti samples after 24 h incubation is shown in Figure 5.
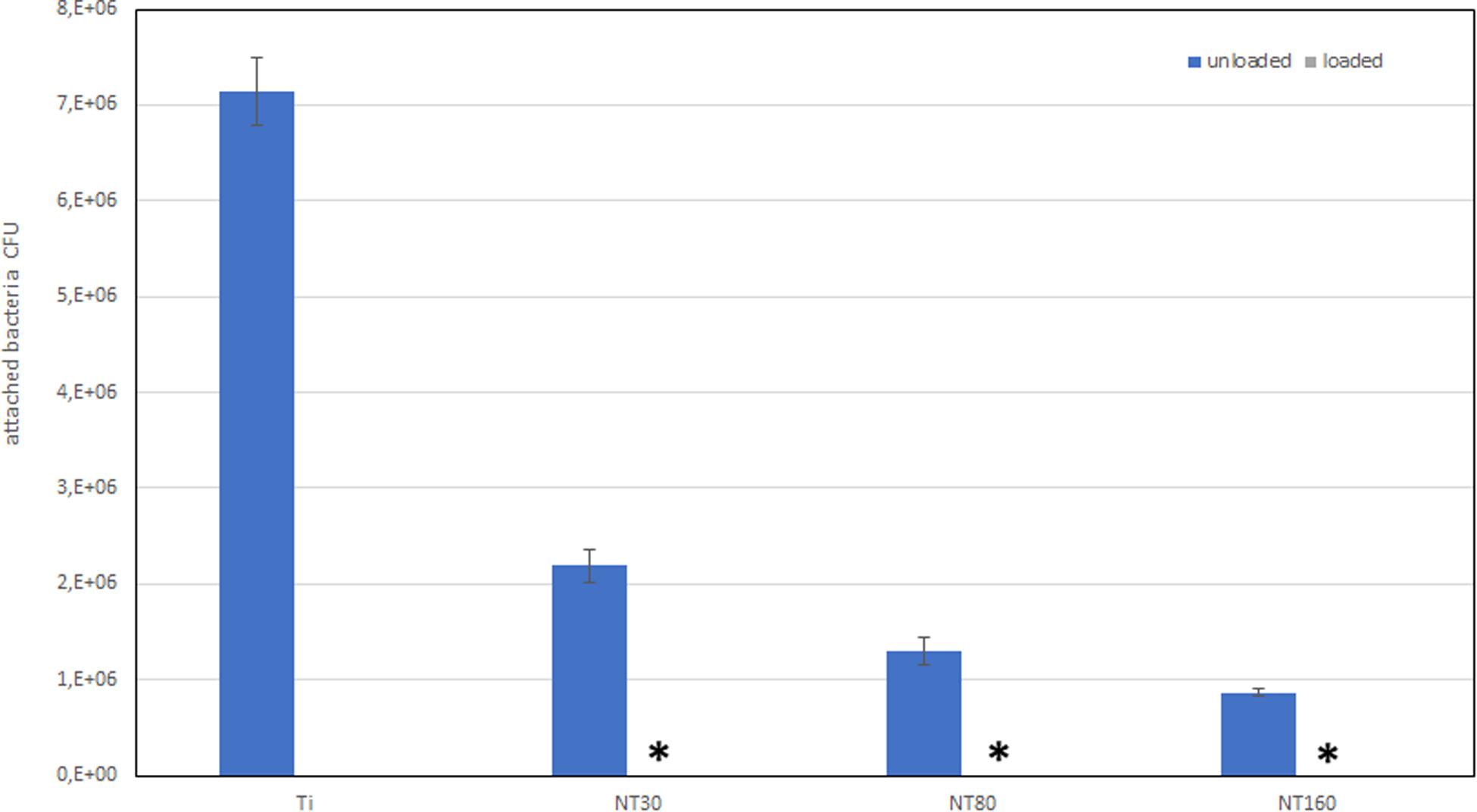
Figure 5. Number of adhered bacteria on Ti surface after 24 h incubation. * indicates that no bacteria were detected.
Compared to untreated Ti disks, nanotube samples show a considerable reduction in bacterial adhesion even without gentamicin. When gentamicin was loaded, bacteria could not be detected.
Interestingly, a significant difference in the number of adhered bacteria was found among nanotube diameters size. Specifically, nanotubes with larger diameters were found to allow a lower bacterial adhesion compared to smaller NTs.
