- 1School of Environment and Material Engineering, Yantai University, Yantai, China
- 2Shandong Institute for Food and Drug Control, Jinan, China
- 3CAS Key Laboratory for Biomedical Effects of Nanomaterials and Nanosafety, Institute of High Energy Physics, Chinese Academy of Sciences, Beijing, China
As an emerging energy storage device, the supercapacitor with high energy density, fast charging/discharging, and good cycle stability has aroused great interest. The performance of supercapacitors mainly depend on the electrode material. Manganese dioxide (MnO2) has emerged as one of the most promising electrode materials for high theoretical specific capacitance, wide potential range, high electrochemical activity, and environmental friendliness. However, its deteriorated volume expansion and inherently low conductivity limit its development and application in supercapacitors. To circumvent the mentioned issues, the porous, thin film, or layered composite materials were prepared to enhance the electrical conductivity and specific surface area of MnO2. Carbon materials are the ideal choice to compound with MnO2 owing to their low electrical resistance, significant thermal stability, large specific surface area, and porosity. Up to now, several kinds of MnO2/carbon composites as supercapacitor electrodes have been designed and fabricated. Herein, we give a concise review of the latest researches on MnO2/carbon supercapacitor electrodes, focusing on the fabrication strategies and analyzing the influencing factors of electrochemical performance of MnO2/carbon materials. An outlook on the possible development directions in future of designing high-performance MnO2/carbon materials for the current challenges is also provided.
Introduction
Nowadays, with the excessive consumption of traditional energy sources such as coal, oil, and natural gas, the increasingly severe global climate and deteriorating ecological environment have caused a global crisis that endangers human survival. The development and utilization of eco-friendly renewable energy become an extremely imminent task all over the world. In the past decade, solar, hydro, wind, and tidal energy and other renewable energy sources have greatly alleviated serious problems in the energy and environmental fields (Yang et al., 2011; Xie X. et al., 2019). However, abundant and clean renewable energy mentioned above cannot be widely applied directly owing to the limitation of natural conditions and the poor tunability and stability of generating electricity. Therefore, reliable electrochemical energy storage (EES), including fuel cells, ion batteries, and supercapacitors, is extremely necessary to achieve efficient storage, conversion, and further utilization of the above energy sources (Palchoudhury et al., 2019). The Ragone plots in Figure 1 shows the relationship between power density and energy density for several typical EES systems (Wang J. G. et al., 2015). Among them, electrochemical capacitors, which are also known as supercapacitors, are considered to be a new generation of green energy storage device (Díaz-Delgado and Doherty, 2016), with greater capacitance than conventional capacitors and higher output power and longer life than lithium-ion batteries (Zhang Q. Z. et al., 2018). Combined with its simple structure, high power density, fast charging, and pollution-free effects in the production process, supercapacitors are widely applied in portable electronics, data backup, hybrid electric vehicle, aerospace, and other fields (Salinas-Torres et al., 2019).
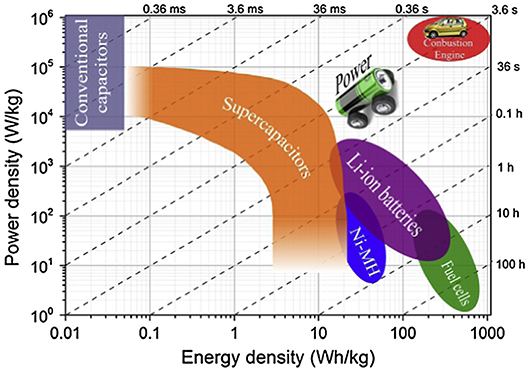
Figure 1. Ragone plots of various electrochemical energy storage (EES) systems. Reproduced from (Wang J. G. et al., 2015), with permission from Pergamon.
According to the charge storage mechanism, supercapacitors are generally classified as electrochemical double-layer capacitors (EDLCs) and pseudocapacitors (Wang G. P. et al., 2012). Energy storage and conversion of EDLCs are accomplished by static charge separation in the Helmholtz layer. Common active materials with double-layer electrodes are mostly carbon materials, which can rapidly complete the charging and discharging process and remain stable, but their capacitance and energy density are relatively low (<10 Wh kg−1) (Liang and Xin, 2015). The storage capacity of pseudocapacitors, compared with EDLCs, can be greatly improved owing to a series of reversible redox reactions on the surface/bulk phase of the electrode material (Conway, 1999). Transition metal oxides (Tajik et al., 2017) and conductive polymers (Snook et al., 2011) are commonly used as electrode materials for pseudocapacitors. The performance of supercapacitors is primarily dependent on the activity and kinetics of the electrode material. Therefore, it is very important to select suitable electrode materials, further optimize their structure, and improve their activity and kinetics to enhance the electrochemical properties of supercapacitors (Trudeau, 2013). Carbon materials and manganese dioxide (MnO2), as the most representative materials of EDLCs and pseudocapacitors, respectively, show their unique advantages in energy storage.
Carbon is one of the most closely and important elements for humans in nature and plays an important role in our existing ecosystems (Candelaria et al., 2012). It has various electronic orbital properties of sp, sp2, and sp3 hybridization, and the anisotropy of sp2 leads to various orientations of crystals and other arrays (Yu et al., 2018). Therefore, different kinds of carbon materials are formed by different chemical bonds. In particular, functional carbon materials considered to be ideal electrode materials for supercapacitors have attracted great attention owing to their low resistance, good electrical conductivity, high porosity, and large specific surface area (He et al., 2013; Borenstein et al., 2017). The EDLCs composed of carbon material form stable electric double layers by attracting mutually opposite charges at the electrode/electrolyte interface, which can achieve energy store through the physical electrostatic adsorption/desorption of charges. The energy storage mechanism is conducive to increasing the conductivity of the electrode to improve the specific capacity and energy density. Moreover, the carbon material can transform the structure and bonding mode of the composite material, thereby improving charging/discharging rate capability and cycle stability. For pure carbon materials, their specific capacitance and energy density (100~300 F g−1, 5~7 Wh kg−1) largely depends on specific surface area, porosity, and pore size distribution (Wang et al., 2019b). In recent years, a large number of functional carbon nanomaterials with related excellent properties, such as activated carbon (AC) (Faraji and Ani, 2015), graphene [including graphene oxide (GO) and reduced GO (rGO)] (Shi et al., 2018), carbon nanotubes (CNTs) (Qian et al., 2012), carbon nanofibers (CNFs) (Peng et al., 2016), and carbon aerogel (CA) (Hao et al., 2014) have emerged, which provide many favorable conditions for improving material properties. However, the specific capacitance and energy density of assembled EDLCs cannot compete with pseudocapacitors owing to their inherent electrostatic surface charging mechanism. Therefore, it is an effective way to prepare high-performance composite electrode materials by combining various functional carbon materials and typical metal oxides or conductive polymers.
Among many transition metal oxides, MnO2 with abundant reserves, low toxicity, and simple preparation process, is widely used in oxidation catalyst materials, aqueous batteries, supercapacitors, and other fields (Zhu M. et al., 2018; Li F. et al., 2019; Luo et al., 2019; Wei et al., 2019). Especially in the case of supercapacitors, MnO2 is considered to be one of the most promising electrode materials. Since Lee and Goodenough (1999) reported the pioneering work of amorphous MnO2 electrodes with excellent pseudocapacitive behavior in KCl electrolytes, a large number of efforts have been made to develop high-performance MnO2-based electrode materials. The reasons of the excellent performance of MnO2 can be summed up in two aspects. From the perspective of electrochemical properties, MnO2 displays outstanding characteristics of (a) a high theoretical capacity (1,370 F g−1) referring to the single-electron redox reaction of each manganese atom; (b) a wide potential window (0.9–1.0 V) (Xie Y. et al., 2019); and (c) excellent electrochemical properties in neutral electrolyte, leading to low chemical corrosion of the collector. From the perspective of environmental protection and economy, MnO2 is abundant in earth, has low price, and is environmentally friendly (Wei et al., 2011). These unique advantages make MnO2 an ideal choice for pseudocapacitor electrode materials. It is worth noting that MnO2 contains a variety of crystal structures, including α-, β-, γ-, δ-, and λ-MnO2; and its energy storage properties are determined by different crystal forms. Research has shown that the chain (Gao et al., 2017) or tunnel (Huang et al., 2019) structure of α-, β-, and γ-MnO2 with large two-dimensional tunneling structure facilitates electron transfer to provide a relatively high capacitance value. The large surface area of δ-MnO2 with a layered or sheet-like structure is more favorable for cation intercalation/deintercalation than the amorphous structure. The three-dimensional hinge structure of λ-MnO2 can provide more active sites for better electrochemical properties (Prélot et al., 2003; Malak-Polaczyk et al., 2010). However, the further development of current MnO2-based supercapacitors is limited by the drawbacks of MnO2 electrode materials, such as low conductivity, poor ion diffusion constant, and poor structural stability (Wang J. G. et al., 2015). Therefore, the improvement of active materials mainly involves high reversible capacitance, structural stability, and rapid cation diffusion at high charge/discharge rates. A useful and direct approach is to uniformly modify MnO2 materials onto layered porous conductive functional carbon materials to construct electrodes (Hu et al., 2018). The carbon materials can be served as a highly conductive and stable current collector, and its interconnection holes are beneficial for ion diffusion, whereas MnO2 can shorten the transmission distance of ions to prepare a high-performance electrode material.
In recent years, a large amount of significant breakthroughs have been made in the design of high-performance MnO2-based composite materials for application in supercapacitor devices. The number of research papers on MnO2/carbon composites for supercapacitors recently published is shown in Figure 2. In Figure 2a, the number of research papers on carbon/MnO2 composites has remained at a high level, which is still the research focus of electrode materials at present. And as shown in Figure 2b, recent work has shown that different types of carbon materials added to MnO2 material are mainly in forms of graphene and CNTs. This paper summarizes the synthesis strategies of MnO2/carbon materials with different morphologies and structures. The electrochemical properties and influencing factors of the electrode materials were further discussed. Finally, the remaining challenges of MnO2/carbon composite supercapacitors are briefly generalized, and an outlook on the possible development directions in the future for designing high-performance MnO2/carbon materials is also provided.
