- 1Microelectronics Research Unit, Faculty of Information Technology and Electrical Engineering, University of Oulu, Oulu, Finland
- 2DiSTAS - Department for Sustainable Food Process, Università Cattolica del Sacro Cuore, Piacenza, Italy
- 3Bio Base Europe Pilot Plant, Ghent, Belgium
Food industry must guarantee food safety and seek sustainable solutions for increasing shelf life and decreasing food waste. Bio-based smart packaging is a potential option, where sustainability and real-time monitoring of food quality are combined assuring health safety and providing economic and environmental benefits. In this context, bio-based refers not only to packaging materials that are from renewable sources and biodegradable, but also to the sensor elements. The scope of this review is to explore the state-of-the-art of bio-based polymers used as food contact materials and to highlight the potential of natural compounds for sensing chemical and physical changes of the environment to monitor the food quality. Finally, different sustainability aspects of the bio-based materials are discussed.
Introduction
Busy lifestyles and growing urban populations mean an increasing demand for food that is fresh, healthy, convenient, and fast. One of the key drivers of this growth is the world’s rising population which by the year 2050 will reach 9.7 billion people with increase of 26% (The United Nations, 2019). With the global population rising, wastage of food including 47% of all fruit and vegetables and 12% of meat and animal products, is one of the greatest challenges to achieve food security (Food and Agriculture Organization of the United Nations, 2019a). Although food is our basic necessity, its production, processing, transportation and storage are rather complex from many aspects and need to fulfill a number of criteria to ensure the health and environmental safety and economic feasibility.
Foods pose potential danger of diseases due to bacterial (Salmonella, Campylobacter, Listeria, and Cholera), viral (Norovirus, Hepatitis A), parasite (tapeworms, trematodes, Ascaris, Cryptosporidium, Entamoeba histolytica, and Giardia), fungal (Aspergillus, Candida, and Fusarium) and even prion infections as consequence of inappropriate handling and processing of the products causing foodborne diseases that affect ∼10% of global population with a death toll of 420,000 deaths each year. Chemical contaminants, which may even accumulate in various food chains, represent further risks. These include phytochemical residues, mycotoxins, marine toxins from algae, cyanogenic glycosides from plants, and different metabolites from products aging and decaying (ethanol, putrescine, cadaverine, histamine, ethylene etc.) but also environmental and industrial pollutants, e.g., dioxins, polychlorinated biphenyls and heavy metals (Pb, Cd, and Hg) (World Health Organization, 2019). Another issue in the context of food safety is deliberate fraud to counterfeit the origin, content or quality (i.e., expiration dates) of products (Europol, 2015). Just diluting a high-quality wine with cheaper one mainly hurts the wallet and pride but more severe cases may endanger health permanently or cost lives (Branigan, 2008). Furthermore, the flip side of food safety is food waste as 1/3 of all produced food is lost or goes into waste (Food and Agriculture Organization of the United Nations, 2019a) meaning safe and edible food products are thrown away although the “best if used before” dates are only recommendations without information of the true status of the food. This is ethically and practically controversial as still today 820 billion people suffer from undernutrition and agricultural production would need to increase with 50% to feed the growing population by 2050 (Food and Agriculture Organization of the United Nations, 2019b).
As a partial solution to complex problems of food safety and decreasing unnecessary food waste is the selection and development of proper food packaging. The function of the food packages has been the same throughout history: to maintain hygiene, protect the food during transportations and storing, and ultimately to increase shelf life. However, modern technology and materials science have introduced new “smart” functions to food packages, which include advanced packaging materials with improved properties, and sensors that can monitor food quality (Yam et al., 2005; Kuswandi et al., 2011). Over the years, several approaches toward smart packaging have been demonstrated including time-temperature indicators, modified atmosphere packaging sensors for CO2 and O2 monitoring, total volatile base nitrogen sensors to detect food decay, fruit ripeness indicators, pathogen sensors, and solutions for food tracking and authentication (RFID tags) (Fuertes et al., 2016; Ghaani et al., 2016; Ahmed et al., 2018; Badia-Melis et al., 2018; Galstyan et al., 2018; Mustafa and Andreescu, 2018; Yousefi et al., 2019).
As of today, plastics (rigid and flexible) have the largest shares of the market in food packaging (37% market), followed by paper and board (34%), glass (11%), and metal (9%) (Muncke, 2012). If we consider the properties of the given food packaging materials, the high market share of paper and board can be explained by the renewable source and recyclability, it is printable, wet and dry food can be stored in paper and board after laminating/covering process and in general paper and board are very suitable for mass production lowering the costs (Kirwan, 2011). Containers made of glass, on the other hand, are among the oldest materials man has used as it can be shaped to practically any form, has high chemical resistance, is impermeable to gasses, absorbs UV and even parts of visible spectrum (amber glass, green and glass partially), is hygienic and reusable, and the consumers associate it to high-quality products (Grayhurst and Girling, 2011). Metal is also an important food packaging material as it is durable withstanding packaging conditions in vacuum or under pressure and high temperature stabilization process for long shelf-life foods. Metal is reusable, UV-resistant and the food contact surface may be coated with different coatings in case the interaction between the product and the plain container would downgrade the shelf-life/quality of the product to an unacceptable level due to, e.g., metal surface corrosion or undesired food coloring as a consequence of combination of metal ions with the food components (Oldring and Nehring, 2007). Typically metal containers or cans are made of steel (tin-coated or tin-free) or aluminum (Kraus and Tarulis, 2009; Reingardt and Nieder, 2009; Robertson, 2012).
The most frequently used plastics in food packages in Europe are PP (19.3%), LDPE and LLDPE (17.5%), and PET (7.4%); and in fact, 39.9% of all produced plastics (61.8 million tons in Europe in 2018) goes to packaging in general (PlasticsEurope, 2019a). The success of plastic as packaging material can be explained by low cost, ease of modification from flexible films to rigid containers, strength, stability, light-weight, impermeability with gasses and many solvents, and enabled sterilization without affecting the food quality (PlasticsEurope, 2019b). Despite of numerous benefits, plastics are also problematic: annual global production of plastics is around 350 million tons of which only 1% is bio-based (European Bioplastics e.V., 2020) and the rest is fossil-derived with large carbon footprint [6% of all produced oil goes to plastics, having a carbon footprint equivalent to the aviation sector (World Economic Forum et al., 2016)]. In addition, plastic pollution is alarming, as polymers do not degrade but break down to smaller pieces ending up in the air, soil and water as microplastics, found even in deep-sea amphipods (Jamieson et al., 2019).
As a response to challenges associated with food safety, storage and transportation, there is a huge market need for more sustainable bio-based plastics and sensors that could alleviate the environmental, public health and economic burden caused by traditional materials. The scope of this short review is to collect the contemporary literature on bio-based smart food packages covering not only bio-based food packaging materials but also bio-based sensors for monitoring various physical, chemical and biological conditions of foodstuff.
Bio-Based Plastic Packaging Materials
Production data for bio-based and biodegradable plastics are available to a limited extent only, although production capacity data are more readily accessible. Currently the production capacities of bio-based and biodegradable plastics are low (Figure 1), however, the market of some bio-based and/or biodegradable plastics are expected to grow significantly during the coming years (Bio-PET, PBS, and PLA) others are expected to consolidate (CA and Bio-PA) (van den Oever et al., 2017). Overall, it is expected that the global bioplastics production capacity is set to increase from around 2.11 million tons in 2019 to approximately 2.43 million tons in 2024 (Figure 2).
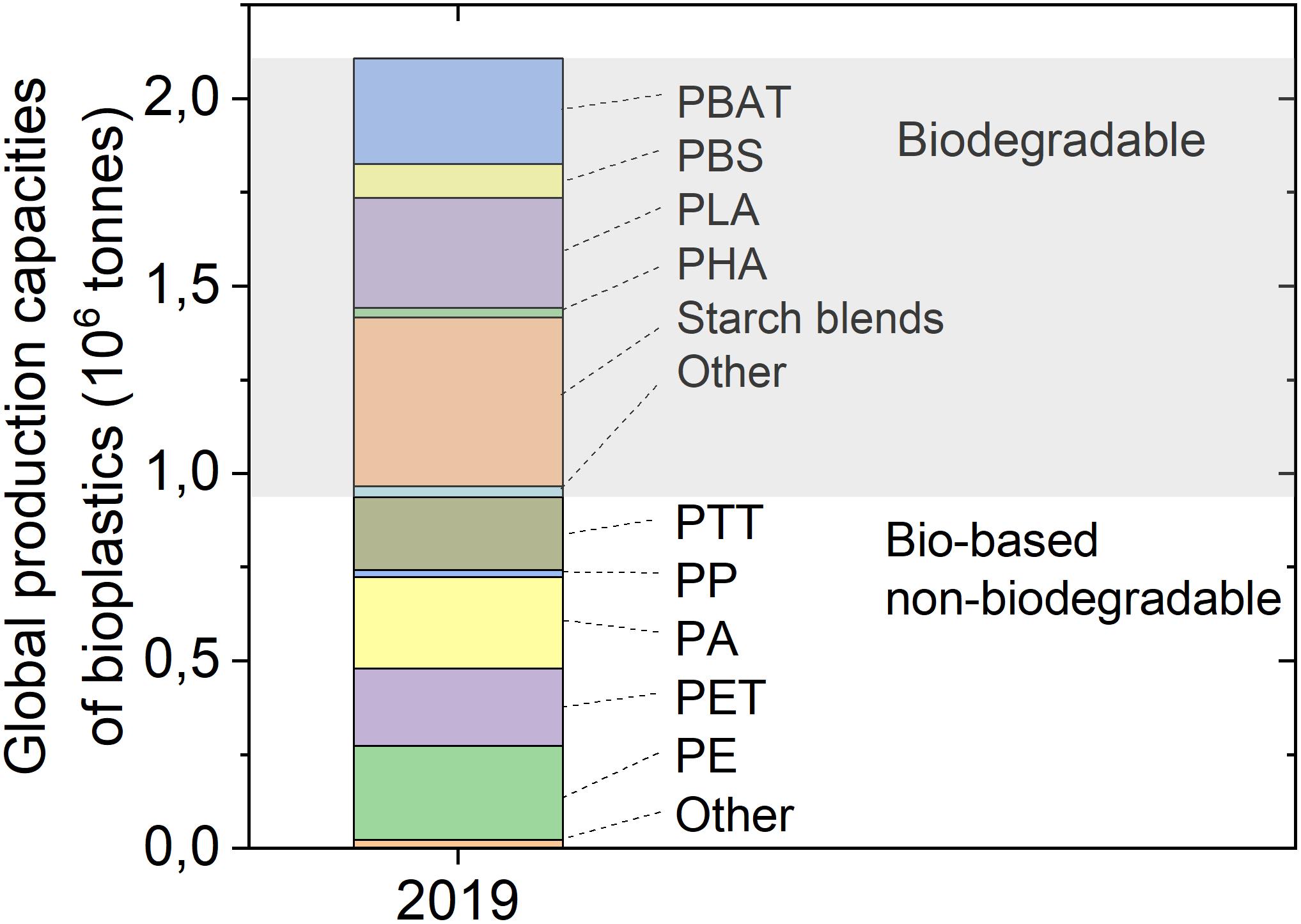
Figure 1. Current global production capacities of bioplastics by material type (European Bioplastics e.V., 2020).
