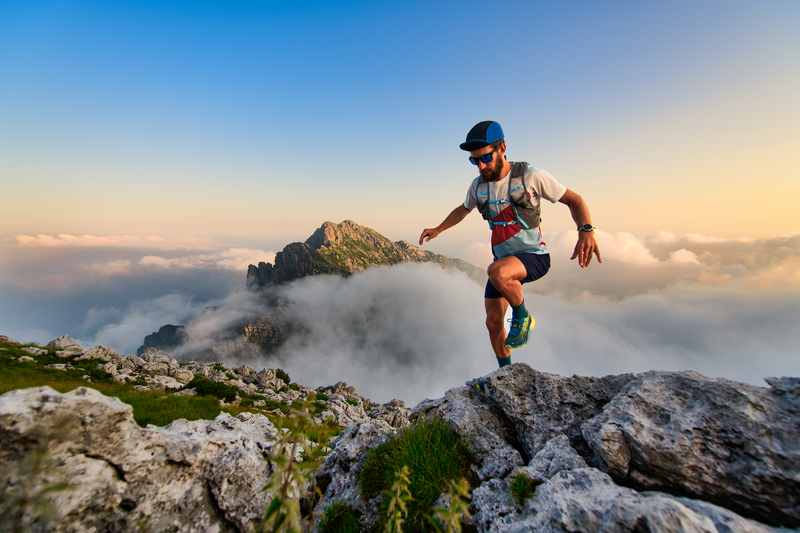
95% of researchers rate our articles as excellent or good
Learn more about the work of our research integrity team to safeguard the quality of each article we publish.
Find out more
REVIEW article
Front. Mater. , 01 November 2019
Sec. Biomaterials
Volume 6 - 2019 | https://doi.org/10.3389/fmats.2019.00268
Strategies to promote bone regeneration have been always the focus of investigations since the skeleton plays important roles like mechanical support, organ protection, and mineral homeostasis maintenance in the normal physiological activities of the human body. Magnetic fields have shown to be highly influential in the regeneration process, arousing tremendous interest in utilizing magnetic materials to enhance osteogenesis. In this work, we attempt a more comprehensive and detailed review of magnetic materials in promoting bone regeneration by including not only the mechanisms of bone regeneration, the history and basic concepts of magnetism, but also the types of magnetic materials as well as their influence parameters, designs and fabrication techniques with a focus on their usage in the field of bone regeneration like 3D printed scaffolds and implants. In addition, we provide some possible ideas on the synergistic action between magnetic and other materials on bone tissue. Finally, we propose the development trend of magnetic materials in the field of bone regeneration in the future. There is a huge demand for a more effective and less traumatic way to accelerate bone regeneration of the patients with diseases like fractures, tumors and osteoporosis that cause severe pains, bone loss, limb deformations, and restrictions of mobility. Magnetic materials have substantial potential to be manufactured into novel clinical applications with the ability to increase the bone regeneration efficiency.
As a highly mineralized tissue, bone not only provides mechanical protection for connective tissue and soft tissue, it also actively participates in the regulation of pH and calcium levels in blood and the formation of blood cells (Porter et al., 2009). Before we explore magnetic materials, the process of bone regeneration must be understood.
“Osteogenesis” is a procedure where bone-forming cells [mainly multipotent mesenchymal stem cells (MSCs)] mature and differentiate into osteoblasts. Osteogenesis is accompanied by mineralization of the extracellular matrix by deposition of calcium hydroxyapatite (Caetano-Lopes et al., 2007). Both of the signaling molecules (e.g., cytokines, growth factors, sex hormones) and interactions with osteoclasts (cells derived from macrophages which have the opposite role to osteoblasts) (Huang et al., 2014), have an influence on proliferation, differentiation, and function of osteoblasts. The relationship between osteoblasts and osteoclasts is related mainly to the receptor activator of nuclear factor-kappa B ligand (RANKL), usually on the surface of developing osteoblastic cells, which can bind with its receptor RANK, resulting in the activation, proliferation and differentiation of pre-osteoclast cells (Khosla, 2001; Atkins et al., 2003). Osteoprotegerin (OPG) is an osteoblast-derived factor, which can bind with RANKL as a “decoy” receptor to inhibit the bone resorption (Theoleyre et al., 2004). Moreover, the secreted protein transforming growth factor (TGF)-β1 is able to promote the aggregation, proliferation, and differentiation of osteoblast and increasing OPG expression while reducing RANKL expression (Janssens et al., 2005). The formation of osteoblasts and osteoclasts is shown schematically in Figure 1.
Bone defects can heal spontaneously to an extent, particularly in younger individuals (Stevens, 2008). However, they cannot cope with extensive mechanical stimuli or damage that is beyond their regeneration capacity, such as fractures, tumors, osteoporosis, and other bone disorders (Fernandez-Yague et al., 2015). Hence, there is a desperate need for more efficacious treatment. Verrier et al. reviewed the approaches to tissue engineering/regeneration from the perspective of vascularization and gene therapy (Verrier et al., 2016). In general, strategies to accelerate bone regeneration can be categorized as biological, physical, or surgical (Table 1).
Of the methods listed in Table 1, physical stimulation has been preferred to use of pharmacologic agents and surgery by clinicians and patients because it is non-invasive. Magnetic materials have caught the researchers' attention because of their low cost and adaptability. Surgical insertion of a bone substitute directly into the bone defect would appear to be the quickest way to repair bone. However, Grado et al. summarized the most commonly used bone substitutes and pointed out a lack of angiogenesis in the center of the replacement (Fernandez de Grado et al., 2018). A review by Xia et al. (2018) introduced studies of magnetic fields and magnetic nanoparticles (MNPs) on osteogenic enhancements.
Here, we highlight use of magnetic materials in bone applications. We explain their background, factors that influence their use, as well as their design and fabrication methods. In addition, the synergistic effects of magnetic materials with other materials are also discussed.
Magnetic materials usually consist of iron (Fe), cobalt (Co), or nickel (Ni). These materials can produce magnetic fields directly or indirectly. They are functional materials with a very long history and wide range of uses. Magnetic fields may also be generated by variation in an electric field. This includes a concentric magnetic field formed around a cylindrical conductive wire, which is called an electromagnetic field (EMF). EMFs can be subdivided into pulse electromagnetic fields (PEMFs), alternating magnetic field (AMFs), or rotating magnetic fields (RMFs). Static magnetic fields (SMFs) are produced by permanent magnets. Among them, SMFs and PEMFs are used most widely.
Magnetic fields have two main properties: direction and magnitude. Two objects can interact with each other even if separated by space. There are many physical quantities used to describe the magnetic properties of materials, some of which are listed in Table 2.
Table 2. Several main physical quantities used to describe the magnetic properties of materials (Bozorth, 1947).
Recent decades have witnessed the rapid development and wide application of magnetic materials in various fields. For example, “soft” magnetic materials are critical for the next generation of electrical machines to enable sustainable electricity worldwide (Silveyra et al., 2018). Moreover, some magnetic materials with coatings have been utilized to separate various molecules and cells (Kudr et al., 2017). Magnetic particles and composites can be used for drug delivery (Amiri et al., 2019), imaging-based diagnosis of cancer (Belyanina et al., 2017) and non-invasive clinical measurements (e.g., measurement of Fe levels in the liver) (Avrin and Kumar, 2007). Scientists have focused on the synthesis of materials with magnetic effects and their biological performance (Tampieri et al., 2014; Cao et al., 2018; Patel et al., 2018). A great advantage of magnetic materials is that they can deliver biological “cues” without the need for contact with tissues. Besides, magnets can be made into various appropriate formulations, making it easier to locate them at the appropriate sites to influence physiological processes.
The factors affecting magnetic properties must be clarified so that better use of materials can be made for research and production. For example, in biosensing, higher Ms is preferred because it provides higher sensitivity and efficiency than lower Ms (Colombo et al., 2012). Here, we focused mainly on the effects of the size, temperature, composition and preparation methods on the magnetic properties of materials because these four parameters have great practical utility and can be manipulated if appropriate methods are employed.
Magnetic materials can be blocks, membranes or even fluids. Nevertheless, it is the size of each magnetic particle not the magnetic material itself that influences the properties of the magnetic composite. A decreasing diameter can enhance Hc; that is, the smaller the diameter, the stronger is the ability to retain magnetism. From 1962 to 1975, researchers reduced the size of a γ-Fe2O3 particle from 1 to 0.3 μm, and Hc increased nearly 30-fold (Aharoni et al., 1962b; Matsui et al., 1976). If a magnetic particle is reduced to a certain size, its magnetic direction changes readily with the external magnetic field (“superparamagnetism”) (Bean and Livingston, 1959). Hence, improving the thermal stability of a magnetic particle while reducing its size could help to obtain higher Hc. Moreover, the magnitude of Ms depends upon the size of nanoparticle (NP) to some extent (Jun et al., 2008). However, Peddis et al. found that large NPs showed lower Hc compared with smaller NPs, suggesting that other factors influence their magnetic properties (Peddis et al., 2008).
In 1895, the future Nobel Prize winner Pierre Curie found that ferromagnetic materials behaved in a paramagnetic manner above a certain temperature, and named it the “Curie temperature” (Tc). There are dozens of types of magnetic materials, each with its own Tc (Oguchi et al., 1983; Mohn and Wohlfarth, 1987). For example, the Tc of α-Fe2O3 is 725°C (Aharoni et al., 1962a).
The different compositions of magnetic materials also have considerable influence on their properties. In 1978, Uehori et al. claimed that the maximum Hc could be reached using 70% of Fe and 30% of Co (Uehori et al., 1978). In 2007, Chaubey et al. found Ms reached a maximum if the molar ratio of Fe:Co was 1.5:1 (Chaubey et al., 2007). The performances of biomedical devices can be improved by adjusting the composition of magnetic materials. But the effects of different compositions are usually detected in vitro, which has resulted in a lack of data for in vivo applications.
