- 1Department of Biomedical Engineering, TOBB University of Economics and Technology, Ankara, Turkey
- 2Department of Biomedical Engineering, Ankara University, Ankara, Turkey
Tissue engineering aims to bring together biomaterials, cells, and signaling molecules within properly designed microenvironments in order to create viable treatment options for the lost or malfunctioning tissues. Design and production of scaffolds and cell-laden grafts that mimic the complex structural and functional features of tissues are among the most important elements of tissue engineering strategy. Although all tissues have their own complex structure, an even more complex case in terms of engineering a proper carrier material is encountered at the tissue interfaces, where two distinct tissues come together. The interfaces in the body can be examined in four categories; cartilage-bone and ligament-bone interfaces at the knee and the spine, tendon-bone interfaces at the shoulder and the feet, and muscle-tendon interface at the skeletal system. These interfaces are seen mainly at the soft-to-hard tissue transitions and they are especially susceptible to injury and tear due to the biomechanical inconsistency between these tissues where high strain fields are present. Therefore, engineering the musculoskeletal tissue interfaces remain a challenge. This review focuses on recent advancements in strategies for musculoskeletal interface engineering using different biomaterial-based platforms and surface modification techniques.
Introduction
According to Langer and Vacanti, “Tissue engineering is an interdiciplinary field that applies the principles of engineering and life sciences toward the development of biologic substitutes that restore, maintain, or improve tissue or organ failure” (Langer and Vacanti, 1993). The basic principle of tissue engineering is the isolation and multiplication of cells followed by the reimplantation of the cell/biomaterial complex designed and produced to meet the requirements of the defect site (Langer and Vacanti, 2016). Tissue engineering aims to mimic the structure, function and composition of the native tissues in the best possible way. In order to achieve this goal, several approaches have been employed including scaffold-based and scaffold-free strategies for engineering of tissues including; bone, cartilage, tendon, ligaments, nervous tissue, and orthopedic interfaces (Freed et al., 1998; Moffat et al., 2009; Yilgor et al., 2010; Erisken et al., 2011; Chen et al., 2015). In these, either cells are directly injected to the damage site, or biomaterials are implanted without cellular content, or cells and biomaterials are combined for in vitro or in vivo applications. Although the strategy appears to be relatively straightforward, there are many challenges associated with each of these approaches. Table 1 summarizes the challenges that are encountered in tissue engineering field (Rice et al., 2005).
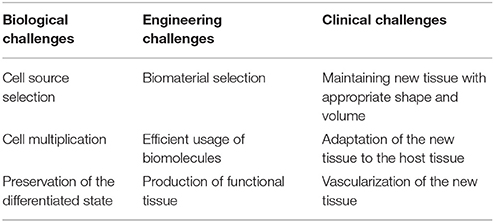
Table 1. Tissue engineering challenges according to in biological, engineering and clinical concerns (Rice et al., 2005).
Biomaterials play a central role in tissue engineering strategy. The definition of the ideal biomaterial has changed considerably during the past decades (Williams, 2009). In early biomaterial design, the aim was to match the mechanical and structural properties of tissues without causing damage or unwanted host response. First generation biomaterials, such as poly(methyl methacrylate) (PMMA) and stainless steel, were used extensively as they were considered to be relatively inert and incited a predictable foreign body response. Second generation biomaterials included metals, ceramics, synthetic, and natural polymers such as titanium, bioglass, poly(lactide-co-glycolide) (PLGA), and collagen. These materials were engineered to be more biologic, having bioactive properties that include osteointegration [titanium, nano-hydroxyapatite (n-HAP)] (Carlsson et al., 1986), tissue integration (Bioglass) (Krishnan and Lakshmi, 2013), and biodegradation (PLGA, collagen) (Glowacki and Mizuno, 2008; Pan and Ding, 2012; Martinez et al., 2015; Mironov et al., 2017). Third generation biomaterials are then employed to provoke molecularly engineered host response. Many different approaches were used in order to regenerate different types of tissues using these biomaterials. Among these, tissue-to-tissue interfaces remain the most challenging cases due to their gradient and complex structural and mechanical requirements. This review will focus on strategies to engineer orthopedic tissue interfaces such as muscle-tendon, tendon/ligament-bone, and cartilage-bone with special emphasis on the use of biomaterials in these approaches.
Muscle-Tendon Interface (Myotendinous Junction)
The myotendinous junction (MTJ) is the meeting point between tendons and muscles and its structure resembles adhesive joints (Woo, 1987; Trotter, 1993). When the functions of the muscle-tendon unit are examined, it is observed that MTJ controls the movements and positions of the joints. MTJ works as an antagonist, it stabilizes the joint, stores, and releases the strain energy (Alexander, 1988). The MTJ carries out vital physiological functions within the muscle-tendon unit. Muscle force is transmitted to the tendon through the MTJ, by force transfer between intracellular muscle proteins and extracellular tendon proteins (Kannus et al., 1992; Hwang et al., 2005). Microscopically, MTJ is not a sharp transition between tissues. It is composed of overlapped fragments of both muscle and tendon and an extensive adhesion surface in between. At the connection point, muscle fibers form structures which integrate with the extracellular matrix (ECM) elements of the tendon. The size and the morphology of these structures vary by gender, age, body mass index (BMI), etc (Kvist et al., 1991; Curzi et al., 2013).