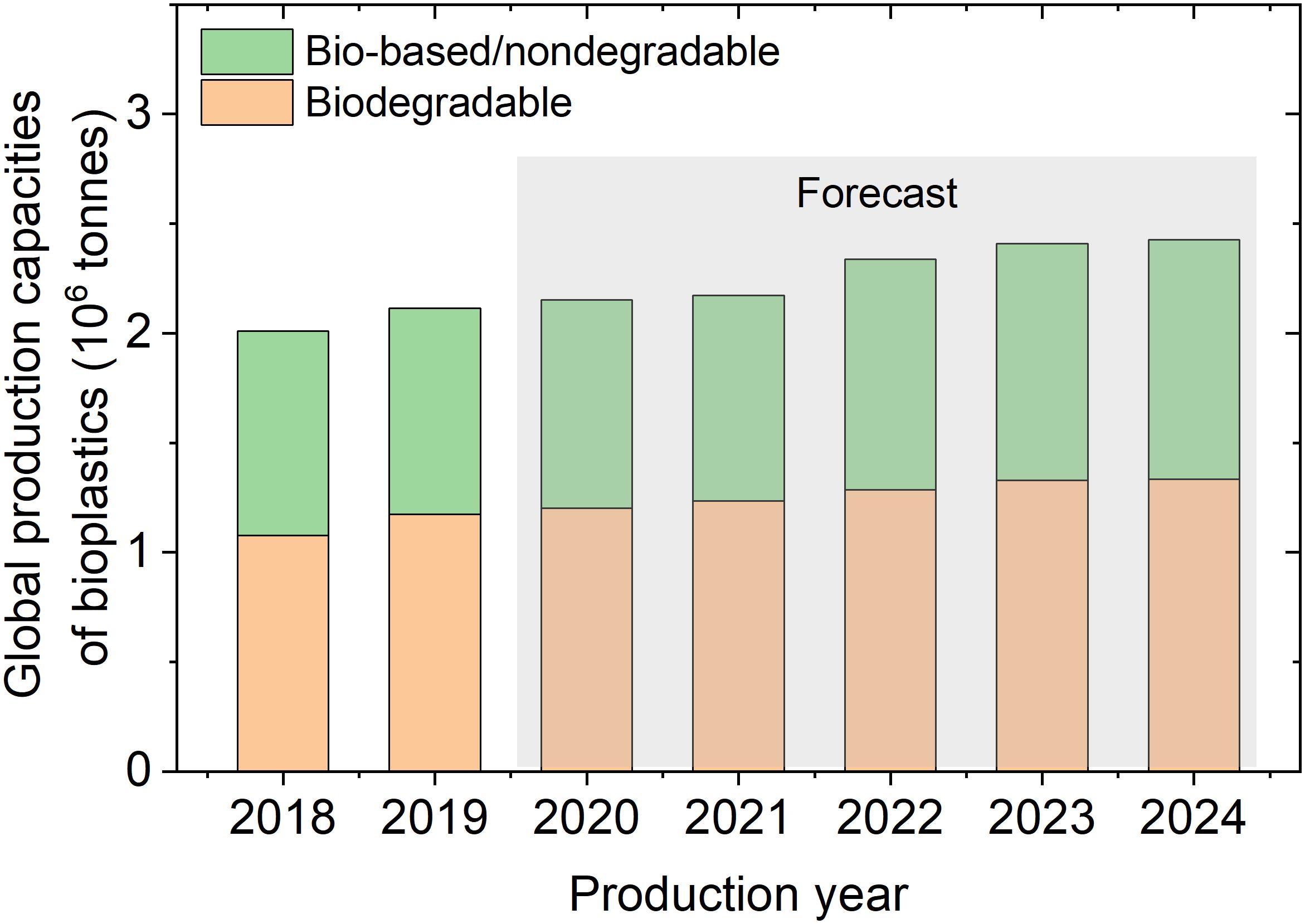
Figure 2. Current and forecast global production capacities of bioplastics (European Bioplastics e.V., 2020).
A number of different routes have been developed during the past decade to produce bio-based materials [i.e., either made of bio-based source or it is biodegradable or contains both of these features (European Bioplastics e.V., 2019)] with a large variety of properties and applications areas (Table 1). Among these, only a few families are made of renewable biomass and are biodegradable [e.g., cellulose and starch thermoplastics, PHAs, PLA, polyester amides (Avérous, 2008)], viz. bio-based polyethylene, PP, polyamide and polyethylene terephthalate are non-biodegradable, and PCLs and PVAs are from non-renewable resources (Chen and Patel, 2012; Geueke, 2014; van Crevel, 2016). The main production routes are as follows:
1. Direct extraction of biopolymers such as starch and cellulose with subsequent thermopressing/molding to make thermoplastic starch polymers (TSPs) or using additional functionalization, e.g., acetylation, carboxymethylation and phosphorylation to produce CA, carboxymethyl cellulose and cellulose diphenyl-phosphate, respectively, which are then polymerized further (Šešlija et al., 2018) or used as additives in polymers (Weinmann and Cotton, 1958).
2. Hydrolysis to sugars followed by bacterial synthesis of polyesters, e.g., PHAs including PHB.
3. Conversion into sugars that are fermented to lactic acid followed by its direct polycondensation or by ring-opening condensation of lactide to PLA (Avérous, 2008).
4. Chemical conversion into monomers followed by polymerization, e.g., amino acids obtained by hydrolysis and separation are polymerized with esters of lactonized unsaturated fatty acids in PEA synthesis.
Based on the technical report published by Wageningen Food & Biobased Research in 2017 (van den Oever et al., 2017), it has been shown that the bio-based and biodegradable plastics are currently more expensive than fossil-based plastics on weight basis (Tables 2, 3). However, specific material properties can allow costs reductions in the use or end-of-life phase. Further, the price of fossil-based plastics is dependent on oil prices and fluctuating with it, while in general the price of bio-based plastics depends on biomass prices that are more stable. When the production scale, conversion into final products and logistics become more favorable, it is expected that the prices of bio-based plastics will come down.
In addition, since most bio-based plastics have a higher density, this directly contributes to their higher price. But there are exceptions when prices are compared on a product level. By selecting specific material properties and redesigning can allow material savings. For example, a traditional HIPS-based cup of 0.89 mm wall thickness could be down-gauged using impact modified PLA to 0.66 mm thickness (Schut, 2016).
Bio-Based Smart Food Packages
Many new concepts in food packaging, like the smart functionalities, have been introduced during the last years in response to the increasing demand of ready-to-eat and higher quality foods (Vanderroost et al., 2014).
Smart functionalities of food packages refer to active coatings and physical/chemical sensors combined with the packaging materials. The purpose of smart antimicrobial coatings is to mitigate the proliferation of various microbes thus prolonging the shelf-life of products, whereas sensors play role in monitoring physical and chemical conditions that influence or reflect the quality of the food products. These add-ons have inevitable positive health, environmental and socio-economic effects, which may be amplified even further by accomplishing the smart functions using renewable natural materials and robust technologies (Arroyo et al., 2019).
Antimicrobial Films
As mentioned, active food packaging involves the use of polymeric films that act as a support for various active compounds such as natural extracts that can be incorporated during the manufacturing process of the packaging itself (Kuorwel et al., 2015; Bassani et al., 2019). Antimicrobial incorporation may result in a material with antibacterial activity which can suppress the growth of bacteria on the material surface (according to the international norm ISO 22196:2011 – Measurement of antibacterial activity on plastics and other non-porous surfaces). In the food sector, a greater interest is toward materials enriched with antimicrobials so that the direct use of food additives in products is limited.
Antimicrobial materials act in two different ways. Antimicrobials can be incorporated into the film or coated either on the surface of the film or on the surface of the food (in the form of edible film). In both cases, the substance may migrate partially or completely through gradual diffusion into the food or headspace (which is typical for essential oils, for example) where it exerts its protective action, or it may not migrate, acting only when the food is in contact with the surface of the film and the target microorganism comes into direct contact with the film (Vermeiren et al., 2002; Brockgreitens and Abbas, 2016) (Figure 3).
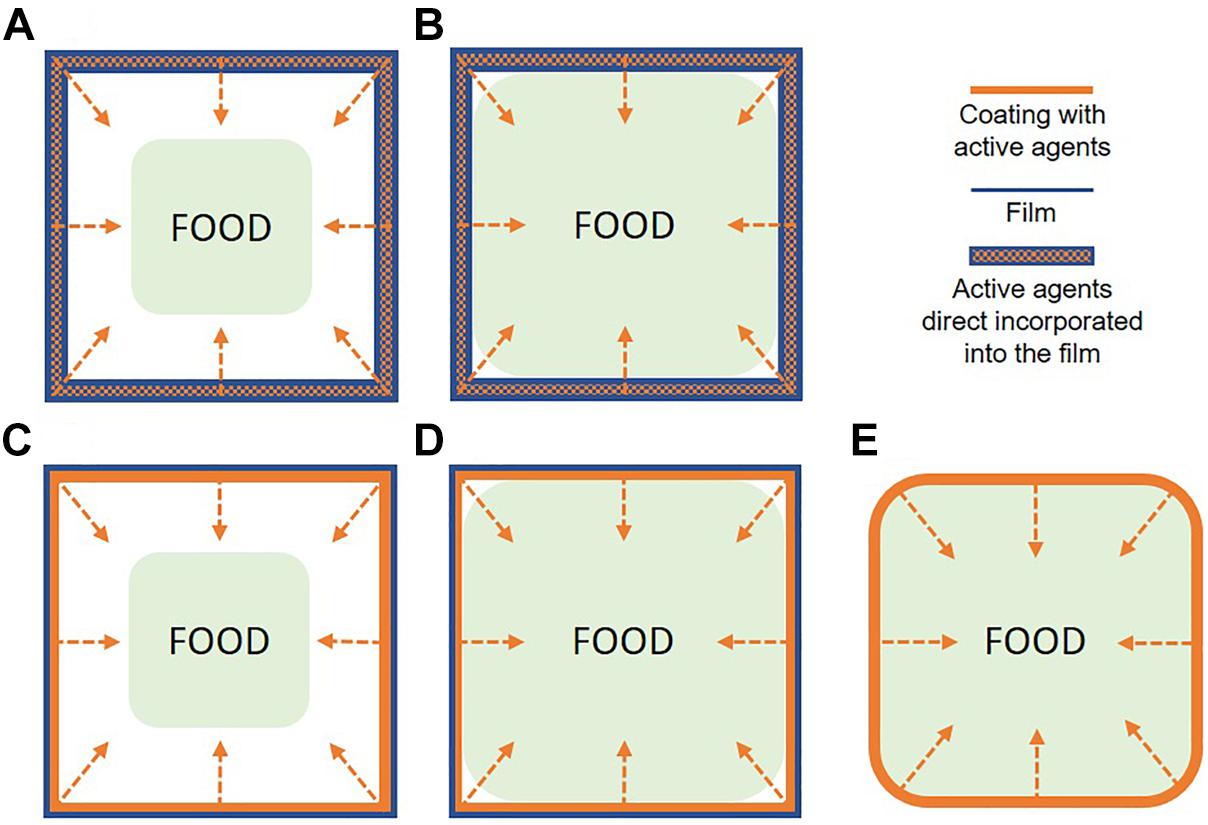
Figure 3. Different ways of incorporation and release of antimicrobial agents into food: (A) direct incorporation into the film before extrusion and migration via gradual diffusion from the material into the headspace; (B) direct incorporation into the film before extrusion and migration via gradual diffusion from the material into the food through direct contact; (C) surface coating on the film and migration via gradual diffusion from the material into the headspace; (D) surface coating on the film and migration via gradual diffusion from the material into the food through direct contact; (E) edible film and migration via gradual diffusion from the material into the food through direct contact.
