- Department of Medical Biosciences, University of the Western Cape, Bellville, South Africa
With regard to biocompatibility, the cardinal requirement for dental implants and other medical devices that are in long-term contact with tissue is that the material does not cause any adverse effect to the patient. To warrant stability and function of the implant, proper osseointegration is a further prerequisite. Cells interact with the implant surface as the interface between bulk material and biological tissue. Whereas structuring, deposition of a thin film, or other modifications of the surface are crucial parameters in determining favorable adhesion of cells, corrosion of metal surfaces and release of ions can affect cell viability. Both parameters are usually tested using in vitro cytotoxicity and adhesion assays with bone or fibroblasts cells. For bioactive surface modifications, further tests should be considered for biocompatibility evaluation. Depending on the type of modification, this may include analysis of specific cell functions or the determination of antimicrobial activities. The latter is of special importance as bacteria and yeast present in the oral cavity can be introduced during the implantation process and this may lead to chronic infections and implant failure. An antimicrobial coating of the implant is a way to avoid that. This review describes the essential biocompatibility assays for evaluation of new implant materials required by International Organization for Standardization 10993 and also provides an overview of recent test methods for specific coatings of dental implants.
Introduction: Why Characterize Biocompatibility?
The implantation of a medical device into the body is a surgical procedure that introduces injury followed by a healing process and a long-term contact with body tissue. Several interactions between the implant surface and the patient’s body happen which will be discussed in more detail later. The most important aspect here is that the implant material shall exist in close contact with body tissue without causing harm to the patient. To assure this, a sequence of specific tests has to be undertaken, before any new medical device can be declared fit for human use by the relevant health organization. That is what biocompatibility is about: a measurement of how safe and compatible a medical device, such as an implant, is with a biological system. As we will see later, the specific physico-chemical properties and nano-structuring of the implant surface are crucial for the adhesion, differentiation, and proper function of bone cells. This can be controlled by modification of the implant’s surface by, e.g., coating with a wide range of substances. From a Research & Development point of view, the observed interaction between implant and biological material (i.e., the outcome of a biocompatibility test) can be used to incrementally improve the (surface) properties until the desired physiological characteristics are achieved. One comprehensive definition of biocompatibility that captures the essence with respect to implant material, interaction with the body, and benefit for the patient was drafted by Williams (2008): “Biocompatibility refers to the ability of a biomaterial to perform its desired function with respect to a medical therapy, without eliciting and undesirable local or systemic effects in the recipient or beneficiary of that therapy, but generating the most appropriate beneficial cellular or tissue response in that specific situation, and optimizing the clinically relevant performance of that therapy.” Same author also defined biomaterial as “…a substance that has been engineered to take a form which, alone or as part of a complex system, is used to direct, by control of interactions with components of living systems, the course of any therapeutic or diagnostic procedure, …” (Williams, 2009).
What Needs to be Investigated?
Biocompatibility characterization has to answer two essential questions: is the material safe for use within the human body? Also, does the material/device have the necessary physico-chemical and mechanical properties to fulfill its purpose? The International Organization for Standardization (ISO) has drafted a set of international standards for the biological evaluation of medical devices (ISO 10993). Biocompatibility tests have to comply with the ISO 10993 for registration of medical devices in Europe, Asia, and USA. Some health administrations, such as the FDA (USA), may need additional testing requirements. ISO 10993 consists of 20 parts spanning a wide range of specific testing procedures from physico-chemical investigation of the device toward its interaction with cells and body. The required tests will be dependent on the specific use of the medical device and contact time with the human body. Part 1 of the ISO 10993 can be used as a framework to select those tests. For dental implants with a contact period exceeding 30 days, several tests are required to determine its biocompatibility. This includes tests for cytotoxicity, sensitization, irritation or intracutaneous (skin) reactivity, acute and sub-chronic toxicity as well as genotoxicity, and implantation. For medical devices (e.g., electrodes, external prostheses) that are merely in contact with the skin surface, only the first three tests have to be performed.