The preparation method also has an important impact on magnetic properties. Traditionally, magnetic powder is made by grinding, during which the grains are deformed readily, causing internal stress, which is unfavorable to the properties of the final magnetic products. In 1989, Coehoorn et al. heated a metal slowly to a certain temperature, maintained it for a sufficient time, and then cooled it at an appropriate rate. This annealing procedure eliminated the internal stress, restored the magnetism, and optimized the properties of permanent magnets. Moreover, the permeability of the magnetic material will decrease whereas the Hc will increase if appropriate annealing measures are not taken (Coehoorn et al., 1989).
The magnetic properties depend upon the synthetic method, too. For example, Clavel et al. observed that when benzyl alcohol was used, co-doped MNPs were ferromagnetic, but when an anisole/benzyl alcohol solvent system was used, the MNPs were antiferromagnetic (Clavel et al., 2007). Hence, using different solvents during the synthesis may produce materials with opposite magnetic properties.
In addition to the four main factors mentioned above, other factors are related to magnetization, such as the relative distribution of the cation in the crystal structure and the shape of magnetic materials. Comparisons of the parameters influencing magnetic properties are listed in Table 3.
Magnetic materials can create magnetic fields (including SMFs and PEMFs), which are closely related to the survival and evolution of creatures on Earth because all organisms are under a geomagnetic field (Lohmann, 2010). Moreover, magnetism arises from moving electrons, but cells, tissues, and organs have magnetic fields of their own, and different magnetic fields may interact with each other. Therefore, several scientific research have paid attention to the possible health effects of magnetic materials.
The therapeutic functions of magnetic fields have attracted the attention of people for many years. The first attempt toward application of magnets was made at the Pitié-Salpêtrière Hospital in Paris (Richet, 1880). Since the twenty-first century, magnetic materials have been used more widely in medicine, such as in breast-cancer therapy (Zheng et al., 2018), treatment of bacterial infections (Xu et al., 2019), cardiovascular repair (Vosen et al., 2016b), neural regeneration (Funnell et al., 2019), and especially in the skeletal system (Thevenot et al., 2013).
There are relatively more research about the effects of magnetic fields on nervous system and skeletal system. It is reported that low-frequency magnetic fields: (i) can active N-methyl-D-aspartate receptors in glutamatergic Ca2+ channels to promote neuronal differentiation (Ozgun et al., 2019); (ii) may improve depressant behavior and cognitive dysfunction mainly by modulating synaptic function (Yang et al., 2019). However, repeated stimulations of intracranial nerves may bring inestimable side effects, and the penetration depth is relatively limited.
SMFs has been reported to have the ability to inhibit proliferation of cancer cells by influencing the orientation of kinase domains of epidermal growth factor receptors (EGFRs) (Zhang et al., 2016a). Also, bare iron-oxide NPs are able to produce significant amounts of reactive oxygen species (ROS), which can disrupt the mitochondrial activity of tumor cells at a certain concentration (Gokduman and Gok, 2020). However, cancer treatment using magnetic materials is achieved mainly by the delivery of special anticancer drugs to cancer cells through MNPs (Fang et al., 2019; Gawali et al., 2019; Kim et al., 2019), rather than reliance only on the influence of magnetic fields. The reason might be due to the excessive intensity (>1 T) of the magnetic fields needed to treat tumor cells (Zhang et al., 2016a; Gokduman and Gok, 2020), which will interfere with other normal physiological activities of the body.
Complexes of lentiviral vectors and MNPs have been used to re-endothelialize and restore vascular function (Vosen et al., 2016a). Bekhite et al. found that SMFs increase cardiomyocyte differentiation of Flk-1+ cells derived from mouse embryonic stem cells via Ca2+ influx and ROS production (Bekhite et al., 2013). Nevertheless, excessive magnetic fields may affect the EGFR pathway (Gellrich et al., 2018).
Studies about the antibacterial properties of magnetic fields are relatively superficial. Magnetic fields can destroy bacterial biofilms to a certain extent, thereby reducing the infection prevalence after some surgical procedures (Chopra et al., 2017; Chang et al., 2018; Junka et al., 2018).
Specific drugs that can affect bone defects directly are not available, which hampers treatment of bone defects (Borrelli et al., 2003). Studies on magnetic materials that promote bone regeneration have demonstrated their efficacy.
Weak SMFs and weak PEMFs have been found to promote the proliferation, orientation, and migration of osteoblast-like cells (Yamamoto et al., 2003; Ba et al., 2011; Kim et al., 2015), and to induce osteogenic differentiation in bone marrow-derived MSCs (Schäfer et al., 2010; Kim et al., 2015; Huang et al., 2017). Histology has shown that magnetic field could enhance the activity of bone cells and activate remodeling of alveolar bone (Stark and Sinclair, 1987; Darendeliler et al., 1995).
The direction and intensity of a magnetic field has an influence on bone regeneration. Kotani et al. reported that cultured MC3T3-E1 cells (which are pre-osteoblasts from the calvarias of C57BL/6 mice) were parallel to magnetic fields after 60-h exposure to a SMF (Kotani et al., 2002), which was the first study to show that the growth direction of adherent cells could be regulated by magnetic fields. Besides, in a certain range, as the magnetic-field intensity increases, the biological effects of a magnetic field become more obvious but, beyond a certain range, the effects will decrease and even become inhibitory (“biological window”) (Markov, 2005).
The beneficial effects of a certain intensity of a SMF on osteogenesis have been demonstrated. In 1964, Kotani et al. found that skeletal anabolic responses were enhanced upon exposure to a high-magnitude SMF in vivo and in vitro (Patel, 1964). Moreover, the SMF could accelerate fracture healing of osteoporotic rats by increasing the local bone mineral density (BMD) (Yan et al., 1998). Miyakoshi et al. described the capacity of SMF to regulate the proliferation, morphology and apoptosis of cells (Miyakoshi, 2005). In 2016, Yun et al. used SMFs with Fe NPs to promote the formation of new bone. And they found the expression of bone morphogenetic protein (BMP)-2 was upregulated and alkaline phosphatase (ALP) activity was enhanced (Yun et al., 2016). Yun and coworkers suggested that these effects may be because the Fe NPs become superparamagnetic due to the SMF, which was conducive to the adhesion and proliferation of osteoblasts. Zhang et al. also reported that SMFs improved expression of bone-associated genes such as BMP-2 and runt-related transcription factor 2 (RUNX2) (Zhang et al., 2018b). Yang and coworkers found that SMFs of different intensity had different effects on osteoblast induction and, simultaneously, the concentration of Fe and mRNA expression of transferrin receptor-1 were also affected, suggesting that Fe may be involved in how a magnetic field acts on osteoblasts (Yang et al., 2018). Meanwhile, SMFs can upregulate expression of collagen type II alpha 1 (COL2A1) and SOX9, which are genes related to chondrogenic differentiation of human bone marrow-derived MSCs, and promote the synthesis and secretion of hyaluronic acid and collagen (Son et al., 2015).
EMFs can be divided into three groups: extremely-low-frequency (ELF) fields (0–300 Hz), intermediate-frequency fields (300–100 kHz), and radiofrequency fields (100 kHz to 300 GHz) (Ahlbom et al., 2008). Azadian et al. analyzed 39 articles associated with the bone tissue application of EMFs. They found the frequency spectrum of PEMFs, in general, was from 1 to 250 Hz. A frequency <75 Hz had a significant impact upon osteogenesis. The osteogenic effects induced by PEMFs were the most obvious within the range 15–35 Hz (Azadian et al., 2019).
In 1990, Tabrah et al. undertook a study in which 20 osteoporosis-prone women were exposed to a 72 Hz of a PEMF 10 h per day for 12 weeks. Data on the BMD of the radii before, during and after exposure period indicated that the appropriate application of PEMFs might have great clinical value to treat osteoporosis (Tabrah et al., 1990). In 1974, Bassett et al. promoted fracture healing with PEMFs in clinics for the first time (Bassett et al., 1974). In 1979, use of EMFs was approved by the US Food and Drug Administration (FDA) as safe and non-invasive treatment. Since then, several research has shown that PEMFs can elicit therapeutic effects against bone diseases (Bassett et al., 1982; Assiotis et al., 2012). PEMFs are also reported to promote osteogenic differentiation of MSCs by stimulating the mRNA expressions of osteogenic related genes such as RUNX2, ALP, BMP-2, and distal-less homeobox 5 (DLX5) (Yang et al., 2010). Sollazzo et al. exposed human osteoblasts (MG-63) to PEMFs for 18 h, and found that PEMFs induced upregulation of expression of homeobox A10 (HOXA10) and signal transducer and activator of transcription 3 (STAT3), which are important factors in bone formation (Sollazzo et al., 2010). Low-frequency PEMFs have also been shown to promote TGF-β1 release (Li et al., 2007; Shen and Zhao, 2010).
Different types and parameters of magnetic fields may have different effects on bone cells, some of which are summarized in Table 4. Irrespective of whether SMFs or PEMFs are employed, the effects on bone regeneration may be related mainly to activation of Wnt/β-catenin (Zhou et al., 2012), RANK/RANKL/OPG (Lei et al., 2017, 2018) or MAPK (mitogen-activated protein kinase) (Yong et al., 2016) signaling pathways. Moreover, bone tissue may be sensitive to low-frequency PEMFs, which may be associated with strong transmembrane potential changes and Ca2+ influx. Also, the capacity of SMFs to promote the proliferation and division of osteoblasts could be due to cytoskeletal changes.
Table 4. Studies on the biological effects and mechanisms of different magnetic materials on bone regeneration in the last two decades.