In both the cases, this kind of packaging is called active packaging [Regulation (EC) No 450/2009 — active and intelligent materials and articles intended to come into contact with food].
Antimicrobial agents used for the preservation of foods are either chemically synthesized or extracted from biomass of plants, animals and microorganisms. Conventional chemical preservatives, including ethanol and other alcohols, organic acids, and their salts (benzoates, propionates, and sorbates) are the predominant food preservatives thanks to their low price and facility to use. However, research has been focusing on replacing them with natural antimicrobial agents such as enzymes, bacteriocins, chitin and its derivative chitosan extracted from crustacean shells, natural extracts, and essential oils (Holley and Patel, 2005; Aider, 2010; Lei et al., 2014; van den Broek et al., 2015; Mlalila et al., 2018). Indeed, natural extracts (e.g., plant extracts or essential oils from different spices, plants, and fruits) have been recognized as potential antioxidant and antimicrobial agents. Some of the most successful examples of the incorporation of natural substances into films have involved grapefruit seed and green tea extracts, which have shown to be active as antioxidants and against different pathogens (e.g., Escherichia coli and Listeria spp.) (Wang and Rhim, 2016; Wrona et al., 2017). Cinnamaldehyde, derived from cinnamon, was also studied for its bioactivity against E. coli and Salmonella spp. (Ma Y. et al., 2018). Moreover, cinnamon oil in the PVA matrix showed repellent effect toward Plodia interpunctella larvae (Jo et al., 2015) and in PP film inhibited the formation of molds (Manso et al., 2015). However, clove and cinnamon in cassava starch films failed to show clear antimicrobial effect even though they reduced the water vapor transmission (Kechichian et al., 2010). Another example was provided by Seydim and Sarikus (2006) who tested edible films made of whey protein isolate loaded with rosemary, oregano and garlic essential oils against E. coli, Staphylococcus aureus, Salmonella enteritidis, Listeria monocytogenes, and Lactobacillus plantarum. Oregano proved to be the most effective against bacteria, while rosemary showed no effect.
The most popular technique to include natural extracts into the final film formulation is the extrusion (Gómez-Estaca et al., 2014). This technique involves the incorporation of the bioactive compounds before extrusion so that the high temperatures of extrusion (the exact values depend on the melting temperature of the processed polymer) allow their effective and homogeneous distribution in the film. However, this technique can often result in thermal degradation of the bioactive compounds and decrease in their activity. For instance, Ha et al. (2001) used high-temperature profile 160–190°C to extrude an antimicrobial LLDPE-based film resulting in high loss of grapefruit seed extract functionality up to complete loss of antimicrobial activity. For this reason, heat-sensitive bioactive agents (i.e., natural extracts) are preferably produced by non-heating method (e.g., electrospinning and surface coating). Among these methods, surface coating is a simple process based on low temperatures. However, this technique may suffer from poor adhesion to plastics and, if applied to make an active packaging, needs to be designed to be in direct contact with the food. Examples of antimicrobial-coated films include chitosan/essential oil-coated PP film (Torlak and Nizamlioğlu, 2011), cinnamaldehyde, garlic oil and rosemary oil-coated PP/LDPE film (Gamage et al., 2009), oregano essential oil and citral-coated PP/EVOH film (Muriel-Galet et al., 2013), chitosan-coated plastic film (Ye et al., 2008a, b), and thyme and oregano-coated LDPE. Interestingly, as reported by Valderrama Solano and de Rojas Gante (2012), antimicrobial films produced by elevated temperature processes showed better microbial inhibition compared to the ones obtained by the coating method. In particular, they found that antimicrobial films produced by extrusion method are more effective against E. coli, Salmonella typhimurium, and L. monocytogenes compared to ionizing-coated antimicrobial films with the identical amount of agent incorporated antimicrobial. The results suggest that the extrusion method allows a better incorporation of the active compounds on the polymer. Given the number of pros and cons highlighted by the literature for both the techniques, more studies comparing the efficacy of two methodologies will be needed in order to address future researches in this field. Indeed, there isn’t any large scale industrial production of active bio-based films yet. For this reason, an estimation of the cost of active films can be based on an average cost of commercial natural extract (about 100 €/kg even though it can greatly vary with extract type) and of PLA film (2 €/kg, see Table 2). Following the steps of the extrusion process, tested by Bassani et al. (2019), with the inclusion of natural extracts encapsulated with β-cyclodextrins (370 €/kg), an estimation of the final price of active films was done resulting in about 6.4 €/kg. It is useful to point out that this evaluation was made considering an addition of encapsulated extract equal to 2% wt as maximum (Bassani et al., 2019) and that this estimation already includes the costs necessary to encapsulate the extract by spray-drying technique.
Bio-Based Sensors
Bio-based sensors have at least one component from bio-based source which may either be the substrate (i.e., the plastics listed in the previous section) or the sensing element. Most of the sensors related to bio-based materials in food packaging are based on colorimetric detection of analytes.
Many fruits, berries, vegetables and flowers with colors covering practically the entire visible spectrum are dyed by natural compounds such as anthocyanins and curcumin known as natural pH indicators (Yoshida et al., 2009; Silva-Pereira et al., 2015; Choi et al., 2017; Dudnyk et al., 2018; Majdinasab et al., 2018; Saliu and Pergola, 2018; Zhai et al., 2018; Kurek et al., 2019). Upon protonation/deprotonation of these molecules, their delocalized electronic structure rearranges and the change of the total number of resonant electrons as well as their confinement result in a change of their color (Figure 4). For instance, Choi et al. (2017) demonstrated a pH sensor made of agar and potato starch with anthocyanin extracts from purple sweet potato that showed color variations at pH 2.0–10.0. Zhai et al. (2018) used a gelatin-gellan gum matrix with red radish anthocyanin having a slightly broader pH range from 2.0 to 12.0. In Table 4 more examples of bio-based sensors developed for food quality monitoring in recent years are listed.
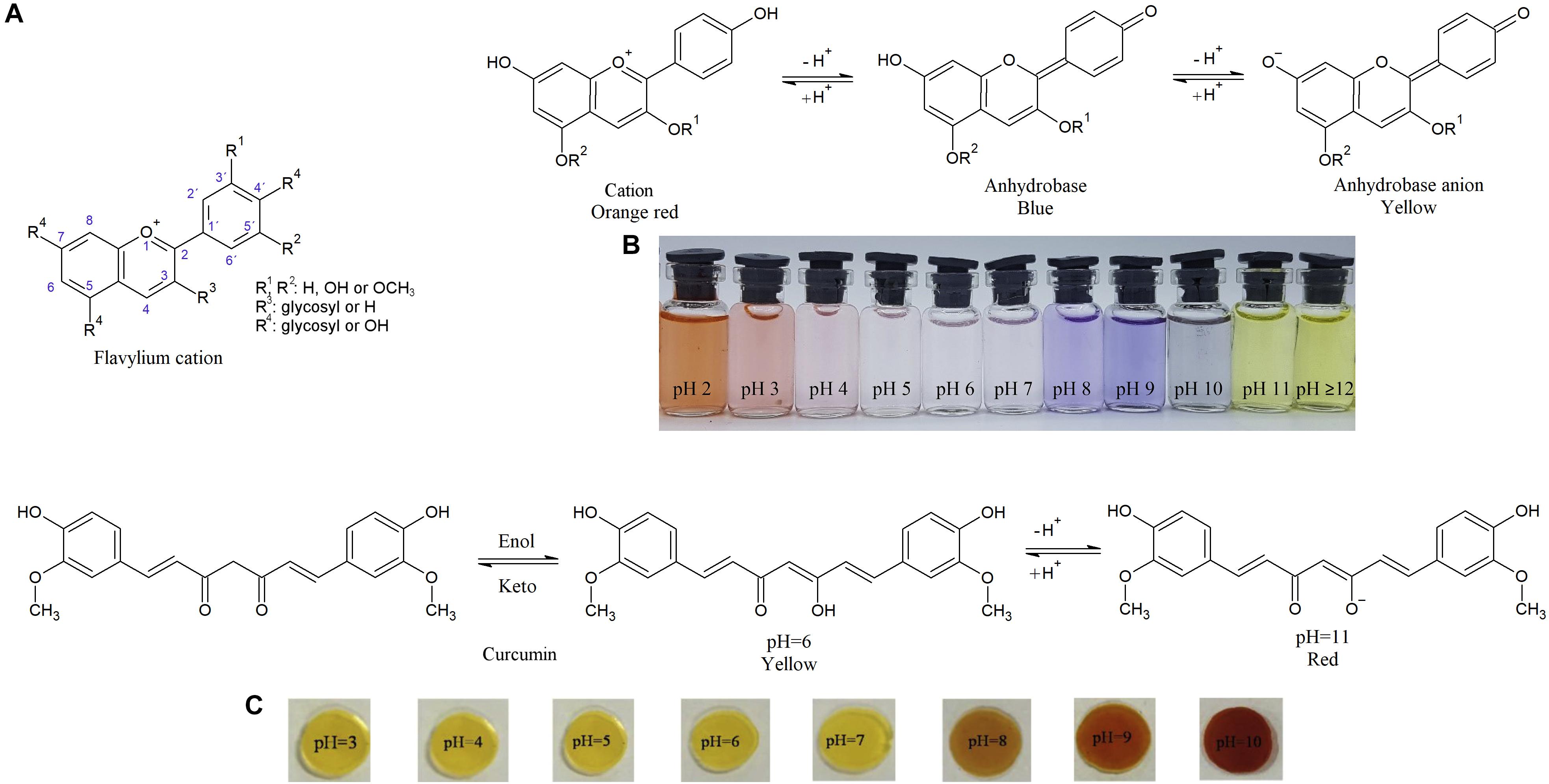
Figure 4. (A) Flavylium cation. In naturally occurring anthocyanidins, the terminating chemical groups (–H, –OH, and –OCH3) in the 3, 5, 6, 7 and the 3′, 4′, and 5′ positions determine the original color of the molecule. pH-sensitivity and color change of (B) pelargonidin and (C) curcumin. [(A) Reprinted from Phytochemistry 64(5), Kong et al. (2003). Copyright (2020) with permission from Elsevier. (B) Reprinted with permission from Zhai et al. (2018). Copyright (2020) American Chemical society. Reprinted from Spectrochim. Acta A 226, Chayavanich et al. (2020) Copyright (2020), with permission from Elsevier. (C) Reprinted from Food Hydrocolloid. 83, Liu et al. (2018) Copyright (2020), with permission from Elsevier].