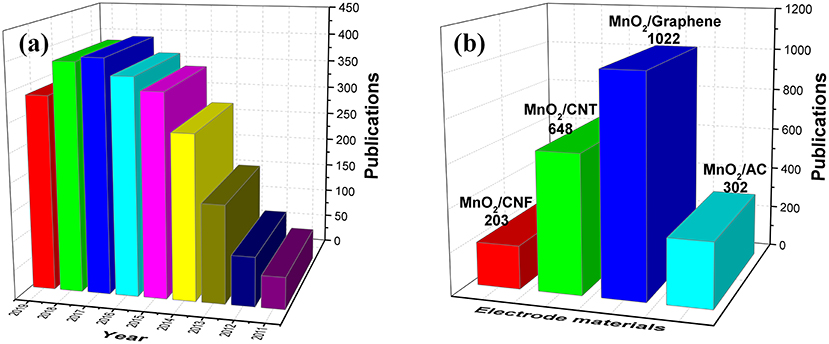
Figure 2. Statistical survey of research papers on MnO2/carbon composites for supercapacitors. (a) Total number of related reports from 2011 to 2019. (b) Literature statistics for different types of carbon materials/MnO2 composites. Source: Web of Science. Search date: August 20, 2019.
Synthesis And Properties of MnO2/Carbon Composites for Supercapacitor
MnO2/Carbon Nanotube Composites
CNTs are one-dimensional quantum materials with a hollow tubular structure and excellent electrical conductivity, large specific surface area, and high chemical stability (Lu et al., 2019). From the perspective of the wall structure, it can be divided into single-walled CNTs (SWCNTs) and multi-walled CNTs (MWCNTs), both of which have been widely used in the energy storage. For SWCNTs, the specific surface area can reach 240–1,250 m2 g−1, and pore size distribution is mostly in the wide range of 3 to 5 nm, with high specific capacitance of 180 F g−1, power density of 20 kW kg−1, and energy density of 6.5–7 Wh kg−1 (Fujiwara et al., 2001). And reduced electrode impedance and increased specific capacitance of SWCNTs can be obtained by the high-temperature heat treatment or addition of surfactants (Byl et al., 2005). For MWCNTs, the specific surface area (~430 m2 g−1), specific capacitance (~180 F g−1), power density (~8 kW kg−1), and energy density (~0.56 Wh kg−1) are slightly lower than those for SWCNTs (Fujiwara et al., 2001; Xie et al., 2017). In addition, carbon atoms in the CNTs are sp2 hybridized, forming a hexagonal network with the surrounding three carbon atoms, and its s orbital composition is relatively large compared with sp3 hybridization. The carbon atoms in the CNTs adopt sp2 hybridization, and the surrounding three carbon atoms form a hexagonal network, which is larger than the sp3 hybrid sulfur orbital composition. This contributed to the high mechanical strength, good flexibility, and excellent corrosion resistance and stability of CNTs that provide advantages for manufacturing flexible electrode. The introduction of carbon–carbon double bond, carboxyl group, hydroxyl group, hydrocarbon bond, and other functional groups on the surface of CNTs can improve their surface activity and help ions in electrolyte to enter into electrode materials. So far, the synthesis of CNTs has entered the commercial stage and production capacity of more than 1,000 tons (Xin and Wei, 2012). As a relatively mature nano-carbon material, the development of CNTs is of great scientific significance in the field of EES.
Considering that the specific capacitance of pure CNTs measuring 20–80 F g−1 (Dubey and Guruviah, 2019) is lower than that of other carbon materials (e.g., CNF is 120–370 F g−1 and AC is 100–300 F g−1) and acts more like a scaffold (Zhang and Zhao, 2009), scientists wisely combined MnO2 with CNTs to form nanocomposites. This composite material exerts a synergistic effect of electrical/mechanical benefits of CNTs and large pseudocapacitance of MnO2, achieving expected high specific capacity and long cycle durability. The preparation of MnO2/CNT composite electrode materials can be achieved by various synthetic methods such as electrodeposition techniques (Li Q. et al., 2014; Jeong et al., 2019), hydrothermal treatment (Ramesh et al., 2017), microwave-assisted methods (Yan et al., 2009; Li M. et al., 2017), chemical coprecipitation (Subramanian et al., 2006), and thermal decomposition (Liu et al., 2017; Bi et al., 2019). In 2006, amorphous δ-MnO2 and SWCNT composite electrode materials were first studied. As shown in Figures 3A,B, Subramanian et al. (2006) successfully prepared a δ-MnO2/SWCNT composite for supercapacitor electrodes using a simple precipitation method. Owing to the synergetic performance of double layer and pseudocapacitance, the δ-MnO2/20 wt% SWCNT composite exhibits an excellent capacitance value of 110 F g−1 at 2 A g−1 and maintains 75% capacity over 750 cycles. Further studies have found that the CNT content has a significant effect on the cycle life of the material in Figure 3C, so the reasonable ratio of CNTs and MnO2 is very critical for the electrochemical performance improvement of the composite. It is proved that the unique structure of CNTs is served as the conductive agent and support material, whereas MnO2 used as a supplier of pseudocapacitors can significantly improve the electrochemical performance of MnO2/CNT composites. This has led to a strong interest in MnO2/CNT composite electrode materials. (Li L. et al., 2019) developed MnO2-MWCNT by hydrothermal treatment directly on Ni foam for adhesive-free electrodes, as shown in Figure 3D. It can be seen from Figure 3E that ultrathin MnO2 nanosheets were uniformly grown on forest-like MWCNTs, covering the surface of the foamed nickel. The areal density, specific capacitance, and capacitance retention of the binderless electrode material prepared in this manner reached 0.775 mg cm−2, 1,350.42 F g−1 at 6.5 A g−1, and 93.9%, respectively (see Figure 3F). This derives from the direct and strong contact between the MWCNT and the current collector that achieves low charge transfer and enhances the electrochemical properties of the composites, indicating that the binderless approach can significantly improve the conductivity of the electrode material. With the great advancement of science and technology and the improvement of people's living needs, flexible and lightweight flexible electronic devices have attracted widespread attention for their soft, deformable, and easy-to-wear properties. To meet these needs, it is necessary to develop an electrode with good flexibility and excellent electrochemical performance. Wang L. et al. (2017) synthesized SWCNTs by low-crystallization hydrothermal treatment, and then mixed they with MnO2 to form an ordered network structure by vacuum filtration (see Figure 3G). The results show that the electrochemical properties of the materials can be improved by effectively regulating the regular fiber network structure. As is shown in Figures 3H,I, the area specific capacitance is 964 mF cm−2, and the capacity retention rate is 81% of the composite. The assembled hybrid supercapacitors also provide high energy densities of 31.8 μWh cm−2 at a power density of 0.815 mW cm−2, implying their great potential applications in flexible device. In addition, this review summarizes the electrochemical performance of some typical MnO2/CNT electrode materials for comparison (see Table 1).
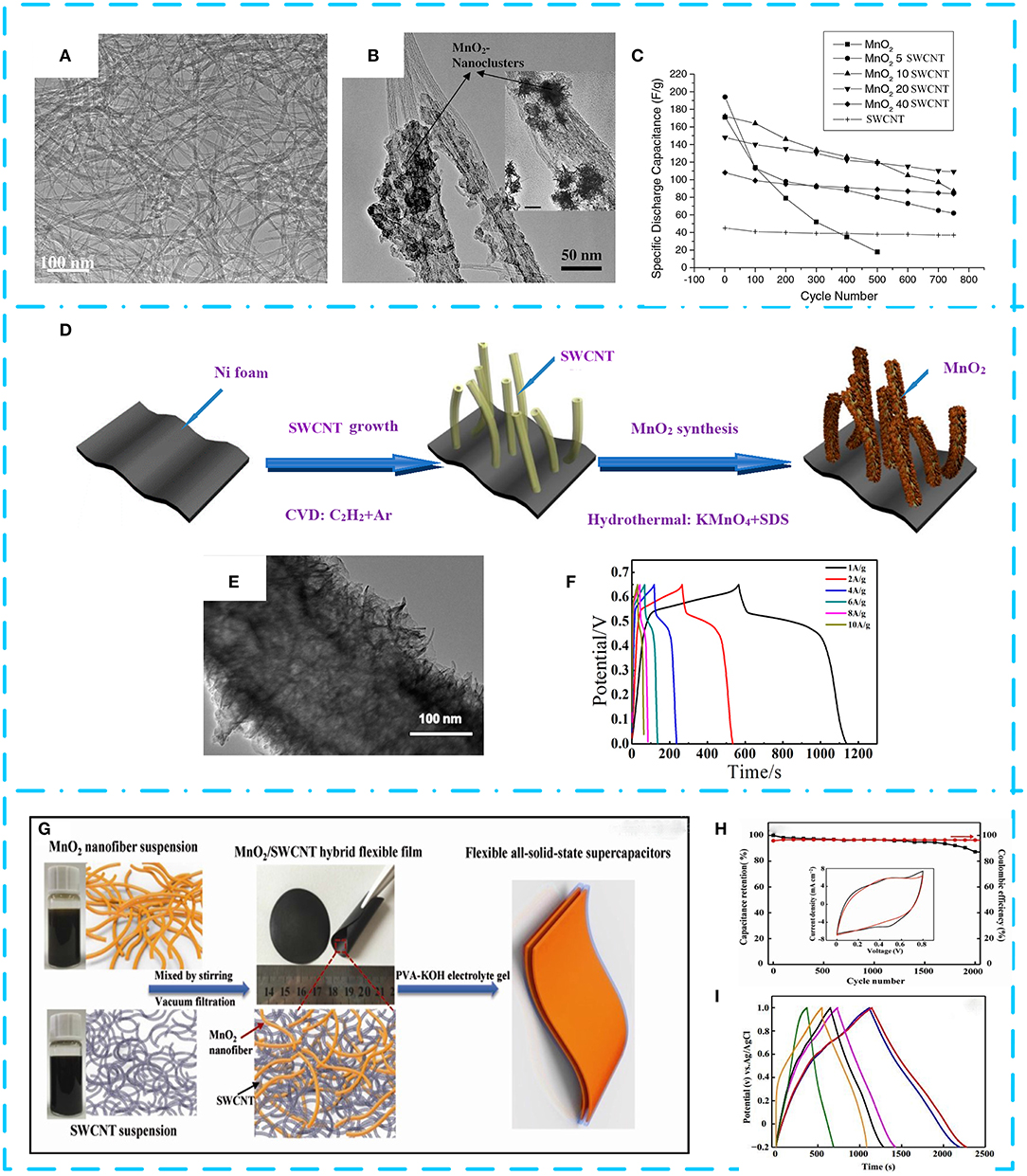
Figure 3. Transmission electron microscopy (TEM) pictures. (A) Pure single-walled carbon nanotube (SWCNT). (B) MnO2:20 wt% SWCNT composite. (C) Cyclic voltammograms of pure MnO2, pure SWCNT, and MnO2:20 wt% SWCNT composite at a scan rate of 2 mV s−1. Adapted from Subramanian et al. (2006) with permission from Elsevier Inc. (D) Production process of MnO2–multi-walled CNT (MWCNT)–Ni foam. (E) MnO2 synthesized uniformly on MWCNTs. (F) Charge/discharge curves at different current densities of the MnO2–MWCNT–Ni foam composite. Adapted from (Li L. et al., 2019) under the Creative Commons CC license. (G) Schematic preparation representation of the flexible all-solid-state δ-MnO2/SWCNT hybrid supercapacitor. (H) Cyclic voltammetry curves from 5 to 100 mV s−1. (I) Galvanostatic charge/discharge (GCD) curves of MnO2/SWCNT composites at a current density of 0.1 mA cm−2 in a 1 M Na2SO4 electrolyte. Reproduced from Wang L. et al. (2017) with permission from the Royal Society of Chemistry.