In general, biocompatible tests can be divided into:
• Tests concerning the implant material, such as extend of degradation in biological fluids or identification of leachable substances.
• In vitro tests that examine the interaction of the implant with cells or microorganisms.
• In vivo animal tests that determine the effect of the implant on the body.
This review will focus on in vitro tests that evaluate the interaction between implant surface and bone cells or microorganisms.
Implantation and Osseointegration
A dental implant usually consists of three parts: the implant itself that is inserted directly into the jaw bone (endosseous implant) and the abutment that connects the implant to the overlying crone or denture (i.e., the visible part). Titanium or its alloys are usually used as implant material because of its unique capability of osseointegration. Osseointegration means the formation of a direct interface between an implant and bone, without intervening soft tissue, cartilage, or protein layer (Karlsson et al., 2014). Titanium naturally forms a thin protective oxide film on its surface that can eventually transform into calcium phosphate apatite that then binds chemically with the bone (Monsees et al., 2005). Also, the interface between titanium implant and superficial soft tissue is similar to the union between tooth and gingivae. To enhance the osseointegration process, endosseous implants commonly have some sort of coating and/or surface modification (Table 1).
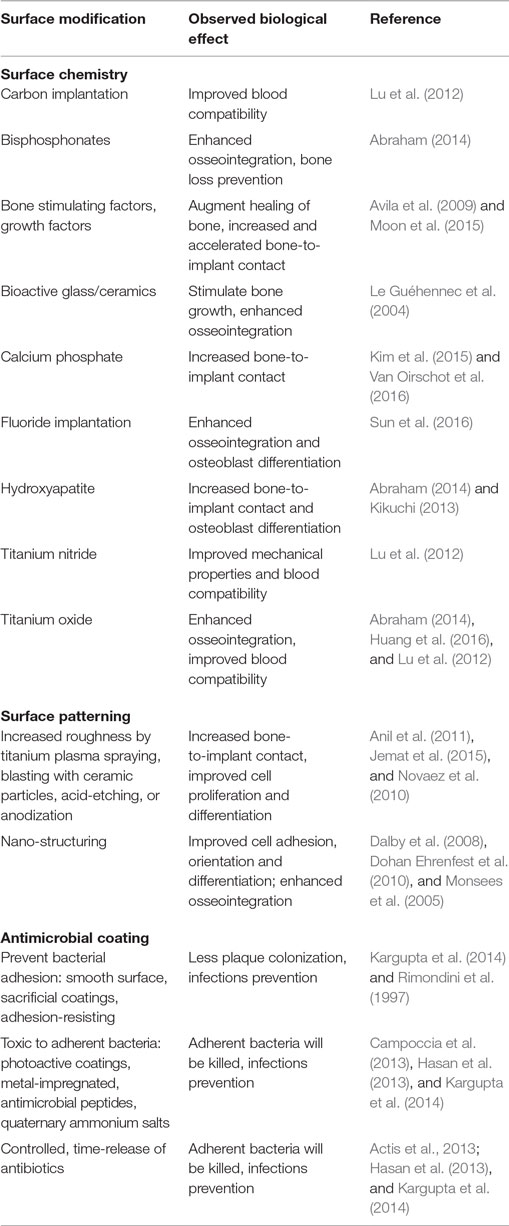
Table 1. Overview on coatings for dental implants that improve osseointegration and/or prevent microbial colonization.