Although colorimetric pH-sensitive sensors are typically not convenient for selective analysis, it is often sufficient to evaluate the food quality based on the change of the pH, as deteriorating proteins produce alkaline volatile nitrogen compounds (cadaverine, putrescine, histamine, and ammonia) (Bulushi et al., 2009; Prester, 2011). Exploiting this indirect sensing mechanism, curcumin based indicator films, e.g., in gelatin (Musso et al., 2017) and bacterial cellulose membranes (Kuswandi et al., 2012), blueberry and red grape skin pomace in chitosan and carboxymethyl cellulose matrix (Kurek et al., 2019), chitosan-corn starch film with red cabbage extract (Silva-Pereira et al., 2015), alginate beads with red cabbage extract (Majdinasab et al., 2018) as well as red cabbage extract in pectin films (Dudnyk et al., 2018) have been shown as feasible indicators of meat, shrimp and fish spoilage by detecting amines and cyclic N-containing compounds. In a similar way, acidic CO2 evolves during the metabolism of pathogens in the food thus lowering pH, which may be detected, e.g., by anthocyanin/polylysine in cellulose matrix in a reversible manner as demonstrated by Saliu and Pergola (2018).
Other natural dyes such as chlorophyll and β-carotene might be also relevant for sensing since both structures are highly sensitive to oxidative species. Silva et al. (2017) showed that replacing the coordinated Mg2+ with Zn2+ in chlorophyll A, the fluorescence of the complex is faded when increasing the concentration of dissolved oxygen in the medium. The mechanism of luminescence suppression is suggested to be caused by an energy transfer to oxygen molecules that collide with the excited molecule. In the case of carotene, one may exploit several mechanisms for sensing. β-carotene is prone to oxidation and subsequent decomposition to shorter cleavage products leading to a gradual disappearance of the orange color (Pénicaud et al., 2011).
Synthetic dyes based on various azo-compounds and polydiacetylenes also hold promise for chemical sensing in food packages. Azo-anthraquinone based dyes immobilized on paper (cellulose) as pH sensors working either in acidic or alkaline conditions, depending on the selected pigment, were shown by Zhang et al. (2019). Selective amine sensing colorimetric indicators utilizing trifluoroacetyl azobenzene dyes developed by Mohr (2004a; 2004b), Reinert and Mohr (2008); Kirchner et al. (2006) have been exploited in colorimetric and electrochemical detection of ammonia, ethylamine, cadaverine and putrescine (Lin et al., 2015). The carbonyl carbon of the trifluoroacetyl group is highly electron-deficient thus readily reacts with electron donors such as amines or alcohols. In the presence of amines (primary, secondary, or tertiary) it forms a hemiaminal group, i.e., the number of delocalized electrons in the diazobenzene backbone is decreased (so as the confinement length of electrons) resulting in a blue shift of optical absorption. Sensors printed on paper could detect vapors of the analytes having a concentration of 1.0–0.1 vol.%. Furthermore, highly sensitive ammonia sensors that operate even at very low temperatures (down to −20°C) were demonstrated by using polydiacetylenes that were polymerized in self-assembled vesicles stabilized with cellulose nanocrystals in the chitosan matrix. The sensing mechanism is based on a conformal change of the polydiacetylene backbone (from planar to non-planar) upon external stimuli such as pH, mechanical stress or temperature. Films of the sensors could detect 100 ppm ammonia (Nquyen et al., 2019) (Figure 5).
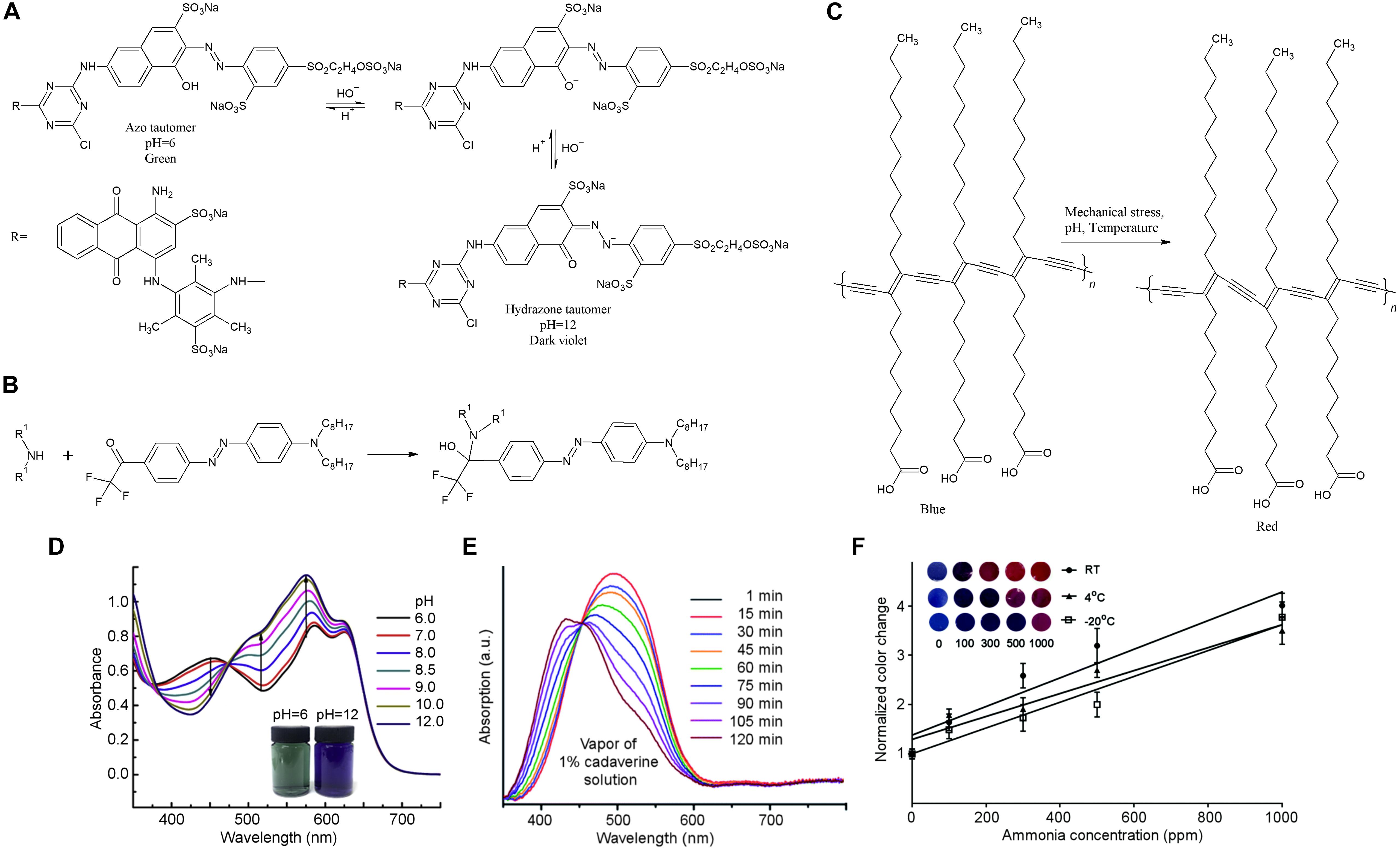
Figure 5. Reaction mechanisms of (A) anthraquinone and (B) trifluoroacetyl azo dyes and (C) polymerized self-assembled polydiacetylene vesicles and the corresponding color change during analyte sensing as displayed in panels (D–F), respectively. Note, that the normalized color change in panel (F) is the ratio of red and blue components in the RGB coordinates in reference to the original values. [(A,D) Reprinted from Sens. Actuat. B 286, Zhang et al. (2019) Copyright (2020), with permission from Elsevier. (B,E) Reproduced from Lin et al. (2015). (C,F) Reproduced from Nquyen et al. (2019) with permission from The Royal Society of Chemistry.].
Colorimetric sensors/indicators may be also accomplished by using enzymatic processes, in which the color change is typically a function of temperature and time. Capitalizing on these, Yan et al. (2008) developed a temperature indicator that combines the coloration of iodine-starch clathrates and the influence of temperature on the hydrolysis of starch in the presence of amylase enzyme. From the kinetic reaction rates of hydrolysis (and the corresponding coloration of the clathrates), one may assess the time-temperature history of cooled products. Another example of time-temperature indicator based on enzymatic oxidation of ABTS [2,20-azino bis-(3-ethyl benzthiazoline-6-sulphonic acid] substrate resulting in green color was demonstrated by Rani and Abraham (2006). By applying a fuse-type melting medium between peroxide and the mixture of the enzyme and substrate, the reaction starts only when the medium is warm enough to melt the separator thus enabling the mixing of the reactants. A similar melting fuse type of color indicator was proposed by Lorite et al. (2017), in which erythrosine B food dye printed on PLA was applied in a microfluidic device in conjunction with a frozen solvent. As soon as the temperature exceeds the melting point, the transparent solvent flows and dissolves the dye producing red staining of the device.
Other potentially viable food packaging sensors include electrical or RFID/NFC based (Lorite et al., 2017; Barandun et al., 2019) and electrochemical devices (Oliveira et al., 2013). Lorite et al. (2017) developed further their solvent melting point based colorimetric temperature sensor by using an electrically conductive film of carbon nanotubes being a part of an RFID tag. When the temperature reached the melting point of the solvent, it flowed through a capillary toward the nanotube film, soaked it and increased its resistance detected by the RFID reader. Very recently, an interesting and simple resistive chemical sensor for water-soluble gasses was proposed by Barandun et al. (2019) on cellulose substrates. As water is always present on the surface of the hygroscopic cellulose, when it is exposed to water-soluble gas analytes, the surface conductivity increases depending on the chemistry and concentration of the interacting moiety, which can be monitored by electrical measurements using carbon electrodes printed on the surface. The devices were highly sensitive to ammonia (down to 200 ppb) among the gasses tested (TMA, H2S, CO2, and CO) and were feasible for monitoring the headspace of meat and fish food packages. The sensors could be integrated into RFID tags and read by an NFC enabled smartphone (Figure 6).