MnO2/Graphene Composites
Graphene is a two-dimensional carbon nanomaterial composed of hexagonal carbon atoms in a honeycomb lattice (Huang et al., 2011; Bayle et al., 2015). With its excellent physical and chemical properties, it has shown broad application prospects in the field of energy storage and conversion (Chen P. et al., 2018; Lu et al., 2018). Interest in the study of graphene has not been attenuated after Novoselov and Geim prepared a single layer of graphene by mechanical stripping at room temperature, breaking the prediction that quasi-two-dimensional crystal materials could not exist alone at room temperature (Novoselov et al., 2004). A two-dimensional planar structure of graphene can be regarded as a building unit for constituting other dimensional carbon materials, such as zero-dimensional fullerene, one-dimensional CNTs, and three-dimensional graphite (Geim and Novoselov, 2007). In addition, the three-dimensional graphene structure can also be constructed by its self-assembling or in combination with other materials. Thanks to its two-dimensional sheet structure that can be used to construct three-dimensional electrode materials with controllable structure, it can meet the needs of no conductive agent and binder addition. The excellent electron migration rate can promote electron transport during charging/discharging to improve electrochemical performance of electrode materials. Due to the special structure of graphene, the theoretically excellent characteristics, such as large specific surface area (~2,630 m2 g−1), excellent electrical conductivity, and excellent mechanical properties (resistance). Tensile strength of 130 GPa and stiffness of 1.5 × 108 psi, and good chemical and thermal stability (Ren et al., 2018) make it a considerable industrial material that most likely to achieves scale application in the short term for EES (Kannappan et al., 2018).
However, the volumetric energy density of most graphene-based electrode materials is very low, resulting in lower energy density of the electrodes. In order to make full use of the excellent properties of graphene, adding MnO2 to graphene has become a popular choice for most researchers. This can effectively prevent graphene from agglomeration owing to strong van der Waals force by the introduction of MnO2 between the sheets (Sheng et al., 2016). And the graphene can act as carbon skeleton that exerts an “elastic constraint” to prevent electrochemical dissolution of MnO2. MnO2/graphene composites prepared by microwave irradiation, low-temperature hydrothermal treatment, in situ reduction method, and electrospinning technology have stimulated their application potential in supercapacitors or other green energy devices. Yan et al. (2010) used a fast and simple microwave radiation method to deposit nanosized MnO2 on the surface of graphene (see Figures 4A,B). The capacitance characteristics of the graphene–MnO2 composite (78 wt% of MnO2) show that the measured specific capacitance is 310 F g−1 at 2 mV s−1 (Figure 4C). This is almost three times better than that of pure graphene (104 F g−1) and birnessite MnO2 (103 F g−1). Interestingly, the weight ratio of MnO2 in the composite has a great influence on the capacitance performance. As the mass ratio increases from 20 to 78%, the specific capacitance value increases significantly. Figure 4D displays schematic preparation process of an ordered MnO2-GO fiber composite supercapacitor electrode material by electrospinning (Saha et al., 2019). The MnO2 particles with an average diameter of 260 nm uniformly distributed on the surface of graphene in Figure 4E, and the composite exhibited excellent electrochemical performance. The specific capacitance of the sample reached 863.0 F g−1 at a current density of 9 A g−1, and the specific capacitance remained 88% of the initial value after 5,000 cycles (Figure 4F). By investigating the electrochemical properties, dielectric behavior, and impedance spectra of the samples, it was found that the ordered composites have higher diffusivity and charge mobility than the disordered MnO2. It is concluded that changing the crystallinity of MnO2 with increased electrical conductivity can improve the performance of GO/MnO2 active materials. As the research progressed, ternary MnO2 composites have been widely used. For example, Zhu G. et al. (2014) anchored MnO2 nanoflakes on a graphene–CNT hybrid substrate to form a three-dimensional hybrid material without a binder (Figures 4G,H). The hybrid structure completely maintains high conductivity and high surface with increase of volume ratio of CNTs, exhibiting a capacitance value of 251 F g−1 at 1 A g−1 (see Figure 4I). A highly conductive MnO2–CNT–graphene–Ni-foamed symmetrical supercapacitor delivers an energy density of 1,200 W kg−1 at a power density of 29 Wh kg−1. It is worth noting that the crystallinity, micromorphology, and mass ratio of MnO2 in nanocomposites have a significant effect on the electrochemical performance for supercapacitors. The friendly method under mild conditions is more conducive to the shape control of MnO2/graphene and the feasibility of expanding production. Furthermore, the control of oxygen-containing functional groups and heteroatom doping of graphene is also a way to manufacture high-performance nanocomposites. The modified nanocomposite can effectively change the electronic properties of graphene and further improve the performance. In addition, this review summarizes the literature on the recently published MnO2/graphene composites in Table 2.
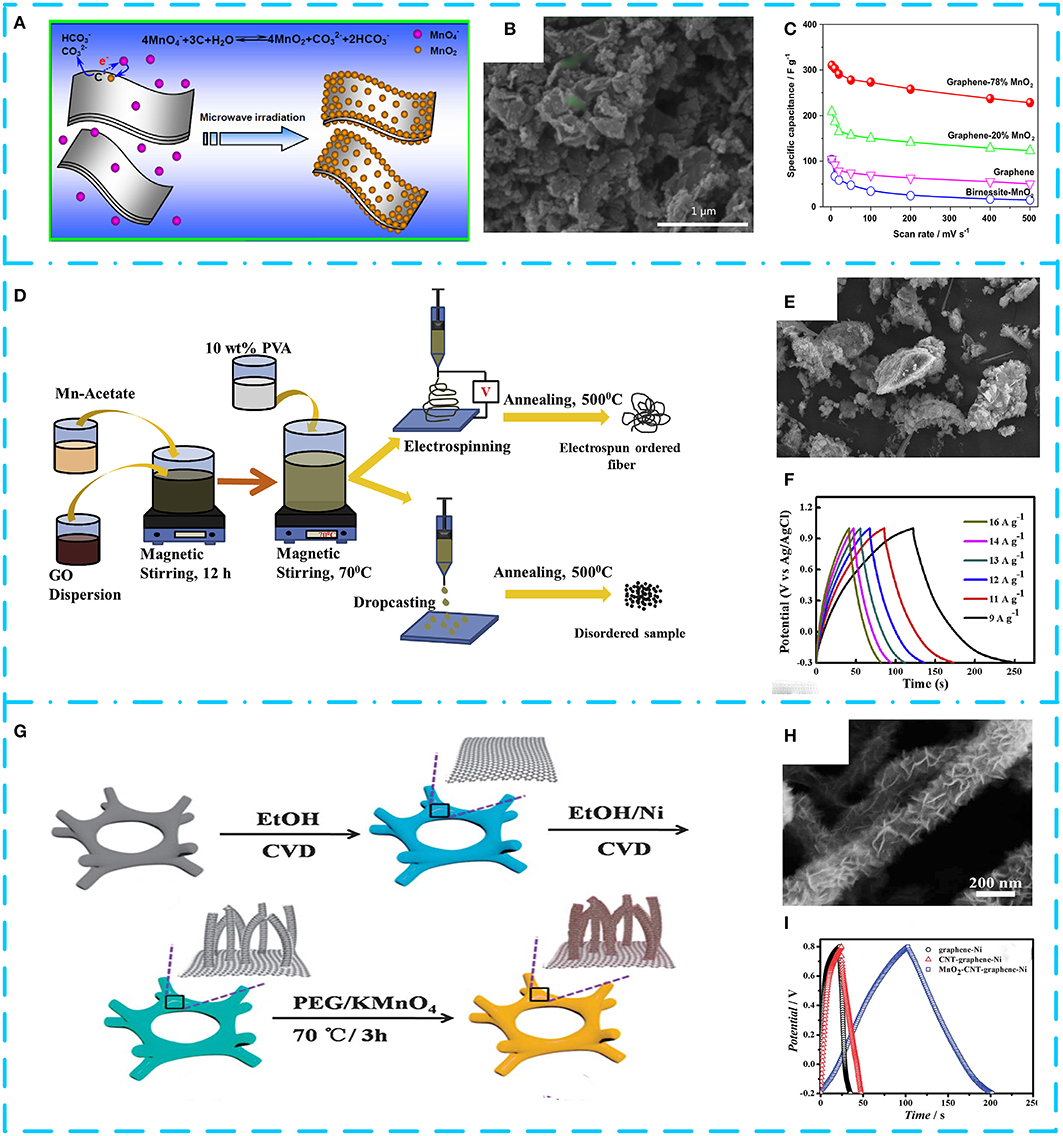
Figure 4. (A) Schematic illustration of the synthesis and electrochemical performance of graphene–MnO2 composite. (B) Low-magnification scanning electron microscopy (SEM) image of graphene−78% MnO2, showing the preferred growth of MnO2 near the edges of graphene. (C) Specific capacitance of composites at different scan rates of 2, 10, 20, 50, 100, 200, and 500 mV s−1. Reproduced from Yan et al. (2010) with permission from Pergamon. (D) Schematic of synthesis procedure. (E) SEM image. (F) Galvanostatic charge/discharge (GCD) curves at different current densities of MnO2/graphene−2D composites in 1 M of Na2SO4 solution. Adapted from Saha et al. (2019) with permission from Pergamon. (G) Schematic illustration. (H) SEM images of the fabricated 3D MnO2-carbon nanotube (CNT)–graphene–Ni hybrids. (I) GCD curves of the graphene–Ni, CNT–graphene–Ni, and MnO2–CNT–graphene–Ni hybrids. Adapted from Zhu G. et al. (2014) with permission from RSC Pub.
MnO2/Carbon Nanofiber Composites
CNFs are a type of carbon material that is internally composed of a layer of graphite carbon oriented along the fiber axis. According to different precursors of raw silk, CNFs are mainly divided into three classifications: polyacrylonitrile (PAN)-based carbon fiber, pitch-based carbon fiber, and rayon carbon fiber (Wazir and Kakakhel, 2009). Compared with AC materials, carbon fiber has obvious advantages in performance. The connection of the large, medium, and large number of small holes on the surface is very favorable for the transport of the electrolyte and the adsorption of the charges. Moreover, the excellent heat resistance, low thermal expansion, chemical stability, and good electrical conductivity make them very suitable as electrode materials for supercapacitors (Kim et al., 2019). Generally, CNFs can be prepared by chemical vapor deposition (CVD) and spinning, using carbon ammonia compounds (including methyl, hexyl, ethyl, and carbon monoxide) as carbon precursors. The ammonium bicarbonate molecules are decomposed at high temperatures under the catalysis of metal catalysts such as iron, diamond, and ruthenium; and carbon atoms diffuse into the graphite layer to form a fibrous structure. The spinning method extrudes the precursor polymer into multiple continuous filaments through wet spinning, gel spinning, baking–melting, dry spinning, and electrostatic spinning, among which electrostatic spinning technology is the most widely applied (Zhang et al., 2015).