When the ECM of the MTJ is examined, it is observed that several adhesion proteins such as laminin, paxillin, integrin, vinculin, fibronectin, and talin are present in addition to the ECM protein content of both tissues (muscle and tendon). These adhesion proteins enable a link between muscle actin filaments and tendoneus collagen fibers (Shear and Bloch, 1985; Bozyczko et al., 1989; Swasdison and Maynei, 1989; Turner, 2000; Conti et al., 2009). Moreover, the adhesion proteins preserve the integrity and aid the transition of the muscle fibers into tendon fibrils. Therefore, it is important to note that any successful approach to engineer MTJ must consider the need for the adhesion complexes inherent to the junction site.
The structural and mechanical differences between muscle and tendon units require the design of a unique interface for two reasons: first, the mechanism which enables muscle contraction is intracellular, while the collagen matrix that gives the tendon its strength is extracellular. In order to transmit the force from the muscle to the tendon, and finally to the bone, the complex tissue requires transmembrane connections which is a challenge for tissue engineering field. Another reason is that the interface is prone to injury due to the mechanical inhomogeneity. Therefore, the inherent structural and functional characteristics of both tissues have to be considered in order to be able to mimic the native tissue properly.
As mentioned before, one of the main functions of the MTJ is to release and store the energy. Therefore, MTJ injuries often occur due to repeated overload, high impact training or overuse (Lysholm and Wiklander, 1987). The most common injuries which occur at the MTJ is seen in the rotator cuff, Achilles tendon, and hamstrings. Current approach for these injuries are suture repairs but these treatments have a high retear rate and high risk of fibrotic scar tissue formation at the wound site (Galatz et al., 2004; Vitale et al., 2007; Sato et al., 2014). In order to engineer MTJ-like constructs to regenerate these cases, a biomaterial platform should be developed which have characteristics of both tissues as well as the MTJ. This engineering challenge is still unmet due to the limitations of the current biomaterial processing techniques. While there are some promising studies available in the literature to engineer muscle and tendon tissues to produce substitutes with structural and biochemical similarity to the native tissues, production of engineered tissues with native contractile properties and functionality have not been reported yet. There are even less studies performed toward engineering of muscle-tendon interface and much remains to be done.
Among the available MTJ-like construct production studies, there are scaffold-based and scaffold-free approaches. Scaffold-based approaches has several advantages like better control on the shape, size, and porosity of the construct. Many scaffolds have been produced, varying from the use of acellular tissues to synthetic gels designed for engineering muscle and tendon units. However, much less effort was spent toward scaffold design for MTJ (Ladd et al., 2011). The reason for this could be associated with several drawbacks including the stress shielding effect. Stiff scaffolds bear much of the stress of an applied load, decreasing the amount experienced by the cells seeded onto the scaffold. Second challenge is the poor adaptation of current scaffold production technologies to engineer multi-segment tissues. While several studies on engineering muscle grafts have been reported using an acellulerized tissue as a scaffold, the use of acellulerized tendon as is was not successful due to the difficulty of seeding cells into its dense protein matrix (Turner and Badylak, 2013). Currently no ideal biological scaffold is defined for the regeneration of MTJ.
More successful attempts can be found in the literature with scaffold-free approaches due to the benefits of this approach. One of the advantages is the prevention of stress shielding. This technique also avoids the complications associated with incompatible structural and mechanical properties of scaffolds. Pioneering studies include heterogeneous muscle and tendon cell isolation and administration to the defect site (Larkin et al., 2006; Kostrominova et al., 2009; Ma et al., 2012). In these studies, histology results showed the presence of interdigitations between muscle and tendon tissues. Local adhesion proteins at the interface were also identified. Studies have reported that these scaffold-free have a potential to interdigitate into native tissue and mature in vivo (Williams et al., 2013).
Tendon/Ligament-Bone Interface
Tendons and ligaments are connective tissues. Tendons bind muscles to bones, while ligamants connect bones to bones. There is a continuously phased area, interface/enthesis, where tendons and ligaments meet the bones. Within the body, the tendon/ligament-bone interface is composed of a continuous gradual transition of three different types of tissues: tendon/ligament, fibrocartilage, and bone (Figure 1) (Thomopoulos, 2011). These transition areas also consist of different cell types, such as fibroblasts, fibrochondrocytes, and osteoblasts. The main component of tendons and ligaments is collagen and the presence of collagens type I and III are the typical of these tissues together with decorin in small amounts. The most critical area of this interface is the fibrocartilage part and it is the meeting point of the tendon/ligament and bone tissues. Fibrocartilage is composed of collagen type II and type III enriched with proteoglycans including aggrecan and decorin in order to give the flexibility to the interface. The tissue is the transition site between tendon/ligament and bone, and it is assumed to have two layers; mineralized and non-mineralized fibrocartilage. The ECM of bone tissue is mainly composed of collagen type I together with other proteins including osteopontin as well as mineralized content (mostly HAP) (Benjamin et al., 1986; Langer and Vacanti, 1993; Genin et al., 2009; Zhang et al., 2012).