There have been concerns regarding the negative effects of magnetic fields on human health during radiography such as magnetic resonance imaging (MRI), which is the most widely used application of magnetic fields, and using between 0.15 T and 3 T (Wada et al., 2010). The International Commission on Non-Ionizing Radiation Protection published exposure guidelines for SMFs and set the limit for SMF exposure at 400 mT (International Commission On Non-ionizing Radiation, 1994).
SMFs are relatively safe because they do not emit electromagnetic radiation. It has been reported that 2-h exposure in a SMF of 3.5–23.0 T does not have serious long-term effects on mice (Tian et al., 2019). Wang and colleagues demonstrated the safety of exposure to SMFs of 2–12 T (Wang et al., 2019).
Reddy et al. found that 8-week-continuous exposure of PEMFs did not increase genetic toxicity and cytotoxicity significantly according to detection of the micronucleus and polychromatic erythrocytes in the bone marrows and blood of adult male mice (Reddy et al., 2010). The effects of ELF fields on the cardiovascular system was studied but insufficient evidence was found to indicate the side-effects according to Jauchem (1997). Azadian et al. summarized nearly one decade of studies and found scant evidence that EMFs (1–100 mT) affected early embryogenesis adversely in mammals (Azadian et al., 2019).
However, a recent study found that prolonged exposure to ELF fields could stimulate the generation of pro-inflammatory cytokines, thus affecting the immunoreaction (Hosseinabadi et al., 2019). Halgamuge showed that exposure to weak EMFs could disrupt melatonin production, which may result in long-term health impact on humans (Halgamuge, 2013). Available data on EMFs and physiological activities are insufficient to make a definitive claim about the side effects of EMFs on health.
In recent decades, magnetic materials have been designed as films, scaffolds, and implants to meet different needs. Co-precipitation, micro-emulsions, thermal decomposition, ultrasonic irradiation, and sol–gel reactions have been described to prepare magnetic materials with stable properties and controllable shapes (Wu et al., 2016).
The relative proportion of the surface and interface of a membranous material is relatively large, and the properties related to the surface are very prominent. Hence, there are a series of physical effects related to the surface and interface. Biomagnetism scaffolds have been reported to guide the proliferation, differentiation and mineralization of bone cells, thus arousing great interest of researchers in bone healing. Compared with the membrane and the scaffold forms, the block has larger volume and density, is easier to operate, and can produce a more powerful and stable magnetic field, which can be implanted directly into the body or placed on body surface to work. Properties of the three forms of magnetic materials above are summarized in Table 5.
In addition to design and syntheses mentioned above, other methods can be employed. For example, by integrating superparamagnetic NPs into calcium phosphate (CaP) ceramics, in 2010, Yao et al. developed a novel CaP–MNP composite to obtain bone repair which had good biocompatibility with bone cells (Wu et al., 2010). In 2015, Qing et al. prepared magnetic nanofibers through electrospinning polylactide and ferromagnetic Fe3O4 NPs to learn the influence of SMFs on the differentiation of osteoblastic cells (Cai et al., 2015). Moreover, chitosan materials, with excellent biocompatibility, antibacterial, and drug-loading properties (Kassem et al., 2019; Sah et al., 2019), have also been combined with magnetic materials for bone regeneration. In 2012, Shi et al. found that chitosan-coated iron-oxide NPs had excellent biocompatibility, and increased osteoblast proliferation, and decreased damage to cell membranes (Shi et al., 2012). In 2017, Aliramaji et al. synthesized iron-oxide MNPs using reverse co-precipitation, and investigated the effects of chitosan-based magnetic scaffolds with MNPs (0, 0.5, 1, and 2%) on the cellular activity of bone tissue (Aliramaji et al., 2017). In 2018, an MNP composite layer containing collagen, chitosan, and hydroxyapatite was fabricated by Paun et al. to ensure the magnetic properties of three-dimensional biomimetic materials that could accelerate osteogenesis in SMFs (Paun et al., 2018).
Developments in biotechnology and biomedical engineering are continuing apace (Anderson, 2006) but improvements in the biological properties of magnetic materials are important. Avoidance of allogeneic reactions by controlling the adsorption of protein on the interface between the material and body is crucial. Therefore, carrying out in-depth study of biomaterial surfaces is important. Other crucial areas are: (i) surface modification of materials and devices in contact with blood (e.g., biological activation, anti-coagulation); (ii) surface functionalization (e.g., wear resistance of the magnetic material, selective immobilization of biomolecules); (iii) morphology and structure of implanted devices (Hench and Polak, 2002).
As early as 1979, it was reported that SmCo magnets implanted in tissues did not elicit side effects on blood cells (Cerny, 1979) and tissue around the implants (Cerny, 1980). In 2004, Taniguchi et al. placed rats suffering from adjuvant arthritis in a SMF for 12 weeks. The femoral BMD increased greatly compared with that of the control group (Taniguchi et al., 2004). Meng et al. suggested that magnetic films could offer potential in treatment of bone diseases (Meng et al., 2010). In 2013, Meng et al. described osteogenesis enhancement of superparamagnetic nanofibrous scaffolds in vivo with external SMFs by implanting the scaffolds into New Zealand white rabbits with lumbar transverse defects (Meng et al., 2013). In 2017, Bambini et al. inserted dental implants with NdFeB magnets into the tibia of New Zealand white rabbits, and found that the SMF generated by the magnetic implants strengthened bone regeneration (Bambini et al., 2017).
In 1979, PEMFs were approved by the US FDA to treat fracture non-unions. Subsequently, various non-invasive devices, such as PEMFs for osteoporotic fractures in rats (Androjna et al., 2014) and a double-coil system to heal fresh tibial fractures of humans (Hinsenkamp et al., 1984), have been developed for clinical treatment.
The clinical applications of magnetic materials for bone regeneration are in their infancy. With technological developments, magnetic materials for clinical application may develop in three main directions. The first direction is interventional and auxiliary devices for minimally invasive or non-invasive treatment. This will involve development of biodegradable materials, micromachining of implanted instruments, surface anticoagulation, and modification of tissue proliferation. The second direction will involve combination with biologically derived materials. Collagen, sodium hyaluronate, chitosan, and silk fibroin have been used widely in the clinic, but the quality and variety of materials must be improved and expanded. Such alterations will improve the biocompatibility, quality and stability of the material to aid combination of the magnetic material with bioderived materials. The third direction is nano materials such as nano-coatings and carriers for controlled release of nano-drugs. In addition, computer-aided three-dimensional printing could provide precise machining, rapid prototyping, and customization of magnetic materials.
Although scholars have created many designs for application of magnetic materials to aid bone regeneration, their optimal dose, side effects, and long-term stability have yet to be determined.
Another advantage of magnetic materials is that they can be combined readily with other stimuli that have effects on bone regeneration. This interaction, in which the total effect is superior to the sum of the individual effect of each stimulus, is called a “synergistic” or “cooperative” effect.
Effects of electric fields and magnetic fields on biological tissues has been studied since the eighteenth century. Electrical stimulation is beneficial to osteogenesis promotion because it increases expression of the genes associated with osteogenic differentiation (Hu et al., 2018). Moreover, the electrical stimulation on tissue grafts has been found to promote formation of blood vessels and collagen tissue (Fonseca et al., 2018).
A changing electric field can produce a magnetic field. Accordingly, changing the magnetic field (e.g., EMF, PEMF) can generate electric currents in tissues. Therefore, PEMFs and EMFs combine electricity and magnetism, which promote bone regeneration.
We synthesized a flexible nanocomposite P (VDF-TrFE) membrane that can mimic the endogenous electric potential and explored its efficacy for bone-defect repair (Zhang et al., 2016b). We found that the membrane accelerated osteogenesis and bone maturity both in vitro and in vivo, thereby offering an innovative method to promote bone regeneration. In 2018, we adjusted the surface potential of the membrane (Zhang et al., 2018a). This is a development trend for biomaterials proposed by Winkler et al. which is a new way to heal bone defects by mimicking the biology of bone healing (Winkler et al., 2018).
However, the coupling of electricity and magnetism is focused mainly on alternating current. Besides, the human body is a conductor of electricity, so the intensity of current must be calibrated very carefully to avoid causing damage to it.
A magnet has two opposite poles: north and south. The same poles produce a repulsive force whereas different poles attract each other. Hence, two magnets can apply a force to each other without making contact. Moreover, an object placed between them is also affected by the magnetic field simultaneously.
In 1986, Jackson and Thomas used magnetic force as an aid to improve retention of osseointegration of rare-earth magnetic applications (Jackson, 1986). The combination of magnetism and force is used often in dentistry. In 1987, Kawata et al. designed the first magnetic brackets, which were made from an alloy of Fe, Co, and chrome. However, they could not provide sufficient force to move teeth, so rare-earth magnets were designed subsequently to replace them (Kawata et al., 1987). In 1995, Darendeliler et al. compared the impact on movement of orthodontic tooth of three forms of stimuli using male Hartley guinea pigs (Darendeliler et al., 1995). Groups with a SmCo magnet or PEMF stimulation combined with a coil-spring force increased the amount of new bone deposited in the tension area compared with the control group with coil-spring force alone. Two years later, they wrote a review introducing clinical applications of magnets in orthodontics and the biological implications (Darendeliler et al., 1997).
Scholars have found that the force generated by magnetic materials is more efficacious with less pathological and traumatic changes in oral environment compared with using only mechanical force to move teeth (Blechman and Smiley, 1978; Tomizuka et al., 2006). A great advantage of magnetic force is that once the magnetic material is placed in an appropriate position, it can act without contact through an external magnetic field. However, adding force to the corresponding area is easy but control is difficult because the magnitude of the force fluctuates greatly with the distance.