When planktonic bacteria were considered (Figure 6), a comparable CFU count was observed for all samples after 7 h. Conversely, after 24 h a statistically significant difference in bacteria number among untreated Ti and all nanotubes samples was observed, with an average 40% reduction. For planktonic bacteria, however, no significant differences were noticed among the different nanotube diameters size.
Cells Adhesion and Proliferation
The normalized number of live cells on the surface of NTs samples after 24 and 72 h are reported for unloaded samples in Figure 7. When gentamicin – loaded samples were considered, superimposable results were found (data not shown). In both cases, statistical analysis did not evidence any significant differences in cell number among NTs with different diameter sizes.
Interestingly, initial cell density is notably lower on NTs samples compared to the control (40% less in average), but the number of cells increases comparatively faster on NTs and the number of cells reaches and overcomes the values on control after 72 h.
Differences in cell morphology were instead observed as a result of change in diameter (Figure 8) and phalloidin staining revealed more spindle-shaped cells on nanotubes with a smaller diameter, whereas more rounded cells were observed for larger NTs.
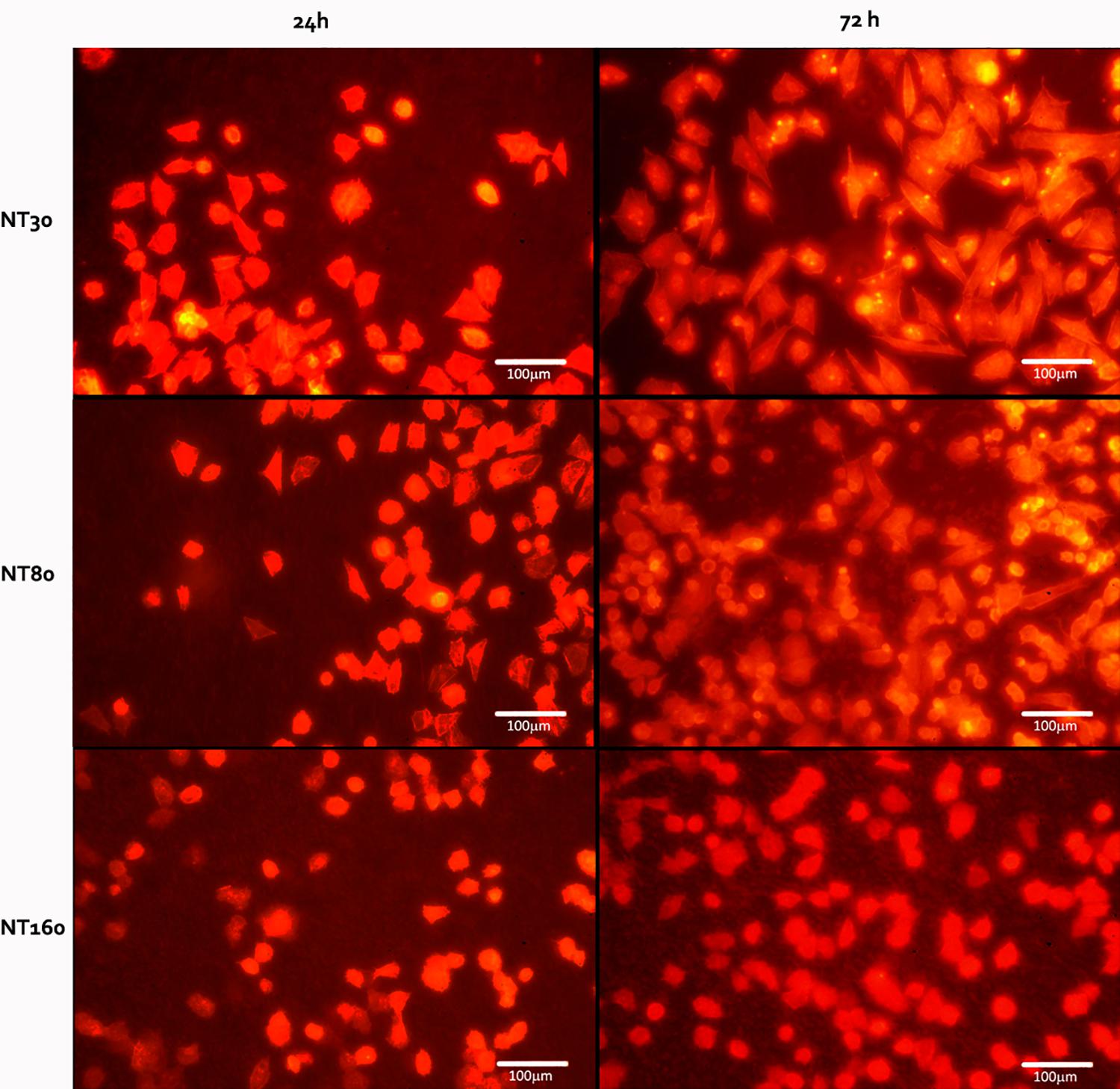
Figure 8. Representative images of the Saos 2 cells on the nanotube samples (NT30, NT80, and NT160) after 24 and 72 h.