Owing to the compact structure, low porosity, and small specific surface area (<10 m2 g−1) of CNFs in practical applications (Sun et al., 2018), using pure CNFs as electrode materials leads to poor electrochemical performance. Therefore, they can be used as a substrate-carrying MnO2 pseudocapacitor material to improve the electrochemical activity of the electrode, thereby preparing a high-performance composite electrode. Zhao H. et al. (2016) obtained bubble CNFs by exploding PAN at temperatures of 1,000°C in Figure 5A. The electrode after decoration with MnO2 nanosheets has a capacitance value of 428 F g−1 at 1 A g−1 owing to the easy electron transport path of CNFs (Figures 5B,C). After 1,500 cycles, the specific capacitance of composites still remained 98.8%. Ma et al. (2016) used electrospun lignin-derived high-graphite electrospun CNF (ECNF; ~200 nm in diameter and ~583 m2 g−1 of specific surface area) as the substrate and then decorated with MnO2 nanowhiskers to obtain three kinds of nanocomposites with different weight percentages of MnO2 (Figure 5D). The electrochemical performance of the sample with a mass ratio of ECNF and MnO2 of 1:1 is optimal. The supercapacitor device prepared by using the composite as an electrode material has a specific capacitance of 83.3 F g−1, an energy density of 84.3 Wh kg−1, and a power density of 5.72 kW kg−1 (Figures 5E,F). As shown in Figure 5G, an effective solar technology was reported by Zhao C. et al. (2017). They successfully recovered regenerated carbon fiber (RCF) from carbon fiber-reinforced polymer (CFRP), and then the α-MnO2 nanowires were uniformly grown on the surface of the high temperature-treated RCF. The MnO2/RCF composite (MRCF) prepared by processing CNF at 150°C has an extremely large potential window (1.6 V) and excellent electrochemical performance (specific capacitance is 228.8 F g−1 at 1 A g−1, and high cycle stability is ~91.2% after 3,000 cycles) in Figures 5H,I. The asymmetric supercapacitor assembled with the composite as the positive electrode has an operating potential window of 2.0 V and exhibits a high energy density of 22.9 Wh kg−1. There are relatively few studies on MnO2/CNF, and some of the results are shown in Table 3. It is found that rationally designing the microstructure of MnO2/CNF composites and enhancing the electrochemical utilization of MnO2 can effectively improve EES.
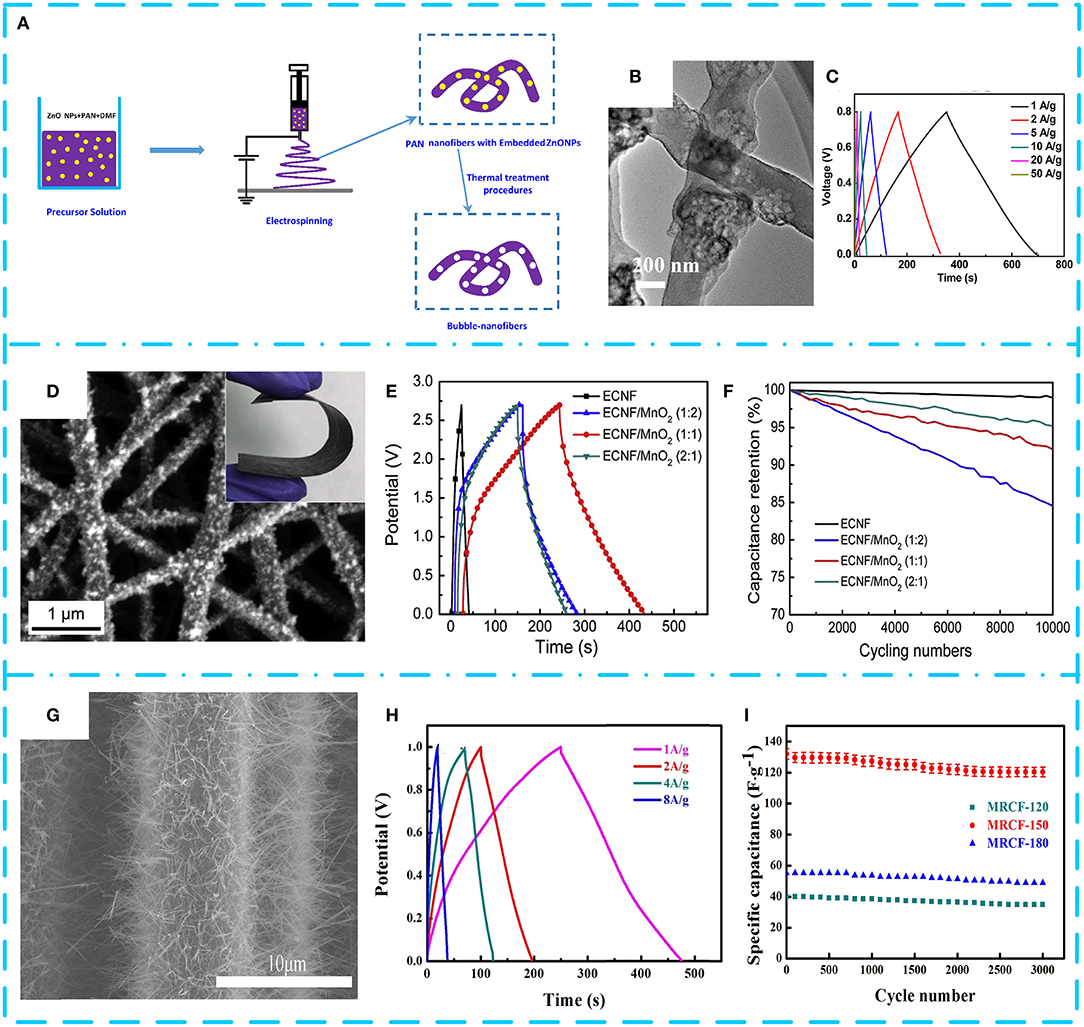
Figure 5. (A) Schematic illustration of bubble carbon nanofibers (B-CNFs). (B) Transmission electron microscopy (TEM) image of B-CNF/MnO2 composites with the B-CNFs calcined at 1,000°C. (C) Galvanostatic charge/discharge curves of B-CNFs/MnO2 at different discharge current densities. Reproduced from Zhao H. et al. (2016) with permission from Pergamon. (D) Scanning electron microscopy (SEM) images of electrospun carbon nanofiber (ECNF)/MnO2 (2:1). (E) Galvanostatic charge/discharge curves acquired from ECNF and three ECNF/MnO2 mats/electrodes at current density of 250 mA g−1. (F) Cycling stability/durability of the ECNF and three ECNF/MnO2 mats/electrodes at high constant current density of 2,000 mA g−1. Reproduced from Ma et al. (2016) with permission from Elsevier S.A. (G) SEM image of regenerated carbon fiber (RCF) prepared at 150°C. (H) Galvanostatic charge/discharge curves at different current densities of MnO2/RCF (MRCF)-150 in 1 M of Na2SO4. (I) Cycle life of the MRCF-120, MRCF-150, and MRCF-180 electrodes at current density of 8 A g−1 in 1 M of Na2SO4 solution. Reproduced from Zhao C. et al. (2017) with permission from Pergamon.
MnO2/Activated Carbon Composites
AC is a carbon material prepared by pyrolysis and activation of carbon-containing raw materials such as wood, coal, and petroleum coke. It has developed pore structure, large specific surface area, and abundant surface chemical groups (Abioye and Ani, 2015). According to the pore size, it can be classified into three types: macropores (≥50 nm), mesopores (2–50 nm), and micropores (≤ 2 nm) (Wei et al., 2016). In the energy storage mechanism, large pores are usually used as ion buffers, mesopores (transition pores) are used for efficient ion diffusion, and micropores are used to store charges. Owing to the rich pore structure of AC, its specific surface area can reach up to 3,000 m2 g−1 (Barbieri et al., 2005), which creates favorable conditions for charge storage. AC possesses characteristics of stable chemical properties, abundant sources, wide operating temperature range, simple preparation process, large specific surface area, and environmental protection (Wang Y. et al., 2019). It has been considered to be the most extensive electric double-layer electrode active material in commercial applications. AC for electrodes of electric double-layer capacitors is usually derived from nature, such as charcoal, husks, and biomass, and can also be obtained by carbonized polymers. Generally, the synthesis process of AC is to carbonize a carbonaceous organic precursor in an inert atmosphere at a high temperature and then activate the carbon materials having a high specific surface area by physical or chemical activation. Physical activation is usually performed by injecting CO2 or water vapor at high temperatures to the system, followed by removing precursor uncarbonized substances and selective oxidation of carbides (López Ch et al., 2015). KOH, ZnCl2, and H3PO4 are usually used as activators for chemical methods with carbonized substances to selectively react to form pores and increase specific surface (Abioye and Ani, 2015). According to the double-layer capacitance theory, the specific capacitance of AC is greatly affected by the specific surface area. The initial research hopes to increase specific capacitance by expanding specific surface area and pore volume of carbon materials. However, it is found that the capacitance of electrode materials is not linearly related to the specific surface area. Even if the specific surface area increases a lot, the increase of specific capacitance is still limited. Moreover, these shortcomings will in turn reduce the energy density and power density of AC electrode.