Hyperthermia can lead to enhancement of tissue perfusion, skeletal muscles relaxation, and tension reduction in soft tissue (Schmidt and Simon, 2001). In recent years, thermotherapy has also been found to have positive effects upon bone formation. Ota et al. assessed the influence of thermal stimulation on osteogenesis in rat and rabbit models. They demonstrated that a heat stimulus could facilitate bone formation, and could be promising treatment for diseases related to bone defects (Ota et al., 2017).
The main challenge of using thermotherapy against diseases is how to apply the appropriate amount of heat to the target area without interfering with other areas to prevent complications (heat has good conductivity in the human body). In recent years, scientists have discovered that magnetic materials can be used to solve this problem. For example, Petryk et al. used alternating magnetic fields (AMFs) with Fe particles to induce hyperthermia locally because heat can be produced from the hysteresis loss of magnetic particles under AMFs (Petryk et al., 2013). Using MNPs, we achieved artificial local control of temperature within selected regions. However, this approach is used mainly for the treatment of tumors and cancers, and has been less studied in bone regeneration.
Moreover, magnetism and hyperthermia can be combined with phototherapy. Lu et al. designed SrFe12O19 MNP chitosan porous scaffolds (MBCSs) with excellent osteogenic capacity and antitumor function (Lu et al., 2018). Not only did the magnetic field produced by the MBCSs promote expression of osteoblast-specific genes and regeneration of new bone, it also improved photothermal conversion. Tumor cells cultured on scaffolds underwent apoptosis due to temperature increases under the irradiation of a near-infrared laser. Therefore, a combination of phototherapy with magnetic materials could be used against tumor-related bone defects.
Bone regeneration, as a highly complex physiological process, has been studied widely. Several methods have been employed to enhance bone formation preclinically and clinically. Nevertheless, reducing the duration of bone regeneration is challenging because of the uncertainties and problems of each method. For example, although bone-like calcium phosphate has been used widely, Islam et al. pointed out that some factors, such as microbial contamination and precursor-phase residues, lead to treatment failure (Islam et al., 2017).
Magnetic fields can be applied to an object without touching it, and the properties of magnetic materials are improving. However, most of the magnetic materials used to promote bone regeneration are based on body implantation, which leads to four main problems. First, postoperative infection and rejection are constant problems and may affect the pattern of bone deposition. Second, magnetic materials may affect some types of examination and treatment (e.g., MRI) because of the metal elements they contain. Third, the alloys used in magnetic materials, if not protected appropriately, are highly susceptible to corrosion by tissue fluids. The fourth problem is the possible local and systemic toxic effects of the alloys used in n magnet synthesis. Consequently, how to apply magnetic materials in a non-invasive way will become a focus of future explorations.
In addition, even though animal models have been used widely, there are several difficulties in controlling the many variables associated with bone regeneration in humans. For example, animals from the same species can have anatomical, biochemical and gene-expression differences, and species differences can hamper data interpretation for clinical application.
Moreover, bone reconstruction is extremely complex, involving many components acting at different time steps. Hence, ascertaining the parameters of magnetic materials that produce the optimal effects (e.g., intensity of the magnetic field, shape of the material, alloy composition, preparation process) is crucial. Also, combination of magnetism with light, sound, or heat may have a stronger effect on bone regeneration that magnetism alone. Hence, clearer understanding at the cellular level of the effects of magnetic fields is important.
Wearing a device that provides magnetism for long periods of time (e.g., 6 months) may be burdensome for some patients. Also, the cost of magnetic devices is a burden. Therefore, a magnetic-material industry with the goals of low energy consumption, low environmental pollution, and low cost could be very important for treatment of bone defects in the future.
LC, JP, and JZ conceived of and wrote the manuscript. JP and JZ are co-first authors of the manuscript. YL, YX, and JN contributed to original draft preparation. LC critically revised the manuscript.
This work was supported by the National Science Fund for Distinguished Young Scholars (Grant No. 31725011) and the National Natural Science Foundation of China (Grant No. 2017YFC1104301).
The authors declare that the research was conducted in the absence of any commercial or financial relationships that could be construed as a potential conflict of interest.
Financial support from the National Science Fund for Distinguished Young Scholars and the National Natural Science Foundation of China (NSFC) is gratefully acknowledged.
Acikan, I., Mehmet, G., Artas, G., Yaman, F., Deniz, G., Bulmus, O., et al. (2018). Systemic melatonin application increases bone formation in mandibular distraction osteogenesis. Braz. Oral Res. 32:e85. doi: 10.1590/1807-3107bor-2018.vol32.0085
Aharoni, A., Frei, E. H., and Schieber, M. (1962a). Curie point and origin of weak ferromagnetism in hematite. Phys. Rev. 127, 439–441. doi: 10.1103/PhysRev.127.439
Aharoni, A., Frei, E. H., and Schieber, M. (1962b). Some properties of γ-Fe2O3 obtained by hydrogen reduction of α-Fe2O3. J. Phys. Chem. Solids 23, 545–554. doi: 10.1016/0022-3697(62)90512-7
Ahlbom, A., Bridges, J., de Seze, R., Hillert, L., Juutilainen, J., Mattsson, M. O., et al. (2008). Possible effects of electromagnetic fields (EMF) on human health–opinion of the scientific committee on emerging and newly identified health risks (SCENIHR). Toxicology 246, 248–250. doi: 10.1016/j.tox.2008.02.004
Albornoz, C., and Jacobo, S. E. (2006). Preparation of a biocompatible magnetic film from an aqueous ferrofluid. J. Magn. Magn. Mater. 305, 12–15. doi: 10.1016/j.jmmm.2005.11.021
Aliramaji, S., Zamanian, A., and Mozafari, M. (2017). Super-paramagnetic responsive silk fibroin/chitosan/magnetite scaffolds with tunable pore structures for bone tissue engineering applications. Mater. Sci. Eng. 70, 736–744. doi: 10.1016/j.msec.2016.09.039
Amiri, M., Salavati-Niasari, M., and Akbari, A. (2019). Magnetic nanocarriers: evolution of spinel ferrites for medical applications. Adv. Colloid Interface Sci. 265, 29–44. doi: 10.1016/j.cis.2019.01.003
Anderson, J. M. (2006). The future of biomedical materials. J. Mater. Sci. 17, 1025–1028. doi: 10.1007/s10856-006-0439-5
Androjna, C., Fort, B., Zborowski, M., and Midura, R. J. (2014). Pulsed electromagnetic field treatment enhances healing callus biomechanical properties in an animal model of osteoporotic fracture. Bioelectromagnetics 35, 396–405. doi: 10.1002/bem.21855
Assiotis, A., Sachinis, N. P., and Chalidis, B. E. (2012). Pulsed electromagnetic fields for the treatment of tibial delayed unions and nonunions. A prospective clinical study and review of the literature. J. Orthop. Surg. Res. 7:24. doi: 10.1186/1749-799X-7-24
Atkins, G. J., Kostakis, P., Pan, B. Q., Farrugia, A., Gronthos, S., Evdokiou, A., et al. (2003). RANKL expression is related to the differentiation state of human osteoblasts. J. Bone Mineral Res. 18, 1088–1098. doi: 10.1359/jbmr.2003.18.6.1088
Avrin, W. F., and Kumar, S. (2007). Noninvasive liver-iron measurements with a room-temperature susceptometer. Physiol. Meas. 28, 349–361. doi: 10.1088/0967-3334/28/4/002
Azadian, E., Arjmand, B., Khodaii, Z., and Ardeshirylajimi, A. (2019). A comprehensive overview on utilizing electromagnetic fields in bone regenerative medicine. Electromagn. Biol. Med. 38, 1–20. doi: 10.1080/15368378.2019.1567527
Ba, X., Hadjiargyrou, M., DiMasi, E., Meng, Y., Simon, M., Tan, Z., et al. (2011). The role of moderate static magnetic fields on biomineralization of osteoblasts on sulfonated polystyrene films. Biomaterials 32, 7831–7838. doi: 10.1016/j.biomaterials.2011.06.053
Bambini, F., Santarelli, A., Putignano, A., Procaccini, M., Orsini, G., Di Iorio, D., et al. (2017). Use of supercharged cover screw as static magnetic field generator for bone healing, 2nd part: in vivo enhancement of bone regeneration in rabbits. J. Biol. Regul. Homeost. Agents 31, 481–485.
Bassett, C. A., Pawluk, R. J., and Pilla, A. A. (1974). Augmentation of bone repair by inductively coupled electromagnetic fields. Science 184, 575–577.
Bassett, C. L., Mitchell, S. N., and Gaston, S. R. (1982). Pulsing electromagnetic field treatment in ununited fractures and failed arthrodeses. JAMA 247, 623–628. doi: 10.1001/jama.1982.03320300027017
Bean, C. P., and Livingston, J. D. (1959). Superparamagnetism. J. Appl. Phys. 30, S120–S129. doi: 10.1063/1.2185850
Bekhite, M. M., Figulla, H. R., Sauer, H., and Wartenberg, M. (2013). Static magnetic fields increase cardiomyocyte differentiation of Flk-1+ cells derived from mouse embryonic stem cells via Ca2+ influx and ROS production. Int. J. Cardiol. 167, 798–808. doi: 10.1016/j.ijcard.2012.02.020
Belyanina, I., Kolovskaya, O., Zamay, S., Gargaun, A., Zamay, T., and Kichkailo, A. (2017). Targeted magnetic nanotheranostics of cancer. Molecules 22:975. doi: 10.3390/molecules22060975
Benson, T., Menezes, T., Campbell, J., Bice, A., Hood, B., and Prisby, R. (2016). Mechanisms of vasodilation to PTH 1-84, PTH 1-34, and PTHrP 1-34 in rat bone resistance arteries. Osteoporos. Int. 27, 1817–1826. doi: 10.1007/s00198-015-3460-z
Blechman, A. M., and Smiley, H. (1978). Magnetic force in orthodontics. Am. J. Orthod. 74, 435–443. doi: 10.1016/0002-9416(78)90066-0
Bonnet, N., Pierroz, D. D., and Ferrari, S. L. (2008). Adrenergic control of bone remodeling and its implications for the treatment of osteoporosis. J. Musculoskelet Neuronal Interact. 8, 94–104.