Discussion and Conclusion
To act as an effective drug reservoir and to offer a high number of anchoring sites for cells, TiO2 nanotube arrays should possess an open top morphology. In this regard, the protocol for NTs preparation optimized in this work appeared extremely effective. In our experience, the balance of water content and fluoride concentration was critical for obtaining nanotubes with an almost 100% open top while containing the process duration. A higher water content, in fact, results in a more open top structure, but generally requires longer treatment times, while time reduction can be obtained by increasing fluoride content in the solution (the morphologies of NTs’ optimization can be found in Supplementary Material).
By changing the anodization voltage, NTs’ diameter can be easily controlled, as a consistent dependence with applied voltage exists and the size of resulting structures is highly predictable.
The reservoir capability of NTs was confirmed by the observed release of loaded gentamicin for up to 48 h. After this time interval, the release quantity was too small to be detected by our assay. However, based on the cumulative release, about half the drug loaded is still present in the nanotubes and is potentially effective for a longer time.
In this work we specifically focused on the effect of nanotubes’ diameters and we tried to analyze the largest range possible to evaluate its effect on drug release and cell adhesion. The diameter of nanotubes was confirmed to be a very relevant variable for both drug release and cell/bacteria interaction. Bigger nanotubes (NT160) were capable of accommodating a comparatively higher quantity of antibiotics than smaller ones, but faster release was observed. On the other hand, smaller nanotubes have a higher available surface, are more likely to withhold the antibiotic molecules more effectively, and showed a slightly slower release.
When Ti samples were incubated with bacteria, no adherent cells were found for all gentamicin samples, to indicate that the quantity of loaded antibiotics is sufficient for an effective antibacterial action. Interestingly, a significant reduction of bacterial adhesion was also observed for unloaded samples, but this phenomenon can possibly be explained by the presence of residual fluoride ions (Ercan et al., 2011; Anitha et al., 2015). The number of adhered bacteria was significantly lower for larger nanotubes (160 nm), and this can also be related to the fact that smaller NTs stimulate bacterial cells to produce pili and attach to the surface (Ercan et al., 2011).
When osteosarcoma cells were seeded on the sample surface, although no statistical difference was observed among NTs with different nanotubes, samples with larger nanotubes appeared to induce cellular apoptosis more than smaller ones. A higher cell compatibility of these latter NTs was also confirmed by the spindle-like morphology observed on their surfaces. This more favorable behavior can be explained with the higher number of anchoring site provided (Roy et al., 2011), as small-sized NTs can promote cell growth and differentiation by supporting a higher density of integrin focal contacts (Zhang et al., 2015).
When using gentamicin, many authors report the use of very high doses (up to 20-fold the recommended concentrations) without appreciable side effect on cells in vitro (Schafer et al., 1972; Sigma-Aldrich, 2002; Kovacikanton et al., 2017). To confirm these results, here no difference in the cell viability between loaded and unloaded samples was observed. However, the chosen gentamicin quantity appears adequate to eliminate the bacteria.
Overall, our results confirm that anodization of titanium substrates to prepare TiO2 nanotubes is an extremely favorable surface treatment and has the potential to both improve osseointegration of orthopedic prosthesis and prevent infection-related failures. Within the tested range, smaller NTs appear as the more promising option for further investigation, as they offer effective antibacterial action together with a more favorable cell interaction.
Data Availability Statement
All datasets generated for this study are included in the article/Supplementary Material.
Author Contributions
RC contributed to the conception and design of the study. LD, VP, and MM performed the experiments and wrote the first draft of the manuscript. MS performed the in vitro tests. All authors contributed to manuscript revision, read and approved the submitted version.