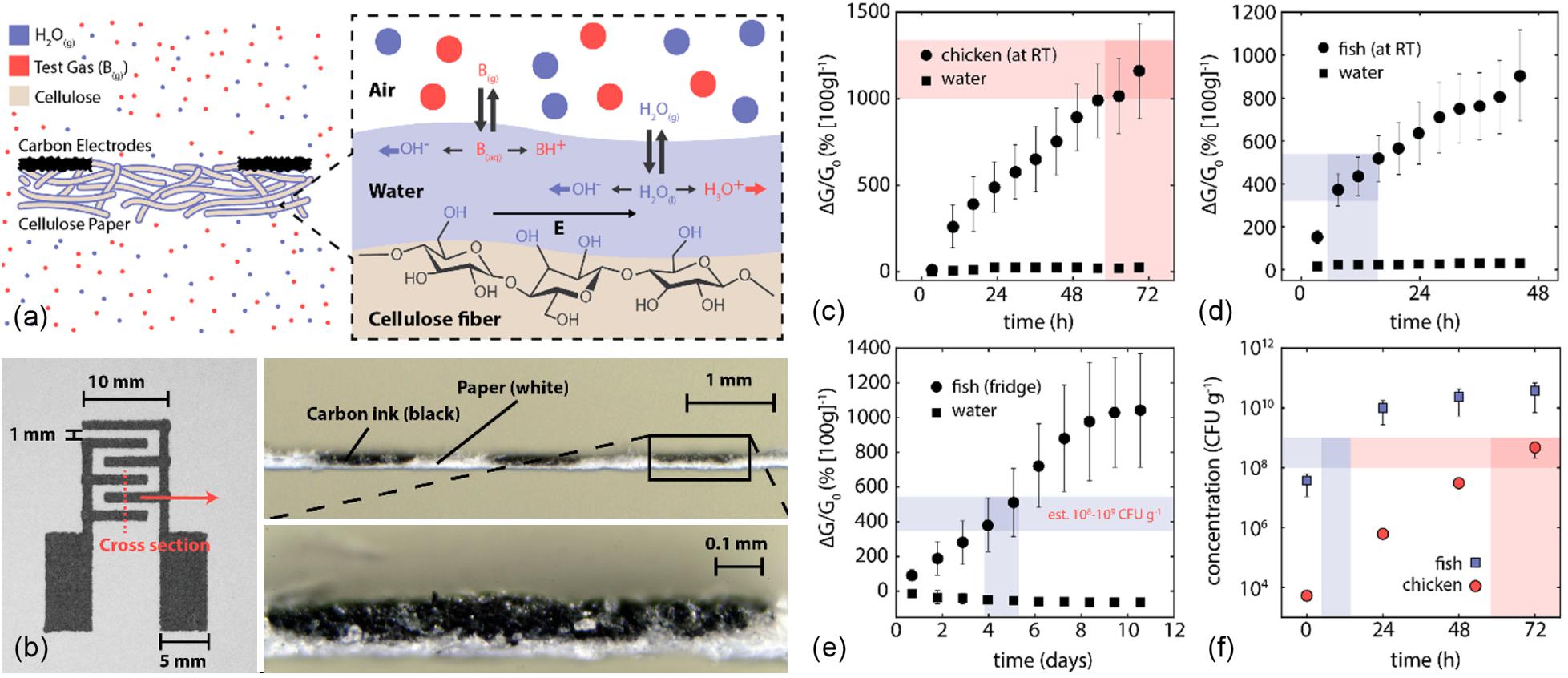
Figure 6. (A) Schematic diagram displaying the equilibrium of surface adsorbed water and vapor in the gas phase. Absorbed and then dissociated base increases the concentration of mobile ions in the liquid. (B) Optical images of the printed carbon electrodes on paper (top and cross-sectional views). Relative change of sensor conductance measured for decaying (C) meat and (D) fish at room temperature, and (E) fish at 4°C. The red and gray bands in the graphs correspond to the healthy limit of microbial contamination determined by microbial cultures displayed in panel (F). [Reproduced from Barandun et al. (2019) with permission from American Chemical Society].
The price of the bio-based food packaging sensors is directly connected to the price of foodstuff as many of them originate from natural sources and are edible. For example, the price of curcumin is around1 380 €/kg (February 2020) or for the wild blueberries the purchase price is typically between 2.5 and 4 €/kg (in Finland) but may rise up to 6–8 €/kg if the availability is limited due to dry summer, for example like in Finland in 2019. However, as pointed out earlier, edible food should not be used as raw material, but rather side streams should be valorized. Luckily the anthocyanin content is reasonable high in pomaces and seeds, so in principle agro-food waste can be used as source for sensor material lowering the price. If we compare the prices of food to prices of typical gas sensor materials, such as titanium dioxide with bulk price around 2–3 €/kg (2016–2017, Industrial Minerals), the anthocyanin source are competitive, especially because colorimetric sensors do not require complex electronics for the output and the waste management can be expected to be cheaper.
Conclusion
Bio-based smart food packaging will be one answer to the global challenges related to the desperate quest for carbon neutrality, food saving and safety, as well as for renewable materials and technologies. In this review, we have collected the contemporary literature on three key components of bio-based smart materials including (i) the packaging materials themselves responsible for providing a safe envelope for the products, (ii) advanced coatings and additives to help preserving foodstuff as well as (iii) renewable sensor materials with enabling technologies that can detect the quality of foods and are potentially feasible for industrial scale-up. Although the corresponding fields of scientific research on bio-based and renewable materials with robust production technologies are becoming more and more relevant today, it is clear that careful life cycle, economic and even user perception analyses have to be made to assess the real environmental and socioeconomic impact of each potentially viable solution.
Author Contributions
NH and KK contributed to the conception and design of the review. NH wrote the first draft of the manuscript. PP contributed with the literature search, illustrations, and writing of the manuscript. AB, CF, RN, and GS wrote sections of the manuscript. All authors contributed to manuscript revision, read and approved the submitted version.
Funding
The authors kindly acknowledge financial support from the EU Horizon 2020 – BBI JU under agreement no. 792261 NewPack, and EU Interreg Nord – Lapin liitto under agreement no. 20201468 Flexible transparent conductive films as electrodes.
Conflict of Interest
The authors declare that the research was conducted in the absence of any commercial or financial relationships that could be construed as a potential conflict of interest.
Abbreviations
Bio-PA, bio-polyamide; Bio-PBS, bio-polybutylene succinate; Bio-PE, bio-polyethylene; Bio-PET, polyethylene terephthalate containing bio-based materials; Bio-PP, bio-polypropylene; CA, cellulose acetate; FDCA, 2,5-furandicarboxylic acid; HDPE, high-density polyethylene; HIPS, high impact polystyrene; LDPE, low-density polyethylene; LLDPE, linear low-density polyethylene; MEG, mono-ethylene glycol; NFC, near-field communication; PA, polyamide; PBAT, poly(butylene adipate-co-terephthalate); PBS, polybutylene succinate; PBSA, polybutylene succinate adipate; PBST, poly(butylene succinate-co-terephthalate); PCL, polycaprolactone; PE, polyethylene; PEA, polyesteramide; PEF, polyethylene furanoate; PES, polyethersulfone; PET, polyethylene terephthalate; PHA, polyhydroxyalkanoate; PHB, polyhydroxybutyrate; PHBV, poly(hydroxybutyrate-co-valerate); PLA, polylactic acid; PP, polypropylene; PS, polystyrene; PTT, polytrimethylene terephthalate; PVA, polyvinyl alcohol; PVC, polyvinyl chloride; PVdC, polyvinylidene chloride; PVOH, polyvinyl alcohol; RFID, radio frequency identification; TA, terephthalic acid; TPS, thermoplastic starch; UV, ultra violet.
Footnotes
References
Abolghasemi, M. M., Sobhi, M., and Piryaei, M. (2016). Preparation of a novel green optical pH sensor based on immobilization of red grape extract on bioorganic agarose membrane. Sens. Actuat. B 224, 391–395. doi: 10.1016/j.snb.2015.10.038
Ackermann, J.-U., Müller, S., Lösche, A., Bley, T., and Babel, W. (1995). Methylobacterium rhodesianum cells tend to double the DNA content under growth limitations and accumulate PHB. J. Biotechnol. 39, 9–20. doi: 10.1016/0168-1656(94)00138-3
Ahmad, N. A., Heng, L. Y., Salam, F., Zaid, M. H. M., and Hanifah, S. A. (2019). A colorimetric pH sensor based on Clitoria sp and Brassica sp for monitoring of food spoilage using chromametry. Sensors 19, :4813. doi: 10.3390/s19214813
Ahmed, I., Lin, H., Zou, L., Li, Z., Brody, A. L., Qazi, I. M., et al. (2018). An overview of smart packaging technologies for monitoring safety and quality of meat and meat products. Packag. Technol. Sci. 31, 449–471. doi: 10.1002/pts.2380
Aider, M. (2010). Chitosan application for active bio-based films production and potential in the food industry: review. LWT— Food Sci. Technol. 43, 837–842. doi: 10.1016/j.lwt.2010.01.021
Arroyo, B. J., Santos, A. P., de Melo, E. D. A., Campos, A., Lins, L., and Boyano-Orozco, L. C. (2019). “Chapter 8 – Bioactive compounds and their potential use as ingredients for food and its application in food packaging,” in Bioactive Compounds: Health Benefits and Potential Applications, ed. M. R. S. Campos (Sawston: Woodhead Publishing), 143–156. doi: 10.1016/B978-0-12-814774-0.00008-6
Avérous, L. (2008). “Chapter 21 – Polylactic acid: synthesis, properties and applications,” in Monomers, Polymers and Composites from Renewable Resources, eds M. N. Belgacem and A. Gandini (Oxford: Elsevier), 433–450. doi: 10.1016/B978-0-08-045316-3.00021-1
Badia-Melis, R., Mc Carthy, U., Ruiz-Carcia, L., Carcia-Hierro, J., and Robla Villalba, J. I. (2018). New trends in cold chain monitoring applications – a review. Food Control 86, 170–182. doi: 10.1016/j.foodcont.2017.11.022
Barandun, G., Soprani, M., Naficy, S., Grell, M., Kasimatis, M., Chiu, K. L., et al. (2019). Cellulose fibers enable near-zero-cost electrical sensing of water-soluble gases. ACS Sens. 4, 1662–1669. doi: 10.1021/acssensors.9b00555
Bassani, A., Montes, S., Jubete, E., Palenzuela, J., Sanjuan, A. P., and Spigno, G. (2019). Incorporation of waste orange peels extracts into PLA films. Chem. Eng. Trans. 74, 1063–1068. doi: 10.3303/CET1974178
Bibi, F., Guillaume, C., Gontard, N., and Sorli, B. (2017). Wheat gluten, a bio-polymer to monitor carbon dioxide in food packaging: electric and dielectric characterization. Sens. Actuat. B 250, 76–84. doi: 10.1016/j.snb.2017.03.164