In order to further obtain high energy density and volume density, typical pseudocapacitive materials such as MnO2 are increasingly anchored on AC to obtain ideal electrode materials. Chen M. D. et al. (2014) selected cotton stalk as carbon precursor and synthesized an amorphous AC material with 1,481 m2 g−1 of specific surface area using H3PO4 as activator by one-step chemical activation. After that, electrolytic MnO2/AC composites with different contents of MnO2 were prepared, as shown in Figure 6A. The galvanostatic charge test confirmed that the composite with 5% MnO2 (labeled as AC4) has optimum specific capacitance (169 F g−1) and excellent cycle life (99.2% after 500 cycles), as shown in Figures 6B,C. With the deepening research, Zhang J. et al. (2019) proposed a novel high-voltage anode electrodeposition technique to deposit graded MnO2 on AC cloth (Figure 6D). Interestingly, it was found that the AC cloth was activated to have a significant improvement in the conductivity and hydrophilicity of the composite electrode. It can be seen from Figure 6E that benefiting from the high conductivity of the closely contacted AC cloth/MnO2 interface, the electrode exhibits a specific capacitance of 400 F g−1 in 1 M of Na2SO4 at 0.5 A g−1 in an asymmetric supercapacitor, and the impressive volumetric energy density reaches 3.82 mWh cm−3. Figure 6F shows the good flexibility and mechanical properties of the composite. In Figures 6G,H, Li H. et al. (2019) combine AC fibers with MnO2 to form electrodes with excellent flexibility and excellent capacitance properties. The area ratio of the composite material is as high as 410 mF cm−2, delivering a high energy density of 36 μWh cm−2 and a high power density of 726 μW cm−2 (Figure 6I). Integrated devices with multiple electrodes can successfully illuminate light-emitting diodes, showing great potential applications. Finally, it is found that the perfect pore structure and proper surface modification that can function as a fast transfer ion and increase the wettability of the electrode surface are beneficial for suitable pore size distribution. Moreover, the presence of the AC can prevent agglomeration of MnO2 and maximize the MnO2 pseudocapacitance. Table 4 summarizes the electrochemical performance of some representative MnO2/AC composites. It shows that MnO2/AC composites, as an environmentally friendly supercapacitor electrode material, have become a preferred choice for researchers.
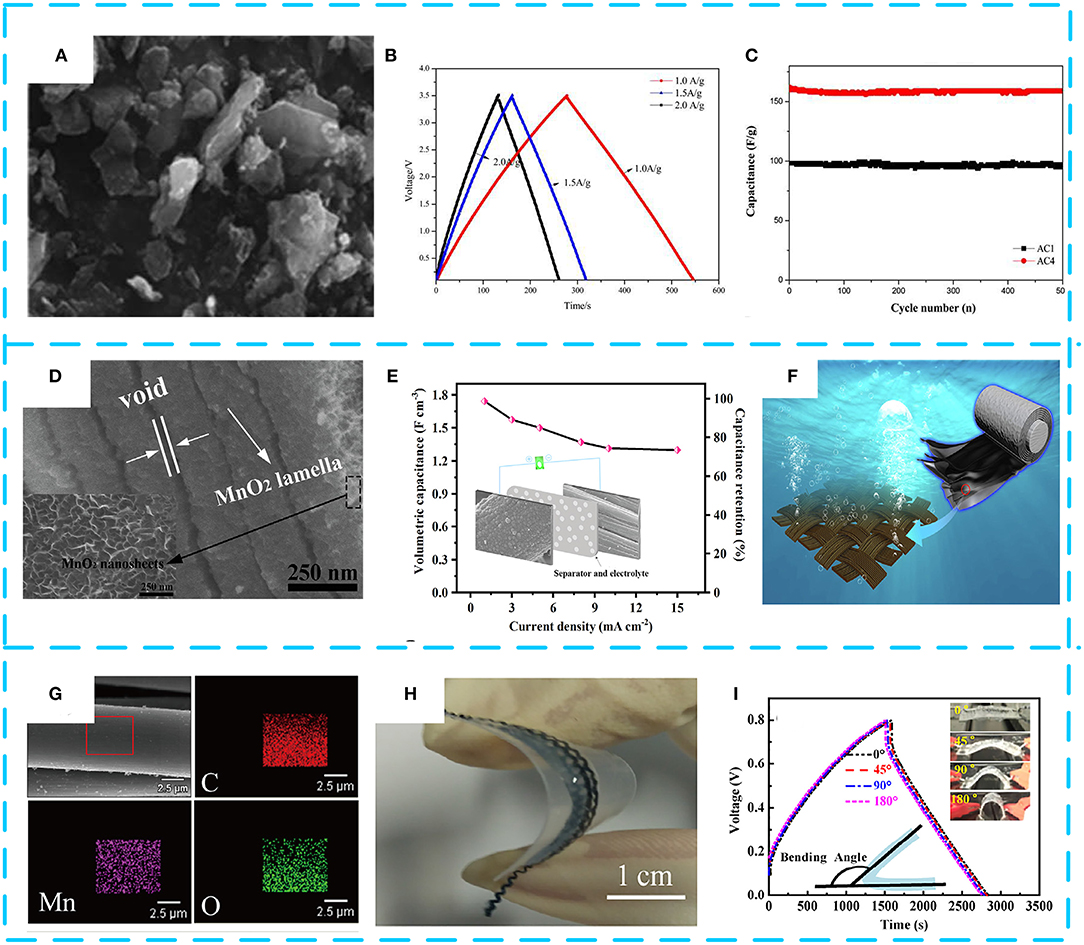
Figure 6. (A) Scanning electron microscopy (SEM) images of AC4. (B) Galvanostatic charge/discharge curves of AC4 at 1.0 A g−1. (C) Charge–discharge cycling stability of AC4 at current density of 2 A g−1. Reproduced from Chen M. D. et al. (2014) with permission from Maney Publishing. (D) Cross-sectional SEM image of MnO2/AC cloth-300. (E) Variations of the capacitance with current densities. (F) Schematic representation of the as-prepared electrode. Reproduced from (Zhang J. et al., 2019) with permission from Elsevier BV. (G) The corresponding energy-dispersive X-ray spectroscopy (EDS) elemental maps of Mn, O, and C. (H) Structure and electrochemical performance. (I) Charge–discharge curves at 0.1 mA cm−2 under different bending states of the MnO2@ACF-6. Reproduced from (Li H. et al., 2019) with permission from Elsevier.
Summary and Outlook
This review summarizes the research achievements in synthesis methods and electrochemical performance of MnO2/carbon composites for supercapacitors in recent years. In this system, MnO2 provides a source of high specific capacitance and high energy density, while carbon materials ensure excellent cycle performance and high power density. Impressively, various functional carbon materials exhibit their own excellent physical and chemical properties in different dimensions, which provides feasibility to rationally optimize the microstructure and maximization of electrochemical properties of MnO2/carbon electrode materials. Among these carbon substrates, one-dimensional carbon materials of CNTs and CNFs can provide high-speed diffusion and shorten ion diffusion paths owing to their high conductivity and good mechanical properties, becoming the preferred choice for flexible supercapacitors. Two-dimensional carbon materials represented by graphene are regarded as ideal conductive substrates for their high specific surface area, ultra-low density, and good electrical conductivity. Other AC materials with typical three-dimensional structure are widely used in preparing energy storage devices with high energy density due to their large number of microporous structures and rich functional groups. In general, carbon nanomaterial with excellent porous structure and high conductivity can maximize the electrochemical performance of MnO2. Orderly and tidy channels (such as mesoporous carbon) can accelerate the transport of electrolyte ions and make it easier to enter MnO2. Meanwhile, enhancing the degree of graphitization of carbon materials (such as CNTs and graphene) is conducive to the improvement of conductivity to ensure rapid transfer of charge. In recent years, numerous efforts have been made to explore high-performance MnO2/carbon composites for supercapacitors, and exciting improvements have been achieved. However, there are still remaining several key drawbacks in those composites, such as the lack of ordered pore channels in the carbon substrate (Wang T. et al., 2015), the violent volume expansion and low electrochemical utilization rate of MnO2 (Lei et al., 2012; Sun et al., 2017), and optimization of the composite structure (Zhang Q. Z. et al., 2018).
Faced with these practical challenges and obstacles, we believe that much more efforts should be focused on developing new-generation MnO2/carbon composites that can better meet the energy storage requirements and standards of advanced supercapacitors in the future. Therefore, we suggest that future research trends may focus on the following aspects:
(1) For MnO2/CNTs or CNFs, a carbon substrate with the optimal pore size distribution and specific surface area should be constructed to increase the specific capacity and power density of the supercapacitor; improve the poor surface wettability of CNTs by surface modification, ultrasound, and other treatments to ensure uniformity of MnO2 deposition; and optimize the design of flexible and foldable CNF substrates that meet different requirements without sacrificing stable power output and long cycle stability.
(2) For MnO2/AC, the low-mass load problem of MnO2 should be improved without sacrificing the power of the composite material to balance the overall performance, effectively exerting the coordination performance of MnO2 and carbon materials.
(3) For MnO2/graphene, MnO2 should be deposited more uniformly on the surface of graphene and should establish an intimate connection by the new composite technology to prevent the damage of composite structure and improve the rate capability and cycle stability.
(4) MnO2 should be combined with economical and green carbon materials to develop light, low-price, and environmentally friendly composite electrode materials.
Author Contributions
All authors listed have made a substantial, direct and intellectual contribution to the work, and approved it for publication.
Funding
This work was supported by research program of the Key Laboratory for Biomedical Effects of Nanomaterials and Nanosafety, Chinese Academy of Sciences (NSKF201908).
Conflict of Interest
The authors declare that the research was conducted in the absence of any commercial or financial relationships that could be construed as a potential conflict of interest.
