- 1Austrian Institute of Technology, Vienna, Austria
- 2Flinders Institute for Nanoscale Science and Technology, College of Science and Engineering, Flinders University, Adelaide, SA, Australia
- 3CEST Kompetenzzentrum für elektrochemische Oberflächentechnologie, Wiener Neustadt, Austria
Membrane proteins perform a large number of essential biological tasks, but the understanding of these proteins has progressed much more slowly than that of globular proteins. The study of membrane proteins is hindered by the inherent complexity of the cellular membrane. Membrane proteins cannot be studied outside their native environment because their natural structure and function is compromised when the protein does not reside in the cell membrane. Model membranes have been developed to provide a controlled, membrane-like environment in which these proteins can be studied in their native form and function without interference from other membrane components. Traditionally used model membranes such as bimolecular or black lipid membranes and floating lipid membranes suffer from several disadvantages including complex assembly protocols and limited stability. Furthermore, these membranes can only be studied with a narrow range of methodologies, severely restricting their use. To increase membrane stability, simplify the assembly process and increase the number of analytical tools that can be used to study the membranes, several strategies of covalently tethering the bilayer to its solid support have been developed. This review provides an overview of the methods used to assemble various membrane architectures, the properties of the resulting membranes and the tools used to study them.
Introduction
The cellular membrane is a crucial component of living cells, hosting a vast array of proteins that are essential for cell-cell communication, nutrient import, signal transduction and many other cellular functions. At the same time, the membrane serves as a protective barrier against toxins and pathogens (van Meer et al., 2008; Groves and Kuriyan, 2010; Lingwood and Simons, 2010). Around 30% of an organism's genome encodes membrane proteins (Römer and Steinem, 2004) and 5% of its genes are responsible for lipid synthesis (van Meer et al., 2008). It is therefore of critical importance to better understand membrane proteins and their behavior, but due to the enormous variety of functions performed by the cellular membrane and the proteins that reside there, this challenge is highly complex. Furthermore, while globular proteins can be studied in isolation, membrane proteins require the unique physicochemical environment provided by a lipid bilayer to retain their native form and function. As a result, the determination of the structure and function of membrane proteins has progressed much more slowly than that of water soluble proteins (White, 2004).
Model membranes were developed in order to provide an environment that replicates the physicochemical properties of the cellular membrane while reducing their inherent complexity. In essence, model membranes are bilayers comprised of phospholipids or lipid-like compounds or a mixture thereof. They can be used in the form of free-standing lipid bilayers (bimolecular lipid membranes, or BLMs, also referred to as black lipid membranes), typically spread across a Teflon aperture with a diameter of a few 100 μm (Figure 1; Sackmann, 1996; Winterhalter, 2000). BLMs are also referred to as black lipid membranes as they appear to turn black when bilayer formation is complete. BLMs possess excellent electrical sealing properties (typically at least 100 MΩ·cm2), which results in very low leakage of background currents. This makes them highly suitable for the study of single ion channels. As they generally remain intact only for a few hours, they are not suitable for long-term studies (Römer and Steinem, 2004). Their stability can be improved by coating the membranes in a hydrogel (Jeon et al., 2008). However, their architecture limits the number of analytical tools that can be applied, generally allowing only electrical and some optical methods of characterization.
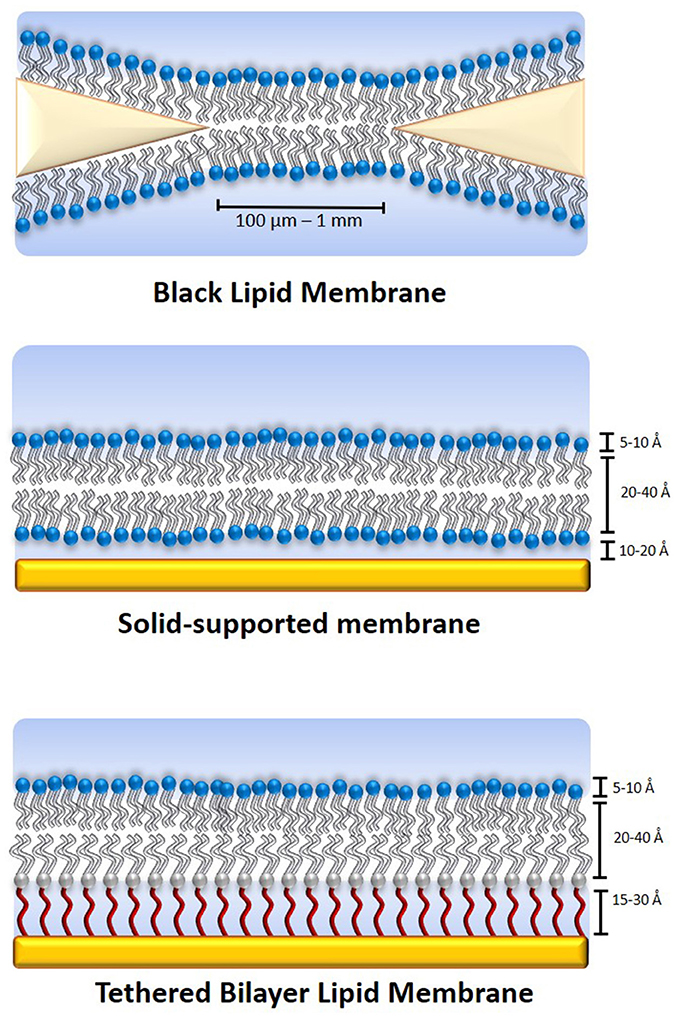
Figure 1. Schematics of various model membrane types. The black lipid membrane (top) is a free-standing lipid bilayer across a small aperture made from a material such as Teflon, which is excellent for the study of single ion channels but has a very limited lifetime, typically of a few hours. It is also severely limited in terms of analytical methods that can be applied to it. A solid-supported membrane (middle) is somewhat more stable and is accessible for a significantly larger number of analytical tools than black lipid membranes. A tethered membrane (bottom) can be stable for weeks or even months and is accessible to the same range of analytical tools. The disadvantage of increased stability is generally reduced lateral lipid mobility.