Branigan, T. (2008). Chinese Figures Show Fivefold Rise in Babies Sick from Contaminated Milk, The Guardian, 8 December. Available online at: https://www.theguardian.com/world/2008/dec/02/china (accessed December 12, 2019)Google Scholar
Brockgreitens, J., and Abbas, A. (2016). Responsive food packaging: recent progress and technological prospects. Compr. Rev. Food Sci. Food Safe. 15, 3–15. doi: 10.1111/1541-4337.12174
Bulushi, I. A., Poole, S., Deeth, H. C., and Dykes, G. A. (2009). Biogenic amines in fish: roles in intoxication, spoilage, and nitrosamine formation - – a review. Crit. Rev. Food Sci. 49, 369–377. doi: 10.1080/10408390802067514
Chayavanich, K., Thiraphibundet, P., and Imyim, A. (2020). Biocompatible film sensors containing red radish extract for meat spoilage observation. Spectrochim. Acta A. 226, 117601. doi: 10.1016/j.saa.2019.117601
Chen, G. Q., and Patel, M. K. (2012). Plastics derived from biological sources: present and future: a technical and environmental review. Chem. Rev. 112, 2082–2099. doi: 10.1021/cr200162d
Chiellini, E., Corti, A., D’Antone, S., and Solaro, B. (2003). Biodegradation of poly (vinyl alcohol) based materials. Prog. Polym. Sci. 28, 963–1014. doi: 10.1016/S0079-6700(02)00149-1
Choi, I., Lee, Y. J., Lacroix, M., and Han, J. (2017). Intelligent pH indicator film composed of agar/potato starch and anthocyanin extracts from purple sweet potato. Food Chem. 218, 122–128. doi: 10.1016/j.foodchem.2016.09.050
da Silva, C. K., da Silveira Mastrantonia, D. J., Costa, J. A. V., and de Morais, M. G. (2019). Innovative pH sensors developed from ultrafine fibers containing açaí (Euterpe oleracea) extract. Food Chem. 294, 397–404. doi: 10.1016/j.foodchem.2019.05.059
Dudnyk, I., Janeèek, E.-R., Vaucher-Joset, J., and Stellacci, F. (2018). Edible sensors for meat and seafood freshness. Sens. Actuat. B 259, 1108–1112. doi: 10.1016/j.snb.2017.12.057
European Bioplastics e.V (2019). What are Bioplastics?. Available online at: https://www.european-bioplastics.org/bioplastics/ (accessed December 13, 2019)Google Scholar
European Bioplastics e.V (2020). Bioplastics Market Data. Available online at: https://www.european-bioplastics.org/market/ (accessed January 20, 2020)Google Scholar
Europol (2015). Operation Opson IV – Case Stories. Available online at: https://www.europol.europa.eu/publications-documents/operation-opson-iv-case-stories (accessed December 12, 2019)Google Scholar
Ezati, P., Tajik, H., Moradi, M., and Molaei, R. (2019). Intelligent pH-sensitive indicator based on starch-cellulose and alizarin dye to track freshness of rainbow trout fillet. Int. J. Biol. Macromol. 132, 157–165. doi: 10.1016/j.ijbiomac.2019.03.173
Food and Agriculture Organization of the United Nations (2019a). Food Loss and Food Waste, The United Nations. Available online at: http://www.fao.org/food-loss-and-food-waste/en/ (accessed December 12, 2019)Google Scholar
Food and Agriculture Organization of the United Nations. (2019b). Sustainable Development Goals, 2 Zero Hunger, The United Nations. Available online at: http://www.fao.org/sustainable-development-goals/goals/goal-2/en/ (accessed December 12, 2019)Google Scholar
Fuertes, G., Soto, I., Carrasco, R., Vargas, M., Sabattin, J., and Lagos, C. (2016). Intelligent packaging systems: sensors and nanosensors to monitor food quality and safety. J. Sensors 2016, :4046061. doi: 10.1155/2016/4046061
Galstyan, V., Bhandari, M. P., Sberveglieri, V., Sberveglieri, G., and Comini, E. (2018). Metal oxide nanostructures in food applications: quality control and packaging. Chemosensors 6, :16. doi: 10.3390/chemosensors6020016
Gamage, G. R., Park, H. J., and Kim, K. M. (2009). Effectiveness of antimicrobial coated oriented polypropylene/polyethylene films in sprout packaging. Food Res. Int. 42, 832–839. doi: 10.1016/j.foodres.2009.03.012
Geueke, B. (2014). Dossier – Bioplastics as Food Contact Materials, Food Packaging Forum. doi: 10.5281/zenodo.33517 Available online at: https://www.foodpackagingforum.org/fpf-2016/wp-content/uploads/2015/11/FPF_Dossier06_Bioplastics.pdf (accessed December 13, 2019)
Ghaani, M., Cozzolino, C. A., Castelli, G., and Farris, S. (2016). An overview of the intelligent packaging technologies in the food sector. Trends Food Sci. Tech. 51, 1–11. doi: 10.1016/j.tifs.2016.02.008
Gómez-Estaca, J., López-de-Dicastillo, C., Hernández-Muñoz, P., Catalá, R., and Gavara, R. (2014). Advances in antioxidant active food packaging. Trends Food Sci. Tech. 35, 42–51. doi: 10.1016/j.tifs.2013.10.008
Grayhurst, P., and Girling, P. J. (2011). “Packaging of food in glass containers,” in Food and Beverage Packaging Technology, 2nd Edn, eds R. Coles and M. Kirwan (Hoboken, NJ: Blackwell Publishing Ltd), 137–156. doi: 10.1002/9781444392180.ch6
Ha, J. U., Kim, Y. M., and Lee, D. S. (2001). Multilayered antimicrobial polyethylene films applied to the packaging of ground beef. Packag. Technol. Sci. 14, 55–62. doi: 10.1002/pts.537
Holley, R. A., and Patel, D. (2005). Improvement in shelf-life and safety of perishable foods by plant essential oils and smoke antimicrobials. Food Microbiol. 22, 273–292. doi: 10.1016/j.fm.2004.08.006
Jamieson, A. J., Brooks, L. S. R., Reid, W. D. K., Piertney, S. B., Narayanaswamy, B. E., and Linley, T. D. (2019). Microplastics and synthetic particles ingested by deep-sea amphipods in six of the deepest marine ecosystems on Earth. Roy. Soc. Open Sci. 6, :180667. doi: 10.1098/rsos.180667
Jo, H.-J., Park, K.-M., Na, J. H., Min, S. C., Park, K. H., Chang, P.-S., et al. (2015). Development of anti-insect food packaging film containing a polyvinyl alcohol and cinnamon oil emulsion at a pilot plant scale. J. Stored Prod. Res. 61, 114–118. doi: 10.1016/j.jspr.2015.01.005
Kechichian, V., Ditchfield, C., Veiga-Santos, P., and Tadini, C. C. (2010). Natural antimicrobial ingredients incorporated in biodegradable films based on cassava starch. LWT— Food Sci. Technol. 43, 1088–1094. doi: 10.1016/j.lwt.2010.02.014
Kirchner, N., Zedler, L., Mayerhöfer, T. G., and Mohr, G. J. (2006). Functional liquid crystal films selectively recognize amine vapours and simultaneously change their colour. Chem. Commun. 14, 1512–1514. doi: 10.1039/B517768E
Kirwan, M. J. (2011). “Paper and paperboard packaging,” in Food and Beverage Packaging Technology, 2nd Edn, eds R. Coles and M. Kirwan (Hoboken, NJ: Blackwell Publishing Ltd), 213–250. doi: 10.1002/9781444392180.ch8
Kong, J.-M., Chia, L.-S., Goh, N.-K., Chia, T.-F., and Brouillard, R. (2003). Analysis and biological activities of anthocyanins. Phytochemistry 64, 923–933. doi: 10.1016/S0031-9422(03)00438-2
Koopman, F., Wierckx, N., de Winde, J. H., and Ruijssenaars, H. J. (2010a). Efficient whole-cell biotransformation of 5-(hydroxymethyl)furfural Into FDCA, 2,5-furandicarboxylic acid. Bioresour. Technol. 101, 6291–6296. doi: 10.1016/j.biortech.2010.03.050
Koopman, F., Wierckx, N., de Winde, J. H., and Ruijssenaars, H. J. (2010b). Identification and characterization of the furfural and 5-(hydroxymethyl)furfural degradation pathways of Cupriavidus basilensis HMF14. Proc. Natl. Acad. Sci. U.S.A. 107, 4919–4924. doi: 10.1073/pnas.0913039107
Kraus, F. J., and Tarulis, G. J. (2009). in Cans, Steel, Encyclopedia of Packaging Technology, 3rd Edn, ed. K. L. Yam (Hoboken, NJ: Wiley), 205–216.