Conflict of Interest
The authors declare that the research was conducted in the absence of any commercial or financial relationships that could be construed as a potential conflict of interest.
Supplementary Material
The Supplementary Material for this article can be found online at: https://www.frontiersin.org/articles/10.3389/fmats.2020.00233/full#supplementary-material
References
Anitha, V. C., Lee, J. H., Lee, J., Narayan Banerjee, A., Woo Joo, S., and Ki Min, B. (2015). Biofilm formation on a TiO2 nanotube with controlled pore diameter and surface wettability. Nanotechnology 26:65102.
Apolinario, A., Sousa, C. T., Ventura, J., Costa, J. D., Leitao, D. C., Moreira, J. M., et al. (2014). The role of the Ti surface roughness in the self-ordering of TiO 2 nanotubes: a detailed study of the growth mechanism. J. Mater. Chem. 2, 9067–9078. doi: 10.1039/c4ta00871e
Bjursten, L. M., Rasmusson, L., Oh, S., Smith, G. C., Brammer, K. S., and Jin, S. (2010). Titanium dioxide nanotubes enhance bone bonding in vivo. J. Biomed. Mater. Res. Part A. 92, 1218–1224.
Brammer, K. S., Oh, S., Cobb, C. J., Bjursten, L. M., van der Heyde, H., and Jin, S. (2009). Improved bone-forming functionality on diameter-controlled TiO2 nanotube surface. Acta Biomat. 5, 3215–3223. doi: 10.1016/j.actbio.2009.05.008
De Nardo, L., Altomare, L., Del Curto, B., Cigada, A., and Draghi, L. (2012). Electrochemical surface modifications of titanium and titanium alloys for biomedical applications. Coat. Biomed. Appl. 2012, 106–142. doi: 10.1533/9780857093677.1.106
Demetrescu, I., Pirvu, C., and Mitran, V. (2010). Effect of nano-topographical features of Ti/TiO2 electrode surface on cell response and electrochemical stability in artificial saliva. Bioelectrochemistry 79, 122–129. doi: 10.1016/j.bioelechem.2010.02.001
Ercan, B., Taylor, E., Alpaslan, E., and Webster, T. J. (2011). Diameter of titanium nanotubes influences anti- bacterial efficacy. Nanotechnology 22:295102. doi: 10.1088/0957-4484/22/29/295102
Farid, S. B. H. (2019). Osteoinduction, osteoconduction, and osseointegration. Bioceramics Mater. Sci. Engin. 10, S96–S101.
Giavaresi, G., Chiesa, R., Fini, M., and Sandrini, E. (2008). Effect of a multiphasic anodic spark deposition coating on the improvement of implant osseointegration in the osteopenic trabecular bone of sheep. Internat. J. Oral Maxillofacial Impl. 23:659-68.
Huang, P., Wang, J., Lai, S., Liu, F., Ni, N., Cao, Q., et al. (2014). Surface modified titania nanotubes containing anti-bacterial drugs for controlled delivery nanosystems with high bioactivity. J. Mater. Chem. B 2, 8616–8625. doi: 10.1039/c4tb01281j
Hudzicki, J. (2009). Kirby-Bauer disk diffusion susceptibility test protocol. Washington, DC : American Society for Microbiology, 1–14.
Ion, R., Necula, M. G., Mazare, A., Mitran, V., Neacsu, P., Schmuki, P., et al. (2020). Drug Delivery Systems Based on Titania Nanotubes and Active Agents for Enhanced Osseointegration of Bone Implants. Curr. Med. Chem. 27, 854–902. doi: 10.2174/0929867326666190726123229
Iwata, N., Nozaki, K., Horiuchi, N., Yamashita, K., Tsutsumi, Y., Miura, H., et al. (2017). Effects of controlled micro-/nanosurfaces on osteoblast proliferation. J. Biomed. Mater. Res. Part A 105, 2589–2596. doi: 10.1002/jbm.a.36118
Jäger, M., Jennissen, H., Dittrich, F., Fischer, A., and Köhling, H. (2017). Antimicrobial and osseointegration properties of nanostructured titanium orthopaedic implants. Materials 10:1302. doi: 10.3390/ma10111302
Kovacikanton, A., Eva, T., Diana, F., Peter, C., Eva, K., Katarina, Z., et al. (2017). In Vitro Assessment of Gentamicin Cytotoxicity on the Selected Mammalian Cell Line (Vero cells). Adv. Res. Life Sci 1, 111–116. doi: 10.1515/arls-2017-0018
Kulkarni, M., Mazare, A., Schmuki, P., and Iglic, A. (2016). Influence of anodization parameters on morphology of TiO2 nanostructured surfaces. Adv. Mater. Lett. 7, 23–28. doi: 10.5185/amlett.2016.6156
LeGeros, R. Z., Coelho, P. G., Holmes, D., Dimaano, F., and LeGeros, J. P. (2016). “Orthopedic and dental implant surfaces and coatings,” in Biological and Biomedical Coatings Handbook. S. Zhang Florida: CRC Press.