Depositing the lipid bilayer on a solid support (Figure 1), either directly or on a polymer cushion increases their stability and adds to the range of analytical tools that can be applied (Andersson and Köper, 2016). Typical substrates that have been used include gold, silicon, mica, and glass (Andersson and Köper, 2016; Moulick et al., 2018). They allow the use of tools such as atomic force microscopy (AFM), ellipsometry and neutron scattering. When a metallic supporting surface is used, surface plasmon resonance (SPR) and surface-plasmon enhanced fluorescence spectroscopy (SPFS) can also be used to study membrane-related processes within 150–200 nm of the substrate surface (Wiltschi et al., 2006). This makes SPFS and SPR highly suitable for the study of tethered and supported lipid bilayer membranes which typically have a thickness of around 8–20 nm (Heinrich et al., 2009; Junghans and Koper, 2010; Andersson et al., 2018). When using gold as a substrate, an excitation wavelength of 520 nm or above must be used for SPFS, which has to be considered when selecting fluorophores for the experiment.
Membranes that are tethered to their support (Figure 1) have been used to gain important insight into medical conditions, for example the effect of the agglomeration of β-amyloid oligomers at lipid membranes during Alzheimer's disease (Valincius et al., 2008), the process the HIV 1-Gag protein binding to a lipid membrane (Datta et al., 2011) and the effects of oxidative damage on the cellular membrane (Knobloch et al., 2015). Furthermore, tethered membranes can serve as stable and highly flexible platforms for biosensing applications (Cornell et al., 1997; Jackman et al., 2012). Model membranes have also been developed that mimic the outer membrane of gram-negative bacteria, which can cause some of the most dangerous multi-drug resistant infections (Clifton et al., 2015; Andersson et al., 2018).
However, when deposited on a solid support, the electrical sealing properties of the membrane are subject to the roughness and defects of the supporting surface. As the lipid bilayer can still move freely because there is no direct linkage between the lipid bilayer and the support, the membrane remains fragile and lacks longevity. Furthermore, because the membrane is in direct contact with the supporting surface there is insufficient space (generally only a few Å; Castellana and Cremer, 2006) underneath the membrane to accommodate the transmembrane domains of proteins. Therefore, proteins cannot readily be incorporated into the membrane as they may be in contact with the supporting material, resulting in impaired function or denaturation of the protein. Lastly, when a bilayer is directly deposited on a gold surface, any defects or roughness of the gold support can cause defects in the lipid bilayer, reducing its ability to resist current transfer.
If a polymer cushion is used to support the membrane, the properties of the supporting material can be partly reduced. Furthermore, the supporting polymer cushion can be designed such that it is thick enough to prevent contact of incorporated proteins with the supporting surface. However, the morphology, swelling behavior and thickness of the polymer cushion are challenging to control; consequently, the electrical qualities of any adsorbed lipid bilayer are usually not adequate for electrochemical studies. This makes polymer-supported lipid membranes highly suitable for the study of proteins and membrane processes that do not involve charge transport events, such as diffusion and binding studies.
Tethered membranes were developed with the aim to obtain a lipid bilayer system with high electrical sealing properties and high stability that avoids direct contact with the solid support. This can for example be achieved through the use of an anchorlipid, a lipid-like molecule functionalized with a spacer segment that suspends it from the solid support. Alternatively, the membrane can also be tethered to its support through the use of a protein, in a so-called protein-tethered lipid membrane. This review focuses on these types of model membranes, highlighting various tethering methods and showcasing a wide range of different applications for tethered membranes.
In addition to their stability and ease of assembly, tethered membranes are accessible to a large number of different analytical tools. These include AFM, neutron scattering, spectroscopic techniques such as ellipsometry, infrared reflection absorption spectroscopy (IRRAS) and SPR as well as electrochemical methods such as electrochemical impedance spectroscopy (EIS) and current-voltage (CV) studies. Furthermore, many tethered membrane systems can be prepared using only self-assembly, making them very accessible tools that do not require specific training. In addition, they enable the use of model membrane architectures in microfluidic devices, for use as sensors or as screening platforms for the pharmaceutical industry. This review will highlight three different approaches for binding a model membrane to a solid support: tethered membranes, sparsely tethered membranes and protein-tethered membranes.
Tethered Bilayer Lipid Membranes
Tethered bilayer lipid membranes (tBLMs, Figure 1) are comprised of an anchorlipid in the inner leaflet and a phospholipid monolayer in the outer leaflet of the membrane. The inner leaflet is tethered to the solid support via an anchoring group that can be tailored to bind to the substrate that is used. Usually, the substrate of choice is gold due to its stability and versatility for a wide range of analytical methods. However, other substrates such as silicon oxide (Vockenroth et al., 2008c) or mercury (Becucci and Guidelli, 2014; Becucci et al., 2015) and aluminum oxide (Roskamp et al., 2008) have also been used.
In fully tethered tBLMs, the inner leaflet is comprised exclusively of an anchoring lipid. This typically results in membranes with excellent electrical sealing properties that are highly suitable for electrochemical studies. A large number of different chemistries have been developed as anchoring lipids. Table 1 shows a summary of commonly used anchorlipid structures and the respective names of each compound. An anchorlipid cosists typically in a hydrophobic part, a spacer unit and an anchoring group, which binds the lipid so a substrate.
The choice of anchoring group can significantly impact that quality of the resulting tethering layer. For example, changing the anchoring group from a single thiol (DPhyTT) to a disulfide (DPhyTL) while using the same length of the spacer molecule, improved the electrical resistance of the resulting bilayer from up to 15 MΩ·cm2 to 55 MΩ·cm2 (Junghans and Koper, 2010). This is possibly because the size of the anchor group more closely matches that of the hydrophobic chains, resulting in improved packing density as the anchorlipid.
In addition to the packing density and surface roughness, the area of the membrane is also a crucial factor affecting membrane quality. A larger surface area results in a higher absolute number of membrane defects that are present. The highest reported resistance of DPhyTL-based tBLMs is 10 GΩ for a membrane diameter with 10 μm (Vockenroth et al., 2008b). Membrane resistance decreased with increasing diameter of the membrane, with a 4 mm radius resulting in membrane resistances of 30 MΩ·cm2. The capacitance of the membranes decreased from 1 μF/cm2 at a diameter of 4 mm to around 0.5 nF at a diameter of 8 μm.
Molecular dynamics simulations were used to predicte that increasing the length of the spacer will cause the structure of a tBLM to become significantly more disordered, strongly reducing its quality and electrical sealing properties. With increasing tether length, the membrane forms lesions and ultimately becomes detached completely from the solid support (Liu and Faller, 2012). This prediction was also investigated experimentally by doubling the length of the anchorlipid DPhyTT from four ethylene glycol units to eight (DPhyOT), which reduced bilayer resistance by three orders of magnitude from ca. 15 MΩ to around 90 kΩ·cm2 (Junghans and Koper, 2010).