Kuorwel, K. K., Cran, M. J., Orbell, J. D., Buddhadasa, S., and Bigger, S. W. (2015). Review of mechanical properties, migration, and potential applications in active food packaging systems containing nanoclays and nanosilver. Compr. Rev. Food Sci. Food Safe.. 14, 411–430. doi: 10.1111/1541-4337.12139
Kurek, M., Garofuliæ, I. E., Bakiæ, M. T., Šèetar, M., Uzelac, V. D., and Galiæ, K. (2018). Development and evaluation of a novel antioxidant and pH indicator film based on chitosan and food waste sources of antioxidants. Food Hydrocolloid. 84, 238–246. doi: 10.1016/j.foodhyd.2018.05.050
Kurek, M., Hlupiæ, L., Šèetar, M., Bosiljkov, T., and Galiæ, K. (2019). Comparison of two pH responsive color changing bio−based films containing wasted fruit pomace as a source of colorants. J. Food Sci. 84, 2490–2498. doi: 10.1111/1750-3841.14716
Kuswandi, B., Jayus, Larasati, T. S., Abdullah, A., and Heng, L. Y. (2012). Real-time monitoring of shrimp spoilage using on-package sticker sensor based on natural dye of curcumin. Food Anal. Methods 5, 881–889. doi: 10.1007/s12161-011-9326-x
Kuswandi, B., Wicaksono, Y., Jayus, Abdullah, A., Heng, L. Y., and Ahmad, M. (2011). Smart packaging: sensors for monitoring of food quality and safety. Sens. & Instrum. rumen. Food Qual. Safe. 5, 137–146. doi: 10.1007/s11694-011-9120-x
Lei, J., Yang, L., Zhan, Y., Wang, Y., Ye, T., Li, Y., et al. (2014). Plasma treated polyethylene terephthalate/polypropylene films assembled with chitosan and various preservatives for antimicrobial food packaging. Colloids. Surf. B: Biointerfaces. 114, 60–66. doi: 10.1016/j.colsurfb.2013.09.052
Liang, T., Sun, G., Cao, L., Li, J., and Wang, L. (2019). A pH and NH3 sensing intelligent film based on Artemisia sphaerocephala Krasch. gum and red cabbage anthocyanins anchored by carboxymethyl cellulose sodium added as a host complex. Food Hydrocoll.loid 87, 858–868. doi: 10.1016/j.foodhyd.2018.08.028
Liang, T., and Wang, L. (2018). A pH-sensing film from tamarind seed polysaccharide with litmus lichen extract as an indicator. Polymers 10, :13. doi: 10.3390/polym10010013
Lin, J.-F., Kukkola, J., Sipola, T., Raut, D., Samikannu, A., Mikkola, J.-P., et al. (2015). Trifluoroacetylazobenzene for optical and electrochemical detection of amines. J. Mater. Chem. A 3, 4687–4694. doi: 10.1039/C4TA05358C
Liu, B., Xu, H., Zhao, H., Liu, W., Zhao, L., and Li, Y. (2017). Preparation and characterization of intelligent starch/PVA films for simultaneous colorimetric indication and antimicrobial activity for food packaging applications. Carbohyd. Polym. 157, 842–849. doi: 10.1016/j.carbpol.2016.10.067
Liu, J., Wang, H., Wang, P., Guo, M., Jiang, S., Li, X., et al. (2018). Films based on κ-carrageenan incorporated with curcumin for freshness monitoring. Food Hydrocoll.oid. 83, 134–142. doi: 10.1016/j.foodhyd.2018.05.012
Lorite, G. S., Selkälä, T., Sipola, T., Palenzuela, J., Jubete, E., Viñuales, A., et al. (2017). Novel, smart and RFID assisted critical temperature indicator for supply chain monitoring. J. Food Eng. 193, 20–28. doi: 10.1016/j.jfoodeng.2016.06.016
Luchese, C. L., Pavoni, J. M. F., Spada, J. C., and Tessaro, I. C. (2019). Influence of blueberry and jaboticaba agroindustrial residue particle size on color change of corn starch based films submitted to different pH values solutions. J. Renew. Mater. 7(3), 235–243, 2019. doi: 10.32604/jrm.2019.00033
Luchese, C. L., Sperotto, N., Spada, J. C., and Tessaro, I. C. (2017). Effect of blueberry agro-industrial waste addition to corn starch-based films for the production of a pH-indicator film. Int. J. Biol. Macromol. 104, 11–18. doi: 10.1016/j.ijbiomac.2017.05.149
Ma, Q., Du, L., and Wang, L. (2017a). Tara gum/polyvinyl alcohol-based colorimetric NH3 indicator films incorporating curcumin for intelligent packaging. Sens. Actuat. B 244, 759–766. doi: 10.1016/j.snb.2017.01.035
Ma, Q., Liang, T., Cao, L., and Wang, L. (2018). Intelligent poly (vinyl alcohol)-chitosan nanoparticles-mulberry extracts films capable of monitoring pH variations. Int. J. Biol. Macromol. 108, 576–584. doi: 10.1016/j.ijbiomac.2017.12.049
Ma, Q., Ren, Y., Gu, Z., and Wang, L. (2017b). Developing an intelligent film containing Vitis amurensis husk extracts: the effects of pH value of the film-forming solution. J. Clean. Prod. 166, 851–859. doi: 10.1016/j.jclepro.2017.08.099
Ma, Y., Li, L., and Wang, Y. (2018). Development of PLA-PHB-based biodegradable active packaging and its application to salmon. Packag. Technol. Sci. 31, 739–746. doi: 10.1002/pts.2408
Machado, P. G., Walter, A., and Cunha, M. (2016). Bio−based propylene production in a sugarcane biorefinery: a techno−economic evaluation for Brazilian conditions. Biofuel. Bioprod. Bior. 10, 623–633. doi: 10.1002/bbb.1674
Maftoonazad, N., and Ramaswamy, H. (2019). Design and testing of an electrospun nanofiber mat as a pH biosensor and monitor the pH associated quality in fresh date fruit (Rutab). Polym. Test. 75, 76–84. doi: 10.1016/j.polymertesting.2019.01.011
Majdinasab, M., Hosseini, S. M. H., Sepidname, M., Negahdarifar, M., and Li, P. (2018). Development of a novel colorimetric sensor based on alginate beads for monitoring rainbow trout spoilage. J. Food Sci. Technol. 55, 1695–1704. doi: 10.1007/s13197-018-3082-5
Manso, S., Becerril, R., Nerín, C., and Gómez-Lus, R. (2015). Influence of pH and temperature variations on vapor phase action of an antifungal food packaging against five mold strains. Food Contr. 47, 20–26. doi: 10.1016/j.foodcont.2014.06.014
Medina-Jaramillo, C., Ochoa-Yepes, O., Bernal, C., and Famá, L. (2017). Active and smart biodegradable packaging based on starch and natural extracts. Carbohyd. Polym. 176, 187–194. doi: 10.1016/j.carbpol.2017.08.079
Mlalila, N., Hilonga, A., Swai, H., Devlieghere, F., and Ragaert, P. (2018). Antimicrobial packaging based on starch, poly (3-hydroxybutyrate) and poly (lactic-co-glycolide) materials and application challenges. Trends Food Sci. Tech. 74, 1–11. doi: 10.1016/j.tifs.2018.01.015
Mohr, G. J. (2004a). Chromo- and fluororeactands: indicators for detection of neutral analytesby using reversible covalent-bond chemistry. Chem. Eur. J. 10, 1082–1090. doi: 10.1002/chem.200305524
Mohr, G. J. (2004b). Tailoring the sensitivity and spectral properties of a chromoreactand for the detection of amines and alcohols. Anal. Chim. Acta 508, 233–237. doi: 10.1016/j.aca.2003.12.005
Muncke, J. (2012). Food Packaging Materials, Food Packaging Forum. Available online at: https://www.foodpackagingforum.org/food-packaging-health/food-packaging-materials (accessed December 13, 2019)Google Scholar
Muriel-Galet, V., Cerisuelo, J. P., López-Carballo, G., Aucejo, S., Gavara, R., and Hernández-Muñoz, P. (2013). Evaluation of EVOH-coated PP films with oregano essential oil and citral to improve the shelf-life of packaged salad. Food Control 30, 137–143. doi: 10.1016/j.foodcont.2012.06.032
Musso, Y. S., Salgado, P. R., and Mauri, A. N. (2017). Smart edible films based on gelatin and curcumin. Food Hydrocoll.oids 66, 8–15. doi: 10.1016/j.foodhyd.2016.11.007
Musso, Y. S., Salgado, P. R., and Mauri, A. N. (2019). Smart gelatin films prepared using red cabbage (Brassica oleracea L.) extracts as solvent. Food Hydrocoll.oid. 89, 674–681. doi: 10.1016/j.foodhyd.2018.11.036
Mustafa, F., and Andreescu, S. (2018). Chemical and biological sensors for food-quality monitoring and smart packaging. Foods 7, :168. doi: 10.3390/foods7100168
Narwade, V. N., Anjum, S. R., Kokol, V., and Khairnar, R. S. (2019). Ammonia-sensing ability of differently structured hydroxyapatite blended cellulose nanofibril composite films. Cellulose 26, 3325–3337. doi: 10.1007/s10570-019-02299-y
Niaounakis, M. (2013). Biopolymers: Reuse, Recycling and Disposal, 1st Edn. Amsterdam: Elsevier. doi: 10.1007/s10570-019-02299-y
Nquyen, L. H., Naficy, S., McConchie, R., Dehghani, F., and Chandrawati, R. (2019). Polydiacetylene-based sensors to detect food spoilage at low temperatures. J. Mater. Chem. C 7, 1919–1926. doi: 10.1039/c8tc05534c
Oldring, P. K. T., and Nehring, U. (2007). Packaging Materials; 7. Metal Packaging for Foodstuffs, The International Life Sciences Institute. Available online at: < https://www.pac.gr/bcm/uploads/7-metal-packaging-for-foodstuffs.pdf (accessed 13 December 13, 2019)Google Scholar
Oliveira, T. M., Fátima Barroso, M., Morais, S., de Lima-Neto, P., Correia, A. N., Oliveira, M. B., et al. (2013). Biosensor based on multi-walled carbon nanotubes paste electrode modified with laccase for pirimicarb pesticide quantification. Talanta 106, 137–143. doi: 10.1016/j.talanta.2012.12.017
Peelman, N., Ragaert, P., De Meulenaer, B., Adons, D., Peeters, R., Cardon, L., et al. (2013). Application of bioplastics for food packaging. Trends Food Sci. Tech. 32, 128–141. doi: 10.1016/j.tifs.2013.06.003
Pénicaud, C., Achir, N., Dhuique-Mayer, C., Dornier, M., and Bohoun, P. (2011). Degradation of β-carotene during fruit and vegetable processing or storage: reaction mechanisms and kinetic aspects: a review. Fruits 66, 417–440. doi: 10.1051/fruits/2011058
Pereira, P. F., and Andrade, C. T. (2017). Optimized pH-responsive film based on a eutectic mixture-plasticized chitosan. Carbohyd. Polym. 165, 238–246. doi: 10.1016/j.carbpol.2017.02.047
Plackett, D. V., Holm, V. K., Johansen, P., Ndoni, S., Nielsen, P. V., Sipilainen-Malm, T., et al. (2006). Characterization of L-potylactide and L-polylactide-polycaprolactone co-polymer films for use in cheese-packaging applications. Packag. Technol. Sci. 19, 1–24. doi: 10.1002/pts.704
PlasticsEurope. (2019a). Plastics – The Facts 2019. Available online at: https://www.plasticseurope.org/application/files/1115/7236/4388/FINAL_web_version_Plastics_the_facts2019_14102019.pdf (accessed December 13, 2019)Google Scholar
PlasticsEurope. (2019b). Plastics in Packaging. Available online at: https://www.plasticseurope.org/en/about-plastics/packaging (accessed December 13, 2019)Google Scholar
Pourjahaver, S., Almasi, H., Meshkini, S., Pirsa, S., and Parandi, E. (2017). Development of a colorimetric pH indicator based on bacterial cellulose nanofibers and red cabbage (Brassica oleraceae) extract. Carbohyd. Polym. 156, 193–201. doi: 10.1016/j.carbpol.2016.09.027
Prester, L. (2011). Biogenic amines in fish, fish products and shellfish: a review. Food Addit. Contam. A 28, 1547–1560. doi: 10.1080/19440049.2011.600728
Prietto, L., Mirapalhete, T. C., Pinto, V. Z., Hoffmann, J. F., Vanier, N. L., Lim, L.-T., et al. (2017). pH-sensitive films containing anthocyanins extracted from black bean seed coat and red cabbage. LWT- Food Sci. Technol. 80, 492–500. doi: 10.1016/j.lwt.2017.03.006
Rani, D. N., and Abraham, T. E. (2006). Kinetic study of a purified anionic peroxidase isolated from Eupatorium odoratum and its novel application as time temperature indicator for food materials. J. Food Eng. 77, 594–600. doi: 10.1016/j.jfoodeng.2005.07.018
Reinert, S., and Mohr, G. J. (2008). Chemosensor for the optical detection of aliphatic amines and diamines. Chem. Commun 19, 2272–2274. doi: 10.1039/B717796H
Reingardt, T., and Nieder, N. F. (2009). in Cans, Aluminium, Encyclopedia of Packaging Technology, 3rd Edn, ed. K. L. Yam (Hoboken, NJ: Wiley), 193–195.