Li, Y., Song, Y., Ma, A., and Li, C. (2019). Surface Immobilization of TiO2 Nanotubes with Bone Morphogenetic Protein-2 Synergistically Enhances Initial Preosteoblast Adhesion and Osseointegration. BioMed. Res. Internat. 2019:5697250.
Lin, W.-T., Tan, H.-L., Duan, Z.-L., Yue, B., Ma, R., He, G., et al. (2014). Inhibited bacterial biofilm formation and improved osteogenic activity on gentamicin-loaded titania nanotubes with various diameters. Int. J. Nanomed. 9, 1215–1230. doi: 10.2147/ijn.s57875
Liu, X., Zhang, Y., Li, S., Wang, Y., Sun, T., Li, Z., et al. (2016). Study of a new bone-targeting titanium implant–bone interface. Internat. J. Nanomed. 11, 6307–6324. doi: 10.2147/ijn.s119520
Maher, A. S., Mazinani, A., Barati, M. R., and Losic, D. (2018). Engineered titanium implants for localized drug delivery: recent advances and perspectives of Titania nanotubes arrays. Exp.Ert Opin. Drug Del. 15, 1021–1037. doi: 10.1080/17425247.2018.1517743
Mathur, A. R. T., Singhal, S., Khan, S., Upadhyay, D. J., and Fatma, T. (2006). Detection of biofilm formation among the clinical isolates of staphylococci: An evaluation of three different screening methods. Indian J. Med. Microbiol. 24, 25–29. doi: 10.4103/0255-0857.19890
Park, J., Bauer, S., Schlegel, K. A., Neukam, F. W., Der Von, Mark, K., et al. (2009). TiO2 nanotube surfaces: 15 nm - an optimal length scale of surface topography for cell adhesion and differentiation. Small 5, 666–671. doi: 10.1002/smll.200801476
Park, J., Bauer, S., Von Der, Mark, K., and Schmuki, P. (2007). Nanosize and vitality: TiO2 nanotube diameter directs cell fate. Nano. Lett. 7, 1686–91.
Pérez-Jorge, C., Conde, A., Arenas, M. A., Pérez-Tanoira, R., Matykina, E., de Damborenea, J. J., et al. (2012). In vitro assessment of Staphylococcus epidermidis and Staphylococcus aureus adhesion on TiO 2 nanotubes on Ti-6Al-4V alloy. J. Biomed. Mater. Res. Part A 100, 1696–1705. doi: 10.1002/jbm.a.34118
Popat, K. C., Eltgroth, M., LaTempa, T. J., Grimes, C. A., and Desai, T. A. (2007). Decreased Staphylococcus epidermis adhesion and increased osteoblast functionality on antibiotic-loaded titania nanotubes. Biomaterials 28, 4880–4888. doi: 10.1016/j.biomaterials.2007.07.037
Regonini, D., Bowen, C. R., Jaroenworaluck, A., and Stevens, R. (2013). A review of growth mechanism, structure and crystallinity of anodized TiO 2 nanotubes. Mater. Sci. Eng. R 74, 377–406. doi: 10.1016/j.mser.2013.10.001
Roy, P., Berger, S., and Schmuki, P. (2011). TiO2 nanotubes: Synthesis and applications. Angew. Chem. Int 50, 2904–2939. doi: 10.1002/anie.201001374
Saha, S., Pramanik, K., and Biswas, A. (2019). Silk fibroin coated TiO2 nanotubes for improved osteogenic property of Ti6Al4V bone implants. Mater. Sci. Engin. C 105:109982. doi: 10.1016/j.msec.2019.109982
Schafer, T., Pascale, A., Shimonaski, G., and Came, P. E. (1972). Evaluation of gentamicin for use in virology and tissue culture. Appl. Microbiol 23, 565–570. doi: 10.1128/aem.23.3.565-570.1972
Schneider, C. A., Rasband, W. S., and Eliceiri, K. W. (2012). NIH Image to ImageJ: 25 years of image analysis. Nat. Methods 9, 671–675. doi: 10.1038/nmeth.2089