In addition to the structures shown in Table 1, peptides have also been explored as tethering moieties to create so-called pep-tBLMs (Chadli et al., 2017). One of the benefits of using a peptide as tethering and anchor group is that a cysteine residue at the N-terminal naturally binds to gold, avoiding the need to functionalize or modify the desired peptide sequence. The C-terminal of the tethering group was modified with a several histidine residues which allow the binding of vesicles containing a chelating lipid to the tethers in the presence of nickel. Once the vesicles were adsorbed to the tethering groups, a fusogenic peptide (derived from a Hepatitis C protein) was used to cause the fusion of the adsorbed vesicles into a continuous lipid bilayer.
If cell-free expression is utilized to synthesize a membrane protein, lipid vesicles can be used to incorporate the protein (Chadli et al., 2017). These vesicles can subsequently be used for tBLM formation, offering a simple pathway to create protein-functionalized tethered membranes. Using this method, the C-X-C motif chemokine receptor 4 was successfully incorporated into a model membrane (Chadli et al., 2017). Bilayer formation was monitored using SPR and the incorporation of the protein was confirmed using AFM and fluorescence recovery after photobleaching (FRAP). The functionality of the receptor was confirmed by observing the binding of the receptor antagonist to the protein with SPR.
Tethered membranes also offer the possibility of combining cell-free expression of a protein with a model membrane, avoiding the need for lengthy protein purification processes (Zieleniecki et al., 2016). The plant membrane transporter Bot1 was expressed in the presence of a DPhyTL-based tBLM, and spontaneously incorporated in its functional form into the lipid bilayer (Zieleniecki et al., 2016).
Very different tethering chemistries have also been explored, for example by furnishing a cholesterol molecule with an thiolated ethyleneoxy-anchor on a nanostructured surface enabling surface-enhance infrared absorption spectroscopy (SEIRA; Wiebalck et al., 2016). The resulting lipid membranes were of lower quality than other tBLMs due to the increased roughness of the substrate that is necessary for SEIRA spectroscopy. When this type of tBLM architecture was deposited on a planar substrate instead, the resistance of the membrane was increased to around 700 kΩ·cm2. This also confirmed the observation that thiols as anchoring moieties produce inherently lower quality membranes than when a disulfide (for example lipoic acid) is used (Junghans and Koper, 2010).
An inner leaflet comprised exclusively of anchorlipids is detrimental to incorporated proteins and the activity of ion transporters. In part, this is likely a consequence of the limited amount of water incorporated underneath the membrane [around 5 volume-%(Junghans and Koper, 2010)], resulting in a reduced capacity to store ions. The low hydration of the sub-membrane compartment was confirmed by polarization-modulation infrared reflection-absorption spectroscopy (PM-IRRAS) of a DPhyTL-based lipid membrane which showed that the ethylene glycol segment takes on a coiled conformation in which the hydrophobic carbon segments of the ethylene glycol chain are exposed to the surroundings, excluding water from the sub-membrane space (Leitch et al., 2009). The authors were able to distinguish between the C-H stretching of the hydrophobic chains and those located in the ethylene glycol spacer by using a deuterated spacer segment.
The high packing density of fully tethered lipid would likely limit the amount of water in the sub-membrane space even if the hydrophobic conformation of the spacer segment was not a significant factor. Experiments were conducted using a different anchorlipid chemistry (DPhyAL), in which the ethylene glycol spacer was replaced with an ester-based segment that is unable to adopt the same helical conformation as a PEG-based spacer and is also more polar than an ethylene glycol chain (Andersson et al., 2017).
Neutron scattering showed that the change in spacer segment composition did not increase the hydration of the sub-membrane space which remained low at around 5 volume-% (Andersson et al., 2017). This suggests that the packing density of the inner leaflet also plays a significant role in determining the hydration of the sub-membrane compartment.
However, the use of ester linkages instead of ether groups in the spacer segment caused ions to remain in the sub-membrane compartment when the buffer was exchanged. When studies were carried out with the ion transporter valinomycin, which selectively transports K+ ions across lipid bilayers, it was shown that membrane resistance did not immediately increase upon replacement of the KCl bathing solution with NaCl (Andersson, 2013). This suggested that K+ remained in the sub-membrane reservoir that slowly leaked out instead of being quickly replaced with the new electrolyte. To restore the membrane resistance, storage of the bilayer under MilliQ for up to 24 h was required to remove the remaining potassium ions from the membrane. PM-IRRAS measurements could provide more detailed insight into the structure and orientation of the new spacer segments.
Sparsely Tethered Membranes
In order to increase the hydration of the sub-membrane spacer and better facilitate protein incorporation into the lipid bilayer, sparsely tethered bilayer lipid membranes (stBLMs) have been developed that use either a larger anchoring segment or a smaller diluting molecule mixed with the anchoring lipid, resulting in a significantly reduced density of the anchoring moieties. Figure 2 shows a comparison between the different types of tethering architectures.
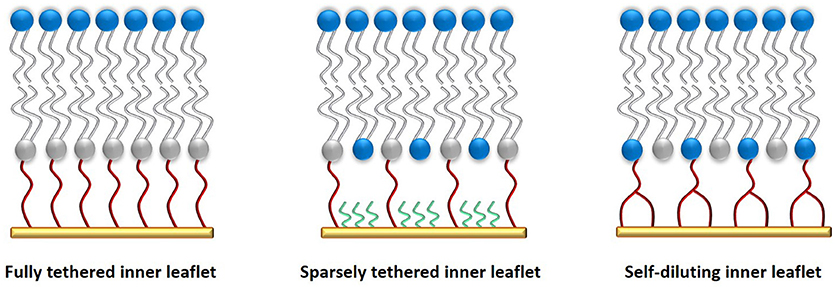
Figure 2. Comparison of various tethered membrane architectures. A fully tethered inner leaflet (left) is comprised of only anchorlipids (gray/red). It offers the highest stability and electrical sealing properties, but limits protein incorporation. A sparsely tethered lipid membrane (middle) is comprised of an anchorlipid mixed with a small molecule (green) that binds competitively with the anchorlipid to the supporting surface, creating a sparsely tethered inner leaflet with greater phospholipid diffusion rates and enhanced functionality of incorporated ion transporters and ion channels. A self-diluting inner leaflet (right) is prepared using an anchorlipid with a bulkier anchoring moiety such that a similar effect to a sparsely tethered inner leaflet is achieved. The synthesis of this type of anchorlipid is more complex, but it affords a more controlled membrane composition while still allowing increased hydration of the sub-membrane compartment.