Surface Interactions
Interaction: Implant Surface with Bone Cells
For osseointegration to work, bone cells must make contact with the implant surface. Osteoblasts are those cells that build new or repair damaged bone. This is a complex process called osteogenesis and starts with the adhesion of osteoblasts to existing bone or the implant surface. Further events involve bone cell proliferation and differentiation, expression of bone cell-specific enzymes and products, secretion, and deposition of the organic bone matrix. When a bone cell, floating in interstitial fluid or blood, approaches the implant it only “feels” the device surface, not the bulk material. This surface displays charged and hydrogen-bond groups and lipophilic patches originating from either implant or adsorbed extracellular matrix. This matrix originates from adsorption of proteins from surrounding tissue fluid or blood and is also secreted by the bone cells. Typical matrix ligands are collagen type I and fibronectin. Some implants display those bio-adhesive ligands via their surface coating (Cohen et al., 2004; Garcia and Reyes, 2005). Initially, there are weak and temporary interactions between charged or polar molecules of cell membrane and implant surface. Over time, those interactions can become stronger and more specific leading to permanent cell adhesion via binding of adhesion receptors (e.g., integrins, cadherins) located on the osteoblast cell membrane to extracellular matrix ligands present on the implant surface. After binding to an extracellular matrix ligand, the integrins associate with the actin cytoskeleton and cytoplasmic plaque proteins (e.g., vinculin) to form focal adhesions (Gumbiner, 1996). At a focal adhesion, the distance between cell membrane receptor and ligand on the implant surface drops to only 15 nm (Cohen et al., 2004). Focal adhesions are crucial for the spreading and flattening of a cell that is important to gain strong adhesion. Together with growth factor receptors, focal adhesions also trigger integrin-activated signaling cascades leading to osteoblast differentiation and physiological function (Garcia and Reyes, 2005). The establishment of integrin receptor–matrix ligand occurs within minutes after the initial surface recognition. Adhesion with proper cell spreading then takes several hours during which the cell–implant contact area increases 100-fold (Cohen et al., 2004). The adhesion process can be greatly influenced by the specific physico-chemical properties – such as charges, chemistry, wettability, roughness – and nano-structuring of the implant surface (Monsees et al., 2005; Ozkucur et al., 2009b; Novaez et al., 2010). Thus, it is extremely important to compare those parameters with the outcome of the biocompatibility tests.
Interaction: Implant Surface with Microorganism
Implanted devices are prone to biofilm formation. A biofilm is a thin but robust layer of microorganisms, such as bacteria, fungi, and yeast that adhere to the implant surface similar to the dental plaque sticking to the teeth (Actis et al., 2013; Kargupta et al., 2014). These microorganisms can cause serious infections around the implant initiating progressive bone loss leading to loosening or even loss of the implant (Wood et al., 2004). In case of titanium implants, the surface oxide coating achieves an amalgamation with the superficial gum that hinders the invasion of oral microorganisms. However, the amount of bacterial colonization can be influenced by implant surface coating or structuring (Table 1). For example, a rougher surface supports bacterial growth and provides protection from saliva and cleansing by the tongue, whereas a smoother surface limits the adhesion of bacteria (Rimondini et al., 1997). Because of this interaction, antibacterial activity tests should also be considered when testing the biocompatibility of a novel coating. Modern antimicrobial coatings are designed to prevent biofilm formation by, e.g., preventing bacterial adhesion, killing bacteria, or by accelerating osteoblast adhesion (reviewed in Hasan et al., 2013; Kargupta et al., 2014).
Evaluation of Biocompatibility In Vitro
Structuring, deposition of a thin film, or other modifications of the implant surface are crucial parameters in determining favorable adhesion and physiological function of cells. Corrosion of metal surfaces and release of ions or other leaching products can affect cell viability. Both parameters are usually tested using in vitro cytotoxicity and adhesion assays with bone cells or fibroblasts. Part 5 of ISO 10993 defines standard tests to evaluate the cytotoxicity of a medical device using in vitro methods.
Cells
There are two principal classes of cells: established cell lines and primary cells. Established cell lines are considered the standard for similar research that makes comparison of results and standardization of procedures possible. Cell lines can be bought commercially from trusted sources, are easy to work with, and can be used for many years. However, because these cells can divide continuously, they may not reflect the true physiology of the original cell. By contrast, primary cells must be isolated directly from the body and can be kept in culture for only a limited time. This makes them difficult and expensive to work with; however, the results are much closer to the in vivo situation. Often used cell types are immortalized neonatal mouse osteoblasts (MC3T3E1), human osteosarcoma (SaOS-2 or MG-63), primary rat calvaria cells, or L929 mouse fibroblasts (Meyer et al., 2005; Monsees et al., 2005; Bierbaum et al., 2006; Subramanian et al., 2015).