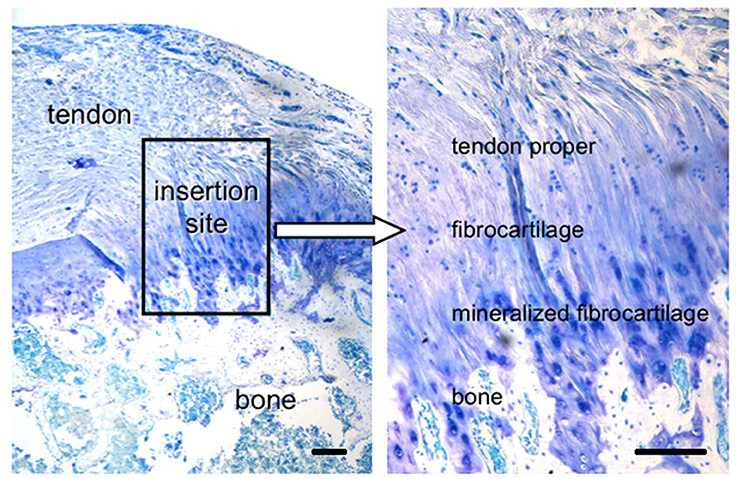
Figure 1. Tendon-to-bone interface, across a functionally graded fibrocartilaginous transition site (toluidine blue-stained section from a rat supraspinatus tendon-to-bone insertion is shown; scale Bar = 200 μm). (Reprinted by permission from Nature Publisher Group, IBMS BoneKEy, Thomopoulos, 2011 copyright © 2011).
Taking this complex hierarchical organization into consideration, various models for scaffolds and grafts have been proposed for the repair of the tendon/ligament-bone interface (Figure 2) (Tellado et al., 2015). However, the scaffolds designed for tendon/ligament interfaces are often far from representing the physiological structure, as they are designed as layers with or without minerals (Moffat et al., 2009; Kim et al., 2014). Moreover, it is also known that the reconstructed graft materials that have been accepted today do not achieve complete success in creating the gradual structure that exists in the native tissue, and that it has been torn again over time (Friedman et al., 1985; Robertson et al., 1986; Kurosaka et al., 1987; Rodeo et al., 1993). Thus, there is a need for new reinforcing materials that can repair and merge the tissues not only mechanically but also biologically in order to mimic the native tissues completely.
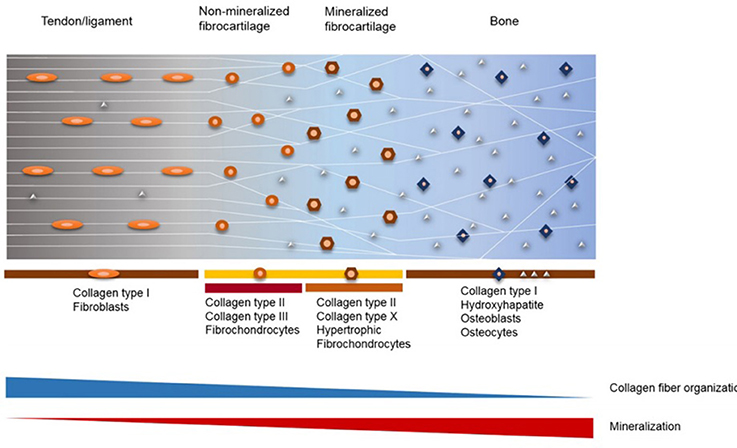
Figure 2. Structure and composition of fibrocartilaginous entheses of tendon/ligament-bone interface (Reprinted by permission from Elsevier, Advanced Drug Delivery Reviews, Tellado et al., 2015 copyright © 2015).
Electrospinning is a common scaffold production technique and it has been widely employed to engineer bone-tendon interface (Agarwal et al., 2008; Sill and von Recum, 2008; Bhardwaj and Kundu, 2010). By using this technique, fiber diameter, alignment, and porosity can be adjusted. For example, alignment can be increased with a rotating mandrel (De Filippo et al., 2008; Bayrak et al., 2017). Also, electrospun fibers can be used as a drug delivery tool due to the biodegradable nature of the polymers used. An electrospun fiber based gene delivery strategy was developed for dermal fibroblasts (Phillips et al., 2008), where it was shown that incorporating a Runx2/Cbfa1 delivery system into the biomaterial platform enables the spatial organization of osteoblasts and fibroblasts at their respective zones. In another study, Yokoya et al. performed a study on rabbits to test the effects of polyglycolic acid (PGA) and poly(ε-caprolactone) (PCL) sheets on tendon-bone interface regeneration. It was shown that the presence of scaffolds increased the potential of generation of a tendon insertion with a fibrocartilage layer for rotator cuff regeneration in vivo. However, the translational potential of this design is limited due to the difference between the shoulder anatomy of rabbits and humans (Yokoya et al., 2008).
There are several scaffold-based approaches for engineering tendon-to-bone interface. Since this interface has a multilayer structure, the proper scaffold should also be multiphasic in order to mimic the native tissue properly (Gulotta et al., 2009; Moffat et al., 2009). Selection of biomaterial is also very crucial due to the need for matching mechanical and degradation properties. Polymers are not the only solution for material selection for tendon-to-bone interface regeneration in the literature.
There are some novel approaches which include metallic implants. For example, Higuera et al. designed an implant to replace the tuberosity of the canine humerus. They observed that almost-natural joint function could be achieved (Higuera et al., 2005). In the design of Inoue et al. an augmented metal bone plate (with bone marrow and trabecular bone graft) was used as a reattachment site at the interface of bone-tendon attachment (Inoue et al., 2002). This design was proven to be successful to increase the strength of bone-tendon attachment.
Cartilage-Bone Interface
Cartilage-bone interface maintains the integrity of the osteochondral unit during daily activities. Osteoarthritis is a worldwide health problem, the most common form of arthritis, and it mainly occurs at the cartilage-bone interface. Many treatment options have been developed including direct injection regimes, however, long term results of these treatments were not able to recapitulate the natural structure (Wallny et al., 2000). Treatment of osteochondral defects still remain a challenge due to the facts that cartilage tissue is avascular and has a poor ability of regeneration. Current treatments mainly focus on pain relief and preservation of joint functions (Fajardo and Di Cesare, 2005). Some of the procedures include arthroscopic lavage (to ease the joint pain), microfracture, arthoplasty, and soft tissue grafts (Redman et al., 2005). Down side of these procedures are that they do not stimulate tissue repair. Tissue engineering strategies gain importance in order to achieve this goal.