Using a combination of molecular dynamics calculations and SEIRA measurements, it was possible to observe the process by which the antimicrobial peptide alamethicin absorbs to and then integrates into a lipid bilayer (Forbrig et al., 2018). Alamethicin acts by assembling into pores comprised of 4–6 individual peptide strands. Two membrane architectures—one sparsely tethered and one fully tethered system—were used for this study. SEIRA enables the direct observation of the orientation of the alamethicin during its interaction with the lipid membrane. In a fully tethered membrane system, alamethicin was not able to penetrate the bilayer. However, in the sparsely tethered system the peptide first adsorbed to the membrane surface, followed by aggregation of individual peptides, and finally the agglomeratedcpeptide inserted into the bilayer to form pores. This highlights the importance of selecting the correct membrane architecture for a study.
Reducing the tethering density of the inner leaflet to create an stBLM results in significantly increased sub-membrane hydration (Junghans and Koper, 2010; Andersson et al., 2017). However, when using a mixture of anchorlipid and a spacer molecule such as mercaptoethanol, the sealing properties of the resulting lipid membrane are reduced. When a modified anchoring segment was used instead of a mixture of anchorlipid and spacer, for example in the case of DPhySDL (Andersson, 2013) or DPhyHDL (Junghans and Koper, 2010), the sealing properties of the membrane remained high, while the sub-membrane hydration was also increased. When a mixture of anchorlipid and a spacer such as mercaptoethanol was used, membrane hydration was also improved but the electrical resistance of the membranes decreased by an order or magnitude (Andersson, 2013). Furthermore, the exact composition of the resulting inner leaflet was no longer known, as it depends on a large number of factors such as the relative solubilities or the compound in the chosen solvent and their binding kinetics. The use of a modified anchoring segment is therefore a useful strategy when designing sparsely tethered lipid bilayer because in addition to retaining a higher quality lipid bilayer, the exact composition of the membrane is known.
Ion transporters and ion channels are also incorporated more effectively and possibly exhibit improved function when they are incorporated into an stBLM. The functionality of the pore-forming toxin alpha-hemolysin was compared when incorporated into a tBLM with a proximal leaflet comprised only of DPhyTL and a proximal leaflet comprised of DPhyHDL, demonstrating that either the protein had significantly improved function in the sparsely tethered architecture, or more proteins were incorporated into the membrane (Vockenroth et al., 2008a). Any ions transported into the sub-membrane space still require stabilization by a hydration shell; therefore, improved sub-membrane hydration should increase ion transport through the lipid bilayer.
DPhyTL forms a densely packed inner membrane leaflet whereas DPhyHDL is designed to provide more space underneath the lipid membrane by using both a longer anchoring segment and a larger anchoring group. DPhyTL forms a more sealing lipid bilayer with a resistance of up to 55 MΩ cm2, but the resistance of the membrane was only reduced by around one order of magnitude upon incorporation of α-hemolysin. A DPhyHDL-based stBLM has a much lower resistance of 1 MΩ cm2 but allows significantly improved ion transport, with α-hemolysin reducing membrane resistance by three orders of magnitude to 1 kΩ cm2 in the presence of KCl. Neutron scattering could be used to ascertain whether the amount of proteins incorporated into the bilayer is affected by the choice of membrane architecture, or the increased sub-membrane hydration improves their function.
In an stBLM comprised of DPhySL or DPhyTL mixed with 40–60% mercaptoethanol as a spacer, membrane resistance was reduced by up to four orders of magnitude when valinomycin was incorporated in the presence of KCl, compared to a reduction of only one or two orders of magnitude in fully tethered membrane systems (Andersson et al., 2017). While the dilution of the inner leaflet with mercaptoethanol reduced the membrane resistance from 5–10 MΩ cm2 to around 1–2 MΩ cm2, this was not the case when DPhySL was diluted with mercaptoethanol, possibly because this spacer chemistry allows for better packing density when diluted than the other spacer architectures.
Neutron scattering showed that using either the self-diluting lipids DPhySDL or DPhyHDL or inner leaflets diluted with mercaptoethanol increases the sub-membrane hydration from 5% to around 25%. Using partially deuterated phospholipids it was demonstrated that when the proximal leaflet is diluted, phospholipids also incorporate into the inner leaflet of the membrane (Andersson et al., 2018).
Another study used a membrane architecture comprised of a mixture of 30% WC14 (see Table 1) and 70% mercaptoethanol to incorporate α-hemolysin (McGillivray et al., 2009). The resulting membrane had a resistance of 100 kΩ cm2 which was reduced by two orders of magnitude to around 2 kΩ cm2 upon incorporation of α-hemolysin in the presence of KCl. The same membrane architecture was also used to show that neutron reflectometry is a viable means of obtaining low-resolution structural information of proteins incorporated into tethered lipid bilayer membranes. These membranes were shown to have very high sub-membrane hydration levels of up to 60% (McGillivray et al., 2007).
A sparsely tethered membrane was also prepared using a mixture of the anchorlipid CPEO3 (see Table 1) and mercaptohexanol (Jeuken et al., 2006). The protein-functionalized lipid bilayer was formed by adding proteoliposomes containing 0.5 mas-% E. coli cytochrome bo3, a ubiquinol oxidase. In the presence of Ca2+, the liposomes fuse into a tBLM with a resistance of 500 kΩ cm2. The density and distribution of the incorporated proteins was investigated via AFM, showing a protein density of 3–8.5 fmol/cm2. Cyclic voltammetry of the cbo3-functionalized membrane in the presence of ubiquinol-8 showed that virtually all proteins that were seen in the AFM studies were active.
Surface-enhanced infrared absorption spectroscopy (SEIRAS) studies have been used to study the water structure at the interface between solid-supported lipid membranes comprised of the phospholipid 1,2-dimyristoyl-sn-glycero-3-phosphocholine (DMPC) and the supporting surface (Uchida et al., 2014). While this study was not carried out on membranes tethered to the solid support, it nevertheless had some interesting implications for supported lipid membranes. The authors found several types of environments for water molecules in bilayers on solid supports. At electrical potentials close to zero, water was bound tightly to the phospholipid head groups. When the potential was increased, aggregates of water molecules began to penetrate into the lipid membrane. At applied potentials lower than −700 mV, resulting in charge densities below −20 μC·cm−2, a layer of liquid water with a thickness of around 1 nm formed below the lipid membrane.