Borrelli, J. Jr., Prickett, W. D., and Ricci, W. M. (2003). Treatment of nonunions and osseous defects with bone graft and calcium sulfate. Clin. Orthop. Relat. Res. 411, 245–254. doi: 10.1097/01.blo.0000069893.31220.6f
Boyce, B. F., Xing, L., Shakespeare, W., Wang, Y., Dalgarno, D., Iuliucci, J., et al. (2003). Regulation of bone remodeling and emerging breakthrough drugs for osteoporosis and osteolytic bone metastases. Kidney Int. Suppl. 63, S2–5. doi: 10.1046/j.1523-1755.63.s85.2.x
Caetano-Lopes, J., Canhao, H., and Fonseca, J. E. (2007). Osteoblasts and bone formation. Acta Reumatol. Port. 32, 103–110.
Cai, Q., Shi, Y., Shan, D., Jia, W., Duan, S., Deng, X., et al. (2015). Osteogenic differentiation of MC3T3-E1 cells on poly(l-lactide)/Fe3O4 nanofibers with static magnetic field exposure. Mater. Sci. Eng. 55, 166–173. doi: 10.1016/j.msec.2015.05.002
Cao, D., Pan, L., Li, J., Cheng, X., Zhao, Z., Xu, J., et al. (2018). Investigation on the structures and magnetic properties of carbon or nitrogen doped cobalt ferrite nanoparticles. Sci. Rep. 8:7916. doi: 10.1038/s41598-018-26341-4
Carter, P. H., and Schipani, E. (2006). The roles of parathyroid hormone and calcitonin in bone remodeling: prospects for novel therapeutics. Endocr. Metab. Immune Disord. Drug Targets 6, 59–76. doi: 10.2174/187153006776056666.
Cerny, R. (1979). The biological effects of implanted magnetic fields. Part I. Mammalian blood cells. Aust. Orthod. J. 6, 64–70.
Cerny, R. (1980). The biological effects of implanted magnetic fields. Part II. Mammalian tissues. Aust. Orthod. J. 6, 114–117.
Chang, M., Lin, W. S., Xiao, W., and Chen, Y. N. (2018). Antibacterial effects of magnetically-controlled Ag/Fe3O4 nanoparticles. Materials. 11:659. doi: 10.3390/ma11050659
Chatterjee, J., Haik, Y., and Chen, C. J. (2004). A biocompatible magnetic film: synthesis and characterization. Biomagn. Res. Technol. 2:2. doi: 10.1186/1477-044x-2-2
Chaubey, G. S., Barcena, C., Poudyal, N., Rong, C., Gao, J., Sun, S., et al. (2007). Synthesis and stabilization of FeCo nanoparticles. J. Am. Chem. Soc. 129, 7214–7215. doi: 10.1021/ja0708969
Chopra, R., Shaikh, S., Chatzinoff, Y., Munaweera, I., Cheng, B., Daly, S. M., et al. (2017). Employing high-frequency alternating magnetic fields for the non-invasive treatment of prosthetic joint infections. Sci. Rep. 7:7520. doi: 10.1038/s41598-017-07321-6
Ciombor, D. M., Lester, G., Aaron, R. K., Neame, P., and Caterson, B. (2002). Low frequency EMF regulates chondrocyte differentiation and expression of matrix proteins. J. Orthop. Res. 20, 40–50. doi: 10.1016/S0736-0266(01)00071-7
Clavel, G., Willinger, M. G., Zitoun, D., and Pinna, N. (2007). Solvent dependent shape and magnetic properties of doped ZnO nanostructures. Adv. Funct. Mater. 17, 3159–3169. doi: 10.1002/adfm.200601142
Coehoorn, R., de Mooij, D. B., and de Waard, C. (1989). Meltspun permanent magnet materials containing Fe3B as the main phase. J. Magn. Magn. Mater. 80, 101–104. doi: 10.1016/0304-8853(89)90333-8.
Colombo, M., Carregal-Romero, S., Casula, M. F., Gutierrez, L., Morales, M. P., Bohm, I. B., et al. (2012). Biological applications of magnetic nanoparticles. Chem. Soc. Rev. 41, 4306–4334. doi: 10.1039/c2cs15337h
Darendeliler, M. A., Darendeliler, A., and Mandurino, M. (1997). Clinical application of magnets in orthodontics and biological implications: a review. Eur. J. Orthod. 19, 431–442. doi: 10.1093/ejo/19.4.431
Darendeliler, M. A., Sinclair, P. M., and Kusy, R. P. (1995). The effects of samarium-cobalt magnets and pulsed electromagnetic fields on tooth movement. Am. J. Orthodont. Dentofac. Orthoped. 107, 578–588. doi: 10.1016/S0889-5406(95)70100-1
Davidovitch, Z., Finkelson, M. D., Steigman, S., Shanfeld, J. L., Montgomery, P. C., and Korostoff, E. (1980). Electric currents, bone remodeling, and orthodontic tooth movement. II. Increase in rate of tooth movement and periodontal cyclic nucleotide levels by combined force and electric current. Am. J. Orthod. 77, 33–47.
de Melo Pereira, D., and Habibovic, P. (2018). Biomineralization-inspired material design for bone regeneration. Adv. Healthc. Mater. 7:e1800700. doi: 10.1002/adhm.201800700
De Santis, R., Gloria, A., Russo, T., D'Amora, U., Zeppetelli, S., Dionigi, C., et al. (2011). A basic approach toward the development of nanocomposite magnetic scaffolds for advanced bone tissue engineering. J. Appl. Polym. Sci. 122, 3599–3605. doi: 10.1002/app.34771
De Santis, R., Russo, A., Gloria, A., D'Amora, U., Russo, T., Panseri, S., et al. (2015). Towards the design of 3D fiber-deposited poly(-caprolactone)/Iron-doped hydroxyapatite nanocomposite magnetic scaffolds for bone regeneration. J. Biomed. Nanotechnol. 11, 1236–1246. doi: 10.1166/jbn.2015.2065.
Eells, J. T., Henry, M. M., Summerfelt, P., Wong-Riley, M. T., Buchmann, E. V., Kane, M., et al. (2003). Therapeutic photobiomodulation for methanol-induced retinal toxicity. Proc. Natl. Acad. Sci. U.S.A. 100, 3439–3444. doi: 10.1073/pnas.0534746100
Fang, Z., Li, X., Xu, Z., Du, F., Wang, W., Shi, R., et al. (2019). Hyaluronic acid-modified mesoporous silica-coated superparamagnetic Fe3O4 nanoparticles for targeted drug delivery. Int. J. Nanomed. 14, 5785–5797. doi: 10.2147/IJN.S213974
Fernandez de Grado, G., Keller, L., Idoux-Gillet, Y., Wagner, Q., Musset, A.-M., Benkirane-Jessel, N., et al. (2018). Bone substitutes: a review of their characteristics, clinical use, and perspectives for large bone defects management. J Tissue Eng. 9:6819. doi: 10.1177/2041731418776819
Fernandez-Yague, M. A., Abbah, S. A., McNamara, L., Zeugolis, D. I., Pandit, A., and Biggs, M. J. (2015). Biomimetic approaches in bone tissue engineering: Integrating biological and physicomechanical strategies. Adv. Drug Deliv. Rev. 84, 1–29. doi: 10.1016/j.addr.2014.09.005
Fonseca, J. H. J., Bagne, L., Meneghetti, D. H., Dos Santos, G. M. T., Esquisatto, M. A. M., de Andrade, T. A. M., et al. (2018). Electrical stimulation: complementary therapy to improve the performance of grafts in bone defects? J. Biomed. Mater. Res. Part B Appl. Biomater. 107, 924–932. doi: 10.1002/jbm.b.34187
Friedenberg, Z. B., Andrews, E. T., Smolenski, B. I., Pearl, B. W., and Brighton, C. T. (1970). Bone reaction to varying amounts of direct current. Surg. Gynecol. Obstet. 131, 894–899.