The cartilage tissue contains collagen type II, aggrecan, decorin, fibromodulin, and biglycan in the ECM (Heinegård and Oldberg, 1989; Bhosale and Richardson, 2008). At the native cartilage-bone interface, the cartilage is connected to the subchondral bone via the osteochondral unit, which contains a layer of hypertrophic chondrocytes embedded in a calcified cartilage matrix (Yang and Temenoff, 2009). The ECM of the calcified cartilage below the tidemark region does not contain proteoglycans but contains collagen type X and alkaline phosphatase (Oegema et al., 1997). Therefore, the elastic modulus of the interface lays in between that of the unmineralized cartilage and the subchondral bone tissue. Characteristics of the interface enable cartilage-bone assembly by supporting the pressurization in the presence of physical loading, and act as a barrier against vascular infiltration (Bullough and Jagannath, 1983). So, in order to regenerate the osteochondral defects, compositional elements must be maintained within the material. Because of the complex structural, compositional, and mechanical properties of the osteochondral interface, a biomaterial based approach could be beneficial rather than a cell-based approach. Since the physical and mechanical properties of the biomaterials could be adjusted as needed, a functional support can be achieved upon implantation providing an advantage for the treatment (Jiang et al., 2010).
Biomaterial-based approaches in the treatment of osteochondral defects can be investigated in two categories: strategies based on polymeric and metallic biomaterials. In treating osteochondral defects, one of the main problems is to maintain the integrity of the newly formed tissues. Moreover, the degradative, mechanical, and structural properties must be adjusted to support mechanical stability during regeneration (Yang and Temenoff, 2009).
Cartilage-bone interface is a multilayered and complex structure, and several approaches were used in the literature in order to mimic this structure. For example, Kayabe et al. used a single-phase scaffold system composed of hyaluronic acid (HA) gels loaded with fibroblast growth factor-2 (FGF-2) and mesenchymal stem cells (MSCs). Results of this study showed that HA did not stimulate the regeneration of the full thickness osteochondral defect on its own, while while MSC proliferation in the presence of FGF-2 showed significant enhancement in the articular cartilage repair (Kayakabe et al., 2006). However, regeneration of the underlying bone tissue was not achieved by this single-phased system. Therefore, researchers design and produce bilayered scaffold systems for interface tissue regeneration which could provide suitable microenvironment for both bone and cartilage tissues. Although several challenges are associated with the fabrication of gradient scaffolds with varying structure, they are still much more suitable for osteochondral tissue regeneration rather than simple designs. In the study of Khanarian et al. a hydrogel based, bilayered scaffold was investigated as an osteochondral construct. A hydrogel-ceramic composite was used, calcium crosslinked alginate hydrogels were prepared and the bilayered construct was maintained by adding HAP to the lower part of the hydrogel. The study showed that the HAP part of the structure enhanced proteoglycan collagen type II rich matrix formation, which are the typical components of the calcified osteochondral interface (Khanarian et al., 2011).
In order to mimic the ECM closely, a nanocomposite multilayered scaffold was developed where the upper layer was prepared from collagen, the intermediate layer was prepared by using HAP and the lower layer was prepared by HAP grafted collagen, respectively (Tampieri et al., 2008). This study showed that the produced scaffolds can be used in the clinic and that significant regeneration of subchondral bone can be achieved. Also, fibrocartilaginous tissue formation was generated in the cartilage layer which indicates the need to enhance the biological acitivity of the scaffold by using bioactive molecules or cells.
Besides the polymeric biomaterials, metallic biomaterials were also used as potential constructs for osteochondral regeneration. Chang et al. produced a titanium-fiber mesh by binding the fibers by gelling the polyvinyl alcohol (PVA). The design promoted strong fixation as cancellous bone ingrowth was achieved into the titanium-fiber mesh. Also the bonding between host bone and penetrating bone and regaining the shock-absorbing function in the joint was achieved (Chang et al., 1998).
Trabecular metals have been widely used for different applications in orthopedic surgery (Bobyn et al., 1999; Cohen, 2002; Gordon et al., 2005). Mrosek et al. used tantalum as a trabecular metal and combine it with PCL in order to achieve cartilage and subchondral bone regeneration. This study was designed to be a proof-of-concept study to determine the feasibility of using tantalum as a metal implant and PCL as a biodegradable implant for osteochondral injuries. Tantalum and PCL were combined with the periosteal grafts and showed bony incorporation in both ways.
On the other hand, chondrogenic potential of the periosteum was not stimulated by the presence of these biomaterials (Mrosek et al., 2010). Nowadays apart from polymeric and metallic biomaterials, the use of magnetic nanoparticles (MNPs) to induce tissue growth through magnetic fields are also gaining importance. Iron doped and iron based biomaterials are used to guide bone regeneration (De Santis et al., 2011). De Santis et al. produced a PCL and polyethylene glycol (PEG) based magnetic nanocomposite scaffold and they showed that this scaffold adequately reproduce viscoelastic properties of trabecular bone and articular cartilage tissues (De Santis et al., 2016).