As SEIRAS can distinguish between hydrogen-bonded and unbonded water molecules, it would be an ideal technique to study the sub-membrane reservoir of various tBLM architectures to gain an understanding of the distribution of water below the membrane and the fluidity of the aqueous environment between the membrane and its support. This could provide important insight into which architectures would be most suited for protein incorporation studies, particularly if the diffusion of membrane components is a factor in their functionality.
The EO3-cholesterol based tether shown in Table 1 has also been mixed with mercaptohexanol to create a sparsely tethered lipid bilayer membrane (Kendall et al., 2010). This membrane had a good electrical resistance of around 1 MΩ cm2 which was reduced by up to four orders of magnitude upon incorporation of valinomycin and other ion transporters.
Much longer tethers are also available, for example a lipid bilayer was prepared using PEG-3400-modified 1,2-distearoyl-sn-glycero-3-phosphoethanolamine functionalized with succinimidyl propionate such that it could bind covalently to a gold surface furnished with amine groups. By doping phospholipid vesicles with 5% of this anchorlipid, a bilayer was created which was used to study the translocation of adenylate cyclase toxin (CyaA), a toxin produced by Bordetella pertussis (the causative agent for whooping cough) across a lipid membrane, primarily using SPR (Veneziano et al., 2013).
CyaA is unique in that it is able to facilitate and catalyze its own transition across the cell membrane without requiring other cellular machinery from the host. The electrical properties of this type of tethered membrane have not been investigated, and it is highly likely that they are insufficient to enable meaningful electrical characterization of the system given the reduced quality of tBLMs with a small fraction of the PEG units present in this system. CyaA binds strongly to calmodulin (CAM), which was also immobilized under the tBLM. If CyaA successfully crossed the membrane, its catalytic domain will bind to the immobilized CAM, and should remain bound to the substrate after removal of the tBLM with a detergent. CyaA required both the presence of Ca2+ and a negative membrane potential to be able to cross the lipid membrane. In the absence of either calcium or a negative potential, no translocation of the toxin across the membrane took place.
As many toxins must first cross the cellular membrane, biomimetic membranes can provide unparalleled insight into their mechanisms of binding and translocation across the membrane. Furthermore, the model systems can be used to explore methods of preventing or slowing the translocation of these toxins across the membrane, aiding the development of novel treatment strategies for diseases caused by pathogens such as Bordetella pertussis.
Sparsely tethered membranes can be combined with mesoporous substrates to increase control over membrane architecture, composition and hydration (Wallin et al., 2015). By using vesicles comprised of 1-palmitoyl-2-oleoyl-sn-glycero-3-phosphocholine (POPC) and a small amount of Distearoylphosphatidylethanolamine (DPSE) furnished with a long-chain poly(ethylene) glycol (PEG-2000) tether and an N-hydroxysuccinimide anchor, a lipid bilayer was formed on mesoporous silica functionalized with amines. Quartz crystal microbalance showed that vesicles bound the substrate via the tethers but remained intact rather than fusing into a continuous lipid bilayer. The adsorbed vesicles were resistant to the application of both osmotic pressure and shear force. Vesicle rupture and fusion into a lipid bilayer was achieved via the addition of a fusogenic peptide, which is an α-helical (AH) peptide with the sequence H-Ser-Gly-Ser-Trp-Leu-Arg-Asp-Val-Trp-Asp-Trp-Ile-Cys-Thr-Val-Leu-Thr-Asp-Phe-Lys-Thr-Trp-Leu-Gln-Ser-Lys-Leu-NH2 that has been reported to promote vesicle rupture (Coutable et al., 2014). AFM showed that the resulting tBLMs had roughnesses between 1 and 3 nm on the mesoporous substrate.
Protein-Tethered Membranes
Tethering the inner leaflet of the membrane to the solid support, even when diluting the anchorlipid, could still hinder the incorporation of proteins into the membrane. By using proteins modified with a His-tag [for example cytochrome C oxidase (Ataka et al., 2004; Giess et al., 2004)], the protein of interest itself can also be used to anchor a lipid bilayer to the solid support, also allowing direct control over the orientation of the protein in the membrane. A schematic of a protein-tethered lipid bilayer membrane is shown in Figure 3.
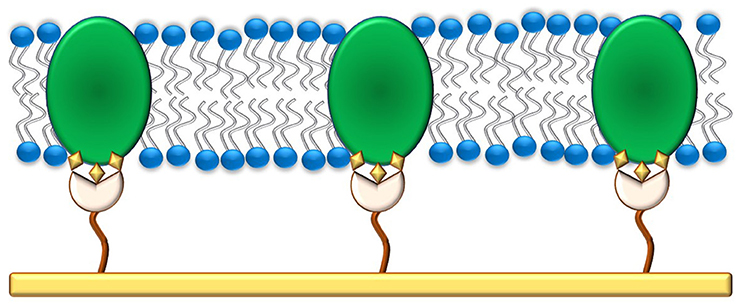
Figure 3. A protein-tethered lipid membrane. It is prepared by first functionalizing the gold surface with a SAM containing nitriloacetic acid groups that can bind to His-tags present in proteins. In the second step, detergent-solubilized protein is added to the substrate which binds to the SAM. The detergent is finally removed via in-situ dialysis or through the use of Bio-beads and replaced with phospholipids to form a lipid bilayer around the adsorbed proteins.
Binding of the protein was facilitated by first modifying the surface with a self-assembled monolayer (SAM) of amino-nitriloacetic acid (NTA) which was then coupled with N-hydroxysuccinimide ester. This surface was then able to complex with Cu2+, to which a His-tagged protein could bind reversibly. Due to their hydrophobic domains, membrane proteins are first solubilized with a detergent which is subsequently replaced with a lipid (such as DPhyPC) via in-situ dialysis. Instead of dialysis, biobeads can also be used to remove the detergent and replace it with a lipid bilayer.
The resulting protein-tethered lipid bilayer membrane had reasonably good electrical sealing properties, with resistances of around 800 kΩ cm2, which while lower than the resistance of some tBLM systems is still indicative of a reasonably good lipid membrane. The resistance was likely reduced by the presence of a significant amount of redox-active protein. Addition of cytochrome c to the tethered membrane reduced membrane resistance by one order of magnitude, showing that the activity of the tethered cytochrome c oxidase protein was not impaired. Subsequent reports showed improved membrane resistance exceeding 10 MΩ cm2 for this architecture (Friedrich et al., 2008); this method is therefore also suitable for biosensing applications. In theory, this methodology can be applied to all membrane proteins containing a His-tag. However, care should be taken that the location of the His-tag does not force an unfavorable orientation of the bound protein.