Fujita, S., Yamaguchi, M., Utsunomiya, T., Yamamoto, H., and Kasai, K. (2008). Low-energy laser stimulates tooth movement velocity via expression of RANK and RANKL. Orthod. Craniofac. Res. 11, 143–155. doi: 10.1111/j.1601-6343.2008.00423.x
Funnell, J. L., Balouch, B., and Gilbert, R. J. (2019). Magnetic composite biomaterials for neural regeneration. Front. Bioeng. Biotechnol. 7:179. doi: 10.3389/fbioe.2019.00179
Gawali, S. L., Barick, K. C., Shetake, N. G., Rajan, V., Pandey, B. N., Kumar, N. N., et al. (2019). pH-labile magnetic nanocarriers for intracellular drug delivery to tumor cells. ACS Omega 4, 11728–11736. doi: 10.1021/acsomega.9b01062
Gellrich, D., Schmidtmayer, U., Eckrich, J., Hagemann, J., Becker, S., and Strieth, S. (2018). Modulation of exposure to static magnetic field affects targeted therapy of solid tumors in vivo. Anticancer Res. 38, 4549–4555. doi: 10.21873/anticanres.12759
Gokduman, K., and Gok, A. (2020). In vitro investigation of therapeutic potential of bare magnetite (Fe3O4) nanoparticles (</=100 ppm) on hepatocellular carcinoma cells. J. Nanosci. Nanotechnol. 20, 1391–1400. doi: 10.1166/jnn.2020.17152
Gutfleisch, O. (2000). Controlling the properties of high energy density permanent magnetic materials by different processing routes. J. Phys. D Appl. Phys. 33:R157–172. doi: 10.1088/0022-3727/33/17/201
Halgamuge, M. N. (2013). Pineal melatonin level disruption in humans due to electromagnetic fields and ICNIRP limits. Radiat. Prot. Dosimetry 154, 405–416. doi: 10.1093/rpd/ncs255
Hench, L. L., and Polak, J. M. (2002). Third-generation biomedical materials. Science 295, 1014–1017. doi: 10.1126/science.1067404
Hinsenkamp, M., Burny, F., Donkerwolcke, M., and Coussaert, E. (1984). Electromagnetic stimulation of fresh fractures treated with hoffmann® external fixation. Orthopedics 7, 411–416. doi: 10.3928/0147-7447-19840301-08
Hosseinabadi, M. B., Khanjani, N., Samaei, S. E., and Nazarkhani, F. (2019). Effect of long-term occupational exposure to extremely low-frequency electromagnetic fields on proinflammatory cytokine and hematological parameters. Int. J. Radiat. Biol. 5:1–8. doi: 10.1080/09553002.2019.1642542
Hu, W. W., Chen, T. C., Tsao, C. W., and Cheng, Y. C. (2018). The effects of substrate-mediated electrical stimulation on the promotion of osteogenic differentiation and its optimization. J. Biomed. Mater. Res. Part B Appl. Biomater. 107:1607–19. doi: 10.1002/jbm.b.34253
Huang, H., Williams, R. C., and Kyrkanides, S. (2014). Accelerated orthodontic tooth movement: molecular mechanisms. Am. J. Orthod. Dentofacial Orthop. 146, 620–632. doi: 10.1016/j.ajodo.2014.07.007
Huang, J., Wang, D., Chen, J., Liu, W., Duan, L., You, W., et al. (2017). Osteogenic differentiation of bone marrow mesenchymal stem cells by magnetic nanoparticle composite scaffolds under a pulsed electromagnetic field. Saudi. Pharm. J. 25, 575–579. doi: 10.1016/j.jsps.2017.04.026
International Commission On Non-ionizing Radiation, P. (1994). Guidelines on limits of exposure to static magnetic fields. Health Phys. 66, 100–106.
Islam, M. T., Felfel, R. M., Abou Neel, E. A., Grant, D. M., Ahmed, I., and Hossain, K. M. Z. (2017). Bioactive calcium phosphate-based glasses and ceramics and their biomedical applications: a review. J. Tissue Eng. 8:19170. doi: 10.1177/2041731417719170
Jackson, T. R. (1986). The application of rare earth magnetic retention to osseointegrated implants. Int J. Oral Maxillofac. Implants 1, 81–92.
Janssens, K., ten Dijke, P., Janssens, S., and Van Hul, W. (2005). Transforming growth factor-beta1 to the bone. Endocr. Rev. 26, 743–774. doi: 10.1210/er.2004-0001
Jauchem, J. R. (1997). Exposure to extremely-low-frequency electromagnetic fields and radiofrequency radiation: cardiovascular effects in humans. Int. Arch. Occup. Environ. Health 70, 9–21. doi: 10.1007/s004200050181
Jing, D., Cai, J., Shen, G., Huang, J., Li, F., Li, J., et al. (2011). The preventive effects of pulsed electromagnetic fields on diabetic bone loss in streptozotocin-treated rats. Osteoporos. Int. 22, 1885–1895. doi: 10.1007/s00198-010-1447-3
Jing, D., Cai, J., Wu, Y., Shen, G., Li, F., Xu, Q., et al. (2014). Pulsed electromagnetic fields partially preserve bone mass, microarchitecture, and strength by promoting bone formation in hindlimb-suspended rats. J. Bone Miner. Res. 29, 2250–2261. doi: 10.1002/jbmr.2260
Jun, Y., Seo, J., and Cheon, J. (2008). Nanoscaling laws of magnetic nanoparticles and their applicabilities in biomedical sciences. Acc. Chem. Res. 41, 179–189. doi: 10.1021/ar700121f
Junka, A. F., Rakoczy, R., Szymczyk, P., Bartoszewicz, M., Sedghizadeh, P. P., and Fijalkowski, K. (2018). Application of rotating magnetic fields increase the activity of antimicrobials against wound biofilm pathogens. Sci. Rep. 8:167. doi: 10.1038/s41598-017-18557-7
Karu, T. I. (2008). Mitochondrial signaling in mammalian cells activated by red and near-IR radiation. Photochem. Photobiol. 84, 1091–1099. doi: 10.1111/j.1751-1097.2008.00394.x
Kassem, A., Ayoub, G. M., and Malaeb, L. (2019). Antibacterial activity of chitosan nano-composites and carbon nanotubes: a review. Sci. Total Environ. 668, 566–576. doi: 10.1016/j.scitotenv.2019.02.446
Kawata, T., Hirota, K., Sumitani, K., Umehara, K., Yano, K., Tzeng, H. J., et al. (1987). A new orthodontic force system of magnetic brackets. Am. J. Orthodont. Dentofac. Orthoped. 92, 241–248. doi: 10.1016/0889-5406(87)90418-5
Kenny, A. M., and Raisz, L. G. (2002). Mechanisms of bone remodeling: implications for clinical practice. J. Reprod. Med. 47, 63–70.
Khosla, S. (2001). Minireview: the OPG/RANKL/RANK system. Endocrinology 142, 5050–5055. doi: 10.1210/en.142.12.5050
Kim, C., Kim, H., Park, H., and Lee, K. Y. (2019). Controlling the porous structure of alginate ferrogel for anticancer drug delivery under magnetic stimulation. Carbohydr. Polym. 223:115045. doi: 10.1016/j.carbpol.2019.115045
Kim, E. C., Leesungbok, R., Lee, S. W., Lee, H. W., Park, S. H., Mah, S. J., et al. (2015). Effects of moderate intensity static magnetic fields on human bone marrow-derived mesenchymal stem cells. Bioelectromagnetics 36, 267–276. doi: 10.1002/bem.21903
Kolhatkar, A. G., Jamison, A. C., Litvinov, D., Willson, R. C., and Lee, T. R. (2013). Tuning the magnetic properties of nanoparticles. Int. J. Mol. Sci. 14, 15977–16009. doi: 10.3390/ijms140815977
Kotani, H., Kawaguchi, H., Shimoaka, T., Iwasaka, M., Ueno, S., Ozawa, H., et al. (2002). Strong static magnetic field stimulates bone formation to a definite orientation in vitro and in vivo. J. Bone Miner. Res. 17, 1814–1821. doi: 10.1359/jbmr.2002.17.10.1814
Kudr, J., Haddad, Y., Richtera, L., Heger, Z., Cernak, M., Adam, V., et al. (2017). Magnetic nanoparticles: from design and synthesis to real world applications. Nanomaterials 7:243. doi: 10.3390/nano7090243
Kumari, M., Widdrat, M., Tompa, É., Uebe, R., Schüler, D., Pósfai, M., et al. (2014). Distinguishing magnetic particle size of iron oxide nanoparticles with first-order reversal curves. J. Appl. Phys. 116:124304. doi: 10.1063/1.4896481
Laurent, S., Forge, D., Port, M., Roch, A., Robic, C., Vander Elst, L., et al. (2008). Magnetic iron oxide nanoparticles: synthesis, stabilization, vectorization, physicochemical characterizations, and biological applications. Chem. Rev. 108, 2064–2110. doi: 10.1021/cr068445e
Lei, T., Li, F., Liang, Z., Tang, C., Xie, K., Wang, P., et al. (2017). Effects of four kinds of electromagnetic fields (EMF) with different frequency spectrum bands on ovariectomized osteoporosis in mice. Sci. Rep. 7:553. doi: 10.1038/s41598-017-00668-w
Lei, T., Liang, Z., Li, F., Tang, C., Xie, K., Wang, P., et al. (2018). Pulsed electromagnetic fields (PEMF) attenuate changes in vertebral bone mass, architecture and strength in ovariectomized mice. Bone 108, 10–19. doi: 10.1016/j.bone.2017.12.008
Leucht, P., Lam, K., Kim, J. B., Mackanos, M. A., Simanovskii, D. M., Longaker, M. T., et al. (2007). Accelerated bone repair after plasma laser corticotomies. Ann. Surg. 246, 140–150. doi: 10.1097/01.sla.0000258559.07435.b3
Li, J. K., Lin, J. C., Liu, H. C., and Chang, W. H. (2007). Cytokine release from osteoblasts in response to different intensities of pulsed electromagnetic field stimulation. Electromagn. Biol. Med. 26, 153–165. doi: 10.1080/15368370701572837
Lin, C. R., Chiang, R. K., Wang, J. S., and Sung, T. W. (2006). Magnetic properties of monodisperse iron oxide nanoparticles. J. Appl. Phys. 99:08N710. doi: 10.1063/1.2172891
Lohmann, K. J. (2010). Q&a: animal behaviour: magnetic-field perception. Nature 464, 1140–1142. doi: 10.1038/4641140a
Lombardi, G., Di Somma, C., Rubino, M., Faggiano, A., Vuolo, L., Guerra, E., et al. (2011). The roles of parathyroid hormone in bone remodeling: prospects for novel therapeutics. J. Endocrinol. Invest. 34, 18–22.
Lu, J. W., Yang, F., Ke, Q. F., Xie, X. T., and Guo, Y. P. (2018). Magnetic nanoparticles modified-porous scaffolds for bone regeneration and photothermal therapy against tumors. Nanomedicine 14, 811–822. doi: 10.1016/j.nano.2017.12.025
Markov, M. S. (2005). “Biological Windows”: a tribute to W. Ross Adey. Environmentalist 25, 67–74. doi: 10.1007/s10669-005-4268-8
Matsui, G., Toda, K., Horiishi, N., Wakimoto, K., Nishiyama, Y., Taketa, T., et al. (1976). Process for Producing Iron Oxide Products From Waste Liquids Containing Ferrous Salts. U.S. Patent No. 3970738. 1976-7-20.