References
Abioye, A. M., and Ani, F. N. (2015). Recent development in the production of activated carbon electrodes from agricultural waste biomass for supercapacitors: a review. Renew. Sust. Energ. Rev. 52, 1282–1293. doi: 10.1016/j.rser.2015.07.129
Amade, R., Jover, E., Caglar, B., Mutlu, T., and Bertran, E. (2011). Optimization of MnO2/vertically aligned carbon nanotube composite for supercapacitor application. J. Power Sources 196, 5779–5783. doi: 10.1016/j.jpowsour.2011.02.029
Amade, R., Muyshegyan-Avetisyan, A., González, J. M., Pino, A. P., György, E., Pascual, E., et al. (2019). Super-capacitive performance of manganese dioxide/graphene nano-walls electrodes deposited on stainless steel current collectors. Materials 12:483. doi: 10.3390/ma12030483
Barbieri, O., Hahn, M., Herzog, A., and Kötz, R. (2005). Capacitance limits of high surface area activated carbons for double layer capacitors. Carbon 43, 1303–1310. doi: 10.1016/j.carbon.2005.01.001
Bayle, M., Reckinger, N., Huntzinger, J. R., Felten, A., Bakaraki, A., Lan Landois, P., et al. (2015). Dependence of the Raman spectrum characteristics on the number of layers and stacking orientation in few-layer graphene. Phys. Status Solidi 252, 2375–2379. doi: 10.1002/pssb.201552204
Bi, T., Fang, H., Jiang, J., He, X., Zhen, X., Yang, H., et al. (2019). Enhance supercapacitive performance of MnO2/3D carbon nanotubes-graphene as a binder-free electrode. J. Alloys Compd. 30, 759–766. doi: 10.1016/j.jallcom.2019.02.117
Borenstein, A., Hanna, O., Attias, R., Luski, S., Brousse, T., and Aurbach, D. (2017). Carbon-based composite materials for supercapacitor electrodes: a review. J. Mater. Chem. 5, 12653–12672. doi: 10.1039/C7TA00863E
Byl, O., Liu, J., and Yates, J. T. (2005). Etching of carbon nanotubes by ozone-a surface area study. Langmuir 21, 4200–4204. doi: 10.1021/la040132w
Candelaria, S. L., Shao, Y., Zhou, W., Li, X., Xiao, J., Zhang, J. G., et al. (2012). Nanostructured carbon for energy storage and conversion. Nano Energy 1, 195–220. doi: 10.1016/j.nanoen.2011.11.006
Chen, M. D., Wumaie, T., Li, W. L., Song, H. H., and Song, R. R. (2014). Electrochemical performance of cotton stalk based activated carbon electrodes modified by MnO2 for supercapacitor. Mater. Technol. 30, A2–A7. doi: 10.1179/1753555714Y.0000000241
Chen, P., Yang, C., He, Z., and Guo, K. (2018). One-pot facile route to fabricate the precursor of sulfonated graphene/N-doped mesoporous carbons composites for supercapacitors. J. Mater. Sci. 54, 4180–4191. doi: 10.1007/s10853-018-3122-6
Chen, Y., Zhang, J., Li, M., Yang, C., Zhang, L., Wang, C., et al. (2018). Strong interface coupling and few-crystalline MnO2/Reduced graphene oxide composites for supercapacitors with high cycle stability. Electrochim. Acta 292, 115–124. doi: 10.1016/j.electacta.2018.09.131
Cheng, Y., Lu, S., Zhang, H., Varanasi, C. V., and Liu, J. (2012). Synergistic effects from graphene and carbon nanotubes enable flexible and robust electrodes for high-performance supercapacitors. Nano Lett. 12, 4206–4211. doi: 10.1021/nl301804c
Conway, B. E. (1999). Electrochemical Supercapacitors: Scientific Fundamentals and Technological Applications. NewYork, NY: Springer. doi: 10.1007/978-1-4757-3058-6
Dai, K., Lu, L., Liang, C., Dai, J., Liu, Q., Zhang, Y., et al. (2014). In situ assembly of MnO2 nanowires/graphene oxide nanosheets composite with high specific capacitance. Electrochim. Acta 116, 111–117. doi: 10.1016/j.electacta.2013.11.036
Díaz-Delgado, R., and Doherty, A. P. (2016). Carbons, ionic liquids, and quinones for electrochemical capacitors. Front. Mater. 3:18. doi: 10.3389/fmats.2016.00018
Dubey, R., and Guruviah, V. (2019). Review of carbon-based electrode materials for supercapacitor energy storage. Ionics 25, 1419–1445. doi: 10.1007/s11581-019-02874-0
Fan, Z., Chen, J., Zhang, B., Liu, B., Zhong, X., and Kuang, Y. (2008a). High dispersion of γ-MnO2 on well-aligned carbon nanotube arrays and its application in supercapacitors. Diamond Relat. Mater. 17, 1943–1948. doi: 10.1016/j.diamond.2008.04.015
Fan, Z., Chen, J., Zhang, B., Sun, F., Liu, B., and Kuang, Y. (2008b). Electrochemically induced deposition method to prepare γ-MnO2/multi-walled carbon nanotube composites as electrode material in supercapacitors. Mater. Res. Bull. 43, 2085–2091. doi: 10.1016/j.materresbull.2007.09.015
Faraji, S., and Ani, F. N. (2015). The development supercapacitor from activated carbon by electroless plating-A review. Renew. Sust. Energ. Rev. 42, 823–834. doi: 10.1016/j.rser.2014.10.068
Fujiwara, A., Ishii, K., Suematsu, H., Kataura, H., Maniwa, Y., Suzuki, S., et al. (2001). Gas adsorption in the inside and outside of single-walled carbon nanotubes. Chem. Phys. Lett. 336, 205–211. doi: 10.1016/S0009-2614(01)00111-7
Gao, F., Tang, X., Yi, H., Chao, C., Na, L., Li, J., et al. (2017). In-situ DRIFTS for the mechanistic studies of NO oxidation over α-MnO2, β-MnO2 and γ-MnO2 catalysts. Chem. Eng. J. 322, 525–537. doi: 10.1016/j.cej.2017.04.006
Ge, J., Yao, H. B., Hu, W., Yu, X. F., Yan, Y. X., Mao, L. B., et al. (2013). Facile dip coating processed graphene/MnO2 nanostructured sponges as high performance supercapacitor electrodes. Nano Energy 2, 505–513. doi: 10.1016/j.nanoen.2012.12.002
Geim, A. K., and Novoselov, K. S. (2007). The rise of graphene. Nat. Mater. 6, 183–191. doi: 10.1038/nmat1849
Hao, P., Zhao, Z., Tian, J., Li, H., Sang, Y., Yu, G., et al. (2014). Hierarchical porous carbon aerogel derived from bagasse for high performance supercapacitor electrode. Nanoscale 6, 12120–12129. doi: 10.1039/C4NR03574G
He, Y., Chen, W., Gao, C., Zhou, J., Li, X., and Xie, E. (2013). An overview of carbon materials for flexible electrochemical capacitors. Nanoscale 5, 8799–8820. doi: 10.1039/c3nr02157b
Hu, Y., Wu, Y., and Wang, J. (2018). Manganese-oxide-based electrode materials for energy storage applications: how close are we to the theoretical capacitance? Adv. Mater. 30:e1802569. doi: 10.1002/adma.201802569
Huang, J., Dai, Y., Singewald, K., Liu, C. C., Saxena, S., and Zhang, H. (2019). Effects of MnO2 of different structures on activation of peroxymonosulfate for bisphenol A degradation under acidic conditions. Chem. Eng. J. 370, 906–915. doi: 10.1016/j.cej.2019.03.238
Huang, X., Yin, Z., Wu, S., Qi, X., He, Q., Zhang, Q., et al. (2011). Graphene-based materials: synthesis, characterization, properties, and applications. Small 7, 1876–1902. doi: 10.1002/smll.201002009
Jadhav, S., Kalubarme, R. S., Terashima, C., Kale, B. B., Godbole, V., Fujishima, A., et al. (2019). Manganese dioxide/reduced graphene oxide composite an electrode material for high-performance solid state supercapacitor. Electrochim. Acta 299, 34–44. doi: 10.1016/j.electacta.2018.12.182
Jeong, J. H., Park, J. W., Lee, D. W., Baughman, R. H., and Kim, S. J. (2019). Electrodeposition of α-MnO2/γ-MnO2 on carbon nanotube for yarn supercapacitor. Sci. Rep. 9:11271. doi: 10.1038/s41598-019-47744-x
Kannappan, S., Hao, Y., Kaliyappan, K., Manian, R. K., Pandian, A. S., Lee, Y. S., et al. (2018). Thiolated-graphene-based supercapacitors with high energy density and stable cycling performance. Carbon 134, 326–333. doi: 10.1016/j.carbon.2018.02.036
Kaushal, I., Sharma, A. K., Saharan, P., Sadasivuni, K. K., and Duhan, S. (2019). Superior architecture and electrochemical performance of MnO2 doped PANI/CNT graphene fastened composite. J. Porous Mat. 26, 1287–1296. doi: 10.1007/s10934-019-00728-8
Kim, C. H., Yang, C. M., Kim, Y. A., and Yang, K. S. (2019). Pore engineering of nanoporous carbon nanofibers toward enhanced supercapacitor performance. Appl. Surf. Sci. 497:143693. doi: 10.1016/j.apsusc.2019.143693
Kim, M., Hwang, Y., Min, K., and Kim, J. (2013). Introduction of MnO2 nanoneedles to activated carbon to fabricate high-performance electrodes as electrochemical supercapacitors. Electrochim. Acta 113, 322–331. doi: 10.1016/j.electacta.2013.09.058
Kim, S., Lee, J., Kang, J. S., Jo, K., Kim, S., Sung, Y. E., et al. (2015). Lithium recovery from brine using a k-MnO2/activated carbon hybrid supercapacitor system. Chemosphere 125, 50–56. doi: 10.1016/j.chemosphere.2015.01.024
Kong, S., Jin, B., Quan, X., Zhang, G., Guo, X., Zhu, Q., et al. (2019). MnO2 nanosheets decorated porous active carbon derived from wheat bran for high-performance asymmetric supercapacitor. J. Electroanal. Chem. 850:113412. doi: 10.1016/j.jelechem.2019.113412
Le, Q., Huang, M., Wang, T., Liu, X., Sun, L., Guo, X., et al. (2019). Biotemplate derived three dimensional nitrogen doped graphene@MnO2 as bifunctional material for supercapacitor and oxygen reduction reaction catalyst. J. Colloid Interface Sci. 544, 155–163. doi: 10.1016/j.jcis.2019.02.089
Lee, H., and Goodenough, J. B. (1999). Supercapacitor behavior with KCl electrolyte. J. Solid State Chem. 144, 220–223. doi: 10.1006/jssc.1998.8128
Lei, Z., Shi, F., and Lu, L. (2012). Incorporation of MnO2-coated carbon nanotubes between graphene sheets as supercapacitor electrode. ACS Appl. Mater. Interfaces 4, 1058–1064. doi: 10.1021/am2016848
Li, F., Wang, J., Liu, L., Qu, J., Li, Y., Bandari, V. K., et al. (2019). Self-assembled flexible and integratable 3D microtubular asymmetric supercapacitors. Adv. Sci. 6:1901051. doi: 10.1002/advs.201901051