Conclusion
Development of tissue engineered products for the musculoskeletal interfaces are highly required, yet still in its infancy. Vast variety of scaffold production techniques must be challenged in the production of graded/complex structures. Numerous scaffold-based and scaffold-free approaches were evaluated for engineering of muscle and tendon separately, but the materials developed for interfaces are very rare because of the requirement of the multilayered/complex structures together with compositional and mechanical properties of the interface. Further research is needed to produce a suitable material for regeneration of interfaces. Similarly, design and production of scaffolds for tendon-to-bone interface and osteochondral defects is challenging and further studies are needed.
Author Contributions
EB: Performed literature review and wrote the manuscript; PY: Supervised the writing and edited the text.
Conflict of Interest Statement
The authors declare that the research was conducted in the absence of any commercial or financial relationships that could be construed as a potential conflict of interest.
Acknowledgments
We gratefully acknowledge Turkish Academy of Sciences for support to PY (TUBA-GEBIP Program).
References
Agarwal, S., Wendorff, J. H., and Greiner, A. (2008). Use of electrospinning technique for biomedical applications. Polymer 49, 5603–5621. doi: 10.1016/j.polymer.2008.09.014
Alexander, R. M. (1988). Elastic Mechanisms in Animal Movement. Cambridge: Cambridge University Press.
Bayrak, E., Ozcan, B., and Erisken, C. (2017). Processing of polycaprolactone and hydroxyapatite to fabricate graded electrospun composites for tendon-bone interface regeneration. J. Polym. Sci. 37, 99–106. doi: 10.1515/polyeng-2016-0017
Benjamin, M., Evans, E. J., and Copp, L. (1986). The histology of tendon attachments to bone in man. J. Anat. 149, 89–100.
Bhardwaj, N., and Kundu, S. C. (2010). Electrospinning: a fascinating fiber fabrication technique. Biotechnol. Adv. 28, 325–347. doi: 10.1016/j.biotechadv.2010.01.004
Bhosale, A. M., and Richardson, J. B. (2008). Articular cartilage: structure, injuries and review of management. Br. Med. Bull. 87, 77–95. doi: 10.1093/bmb/ldn025
Bobyn, J. D., Stackpool, G. J., Hacking, S. A., Tanzer, M., and Krygier, J. J. (1999). Characteristics of bone ingrowth and interface mechanics of a new porous tantalum biomaterial. J. Bone Joint Surg. Br. 81, 907–914. doi: 10.1302/0301-620X.81B5.9283
Bozyczko, D., Decker, C., Muschler, J., and Horwitz, A. F. (1989). Integrin on developing and adult skeletal muscle. Exp. Cell Res. 183, 72–91. doi: 10.1016/0014-4827(89)90419-9
Bullough, P. G., and Jagannath, A. (1983). The morphology of the calcification front in articular cartilage, its significance in joint function. J. Bone Joint Surg. 344, 65, 72–78. doi: 10.1302/0301-620X.65B1.6337169
Carlsson, L., Röstlund, T., Albrektsson, B., Albrektsson, T., and Brånemar, P. I. (1986). Osseointegration of titanium implants. Acta Orthop. Scand. 57, 285–289. doi: 10.3109/17453678608994393
Chang, Y. S., Gu, H. O., Kobayashi, M., and Oka, M. (1998). Comparison of the bony ingrowth into an osteochondral defect and an artificial osteochondral composite device in load-bearing joints. Knee 5, 205–213. doi: 10.1016/S0968-0160(97)10001-1
Chen, P., Piao, X., and Bonaldo, P. (2015). Role of macrophages in Wallerian degeneration and axonal regeneration after peripheral nerve injury. Acta Neuropathol. 130, 605–618. doi: 10.1007/s00401-015-1482-4
Conti, F. J., Monkley, S. J., Wood, M. R., Critchley, D. R., and Müller, U. (2009). Talin 1 and 2 are required for myoblast fusion, sarcomere assembly and the maintenance of myotendinous junctions. Development 136, 3597–3606. doi: 10.1242/dev.035857
Curzi, D., Ambrogini, P., Falcieri, E., and Burattini, S. (2013). Morphogenesis of rat myotendinous junction. Muscles Ligaments Tendons J. 3, 275–280.
De Filippo, R. E., Bishop, C. E., Filho, L. F., Yoo, J. J., and Atala, A. (2008). Tissue engineering a complete vaginal replacement from a small biopsy of autologous tissue. Transplantation 86, 208–214. doi: 10.1097/TP.0b013e31817f1686
De Santis, R., Gloria, A., Russo, T., D'Amora, U., Zeppetelli, S., Tampieri, A., et al. (2011). A route toward the development of 3D magnetic scaffolds with tailored mechanical and morphological properties for hard tissue regeneration: preliminary study. Virtual Phys. Prototyp. 6, 189–195. doi: 10.1080/17452759.2011.631324
De Santis, R., Gloria, A., Russo, T., Ronca, A., D'Amora, U., Negri, G., et al. (2016). Viscoelastic properties of rapid prototyped magnetic nanocomposite scaffolds for ostechondral tissue regeneration. Procedia CIRP. 49, 76–82. doi: 10.1016/j.procir.2015.07.037
Erisken, C., Kalyon, D. M., Wang, H., Ornek-Ballanco, C., and Xu, J. (2011). Osteochondral tissue formation through adipose-derived stromal cell differentiation on biomimetic polycaprolactone nanofibrous scaffolds with graded insulin and Beta-glycerophosphate concentrations. Tissue Eng. Part A 17, 1239–1252. doi: 10.1089/ten.tea.2009.0693
Fajardo, M., and Di Cesare, P. E. (2005). Disease-modifying therapies for osteoarthritis: current status. Drugs Aging 22, 141–161. doi: 10.2165/00002512-200522020-00005
Freed, L. E., Hollander, A. P., Martin, I., Barry, J. R., Langer, R., and Vunjak-Novakovic, G. (1998). Chondrogenesis in a cell-polymer-bioreactor system. Exp. Cell Res. 240, 58–65.