McDonald, F. (1993). Effect of static magnetic fields on osteoblasts and fibroblasts in vitro. Bioelectromagnetics 14, 187–196.
Meng, J., Xiao, B., Zhang, Y., Liu, J., Xue, H., Lei, J., et al. (2013). Super-paramagnetic responsive nanofibrous scaffolds under static magnetic field enhance osteogenesis for bone repair in vivo. Sci. Rep. 3:2655. doi: 10.1038/srep02655
Meng, J., Zhang, Y., Qi, X., Kong, H., Wang, C., Xu, Z., et al. (2010). Paramagnetic nanofibrous composite films enhance the osteogenic responses of pre-osteoblast cells. Nanoscale 2, 2565–2569. doi: 10.1039/c0nr00178c
Miyakoshi, J. (2005). Effects of static magnetic fields at the cellular level. Prog. Biophys. Mol. Biol. 87, 213–223. doi: 10.1016/j.pbiomolbio.2004.08.008
Mohn, P., and Wohlfarth, E. P. (1987). The Curie temperature of the ferromagnetic transition metals and their compounds. J. Phys. 17:2421.
Nagai, N., Inoue, M., Ishiwari, Y., Nagatsuka, H., Tsujigiwa, H., Nakano, K., et al. (2000). Age and magnetic effects on ectopic bone formation induced by purified bone morphogenetic protein. Pathophysiology 7, 107–114. doi: 10.1016/s0928-4680(00)00036-5
Nishimura, M., Chiba, M., Ohashi, T., Sato, M., Shimizu, Y., Igarashi, K., et al. (2008). Periodontal tissue activation by vibration: intermittent stimulation by resonance vibration accelerates experimental tooth movement in rats. Am. J. Orthod. Dentofacial Orthop. 133, 572–583. doi: 10.1016/j.ajodo.2006.01.046
Oguchi, T., Terakura, K., and Hamada, N. (1983). Magnetism of iron above the Curie temperature. J. Phys. 13:145.
Ota, T., Nishida, Y., Ikuta, K., Kato, R., Kozawa, E., Hamada, S., et al. (2017). Heat-stimuli-enhanced osteogenesis using clinically available biomaterials. PLoS ONE 12:e0181404. doi: 10.1371/journal.pone.0181404
Ozgun, A., Marote, A., Behie, L. A., Salgado, A., and Garipcan, B. (2019). Extremely low frequency magnetic field induces human neuronal differentiation through NMDA receptor activation. J. Neural Transm. 126:1281–1290. doi: 10.1007/s00702-019-02045-5
Patel, C. K. N. (1964). Selective excitation through vibrational energy transfer and optical Maser action in N2-CO2. Phys. Rev. Lett. 13, 617–619. doi: 10.1103/PhysRevLett.13.617
Patel, K., Zhang, J., and Ren, S. (2018). Rare-earth-free high energy product manganese-based magnetic materials. Nanoscale 10, 11701–11718. doi: 10.1039/c8nr01847b
Paun, A. I., Popescu, C. R., Calin, S. B., Mustaciosu, C. C., Dinescu, M., and Luculescu, R. C. (2018). 3D biomimetic magnetic structures for static magnetic field stimulation of osteogenesis. Int. J. Mol. Sci. 19:E495. doi: 10.3390/ijms19020495
Peddis, D., Mansilla, M. V., Morup, S., Cannas, C., Musinu, A., Piccaluga, G., et al. (2008). Spin-canting and magnetic anisotropy in ultrasmall CoFe2O4 nanoparticles. J. Phys. Chem. 112, 8507–8513. doi: 10.1021/jp8016634
Petryk, A. A., Giustini, A. J., Gottesman, R. E., Kaufman, P. A., and Hoopes, P. J. (2013). Magnetic nanoparticle hyperthermia enhancement of cisplatin chemotherapy cancer treatment. Int. J. Hyperthermia 29, 845–851. doi: 10.3109/02656736.2013.825014
Porter, J. R., Ruckh, T. T., and Popat, K. C. (2009). Bone tissue engineering: a review in bone biomimetics and drug delivery strategies. Biotechnol. Prog. 25, 1539–1560. doi: 10.1002/btpr.246
Reddy, S. B., Weller, J., Desjardins-Holmes, D., Winters, T., Keenliside, L., Prato, F. S., et al. (2010). Micronuclei in the blood and bone marrow cells of mice exposed to specific complex time-varying pulsed magnetic fields. Bioelectromagnetics 31, 445–453. doi: 10.1002/bem.20576
Sah, A. K., Dewangan, M., and Suresh, P. K. (2019). Potential of chitosan-based carrier for periodontal drug delivery. Colloids Surfaces B 178, 185–198. doi: 10.1016/j.colsurfb.2019.02.044
Saunders, R. (2005). Static magnetic fields: animal studies. Prog. Biophys. Mol. Biol. 87, 225–239. doi: 10.1016/j.pbiomolbio.2004.09.001
Schäfer, R., Bantleon, R., Kehlbach, R., Siegel, G., Wiskirchen, J., Wolburg, H., et al. (2010). Functional investigations on human mesenchymal stem cells exposed to magnetic fields and labeled with clinically approved iron nanoparticles. BMC Cell Biol. 11, 22–22. doi: 10.1186/1471-2121-11-22
Schmidt, K. L., and Simon, E. (2001). Thermotherapy of pain, trauma, and inflammatory and degenerative rheumatic disease. Thermother. Neoplasia Inflamm. Pain 2001, 527–539. doi: 10.1007/978-4-431-67035-3_61
Shen, W. W., and Zhao, J. H. (2010). Pulsed electromagnetic fields stimulation affects BMD and local factor production of rats with disuse osteoporosis. Bioelectromagnetics 31, 113–119. doi: 10.1002/bem.20535
Shi, S. F., Jia, J. F., Guo, X. K., Zhao, Y. P., Chen, D. S., Guo, Y. Y., et al. (2012). Biocompatibility of chitosan-coated iron oxide nanoparticles with osteoblast cells. Int. J. Nanomed. 7, 5593–5602. doi: 10.2147/IJN.S34348
Shimizu, Y. (1986). Movement of the lateral incisors in Macaca fuscata as loaded by a vibrating force. Nihon Kyosei Shika Gakkai Zasshi 45, 56–72.
Silveyra, J. M., Ferrara, E., Huber, D. L., and Monson, T. C. (2018). Soft magnetic materials for a sustainable and electrified world. Science 362:eaao0195. doi: 10.1126/science.aao0195
Singh, R. K., Patel, K. D., Lee, J. H., Lee, E.-J., Kim, J.-H., Kim, T.-H., et al. (2014). Potential of magnetic nanofiber scaffolds with mechanical and biological properties applicable for bone regeneration. PLoS ONE 9:e91584. doi: 10.1371/journal.pone.0091584
Sollazzo, V., Palmieri, A., Pezzetti, F., Massari, L., and Carinci, F. (2010). Effects of pulsed electromagnetic fields on human osteoblastlike cells (MG-63): a pilot study. Clin. Orthop. Relat. Res. 468, 2260–2277. doi: 10.1007/s11999-010-1341-5
Son, B., Kim, H. D., Kim, M., Kim, J. A., Lee, J., Shin, H., et al. (2015). Physical stimuli-induced chondrogenic differentiation of mesenchymal stem cells using magnetic nanoparticles. Adv. Healthc. Mater. 4, 1339–1347. doi: 10.1002/adhm.201400835
Stark, T. M., and Sinclair, P. M. (1987). Effect of pulsed electromagnetic fields on orthodontic tooth movement. Am. J. Orthod. Dentofacial Orthop. 91, 91–104. doi: 10.1016/0889-5406(87)90465-3
Stevens, M. M. (2008). Biomaterials for bone tissue engineering. Mater. Today 11, 18–25. doi: 10.1016/S1369-7021(08)70086-5
Tabrah, F., Hoffmeier, M., Gilbert, F. Jr., Batkin, S., and Bassett, C. A. (1990). Bone density changes in osteoporosis-prone women exposed to pulsed electromagnetic fields (PEMFs). J. Bone Miner. Res. 5, 437–442. doi: 10.1002/jbmr.5650050504
Tampieri, A., Iafisco, M., Sandri, M., Panseri, S., Cunha, C., Sprio, S., et al. (2014). Magnetic bioinspired hybrid nanostructured collagen-hydroxyapatite scaffolds supporting cell proliferation and tuning regenerative process. ACS Appl. Mater. Interfaces 6, 15697–15707. doi: 10.1021/am5050967
Taniguchi, N., Kanai, S., Kawamoto, M., Endo, H., and Higashino, H. (2004). Study on application of static magnetic field for adjuvant arthritis rats. Evid. Based Complement. Alternat. Med. 1, 187–191. doi: 10.1093/ecam/neh024
Theoleyre, S., Wittrant, Y., Tat, S. K., Fortun, Y., Redini, F., and Heymann, D. (2004). The molecular triad OPG/RANK/RANKL: involvement in the orchestration of pathophysiological bone remodeling. Cytokine Growth Factor Rev. 15, 457–475. doi: 10.1016/j.cytogfr.2004.06.004
Thevenot, J., Oliveira, H., Sandre, O., and Lecommandoux, S. (2013). Magnetic responsive polymer composite materials. Chem. Soc. Rev. 42, 7099–7116. doi: 10.1039/c3cs60058k
Tian, X., Wang, D., Feng, S., Zhang, L., Ji, X., Wang, Z., et al. (2019). Effects of 3.5-23.0T static magnetic fields on mice: a safety study. Neuroimage 199, 273–280. doi: 10.1016/j.neuroimage.2019.05.070
Tomizuka, R., Kanetaka, H., Shimizu, Y., Suzuki, A., Igarashi, K., and Mitani, H. (2006). Effects of gradually increasing force generated by permanent rare earth magnets for orthodontic tooth movement. Angle Orthod. 76, 1004–1009. doi: 10.2319/071805-237