Robertson, G. L. (2012). Food Packaging: Principles and Practice, 3rd Edn. Boca Raton, FL: CRC Press.
Saliu, F., and Pergola, R. D. (2018). Carbon dioxide colorimetric indicators for food packaging applications: applicability of anthocyanin and poly-lysine mixtures. Sens. Actuat. B 258, 1117–1124. doi: 10.1016/j.snb.2017.12.007
Sarfraz, J., Määttänen, A., Ihalainen, P., Keppeler, M., Lindén, M., and Peltonen, J. (2012). Printed copper acetate based H2S sensor on paper substrate. Sens. Actuat. B 173, 868–873. doi: 10.1016/j.snb.2012.08.008
Schut, J. H. (2016). Pioneering Sustainability; biopolymer applications are expanding, in fitsand starts. Plast. ics Eng.ineering 72, 6–13. doi: 10.1002/j.1941-9635.2016.tb01490.x
Šešlija, S., Nešiæ, A., Škoriæ, M. L., Krušiæ, M. K., Santagata, G., and Malinconico, M. (2018). Pectin/carboxymethylcellulose films as a potential food packaging material. Macromol. Symp. 378, :1600163. doi: 10.1002/masy.201600163
Seydim, A. C., and Sarikus, G. (2006). Antimicrobial activity of whey protein based edible films incorporated with oregano, rosemary and garlic essential oils. Food Res. Intl. 39, 639–644. doi: 10.1016/j.foodres.2006.01.013
Shukla, V., Kandeepan, G., Vishnuraj, M. R., and Soni, A. (2016). Anthocyanins based indicator sensor for intelligent packaging application. Agric. Res 5, 205–209. doi: 10.1007/s40003-016-0211-0
Silva, E. B., Ferreira, P. V., Chretien, J. B., Miranca, J. I. S., Pinho, H. A., Timbó, ÁP., et al. (2017). Green optical dissolved oxygen sensor based on a chlorophyll–zinc complex extracted from the plant Brassica oleracea L. Appl. Optics 36, 9951–9956. doi: 10.1364/AO.56.009951
Silva-Pereira, M. C., Teixeira, J. A., Pereira-Júnior, V. A., and Stefani, R. (2015). Chitosan/corn starch blend films with extract from Brassica oleraceae (red cabbage) as a visual indicator of fish deterioration. LWT— Food Sci. Technol. 61, 258–262. doi: 10.1016/j.lwt.2014.11.041
The United Nations. (2019). World Population Prospects 2019: Highlights, Department of Economic and Social Affairs. Available online at: https://www.un.org/development/desa/publications/world-population-prospects-2019-highlights.html (accessed December 18, 2019)Google Scholar
Torlak, E., and Nizamlioğlu, M. (2011). Antimicrobial effectiveness of chitosan-essential oil coated plastic films against foodborne pathogens. J. Plast. Film Sheet. 27, 235–248. doi: 10.1177/8756087911407391
Valderrama Solano, A. C., and de Rojas Gante, C. (2012). Two different processes to obtain antimicrobial packaging containing natural oils. Food Bioprocess. Tech. 5, 2522–2528. doi: 10.1007/s11947-011-0626-3
van Crevel, R. (2016). Bio-Based Food Packaging in Sustainable Development, Food and Agriculture Organization of the United Nations. Available online at: http://www.fao.org/forestry/45849-023667e93ce5f79f4df3c74688c2067cc.pdf (accessed 17 December 2019)Google Scholar
van den Broek, L. A. M., Knoop, R. J. I., Kappen, F. H. J., and Boeriu, C. G. (2015). Chitosan films and blends for packaging material. Carbohydr. Polym. 116, 237–242. doi: 10.1016/j.carbpol.2014.07.039
van den Oever, M., Molenveld, K., van der Zee, M., and Bos, H. (2017). Bio-Based and Biodegradable Plastics : Facts and Figures : Focus on Food Packaging in the Netherlands, Wageningen Food & Biobased Research No. 1722. Wageningen: Food & & Biobased Research, doi: 10.18174/408350
Vanderroost, M., Ragaert, P., Devlieghere, F., and De Meulenaer, B. (2014). Intelligent food packaging: the next generation. Trends Food Sci. Tech. 39, 47–62. doi: 10.1016/j.tifs.2014.06.009
Vermeiren, L., Devlieghere, F., and Debevere, J. (2002). Effectiveness of some recent antimicrobial packaging concepts. Food Addit. Contam. 19, 163–171. doi: 10.1080/02652030110104852
Wang, L. F., and Rhim, J. W. (2016). Grapefruit seed extract incorporated antimicrobial LDPE and PLA films: effect of type of polymer matrix. LWT- Food Sci. Technol. 74, 338–345. doi: 10.1016/j.lwt.2016.07.066
Weinmann, R. C., and Cotton, R. A. (1958). United States Patent Office Patent Number 2,849,319. Available online at: https://patentimages.storage.googleapis.com/da/c8/a2/5bacc8e97bef21/US2849319.pdf (accessed December 15, 2019).
World Economic Forum, Ellen MacArthur Foundation and McKinsey Company. (2016). The New Plastics Economy — Rethinking the Future of Plastics. Available online at: https://www.ellenmacarthurfoundation.org/publications/the-new-plastics-economy-rethinking-the-future-of-plastics (accessed December 13, 2019)Google Scholar
World Health Organization. (2019). Food Safety, The United Nations. Available online at: https://www.who.int/news-room/fact-sheets/detail/food-safety (accessed 12 December 12, 2019)Google Scholar
Wrona, M., Nerín, C., Alfonso, M. J., and Caballero, M. Á (2017). Antioxidant packaging with encapsulated green tea for fresh minced meat, Innov. Food Sci. Emerg. 41, 307–313. doi: 10.1016/j.ifset.2017.04.001
Xiaowei, H., Xiaobo, Z., Jiewen, Z., Jiyong, S., Zhihua, L., and Tingting, S. (2015). Monitoring the biogenic amines in Chinese traditional salted pork in jelly (Yao−meat) by colorimetric sensor array based on nine natural pigments. Int. J. Food Sci. Technol. 50, 203–209. doi: 10.1111/ijfs.12620
Yam, K. L., Takhistrov, P. T., and Miltz, J. (2005). Intelligent packaging: concepts and applications. J. Food. Sci. 70, R1–R10. doi: 10.1111/j.1365-2621.2005.tb09052.x
Yan, S., Huawei, C., Limin, Z., Fazheng, R., Luda, Z., and Hengtao, Z. (2008). Development and characterization of a new amylase type time–temperature indicator. Food Control 19, 315–319. doi: 10.1016/j.foodcont.2007.04.012
Yanxiao, L., Xiao-bo, Z., Xiao-wei, H., Ji-yong, S., Jie-wen, Z., Holmes, M., et al. (2015). A new room temperature gas sensor based on pigment-sensitized TiO2 thin film for amines determination. Biosens. Bioelectron. 67, 35–41. doi: 10.1016/j.bios.2014.05.040
Ye, M., Neetoo, H., and Chen, H. (2008a). Control of Listeria monocytogenes on ham steaks by antimicrobials incorporated into chitosan-coated plastic films. Food Microbiol. 25, 260–268. doi: 10.1016/j.fm.2007.10.014
Ye, M., Neetoo, H., and Chen, H. (2008b). Effectiveness of chitosan-coated plastic films incorporating antimicrobials in inhibition of Listeria monocytogenes on cold-smoked salmon. Int. J. Food Microbiol. 127, 235–240. doi: 10.1016/j.ijfoodmicro.2008.07.012
Yoshida, C. M. P., Maciel, V. B. V., Mendonça, M. E. D., and Franco, T. T. (2014). Chitosan biobased and intelligent films: monitoring pH variations. LWT- Food Sci. Technol. 55, 83–89. doi: 10.1016/j.lwt.2013.09.015
Yoshida, K., Mori, M., and Kondo, T. (2009). Blue flower color development by anthocyanins: from chemical structure to cell physiology. Nat. Prod. Rep. 26, 884–915. doi: 10.1039/b800165k
Yousefi, H., Su, H.-M., Imani, S. M., Alkhaldi, K., Filipe, C. D. M., and Didar, T. F. (2019). Intelligent food packaging: a review of smart sensing technologies for monitoring food quality. ACS Sens. 4, 808–821. doi: 10.1021/acssensors.9b00440
Zhai, X., Li, Z., Shi, J., Huang, X., Sun, Z., Zhang, D., et al. (2019). A colorimetric hydrogen sulfide sensor based on gellan gum-silver nanoparticles bionanocomposite for monitoring of meat spoilage in intelligent packaging. Food Chem. 290, 135–143. doi: 10.1016/j.foodchem.2019.03.138
Zhai, X., Li, Zhang, J., Shi, J., Zou, X., Huang, X., et al. (2018). Natural biomaterial-based edible and pH-sensitive films combined with electrochemical writing for intelligent food packaging. J. Agric. Food Chem. 66, 2836–12846. doi: 10.1021/acs.jafc.8b04932
Zhai, X., Shi, J., Zou, X., Wang, S., Jiang, C., Zhang, J., et al. (2017). Novel colorimetric films based on starch/polyvinyl alcohol incorporated with roselle anthocyanins for fish freshness monitoring. Food Hydrocolloid. 69, 308–317. doi: 10.1016/j.foodhyd.2017.02.014
Keywords: bio-based, smart packaging, food packaging, food safety, bio-based sensor
Citation: Halonen N, Pálvölgyi PS, Bassani A, Fiorentini C, Nair R, Spigno G and Kordas K (2020) Bio-Based Smart Materials for Food Packaging and Sensors – A Review. Front. Mater. 7:82. doi: 10.3389/fmats.2020.00082
Received: 20 December 2019; Accepted: 20 March 2020;
Published: 15 April 2020.
Edited by:
Zhengfei DAI, Xi’an Jiaotong University, ChinaReviewed by:
Ji-Wook Yoon, Chonbuk National University, South KoreaYing Ying Wang, University of Namur, Belgium
Yun Zheng, Jianghan University, China
Copyright © 2020 Halonen, Pálvölgyi, Bassani, Fiorentini, Nair, Spigno and Kordas. This is an open-access article distributed under the terms of the Creative Commons Attribution License (CC BY). The use, distribution or reproduction in other forums is permitted, provided the original author(s) and the copyright owner(s) are credited and that the original publication in this journal is cited, in accordance with accepted academic practice. No use, distribution or reproduction is permitted which does not comply with these terms.
*Correspondence: Niina Halonen, bmlpbmEuaGFsb25lbkBvdWx1LmZp