Friedman, M. J., Sherman, O. H., Fox, J. M., Delpizzo, W., Snyder, S. J., and Ferkel, R. J. (1985). Autogeneic anterior cruciate ligament (ACL) anterior reconstruction of the knee - a review. Clin. Orthop. Relat. Res. 196, 9–14. doi: 10.1097/00003086-198506000-00004
Galatz, L. M., Ball, C. M., Teefey, S. A., Middleton, W. D., and Yamaguchi, K. (2004). The outcome and repair integrity of completely arthroscopically repaired large and massive rotator cuff tears. J. Bone Joint Surg. 86, 219–224. doi: 10.2106/00004623-200402000-00002
Genin, G. M., Kent, A., Birman, V., Wopenka, B., Pasteris, J. D., Marquez, P. J., et al. (2009). Functional grading of mineral and collagen in the attachment of tendon to bone. Biophys. J. 97, 976–985. doi: 10.1016/j.bpj.2009.05.043
Glowacki, J., and Mizuno, S. (2008). Collagen scaffolds for tissue engineering. Biopolymers 89, 338–344. doi: 10.1002/bip.20871
Gordon, W. J., Conzemius, M. G., Birdsall, E., Wannemuehler, Y., Mallapragada, S., Lewallen, D. G., et al. (2005). Chondroconductive potential of tantalum trabecular metal. J. Biomed. Mater. Res. Part B Appl. Biomater. 75, 229–233. doi: 10.1002/jbm.b.30242
Gulotta, L. V., Kovacevic, D., Ehteshami, J. R., Dagher, E., Packer, J. D., and Rodeo, S. A. (2009). Application of bone marrow-derived mesenchymal stem cells in a rotator cuff repair model. Am. J. Sports Med. 37, 2126–2133. doi: 10.1177/0363546509339582
Heinegård, D., and Oldberg, A. (1989). Structure and biology of cartilageand bone matrix noncollagenous macromolecules. FASEB J. 3, 2042–2051. doi: 10.1096/fasebj.3.9.2663581
Higuera, C. A., Inoue, N., Lim, J. S., Zhang, R., Dimaano, N., Frassica, F. J., et al. (2005). Tendon reattachment to a metallic implant using an allogenic bone plate augmented with rhOP-1 vs. autogenous cancellous bone and marrow in a canine model. J. Orthopaed. Res. 23, 1091–1099. doi: 10.1016/j.orthres.2005.01.017
Hwang, W., Kelly, N. G., and Boriek, A. M. (2005). Passive mechanics of muscle tendinous junction of canine diaphragm. J. Appl. Physiol. 98, 1328–1333. doi: 10.1152/japplphysiol.00816.2004
Inoue, N., Ikeda, K., Aro, H. T., Frassica, F. J., Sim, F. H., and Chao, E. Y. (2002). Biologic fixation to metallic implant augmented with autogenous cancellous bone graft and bone marrow in a canine model. J. Orthopaed. Res. 20, 957–966. doi: 10.1016/S0736-0266(02)00037-2
Jiang, J., Tang, A., Ateshian, G. A., Guo, X. E., Hung, C. T., and Lu, H. H. (2010). Bioactive stratified polymer ceramic-hydrogel scaffold for integrative osteochondral repair. Ann. Biomed. Eng. 38, 2183–2196. doi: 10.1007/s10439-010-0038-y
Kannus, P., Jozsa, L., Kvist, M., Lehto, M., and Järvinen, M. (1992). The effect of immobilization on myotendinous junction: an ultrastructural, histochemical and immunohistochemical study. Acta Physiol. Scand. 144, 387–394. doi: 10.1111/j.1748-1716.1992.tb09309.x
Kayakabe, M., Tsutsumi, S., Watanabe, H., Kato, Y., and Takagishi, K. (2006). Transplantation of autologous rabbit BM-derived mesenchymal stromal cells embedded in hyaluronic acid gel sponge into osteochondral defects of the knee. Cytotherapy 8, 343–353. doi: 10.1080/14653240600845070
Khanarian, N. T., Jiang, J., Wan, L. Q., Mow, V. C., and Lu, H. H. (2011). A hydrogel-mineral composite scaffold for osteochondral interface tissue engineering. Tissue Eng. Part A 18, 533–545. doi: 10.1089/ten.tea.2011.0279
Kim, B. S., Kim, E. J., Choi, J. S., Jeong, J. H., Jo, C. H., and Cho, Y. W. (2014). Human collagen-based multilayer scaffolds for tendon-to-bone interface tissue engineering. J. Biomed. Mater. Res. Part A 102, 4044–4054. doi: 10.1002/jbm.a.35057
Kostrominova, T. Y., Calve, S., Arruda, E. M., and Larkin, L. M. (2009). Ultrastructure of myotendinous junctions in tendon-skeletal muscle constructs engineered in vitro. Histol. Histopathol. 24, 541–550. doi: 10.14670/HH-24.541
Krishnan, V., and Lakshmi, T. (2013). Bioglass: a novel biocompatible innovation. J. Adv. Pharm. Technol. Res. 4, 78–83. doi: 10.4103/2231-4040.111523
Kurosaka, M., Yoshiya, S., and Andrish, J. T. (1987). A biomechanical comparison of different surgical techniques of graft fixation in anterior cruciate ligament reconstruction. Am. J. Sports Med. 15, 225–229. doi: 10.1177/036354658701500306