Another approach to tethering lipid bilayers on a solid support is the use of biotin-streptavidin interactions. By using biotin-functionalized bovine serum albumin (BSA), a monolayer of biotin could be readily assembled on a gold substrate (Taylor et al., 2009). A lipid bilayer was then formed by adding vesicles containing biotinylated phospholipids. Once adsorbed to the support, bilayer formation was caused by using a fusogenic peptide or PEG-8000. The tethering density was controlled by mixing biotin-BSA with native BSA and proteins were inserted into the membrane by incorporating them into the vesicles used for bilayer formation. The biotin-BSA tethered lipid bilayer membrane could be regenerated, as the tBLM could be removed by simply rinsing the bilayer with a surfactant such as Triton X. This is a significant advantage compared to other tethering systems for the use in microfluidic devices, allowing the development of multi-use devices.
AFM studies of membrane proteins in a native conformation can provide valuable insight into their structure and function, but they are challenging to carry out. Using an architecture comprised of tris-NTA (to which the protein can bind trough a His-tag) and anchorlipid bound to the solid support via a PEG-chain, an environment was created that enabled the study of bacteriorhodopsin as a model for membrane proteins (Bronder et al., 2016).
Conclusion
Biomimetic membranes that are covalently tethered to a solid support provide significant advantages, among them increased stability, ease of assembly and a wide variety of applicable tools. Depending upon the intended application, the bilayer can be optimized to maximize its electrical sealing properties by using a fully tethered inner leaflet, to study ion transporters by using sparsely tethered membranes or for the incorporation of functional proteins by anchoring the membrane to its support using the embedded proteins. A fully tethered inner leaflet is the most accessible means of assembling a highly-sealing lipid membrane, whereas sparsely tethered membranes require somewhat more complex assembly procedures, but afford excellent compromises between membrane fluidity, stability and protein function.
Future work with lipid membranes will likely focus further on the functional incorporation of membrane proteins. As the cellular membrane is a crucial barrier and portal between the cell and its surroundings, many biotechnological applications, particularly in biosensing, could result from the successful incorporation of functional membrane proteins into model membranes. The amphiphilic structure of lipid bilayers has also been successfully replicated using block copolymers (Zhang et al., 2012) comprised of linked hydrophobic and hydrophilic domains. Firstly, these polymers provide a robust membrane-like structure that could be designed to resist environments that would damage or destroy lipid bilayers (for example exposure to gases or vacuum). In addition, block copolymers can be designed to assume highly complex shapes and geometries not available to lipid bilayers (Amado and Kressler, 2011). This could also open the door to radically new biotechnological applications for membrane proteins.
Author Contributions
JA: writing of article; IK: editorial input and revision; WK: editorial input and revision.
Conflict of Interest Statement
The authors declare that the research was conducted in the absence of any commercial or financial relationships that could be construed as a potential conflict of interest.
The handling editor declared a past co-authorship with one of the authors WK.
References
Amado, E., and Kressler, J. (2011). Interactions of amphiphilic block copolymers with lipid model membranes. Curr. Opin. Colloid Interface Sci. 16, 491–498. doi: 10.1016/j.cocis.2011.07.003
Andersson, J. (2013). New Tether Structures of Tethered Bilayer Lipid Membranes. Bachelor of Science (Honours), Flinders University.
Andersson, J., Fuller, M. A., Wood, K., Holt, S. A., and Köper, I. (2018). A tethered bilayer lipid membrane that mimics microbial membranes. Phys. Chem. Chem. Phys. 20, 12958–12969. doi: 10.1039/C8CP01346B
Andersson, J., Knobloch, J. J., Perkins, M. V., Holt, S. A., and Köper, I. (2017). Synthesis and characterization of novel anchorlipids for tethered bilayer lipid membranes. Langmuir 33, 4444–4451. doi: 10.1021/acs.langmuir.7b00778
Andersson, J., and Köper, I. (2016). Tethered and polymer supported bilayer lipid membranes: structure and function. Membranes 6:30. doi: 10.3390/membranes6020030
Ataka, K., Giess, F., Knoll, W., Naumann, R., Haber-Pohlmeier, S., Richter, B., et al. (2004). Oriented attachment and membrane reconstitution of His-tagged cytochrome c oxidase to a gold electrode: in situ monitoring by surface-enhanced infrared absorption spectroscopy. J. Am. Chem. Soc. 126, 16199–16206. doi: 10.1021/ja045951h
Atanasov, V., Atanasova, P. P., Vockenroth, I. K., Knorr, N., and Koper, I. (2006). A molecular toolkit for highly insulating tethered bilayer lipid membranes on various substrates. Bioconjug. Chem. 17, 631–637. doi: 10.1021/bc050328n
Basit, H., Van der Heyden, A., Gondran, C., Nysten, B., Dumy, P., and Labbe, P. (2011). Tethered bilayer lipid membranes on mixed self-assembled monolayers of a novel anchoring thiol: impact of the anchoring thiol density on bilayer formation. Langmuir 27, 14317–14328. doi: 10.1021/la202847r
Becucci, L., Faragher, R. J., and Schwan, A. (2015). The effect of the hydrophilic spacer length on the functionality of a mercury-supported tethered bilayer lipid membrane. Bioelectrochemistry 101, 92–96. doi: 10.1016/j.bioelechem.2014.08.012