Li, H., Liang, J., Li, H., Zheng, X., Tao, Y., Huang, Z. H., et al. (2019). Activated carbon fibers with manganese dioxide coating for flexible fiber supercapacitors with high capacitive performance. J. Energy Chem. 31, 95–100. doi: 10.1016/j.jechem.2018.05.008
Li, L., Chen, L., Qian, W., Xie, F., and Dong, C. (2019). Directly grown multiwall carbon nanotube and hydrothermal MnO2 composite for high-performance supercapacitor electrodes. Nanomaterials 9:703. doi: 10.3390/nano9050703
Li, M., Chen, Q., and Zhan, H. (2017). Ultrathin manganese dioxide nanosheets grown on partially unzipped nitrogen-doped carbon nanotubes for high-performance asymmetric supercapacitors. J. Alloys Compd. 702, 236–243. doi: 10.1016/j.jallcom.2017.01.244
Li, Q., Lu, X. F., Xu, H., Tong, Y. X., and Li, G. R. (2014). Carbon/MnO2 double-walled nanotube arrays with fast ion and electron transmission for high-performance supercapacitors. ACS Appl. Mater. Interfaces 6, 2726–2733. doi: 10.1021/am405271q
Li, W. S., Chang, M. L., Chuang, K. C., Li, Y. S., Luo, J. D., and Cheng, H. C. (2019). Electrochemical properties of CNT/MnO2 hybrid nanostructure with low-temperature hydrothermal synthesis as high-performance supercapacitor. J. Electrochem. Soc. 166, A2194–A2198. doi: 10.1149/2.1551910jes
Li, Z., Liu, Z., Li, D., Li, B., Li, Q., Huang, Y., et al. (2014). Facile synthesis of α-MnO2 nanowires/spherical activated carbon composite for supercapacitor application in aqueous neutral electrolyte. J. Mater. Sci.-Mater. Electron. 26, 353–359. doi: 10.1007/s10854-014-2407-z
Liang, M., and Xin, H. (2015). Microwave to terahertz: characterization of carbon-based nanomaterials. IEEE Microw. Mag. 15, 40–51. doi: 10.1109/MMM.2013.2288708
Liu, R., Liu, E., Ding, R., Liu, K., Teng, Y., Luo, Z., et al. (2015). Facile in-situ redox synthesis of hierarchical porous activated carbon@MnO2 core/shell nanocomposite for supercapacitors. Ceram. Int. 41, 12734–12741. doi: 10.1016/j.ceramint.2015.06.106
Liu, Y., Zhou, X., Liu, R., Li, X., Bai, Y., and Yuan, G. (2017). Preparation of three-dimensional compressible MnO2@carbon nanotube sponges with enhanced supercapacitor performance. N. J. Chem. 41, 14906–14913. doi: 10.1039/C7NJ03323K
López Ch, L. T., Chejne, F., and Bhatia, S. K. (2015). Effect of activating agents: flue gas and CO2 on the preparation of activated carbon for methane storage. Energy Fuels 29, 6296–6305. doi: 10.1021/acs.energyfuels.5b01438
Lu, S., Guo, K. K., Xie, Y., and Ning, J. (2018). Ordered mesoporous carbons loading on sulfonated graphene by multi-components Co-assembly for supercapacitor applications. Energy Technol. 6, 1975–1985. doi: 10.1002/ente.201800116
Lu, Z., Raad, R., Safaei, F., Xi, J., Liu, Z., and Foroughi, J. (2019). Carbon nanotube based fiber supercapacitor as wearable energy storage. Front. Mater. 6:138. doi: 10.3389/fmats.2019.00138
Luo, S., Xie, L., Han, F., Wei, W., Huang, Y., Zhang, H., et al. (2019). Nanoscale parallel circuitry based on interpenetrating conductive sssembly for flexible and high-power zinc ion battery. Adv. Funct. Mater. 29:1901336. doi: 10.1002/adfm.201901336
Lv, P., Zhang, P., Feng, Y., Li, Y., and Feng, W. (2012). High-performance electrochemical capacitors using electrodeposited MnO2 on carbon nanotube array grown on carbon fabric. Electrochim. Acta 78, 515–523. doi: 10.1016/j.electacta.2012.06.085
Ma, X., Kolla, P., Zhao, Y., Smirnova, A. L., and Fong, H. (2016). Electrospun lignin-derived carbon nanofiber mats surface-decorated with MnO2 nanowhiskers as binder-free supercapacitor electrodes with high performance. J. Power Sources 325, 541–548. doi: 10.1016/j.jpowsour.2016.06.073
Malak-Polaczyk, A., Matei-Ghimbeu, C., Vix-Guterl, C., and Frackowiak, E. (2010). Carbon/λ-MnO2 composites for supercapacitor electrodes. J. Solid State Chem. 183, 969–974. doi: 10.1016/j.jssc.2010.02.015
Nataraj, S. K., Song, Q., Al-Muhtaseb, S. A., Dutton, S. E., Zhang, Q., and Sivaniah, E. (2013). Thin, flexible supercapacitors made from carbon nanofiber electrodes decorated at room temperature with manganese oxide nanosheets. J. Nanomater. 2013, 1–6. doi: 10.1155/2013/272093
Novoselov, K. S., Geim, A. K., Morozov, S. V., Jiang, D., Zhang, Y., Dubonos, S. V., et al. (2004). Electric field effect in atomically thin carbon films. Science 306, 666–669. doi: 10.1126/science.1102896
Palchoudhury, S., Ramasamy, K., Gupta, R. K., and Gupta, A. (2019). Flexible supercapacitors: a materials perspective. Front. Mater. 5:83. doi: 10.3389/fmats.2018.00083
Peng, S., Li, L., Yoong, J. L. K., Tian, L., Srinivasan, M., Adams, S., et al. (2016). Electrospun carbon nanofibers and their hybrid composites as advanced materials for energy conversion and storage. Nano Energy 22, 361–395. doi: 10.1016/j.nanoen.2016.02.001
Prélot, B., Poinsignon, C., Thomas, F., Schouller, E., and Villiéras, F. (2003). Structural-chemical disorder of manganese dioxides: 1. Influence on surface properties at the solid-electrolyte interface. J. Colloid Interface Sci. 257, 77–84. doi: 10.1016/S0021-9797(02)00013-9
Qian, W., Xu, G., Fei, W., Chao, Z., Cui, C., Zhao, M., et al. (2012). Carbon nanotubes for supercapacitors:consideration of cost and chemical vapor deposition techniques. J. Energy Chem. 21, 233–240. doi: 10.1016/S1003-9953(11)60358-7
Ramesh, S., Kim, H. S., Haldorai, Y., Han, Y. K., and Kim, J. H. (2017). Fabrication of nanostructured MnO2/carbon nanotube composite from 3D precursor complex for high-performance supercapacitor. Mater. Lett. 196, 132–136. doi: 10.1016/j.matlet.2017.03.044
Ren, S., Rong, P., and Yu, Q. (2018). Preparations, properties and applications of graphene in functional devices: a concise review. Ceram. Int. 44, 11940–11955. doi: 10.1016/j.ceramint.2018.04.089
Saha, S., Maji, P., Pethsangave, D. A., Roy, A., Ray, A., Some, S., et al. (2019). Effect of morphological ordering on the electrochemical performance of MnO2-graphene oxide composite. Electrochim. Acta 317, 199–210. doi: 10.1016/j.electacta.2019.05.148
Salinas-Torres, D., Ruiz-Rosas, R., Morallón, E., and Cazorla-Amorós, D. (2019). Strategies to enhance the performance of electrochemical capacitors based on carbon materials. Front. Mater. 6:115. doi: 10.3389/fmats.2019.00115
Seung Woo, L., Junhyung, K., Shuo, C., Hammond, P. T., and Yang, S. H. (2010). Carbon nanotube/manganese oxide ultrathin film electrodes for electrochemical capacitors. ACS Nano 4, 3889–3896. doi: 10.1021/nn100681d
Shen, H., Zhang, Y., Song, X., Liu, Y., Wang, H., Duan, H., et al. (2019). Facile hydrothermal synthesis of actiniaria-shaped α-MnO2/activated carbon and its electrochemical performances of supercapacitor. J. Alloys Compd. 770, 926–933. doi: 10.1016/j.jallcom.2018.08.228
Sheng, L., Jiang, L., Wei, T., and Fan, Z. (2016). High volumetric energy density asymmetric supercapacitors based on well-balanced graphene and graphene-MnO2 electrodes with densely stacked architectures. Small 12, 5217–5227. doi: 10.1002/smll.201601722
Shi, X., Zheng, S., Wu, Z. S., and Bao, X. (2018). Recent advances of graphene-based materials for high-performance and new-concept supercapacitors. J. Energy Chem. 27, 25–42. doi: 10.1016/j.jechem.2017.09.034
Snook, G. A., Kao, P., and Best, A. S. (2011). Conducting-polymer-based supercapacitor devices and electrodes. J. Power Sources 196, 1–12. doi: 10.1016/j.jpowsour.2010.06.084
Subagio, A., Hakim, Y. A., Ristiawan1, M. W., Kholil1, M. A., and Priyono (2019). Structural and morphological properties of MnO2/MWCNT composite grown using the hydrothermal method for supercapacitor application. J. Inorg. Organomet. Polym. Mater. 14, 9936–9947. doi: 10.20964/2019.10.52
Subramanian, V., Zhu, H., and Wei, B. (2006). Synthesis and electrochemical characterizations of amorphous manganese oxide and single walled carbon nanotube composites as supercapacitor electrode materials. Electrochem. Commun. 8, 827–832. doi: 10.1016/j.elecom.2006.02.027
Sun, J., Cui, B., Chu, F., Yun, C., He, M., Li, L., et al. (2018). Printable Nanomaterials for the fabrication of high-performance supercapacitors. Nanomaterials 8:528. doi: 10.3390/nano8070528
Sun, P., Yi, H., Peng, T., Jing, Y., Wang, R., Wang, H., et al. (2017). Ultrathin MnO2 nanoflakes deposited on carbon nanotube networks for symmetrical supercapacitors with enhanced performance. J. Power Sources 341, 27–35. doi: 10.1016/j.jpowsour.2016.11.112
Tajik, S., Dubal, D. P., Gomez-Romero, P., Yadegari, A., Rashidi, A., Nasernejad, B., et al. (2017). Nanostructured mixed transition metal oxides for high performance asymmetric supercapacitors: facile synthetic strategy. Int. J. Hydrog. Energy 42, 12384–12395. doi: 10.1016/j.ijhydene.2017.03.117
Trudeau, M. L. (2013). Advanced materials for energy storage. MRS Bull. 24, 23–26. doi: 10.1557/S0883769400053410
Wang, G. P., Zhang, L., and Zhang, J. J. (2012). A review of electrode materials for electrochemical supercapacitors. Chem. Soc. Rev. 41, 797–828. doi: 10.1039/C1CS15060J
Wang, H., Fu, Q., and Pan, C. (2019). Green mass synthesis of graphene oxide and its MnO2 composite for high performance supercapacitor. Electrochim. Acta 312, 11–21. doi: 10.1016/j.electacta.2019.04.178
Wang, J. G., Kang, F., and Wei, B. (2015). Engineering of MnO2-based nanocomposites for high-performance supercapacitors. Prog. Mater. Sci. 74, 51–124. doi: 10.1016/j.pmatsci.2015.04.003