Schneider Frutos, P. C., Díez Peña, E., Barrales-Rienda, J. M., and Frutos, G. (2000). Validation and in vitro characterization of antibiotic-loaded bone cement release. Int. J. Pharm. 209, 15–26. doi: 10.1016/s0378-5173(00)00520-2
Stewart, C., Akhavan, B., Wise, S. G., and Bilek, M. M. (2019). A review of biomimetic surface functionalization for bone-integrating orthopedic implants: Mechanisms, current approaches, and future directions. Progr. Mater. Sci. 106:100588. doi: 10.1016/j.pmatsci.2019.100588
Sul, Y. T. (2010). Electrochemical growth behavior, surface properties, and enhanced in vivo bone response of TiO2 nanotubes on microstructured surfaces of blasted, screw-shaped titanium implants. Internat. J. Nanomed. 5, 87–100. doi: 10.2147/ijn.s8012
Tao, B., Deng, Y., Song, L., Ma, W., Qian, Y., Lin, C., et al. (2019). BMP2-loaded titania nanotubes coating with pH-responsive multilayers for bacterial infections inhibition and osteogenic activity improvement. Colloids Surfaces B Biointer. 177, 242–252. doi: 10.1016/j.colsurfb.2019.02.014
von, der Mark, K., Bauer, S., Park, J., and Schmuki, P. (2009). Another look at ‘Stem cell fate dictated solely by altered nanotube dimension. Proc. Natl. Acad. Sci 106, E60.
von Wilmowsky, C., Bauer, S., Lutz, R., Meisel, M., Neukam, F. W., Toyoshima, T., et al. (2009). In Vivo Evaluation of Anodic TiO2 Nanotubes; An Experimental Study in the Pig. J. Biomed. Mater. Res. Part B Appl. Biomater 89, 165–171.
Xia, L., Feng, B., Wang, P., Ding, S., Liu, Z., Zhou, J., et al. (2012). In vitro and in vivo studies of surface-structured implants for bone formation. Internat. J. Nanomed. 7, 4873–4881. doi: 10.2147/ijn.s29496
Zhang, R., Wu, H., Ni, J., Zhao, C., Chen, Y., Zheng, C., et al. (2015). Guided proliferation and bone- forming functionality on highly ordered large diameter TiO2 nanotube arrays, Mater. Sci. Eng. 53, 272–279. doi: 10.1016/j.msec.2015.04.046
Zhao, H., Huang, Y., Zhang, W., Guo, Q., Cui, W., Sun, Z., et al. (2018). Mussel-inspired peptide coatings on titanium implant to improve osseointegration in osteoporotic condition. ACS Biomater. Sci. Engin. 4, 2505–2515. doi: 10.1021/acsbiomaterials.8b00261
Keywords: nanotubes, titanium oxide, drug delivery, anodization, antibacterial
Citation: Draghi L, Preda V, Moscatelli M, Santin M and Chiesa R (2020) Gentamicin-Loaded TiO2 Nanotubes as Improved Antimicrobial Surfaces for Orthopedic Implants. Front. Mater. 7:233. doi: 10.3389/fmats.2020.00233
Received: 05 October 2019; Accepted: 25 June 2020;
Published: 24 September 2020.
Edited by:
Hasan Uludag, University of Alberta, CanadaReviewed by:
Shun Duan, Beijing University of Chemical Technology, ChinaGabriella Epasto, University of Messina, Italy
Copyright © 2020 Draghi, Preda, Moscatelli, Santin and Chiesa. This is an open-access article distributed under the terms of the Creative Commons Attribution License (CC BY). The use, distribution or reproduction in other forums is permitted, provided the original author(s) and the copyright owner(s) are credited and that the original publication in this journal is cited, in accordance with accepted academic practice. No use, distribution or reproduction is permitted which does not comply with these terms.
*Correspondence: Roberto Chiesa, cm9iZXJ0by5jaGllc2FAcG9saW1pLml0