Becucci, L., and Guidelli, R. (2014). Mercury-supported biomimetic membranes for the investigation of antimicrobial peptides. Pharmaceuticals 7, 136–168. doi: 10.3390/ph7020136
Boden, N., Bushby, R. J., Clarkson, S., Evans, S. D., Knowles, P. F., and Marsh, A. (1997). The design and synthesis of simple molecular tethers for binding biomembranes to a gold surface. Tetrahedron 53, 10939–10952. doi: 10.1016/S0040-4020(97)00698-4
Bronder, A. M., Bieker, A., Elter, S., Etzkorn, M., Häussinger, D., and Oesterhelt, F. (2016). Oriented membrane protein reconstitution into tethered lipid membranes for AFM force spectroscopy. Biophys. J. 111, 1925–1934. doi: 10.1016/j.bpj.2016.08.051
Budvytyte, R., Valincius, G., Niaura, G., Voiciuk, V., Mickevicius, M., Chapman, H., et al. (2013). Structure and properties of tethered bilayer lipid membranes with unsaturated anchor molecules. Langmuir 29, 8645–8656. doi: 10.1021/la401132c
Castellana, E. T., and Cremer, P. S. (2006). Solid supported lipid bilayers: from biophysical studies to sensor design. Surf. Sci. Rep. 61, 429–444. doi: 10.1016/j.surfrep.2006.06.001
Chadli, M., Rebaud, S., Maniti, O., Tillier, B., Cortès, S., and Girard-Egrot, A. (2017). New tethered phospholipid bilayers integrating functional G-protein-coupled receptor membrane proteins. Langmuir 33, 10385–10401. doi: 10.1021/acs.langmuir.7b01636
Clifton, L. A., Holt, S. A., Hughes, A. V., Daulton, E. L., Arunmanee, W., Heinrich, F., et al. (2015). An accurate in vitro model of the E. coli envelope. Angew. Chem. Int. Ed. Engl. 54, 11952–11955. doi: 10.1002/anie.201504287
Cornell, B., Braach-Maksvytis, V., King, L., Osman, P., Raguse, B., Wieczorek, L., et al. (1997). A biosensor that uses ion-channel switches. Nature 387, 580–582. doi: 10.1038/42432
Coutable, A., Thibault, C., Chalmeau, J., François, J. M., Vieu, C., Noireaux, V., et al. (2014). Preparation of tethered-lipid bilayers on gold surfaces for the incorporation of integral membrane proteins synthesized by cell-free expression. Langmuir 30, 3132–3141. doi: 10.1021/la5004758
Datta, S. A., Heinrich, F., Raghunandan, S., Krueger, S., Curtis, J. E., Rein, A., et al. (2011). HIV-1 Gag extension: conformational changes require simultaneous interaction with membrane and nucleic acid. J. Mol. Biol. 406, 205–214. doi: 10.1016/j.jmb.2010.11.051
Forbrig, E., Staffa, J. K., Salewski, J., Mroginski, M. A., Hildebrandt, P., and Kozuch, J. (2018). Monitoring the orientational changes of alamethicin during incorporation into bilayer lipid membranes. Langmuir 34, 2373–2385. doi: 10.1021/acs.langmuir.7b04265
Friedrich, M. G., Plum, M. A., Santonicola, M. G., Kirste, V. U., Knoll, W., Ludwig, B., et al. (2008). In situ monitoring of the catalytic activity of cytochrome c oxidase in a biomimetic architecture. Biophys. J. 95, 1500–1510. doi: 10.1529/biophysj.107.122747
Giess, F., Friedrich, M. G., Heberle, J., Naumann, R. L., and Knoll, W. (2004). The protein-tethered lipid bilayer: a novel mimic of the biological membrane. Biophys. J. 87, 3213–3220. doi: 10.1529/biophysj.104.046169
Groves, J. T., and Kuriyan, J. (2010). Molecular mechanisms in signal transduction at the membrane. Nat. Struct. Mol. Biol. 17, 659–665. doi: 10.1038/nsmb.1844
Heinrich, F., Ng, T., Vanderah, D. J., Shekhar, P., Mihailescu, M., Nanda, H., et al. (2009). A new lipid anchor for sparsely tethered bilayer lipid membranes. Langmuir 25, 4219–4229. doi: 10.1021/la8033275
Jackman, J. A., Knoll, W., and Cho, N. J. (2012). Biotechnology applications of tethered lipid bilayer membranes. Materials 5, 2637–2657. doi: 10.3390/ma5122637
Jeon, T.-J., Malmstadt, N., Poulos, J. L., and Schmidt, J. J. (2008). Black lipid membranes stabilized through substrate conjugation to a hydrogel. Biointerphases 3, FA96–FA100. doi: 10.1116/1.2948314
Jeuken, L. J. C., Connell, S. D., Henderson, P. J. F., Gennis, R. B., Evans, S. D., and Bushby, R., J. (2006). Redox enzymes in tethered membranes. J. Am. Chem. Soc. 128, 1711–1716. doi: 10.1021/ja056972u
Junghans, A., and Koper, I. (2010). Structural analysis of tethered bilayer lipid membranes. Langmuir 26, 11035–11040. doi: 10.1021/la100342k
Kendall, J. K., Johnson, B. R., Symonds, P. H., Imperato, G., Bushby, R. J., and Gwyer, J. D. (2010). Effect of the structure of cholesterol-based tethered bilayer lipid membranes on ionophore activity. ChemPhysChem. 11, 2191–2198. doi: 10.1002/cphc.200900917
Kibrom, A., Roskamp, R. F., Jonas, U., Menges, B., Knoll, W., Paulsen, H., et al. (2011). Hydrogel-supported protein-tethered bilayer lipid membranes: a new approach toward polymer-supported lipid membranes. Soft Matter 7, 237–246. doi: 10.1039/C0SM00618A
Knobloch, J. J., Nelson, A. R. J., Köper, I., James, M., and McGillivray, D. J. (2015). Oxidative damage to biomimetic membrane systems: in situ Fe(II)/ascorbate initiated oxidation and incorporation of synthetic oxidized phospholipids. Langmuir 31, 12679–12687. doi: 10.1021/acs.langmuir.5b02458
Leitch, J., Kunze, J., Goddard, J. D., Schwan, A. L., Faragher, R. J., Naumann, R., et al. (2009). In situ PM-IRRAS studies of an archaea analogue thiolipid assembled on a Au(111) electrode surface. Langmuir 25, 10354–10363. doi: 10.1021/la900907d
Lingwood, D., and Simons, K. (2010). Lipid rafts as a membrane-organizing principle. Science 327, 46–50. doi: 10.1126/science.1174621
Liu, C., and Faller, R. (2012). Conformational, dynamical, and tensional study of tethered bilayer lipid membranes in coarse-grained molecular simulations. Langmuir 28, 15907–15915. doi: 10.1021/la303511p
McGillivray, D. J., Valincius, G., Heinrich, F., Robertson, J. W., Vanderah, D. J., Febo-Ayala, W., et al. (2009). Structure of functional Staphylococcus aureus α-hemolysin channels in tethered bilayer lipid membranes. Biophys. J. 96, 1547–1553. doi: 10.1016/j.bpj.2008.11.020