Uehori, T., Hosaka, A., Tokuoka, Y., Izumi, T., and Imaoka, Y. (1978). Magnetic properties of iron-cobalt alloy particles for magnetic recording media. IEEE Trans. Magn. 14, 852–854. doi: 10.1109/TMAG.1978.1059929
Verrier, S., Alini, M., Alsberg, E., Buchman, S. R., Kelly, D., Laschke, M. W., et al. (2016). Tissue engineering and regenerative approaches to improving the healing of large bone defects. Eur. Cell Mater. 32, 87–110. doi: 10.22203/eCM.v032a06
Vosen, S., Rieck, S., Heidsieck, A., Mykhaylyk, O., Zimmermann, K., Bloch, W., et al. (2016a). Vascular repair by circumferential cell therapy using magnetic nanoparticles and tailored magnets. ACS Nano 10, 369–376. doi: 10.1021/acsnano.5b04996
Vosen, S., Rieck, S., Heidsieck, A., Mykhaylyk, O., Zimmermann, K., Plank, C., et al. (2016b). Improvement of vascular function by magnetic nanoparticle-assisted circumferential gene transfer into the native endothelium. J. Control. Release 241, 164–173. doi: 10.1016/j.jconrel.2016.09.024
Wada, H., Sekino, M., Ohsaki, H., Hisatsune, T., Ikehira, H., and Kiyoshi, T. (2010). Prospect of high-field MRI. IEEE Trans. Appl. Superconduct. 20, 115–122. doi: 10.1109/TASC.2010.2043939
Wang, S., Luo, J., Lv, H., Zhang, Z., Yang, J., Dong, D., et al. (2019). Safety of exposure to high static magnetic fields (2 T-12 T): a study on mice. Eur. Radiol. 29, 6029–6037. doi: 10.1007/s00330-019-06256-y
Winkler, T., Sass, F. A., Duda, G. N., and Schmidt-Bleek, K. (2018). A review of biomaterials in bone defect healing, remaining shortcomings and future opportunities for bone tissue engineering: the unsolved challenge. Bone Joint Res. 7, 232–243. doi: 10.1302/2046-3758.73.BJR-2017-0270.R1
Wu, W., Wu, Z., Yu, T., Jiang, C., and Kim, W. S. (2016). Recent progress on magnetic iron oxide nanoparticles: synthesis, surface functional strategies and biomedical applications. Sci. Tech. Adv. Mater. 16:023501. doi: 10.1088/1468-6996/16/2/023501
Wu, Y., Jiang, W., Wen, X., He, B., Zeng, X., Wang, G., et al. (2010). A novel calcium phosphate ceramic-magnetic nanoparticle composite as a potential bone substitute. Biomed. Mater. 5:15001. doi: 10.1088/1748-6041/5/1/015001
Xia, Y., Sun, J., Zhao, L., Zhang, F., Liang, X. J., Guo, Y., et al. (2018). Magnetic field and nano-scaffolds with stem cells to enhance bone regeneration. Biomaterials 183, 151–170. doi: 10.1016/j.biomaterials.2018.08.040
Xu, C., Akakuru, O. U., Zheng, J., and Wu, A. (2019). Applications of iron oxide-based magnetic nanoparticles in the diagnosis and treatment of bacterial infections. Front. Bioeng. Biotechnol. 7:141. doi: 10.3389/fbioe.2019.00141
Xu, S., Okano, H., Tomita, N., and Ikada, Y. (2011). Recovery effects of a 180 mt static magnetic field on bone mineral density of osteoporotic lumbar vertebrae in ovariectomized rats. Evid. Based Complement. Alternat. Med. 2011:620984. doi: 10.1155/2011/620984
Yamamoto, Y., Ohsaki, Y., Goto, T., Nakasima, A., and Iijima, T. (2003). Effects of static magnetic fields on bone formation in rat osteoblast cultures. J. Dent. Res. 82, 962–966. doi: 10.1177/154405910308201205
Yan, Q. C., Tomita, N., and Ikada, Y. (1998). Effects of static magnetic field on bone formation of rat femurs. Med. Eng. Phys. 20, 397–402. doi: 10.1016/S1350-4533(98)00051-4
Yan, W., Xuehui, Z., Yu, S., Bing, H., Xiaoyang, H., Xinzhi, W., et al. (2011). Magnetic biodegradable Fe3O4 /CS/PVA nanofibrous membranes for bone regeneration. Biomed. Mater. 6:055008. doi: 10.1088/1748-6041/6/5/055008
Yang, J., Wang, L., Wang, F., Tang, X., Zhou, P., Liang, R., et al. (2019). Low-frequency pulsed magnetic field improves depression-like behaviors and cognitive impairments in depressive rats mainly via modulating synaptic function. Front. Neurosci. 13:820. doi: 10.3389/fnins.2019.00820
Yang, J., Zhang, J., Ding, C., Dong, D., and Shang, P. (2018). Regulation of osteoblast differentiation and iron content in MC3T3-E1 cells by static magnetic field with different intensities. Biol. Trace Elem. Res. 184, 214–225. doi: 10.1007/s12011-017-1161-5
Yang, Y., Tao, C., Zhao, D., Li, F., Zhao, W., and Wu, H. (2010). EMF acts on rat bone marrow mesenchymal stem cells to promote differentiation to osteoblasts and to inhibit differentiation to adipocytes. Bioelectromagnetics 31, 277–285. doi: 10.1002/bem.20560
Yong, Y., Ming, Z. D., Feng, L., Chun, Z. W., and Hua, W. (2016). Electromagnetic fields promote osteogenesis of rat mesenchymal stem cells through the PKA and ERK1/2 pathways. J. Tissue Eng. Regen. Med. 10, E537–E545. doi: 10.1002/term.1864
Yun, H. M., Ahn, S. J., Park, K. R., Kim, M. J., Kim, J. J., Jin, G. Z., et al. (2016). Magnetic nanocomposite scaffolds combined with static magnetic field in the stimulation of osteoblastic differentiation and bone formation. Biomaterials 85, 88–98. doi: 10.1016/j.biomaterials.2016.01.035
Zhang, C., Liu, W., Cao, C., Zhang, F., Tang, Q., Ma, S., et al. (2018a). Modulating surface potential by controlling the beta phase content in poly(vinylidene fluoridetrifluoroethylene) membranes enhances bone regeneration. Adv. Healthc. Mater. 7:e1701466. doi: 10.1002/adhm.201701466
Zhang, H., Gan, L., Zhu, X., Wang, J., Han, L., Cheng, P., et al. (2018b). Moderate-intensity 4mT static magnetic fields prevent bone architectural deterioration and strength reduction by stimulating bone formation in streptozotocin-treated diabetic rats. Bone 107, 36–44. doi: 10.1016/j.bone.2017.10.024
Zhang, L., Wang, J., Wang, H., Wang, W., Li, Z., Liu, J., et al. (2016a). Moderate and strong static magnetic fields directly affect EGFR kinase domain orientation to inhibit cancer cell proliferation. Oncotarget 7, 41527–41539. doi: 10.18632/oncotarget.9479
Zhang, X., Zhang, C., Lin, Y., Hu, P., Shen, Y., Wang, K., et al. (2016b). Nanocomposite membranes enhance bone regeneration through restoring physiological electric microenvironment. ACS Nano 10, 7279–7286. doi: 10.1021/acsnano.6b02247
Zhen, G., Muir, B. W., Moffat, B. A., Harbour, P., Murray, K. S., Moubaraki, B., et al. (2011). Comparative study of the magnetic behavior of spherical and cubic superparamagnetic iron oxide nanoparticles. J. Phys. Chem. C 115, 327–334. doi: 10.1021/jp104953z
Zheng, J., Ren, W., Chen, T., Jin, Y., Li, A., Yan, K., et al. (2018). Recent advances in superparamagnetic iron oxide based nanoprobes as multifunctional theranostic agents for breast cancer imaging and therapy. Curr. Med. Chem. 25, 3001–3016. doi: 10.2174/0929867324666170705144642
Keywords: magnetic materials, bone diseases, bone regeneration, magnetic fields, influence factors, synergistic effects
Citation: Peng J, Zhao J, Long Y, Xie Y, Nie J and Chen L (2019) Magnetic Materials in Promoting Bone Regeneration. Front. Mater. 6:268. doi: 10.3389/fmats.2019.00268
Received: 16 July 2019; Accepted: 14 October 2019;
Published: 01 November 2019.
Edited by:
Jian Yang, Pennsylvania State University, United StatesReviewed by:
Hae-Won Kim, Institute of Tissue Regeneration Engineering (ITREN), South KoreaCopyright © 2019 Peng, Zhao, Long, Xie, Nie and Chen. This is an open-access article distributed under the terms of the Creative Commons Attribution License (CC BY). The use, distribution or reproduction in other forums is permitted, provided the original author(s) and the copyright owner(s) are credited and that the original publication in this journal is cited, in accordance with accepted academic practice. No use, distribution or reproduction is permitted which does not comply with these terms.
*Correspondence: Lili Chen, bGlseS1jMTAzMEAxNjMuY29t
†Co-first authors
Disclaimer: All claims expressed in this article are solely those of the authors and do not necessarily represent those of their affiliated organizations, or those of the publisher, the editors and the reviewers. Any product that may be evaluated in this article or claim that may be made by its manufacturer is not guaranteed or endorsed by the publisher.
Research integrity at Frontiers
Learn more about the work of our research integrity team to safeguard the quality of each article we publish.