Kvist, M., Jozsa, L., Kannus, P., Isola, J., Vieno, T., and Järvinen, M. (1991). Morphology and histochemistry of the myotendineal junction of the rat calf muscles. Histochemical, immunohistochemical and electron-microscopic study. Acta Anat. 141, 199–205. doi: 10.1159/000147122
Ladd, M. R., Lee, S. J., Stitzel, J. D., Atala, A., and Yoo, J. J. (2011). Co-electrospun dual scaffolding system with potential for muscle–tendon junction tissue engineering. Biomaterials 32, 1549–1559. doi: 10.1016/j.biomaterials.2010.10.038
Langer, R., and Vacanti, J. P. (1993). Tissue engineering. Science 260, 920–926. doi: 10.1126/science.8493529
Langer, R., and Vacanti, J. (2016). Advances in tissue engineering. J. Pediatr. Surg. 51, 8–12. doi: 10.1016/j.jpedsurg.2015.10.022
Larkin, L. M., Calve, S., Kostrominova, T. Y., and Arruda, E. M. (2006). Structure and functional evaluation of tendon-skeletal muscle constructs engineered in vitro. Tissue Eng. 12, 3149–3158. doi: 10.1089/ten.2006.12.3149
Lysholm, J., and Wiklander, J. (1987). Injuries in runners. Am. J. Sports Med. 15, 168–171. doi: 10.1177/036354658701500213
Ma, J., Smietana, M. J., Kostrominova, T. Y., Wojtys, E. M., Larkin, L. M., and Arruda, E. M. (2012). Three-dimensional engineered bone-ligament-bone constructs for anterior cruciate ligament replacement. Tissue Eng. Part A 18, 103–116. doi: 10.1089/ten.tea.2011.0231
Martinez, A., Blanco, M. D., Davidenko, N., and Cameron, R. E. (2015). Tailoring chitosan/collagen scaffolds for tissue engineering: effect of composition and different crosslinking agents on scaffolds preparation. Carbohydr. Polym. 132, 606–619. doi: 10.1016/j.carbpol.2015.06.084
Mironov, A. V., Grigoryev, A. M., Krotova, L. I., Skaletsky, N. N., Popov, V. K., and Sevastianov, V. I. (2017). 3D printing of PLGA scaffolds for tissue engineering. J. Biomed. Mater. Res. A 105, 104–109. doi: 10.1002/jbm.a.35871
Moffat, K. L., Kwei, A. S., Spalazzi, J. P., Doty, S. B., Levine, W. N., and Lu, H. H. (2009). Novel nanofiber-based scaffold for rotator cuff repair and augmentation. Tissue Eng. Part A 15, 115–126. doi: 10.1089/ten.tea.2008.0014
Mrosek, E. H., Schagemann, J. C., Chung, H. W., Fitzsimmons, J. S., Yaszemski, M. J., Mardones, R. M., et al. (2010). Porous tantalum and poly-e-caprolactone biocomposites for osteochondral defect repair: preliminary studies in rabbits. J. Orthopaed. Res. 28, 141–148. doi: 10.1002/jor.20983
Oegema, T. R., Carpenter, R. J., Hofmeister, F., and Thompson, R. C. (1997). The interaction of the zone of calcified cartilage andsubchondral bone in osteoarthritis. Microsc. Res. Tech. 37, 324–332. doi: 10.1002/(SICI)1097-0029(19970515)37:4<324::AID-JEMT7>3.0.CO;2-K
Pan, Z., and Ding, J. (2012). Poly(lactide-co-glycolide) porous scaffolds for tissue engineering and regenerative medicine. Interface Focus 2, 366–377. doi: 10.1098/rsfs.2011.0123
Phillips, J. E., Burns, K. L., Le Doux, J. M., Guldberg, R. E., and García, A. J. (2008). Engineering graded tissue interfaces. Proc. Natl. Acad. Sci. U.S.A. 105, 12170–12175. doi: 10.1073/pnas.0801988105
Redman, S. N., Oldfield, S. F., and Archer, C. W. (2005). Current strategies for articular cartilage repair. Eur. J. Cells Mater. 9, 23–32. doi: 10.22203/eCM.v009a04
Rice, M. A., Dodson, B. T., Arthur, J. A., and Anseth, K. S. (2005). Cell-based therapies and tissue engineering. Otolaryngol. Clin. North Am. 38, 199–214. doi: 10.1016/j.otc.2004.10.010
Robertson, D. B., Daniel, D. M., and Biden, E. (1986). Soft-tissue fixation to bone. Am. J. Sports Med. 14, 398–403. doi: 10.1177/036354658601400512
Rodeo, S. A., Arnoczky, S. P., Torzilli, P. A., Hidaka, C., and Warren, R. F. (1993). Tendon-healing in a bone tunnel - a biomechanical and histological study in the dog. J. Bone Joint Surg. Am. 75A, 1795–1803. doi: 10.2106/00004623-199312000-00009
Sato, E. J., Killian, M. L., Choi, A. J., Lin, E., Esparza, M. C., Galatz, L. M., et al. (2014). Skeletal muscle fibrosis and stiffness increase after rotator cuff tendon injury and neuromuscular compromise in a rat model. J. Orthopaed. Res. 32, 1111–1116. doi: 10.1002/jor.22646
Shear, C. R., and Bloch, R. J. (1985). Vinculin in subsarcolemmal densities in chicken skeletal muscle: localization and relationship to intracellular and extracellular structures. J. Cell Biol. 101, 240–256. doi: 10.1083/jcb.101.1.240