McGillivray, D. J., Valincius, G., Vanderah, D. J., Febo-Ayala, W., Woodward, J. T., Heinrich, F., et al. (2007). Molecular-scale structural and functional characterization of sparsely tethered bilayer lipid membranes. Biointerphases 2, 21–33. doi: 10.1116/1.2709308
Moulick, R. G., Panaitov, G., Choi, S.-E., Mayer, D., and Offenhäusser, A. (2018).Patterning artificial lipid bilayer on nanostructured surfaces. Int. J. Nanomedicine 13, 55–58. doi: 10.2147/IJN.S125005
Römer, W., and Steinem, C. (2004). Impedance analysis and single-channel recordings on nano-black lipid membranes based on porous alumina. Biophys. J. 86, 955–965. doi: 10.1016/S0006-3495(04)74171-5
Roskamp, R. F., Vockenroth, I. K., Eisenmenger, N., Braunagel, J., and Koeper, I. (2008). Functional tethered bilayer lipid membranes on aluminum oxide. Chemphyschem. 9, 1920–1924. doi: 10.1002/cphc.200800248
Sackmann, E. (1996). Supported membranes: scientific and practical applications. Science 271, 43–48. doi: 10.1126/science.271.5245.43
Schiller, S. M., Naumann, R., Lovejoy, K., Kunz, H., and Knoll, W. (2003). Archaea analogue thiolipids for tethered bilayer lipid membranes on ultrasmooth gold surfaces. Angew. Chem. Int, Ed. 42, 208–211. doi: 10.1002/anie.200390080
Taylor, J. D., Linman, M. J., Wilkop, T., and Cheng, Q. (2009). Regenerable tethered bilayer lipid membrane arrays for multiplexed label-free analysis of lipid-protein interactions on poly(dimethylsiloxane) microchips using spr imaging. Anal. Chem. 81, 1146–1153. doi: 10.1021/ac8023137
Uchida, T., Osawa, M., and Lipkowski, J. (2014). SEIRAS studies of water structure at the gold electrode surface in the presence of supported lipid bilayer. J. Electroanal. Chem. 716, 112–119. doi: 10.1016/j.jelechem.2013.10.015
Valincius, G., Heinrich, F., Budvytyte, R., Vanderah, D. J., McGillivray, D. J., Sokolov, Y., et al. (2008). Soluble amyloid beta-oligomers affect dielectric membrane properties by bilayer insertion and domain formation: implications for cell toxicity. Biophys. J. 95, 4845–4861. doi: 10.1529/biophysj.108.130997
van Meer, G., Voelker, D. R., and Feigenson, G. W. (2008). Membrane lipids: where they are and how they behave. Nat. Rev. Mol. Cell Biol. 9, 112–124. doi: 10.1038/nrm2330
Veneziano, R., Rossi, C., Chenal, A., Devoisselle, J.-M., Ladant, D., and Chopineau, J. (2013). Bordetella pertussis adenylate cyclase toxin translocation across a tethered lipid bilayer. Proc. Nat. Acad. Sci. 110, 20473–20478. doi: 10.1073/pnas.1312975110
Vockenroth, I. K., Atanasova, P. P., Jenkins, A. T., and Koper, I. (2008a). Incorporation of alpha-hemolysin in different tethered bilayer lipid membrane architectures. Langmuir 24, 496–502. doi: 10.1021/la7030279
Vockenroth, I. K., Atanasova, P. P., Long, J. R., Jenkins, A. T., Knoll, W., and Koper, I. (2007). Functional incorporation of the pore forming segment of AChR M2 into tethered bilayer lipid membranes. Biochim. Biophys. Acta 1768, 1114–1120. doi: 10.1016/j.bbamem.2007.02.006
Vockenroth, I. K., Fine, D., Dodobalapur, A., Jenkins, A. T., and Köper, I. (2008b). Tethered bilayer lipid membranes with giga-ohm resistances. Electrochem. Commun. 10, 323–328. doi: 10.1016/j.elecom.2007.12.018
Vockenroth, I. K., Ohm, C., Robertson, J. W., McGillivray, D. J., Lösche, M., and Köper, I. (2008c). Stable insulating tethered bilayer lipid membranes. Biointerphases 3, FA68–FA73. doi: 10.1116/1.2912097
Wallin, M., Choi, J.-H., Kim, S. O., Cho, N.-J., and Andersson, M. (2015). Peptide-induced formation of a tethered lipid bilayer membrane on mesoporous silica. Eur. Biophys. J. 44, 27–36. doi: 10.1007/s00249-014-0998-1
White, S. H. (2004). The progress of membrane protein structure determination. Protein Sci. 13, 1948–1949. doi: 10.1110/ps.04712004
Wiebalck, S., Kozuch, J., Forbrig, E., Tzschucke, C. C., Jeuken, L. J. C., and Hildebrandt, P. (2016). Monitoring the transmembrane proton gradient generated by cytochrome bo3 in tethered bilayer lipid membranes using SEIRA spectroscopy. J. Phys. Chem. B 120, 2249–2256. doi: 10.1021/acs.jpcb.6b01435
Wiltschi, B., Knoll, W., and Sinner, E.-K. (2006). Binding assays with artificial tethered membranes using surface plasmon resonance. Methods 39, 134–146. doi: 10.1016/j.ymeth.2006.05.007
Winterhalter, M. (2000). Black lipid membranes. Curr. Opin. Colloid Interface Sci. 5, 250–255. doi: 10.1016/S1359-0294(00)00063-7
Zhang, X., Tanner, P., Graff, A., Palivan, C. G., and Meier, W. (2012). Mimicking the cell membrane with block copolymer membranes. J. Polym. Sci. A Polym. Chem. 50, 2293–2318. doi: 10.1002/pola.26000
Keywords: model membranes, tethered membranes, lipid bilayer, solid-supported membranes, membrane biophysics, protein-tethered membrane
Citation: Andersson J, Köper I and Knoll W (2018) Tethered Membrane Architectures—Design and Applications. Front. Mater. 5:55. doi: 10.3389/fmats.2018.00055
Received: 25 July 2018; Accepted: 20 August 2018;
Published: 07 September 2018.
Edited by:
Nam-Joon Cho, Nanyang Technological University, SingaporeReviewed by:
Tomohiro Hayashi, Tokyo Institute of Technology, JapanMarkus Gallei, Technische Universität Darmstadt, Germany
Copyright © 2018 Andersson, Köper and Knoll. This is an open-access article distributed under the terms of the Creative Commons Attribution License (CC BY). The use, distribution or reproduction in other forums is permitted, provided the original author(s) and the copyright owner(s) are credited and that the original publication in this journal is cited, in accordance with accepted academic practice. No use, distribution or reproduction is permitted which does not comply with these terms.
*Correspondence: Jakob Andersson, amFrb2IuYW5kZXJzc29uQGFpdC5hYy5hdA==