Wang, J. G., Yang, Y., Huang, Z. H., and Kang, F. (2011). Coaxial carbon nanofibers/MnO2 nanocomposites as freestanding electrodes for high-performance electrochemical capacitors. Electrochim. Acta 56, 9240–9247. doi: 10.1016/j.electacta.2011.07.140
Wang, J. G., Yang, Y., Huang, Z. H., and Kang, F. (2012). Synthesis and electrochemical performance of MnO2/CNTs–embedded carbon nanofibers nanocomposites for supercapacitors. Electrochim. Acta 75, 213–219. doi: 10.1016/j.electacta.2012.04.088
Wang, J. G., Yang, Y., Huang, Z. H., and Kang, F. (2013). Effect of temperature on the pseudo-capacitive behavior of freestanding MnO2@carbon nanofibers composites electrodes in mild electrolyte. J. Power Sources 224, 86–92. doi: 10.1016/j.jpowsour.2012.09.075
Wang, L., Huang, M., Chen, S., Kang, L., He, X., Lei, Z., et al. (2017). δ-MnO2 nanofiber/single-walled carbon nanotube hybrid film for all-solid-state flexible supercapacitors with high performance. J. Mater. Chem. A 5, 19107–19115. doi: 10.1039/C7TA04712F
Wang, N., Wu, C., Li, J., Dong, G., and Guan, L. (2011). Binder-free manganese oxide/carbon nanomaterials thin film electrode for supercapacitors. ACS Appl. Mater. Interfaces 3, 4185–4189. doi: 10.1021/am201145k
Wang, T., Song, D., Zhao, H., Chen, J., Zhao, C., Chen, L., et al. (2015). Facilitated transport channels in carbon nanotube/carbon nanofiber hierarchical composites decorated with manganese dioxide for flexible supercapacitors. J. Power Sources 274, 709–717. doi: 10.1016/j.jpowsour.2014.10.102
Wang, X., Chen, L., Zhang, S., Chen, X., Li, Y., Liu, J., et al. (2019a). Compounding δ-MnO2 with modified graphene nanosheets for highly stable asymmetric supercapacitors. Colloid Surf. 573, 57–66. doi: 10.1016/j.colsurfa.2019.04.040
Wang, X., Wu, D., Song, X., Du, W., Zhao, X., and Zhang, D. (2019b). Review on carbon/polyaniline hybrids: design and synthesis for supercapacitor. Molecules 24:2263. doi: 10.3390/molecules24122263
Wang, Y., and Igor, Z. (2009). Electrophoretic deposition of manganese dioxide-multiwalled carbon nanotube composites for electrochemical supercapacitors. Langmuir 25, 9684–9689. doi: 10.1021/la900937e
Wang, Y., Qu, Q., Gao, S., Tang, G., Liu, K., He, S., et al. (2019). Biomass derived carbon as binder-free electrode materials for supercapacitors. Carbon 155, 706–726. doi: 10.1016/j.carbon.2019.09.018
Wazir, A. H., and Kakakhel, L. (2009). Preparation and characterization of pitch-based carbon fibers. N. Carbon Mater. 24, 83–88. doi: 10.1016/S1872-5805(08)60039-6
Wei, H., Wang, X., Zhang, Y., Du, W., Sun, X., Jiang, F., et al. (2019). Facile synthesis of lotus seedpod-based 3D hollow porous activated carbon/manganese dioxide composite for supercapacitor electrode. J. Electroanal. Chem. 853:113561. doi: 10.1016/j.jelechem.2019.113561
Wei, L., Liu, J., and Zhao, D. (2016). Mesoporous materials for energy conversion and storage devices. Nat. Rev. Mater. 1:16023. doi: 10.1038/natrevmats.2016.23
Wei, W., Cui, X., Chen, W., and Ivey, D. G. (2011). Manganese oxide-based materials as electrochemical supercapacitor electrodes. Chem. Soc. Rev. 40, 1697–1721. doi: 10.1039/C0CS00127A
Wu, Z. S., Ren, W., Wang, D. W., Li, F., Liu, B., and Chen, H. M. (2010). High-energy MnO2 nanowire/graphene and graphene asymmetric electrochemical capacitors. ACS Nano 4, 5835–5842. doi: 10.1021/nn101754k
Xiao, W., Xia, H., Fuh, J. Y. H., and Lu, L. (2010). Electrophoretic-deposited CNT/MnO2 composites for high-power electrochemical energy storage/conversion applications. Phys. Scr. 139:014008. doi: 10.1088/0031-8949/2010/T139/014008
Xie, S., Si, L., Cheng, F., and Lu, X. (2017). Recent advances for achieving high-performance carbon fiber materials for supercapacitors. ChemElectroChem 5, 571–582. doi: 10.1002/celc.201701020
Xie, X., Ni, C., Wang, B., Zhang, Y., Zhao, X., Liu, L., et al. (2019). Recent advances in hydrogen generation process via hydrolysis of Mg-based materials: a short review. J. Alloys Compd. 816:152634. doi: 10.1016/j.jallcom.2019.152634
Xie, Y., Yang, C., Chen, P., Yuan, D., and Guo, K. (2019). MnO2-decorated hierarchical porous carbon composites for high-performance asymmetric supercapacitors. J. Power Sources 425, 1–9. doi: 10.1016/j.jpowsour.2019.03.122
Xin, L., and Wei, B. (2012). Facile synthesis and super capacitive behavior of SWNT/MnO2 hybrid films. Nano Energy 1, 479–487. doi: 10.1016/j.nanoen.2012.02.011
Yan, J., Fan, Z., Wei, T., Cheng, J., Shao, B., Wang, K., et al. (2009). Carbon nanotube/MnO2 composites synthesized by microwave-assisted method for supercapacitors with high power and energy densities. J. Power Sources 194, 1202–1207. doi: 10.1016/j.jpowsour.2009.06.006
Yan, J., Fan, Z., Wei, T., Qian, W., Zhang, M., and Wei, F. (2010). Fast and reversible surface redox reaction of graphene-MnO2 composites as supercapacitor electrodes. Carbon 48, 3825–3833. doi: 10.1016/j.carbon.2010.06.047
Yang, Z., Zhang, J., Kintner-Meyer, M. C., Lu, X., Choi, D., Lemmon, J. P., et al. (2011). Electrochemical energy storage for green grid. Chem. Rev. 111, 3577–3613. doi: 10.1021/cr100290v
Yu, Z., Shang, J., Fu, W., Li, Z., and Cai, Y. (2018). A sp2 + sp3 hybridized carbon allotrope transformed from AB stacking graphyne and THD-graphene. AIP Adv. 8:015028. doi: 10.1063/1.5016387
Zhang, B., Kang, F., Tarascon, J. M., and Kim, J. K. (2015). Recent advances in electrospun carbon nanofibers and their application in electrochemical energy storage. Prog. Mater. Sci. 76, 319–380. doi: 10.1016/j.pmatsci.2015.08.002
Zhang, J., Sun, J., Shifa, T. A., Wang, D., Wu, X., and Cui, Y. (2019). Hierarchical MnO2/activated carbon cloth electrode prepared by synchronized electrochemical activation and oxidation for flexible asymmetric supercapacitors. Chem. Eng. J. 372, 1047–1055. doi: 10.1016/j.cej.2019.04.202
Zhang, J., Wang, Y., Zang, J., Xin, G., Yuan, Y., and Qu, X. (2012). Electrophoretic deposition of MnO2-coated carbon nanotubes on a graphite sheet as a flexible electrode for supercapacitors. Carbon 50, 5196–5202. doi: 10.1016/j.carbon.2012.07.002
Zhang, L. L., and Zhao, X. S. (2009). Carbon-based materials as supercapacitor electrodes. Chem. Soc. Rev. 38, 2520–2531. doi: 10.1039/b813846j
Zhang, Q., Wu, X., Zhang, Q., Yang, F., Dong, H., Sui, J., et al. (2019). One-step hydrothermal synthesis of MnO2/graphene composite for electrochemical energy storage. J. Electroanal. Chem. 837, 108–115. doi: 10.1016/j.jelechem.2019.02.031
Zhang, Q. Z., Zhang, D., Miao, Z. C., Zhang, X. L., and Chou, S. L. (2018). Research progress in MnO2-carbon based supercapacitor electrode materials. Small 14:1702883. doi: 10.1002/smll.201702883
Zhao, C., Ge, Z., Zhou, Y., Huang, Y., Wang, G., and Qian, X. (2017). Solar-assisting pyrolytically reclaimed carbon fiber and their hybrids of MnO2/RCF for supercapacitor electrodes. Carbon 114, 230–241. doi: 10.1016/j.carbon.2016.12.025
Zhao, H., Han, W., Lan, W., Zhou, J., Zhang, Z., Fu, W., et al. (2016). Bubble Carbon-nanofibers decorated with MnO2 nanosheets as high-performance supercapacitor electrode. Electrochim. Acta 222, 1931–1939. doi: 10.1016/j.electacta.2016.12.007
Zhi, M., Manivannan, A., Meng, F., and Wu, N. (2012). Highly conductive electrospun carbon nanofiber/MnO2 coaxial nano-cables for high energy and power density supercapacitors. J. Power Sources 208, 345–353. doi: 10.1016/j.jpowsour.2012.02.048
Zhou, M., Gomez, J., Li, B., Jiang, Y. B., and Deng, S. (2017). Oil tea shell derived porous carbon with an extremely large specific surface area and modification with MnO2 for high-performance supercapacitor electrodes. Appl. Mater. Today 7, 47–54. doi: 10.1016/j.apmt.2017.01.008
Zhu, G., He, Z., Chen, J., Zhao, J., Feng, X., Ma, Y., et al. (2014). Highly conductive three-dimensional MnO2-carbon nanotube-graphene-Ni hybrid foam as a binder-free supercapacitor electrode. Nanoscale 6, 1079–1085. doi: 10.1039/C3NR04495E
Zhu, M., Wang, Z., Li, H., Yuan, X., and Zhi, C. (2018). Light-permeable, photoluminescent microbatteries embedded in the color filter of a screen. Energy Environ. Sci. 11, 2414–2422. doi: 10.1039/C8EE00590G
Nomenclature
Keywords: MnO2, carbon materials, composites, electrode material, supercapacitor
Citation: Wu D, Xie X, Zhang Y, Zhang D, Du W, Zhang X and Wang B (2020) MnO2/Carbon Composites for Supercapacitor: Synthesis and Electrochemical Performance. Front. Mater. 7:2. doi: 10.3389/fmats.2020.00002
Received: 28 September 2019; Accepted: 06 January 2020;
Published: 11 February 2020.
Edited by:
M. Jasim Uddin, University of Texas Rio Grande Valley Edinburg, United StatesReviewed by:
Minshen Zhu, Leibniz-Institut für Festkörper- und Werkstoffforschung (IFW Dresden), GermanyAminur Rashid Chowdhury, University of Texas at Austin, United States
Copyright © 2020 Wu, Xie, Zhang, Zhang, Du, Zhang and Wang. This is an open-access article distributed under the terms of the Creative Commons Attribution License (CC BY). The use, distribution or reproduction in other forums is permitted, provided the original author(s) and the copyright owner(s) are credited and that the original publication in this journal is cited, in accordance with accepted academic practice. No use, distribution or reproduction is permitted which does not comply with these terms.
*Correspondence: Wei Du, ZHV3ZWlAeXR1LmVkdS5jbg==; Xiaoyu Zhang, emhhbmd4aWFveXVAeXR1LmVkdS5jbg==