Sill, T. J., and von Recum, H. A. (2008). Electrospinning: applications in drug delivery and tissue engineering. Biomaterials 29, 1989–2006. doi: 10.1016/j.biomaterials.2008.01.011
Swasdison, S., and Maynei, R. (1989). Location of the integrin complex and extracellular matrix molecules at the chicken myotendinous junction. Cell Tiss. Res. 257, 537–543. doi: 10.1007/BF00221463
Tampieri, A., Sandri, M., Landi, E., Pressato, D., Francioli, S., Quarto, R., et al. (2008). Design of graded biomimetic osteochondral composite scaffolds. Biomaterials 29, 3539–3546. doi: 10.1016/j.biomaterials.2008.05.008
Tellado, S. F., Balmayor, E. R., and Griensven, M. V. (2015). Strategies to engineer tendon/ligament to bone interface: biomaterials, cells and growth factors. Adv. Drug Deliv. Rev. 94, 126–140. doi: 10.1016/j.addr.2015.03.004
Thomopoulos, S. (2011). The role of mechanobiology in the attachment of tendon to bone. Int. Bone Miner. Soc. 8, 271–285. doi: 10.1138/20110515
Trotter, J. A. (1993). Functional morphology of force transmission in skeletal muscle. Acta Anat. 146, 205–222. doi: 10.1159/000147459
Turner, N. J., and Badylak, S. F. (2013). Biologic scaffolds for musculotendinous tissue repair. Eur. Cell. Mater. 25, 130–143. doi: 10.22203/eCM.v025a09
Turner, C. E. (2000). Paxillin and focal adhesion signaling. Nat. Cell Biol. 2, 231–236. doi: 10.1038/35046659
Vitale, M. A., Vitale, M. G., Zirin, J. G., Braman, J. P., Bigliani, L. U., and Flatow, E. L. (2007). Rotator cuff repair: an analysis of uility scores and cost effectiveness. J. Shoulder Elbow Surg. 16, 181–187. doi: 10.1016/j.jse.2006.06.013
Wallny, T., Brackmann, H. H., Semper, H., Schumpe, G., Effenberger, W., Hess, L., et al. (2000). Intra-articular hyaluronic acid in the treatment of haemophilic arthropathy of the knee: clinical, radiological and sonographical assessment. Haemophilia 6, 566–570. doi: 10.1046/j.1365-2516.2000.00413.x
Williams, M. L., Kostrominova, T. Y., Arruda, E. M., and Larkin, L. M. (2013). Effect of implantation on engineered skeletal muscle constructs. J. Tissue Eng. Regen. Med. 7, 434–442. doi: 10.1002/term.537
Williams, D. F. (2009). On the nature of biomaterials. Biomaterials 30, 5897–5909. doi: 10.1016/j.biomaterials.2009.07.027
Woo, S. L. Y. (1987). “Injury and repair of the musculoskeletal soft tissues: workshop,” in Chapter 5: Garrett, W. And Tidball, J. G. Myotendinous Junction: Structure, Function, and Failure, ed J. A. Buckwalter (American Academy of Orthopaedic Surgeons), 171–207.
Yang, P. J., and Temenoff, J. S. (2009). Engineering orthopaedic tissue interfaces. Tissue Eng. Part B 15, 127–141. doi: 10.1089/ten.teb.2008.0371
Yilgor, P., Sousa, R. A., Reis, R. L., Hasirci, N., and Hasirci, V. (2010). Effect of scaffold architecture and BMP-2/BMP-7 delivery on in vitro bone regeneration. J. Mater. Sci. Mater. Med. 21, 2999–3008. doi: 10.1007/s10856-010-4150-1
Yokoya, S., Mochizuki, Y., Nagata, Y., Deie, M., and Ochi, M. (2008). Tendon-Bone insertion repair and regeneration using polyglycolic acid sheet in the rabbit rotator cuff injury model. Am. J. Sports Med. 36, 1298–1309. doi: 10.1177/0363546508314416
Keywords: musculoskeletal tissue interfaces, scaffolds, polymeric surfaces, metallic surfaces, functional biomaterials, tissue engineering
Citation: Bayrak E and Yilgor Huri P (2018) Engineering Musculoskeletal Tissue Interfaces. Front. Mater. 5:24. doi: 10.3389/fmats.2018.00024
Received: 26 January 2018; Accepted: 09 April 2018;
Published: 30 April 2018.
Edited by:
Cosmin Mihai Cotrut, Politehnica University of Bucharest, RomaniaReviewed by:
Ana Maria Rosca, Institute of Cellular Biology and Pathology (ICBP), RomaniaThomas Monsees, University of the Western Cape, South Africa
Copyright © 2018 Bayrak and Yilgor Huri. This is an open-access article distributed under the terms of the Creative Commons Attribution License (CC BY). The use, distribution or reproduction in other forums is permitted, provided the original author(s) and the copyright owner are credited and that the original publication in this journal is cited, in accordance with accepted academic practice. No use, distribution or reproduction is permitted which does not comply with these terms.
*Correspondence: Pinar Yilgor Huri, cGh1cmlAYW5rYXJhLmVkdS50cg==