Cytotoxicity
Cytotoxicity may be tested using an extract or the implant material itself. The conditions of extraction should meet the intended clinical use. Suitable eluents are cell culture media or physiological saline. The tests must include a negative control (culture medium) and positive control (e.g., 6% DMSO in culture medium). Cells must be incubated for a minimum period of 24 h. For testing cytotoxicity in direct cell contact, cells are applied to the surface of suitable samples under sterile conditions and then incubated for 1–3 days. Alternatively, the implant sample is carefully placed on top of the cell layer. Other approved tests are the Agar and the Filter Diffusion assay. Both are indirect contact tests that evaluate if substances from the implant can be dissolved and move through a thin barrier (agar layer or inert filter) toward the cells.
For transparent samples, cell numbers and morphology can be checked via simple light microscopy. Living cells should have their typical form and are well attached and spread; they usually form clusters (Subramanian et al., 2015). Damaged or dying cells develop vacuoles, may shrink, become rounder in appearance, and eventually detach from the surface. Thus, on a sub-optimal surface, cell numbers are lower. For non-transparent samples, cells can be visualized using an epifluorescence or scanning electron microscope (Ozkucur et al., 2009a). For quantitative measurement of cytotoxicity, suitable parameters are inhibition of cell proliferation, numbers of dead and live cells, reduction of vital dyes, and release of cytoplasmic enzymes as a result of cell membrane damage. The MTT assay measures the activity of mitochondrial enzymes, which not only reflects the number of viable cells present (used as proliferation assay) but also mirrors the degree of mitochondrial activity that indicates cellular stress or dying cells (used as cytotoxicity test). Technically, this assay works by reduction of the yellow tetrazolium dye MTT (3-(4,5-dimethylthiazol-2-yl)-2,5-diphenyltetrazolium bromide) to a purple insoluble formazan. This color increase can be measured using a photometer. Ozkucur et al. (2009a) showed significantly higher MTT-values, thus more living cells attached on metal-coated polyurethane compared to the uncoated surfaces. Also, the titanium thin film seems to be more favorable for adhesion than the zirconium coating. Other related tetrazolium dyes are XTT (2,3-bis-(2-methoxy-4-nitro-5-sulfophenyl)-2H-tetrazolium-5-carboxanilide), MTS (3-(4,5-dimethylthiazol-2-yl)-5-(3-carboxymethoxyphenyl)-2-(4-sulfophenyl)-2H-tetrazolium), and WSTs (water-soluble tetrazolium salts). These colorimetric assays provide average values off many thousands cells but do not give any information about the fate of individual cells. Here, Live/Dead assays using a pair of fluorophores are the better choice. The general principle is that one of these fluorophores can enter the living cell (intact membrane), whereas the other one cannot. The second dye can only enter if the cell membrane is compromised indicating a dead or dying cell. This second dye usually then binds to DNA in the cell nuclei. An example is the calcein-AM – ethidium homodimer, EthD-1 assay that shows living cells in green, whereas dead cells emit red fluorescence (Huang et al., 2016).
Cell Adhesion
The sequence leading to cell adhesion and spreading gives us some important parameter for additional characterization. There are several cellular components that can be visualized, e.g., using specific fluorescent dye-tagged antibodies. Often used targets are the actin stress fibers that mark the cell morphology; vinculin, a protein located in focal adhesions; and DNA to visualize the cell nuclei. In this way, different degrees of cell adhesion and spreading can be clearly distinguished (Monsees et al., 2005). Ozkucur et al. (2009a) depicted human umbilical vein endothelial cells grown on titanium thin-film-coated polyurethane: cells are well spread with regularly organized actin cytoskeleton and many focal adhesion contacts. By contrast, cell numbers and spreading on the uncoated polyurethane were very poor. Similarly, adhesion and spreading of endothelial cells is poor on polyurethane, but is dramatically improved on a 30-nm titanium film as shown with scanning electron microscopy. The more sophisticated Wound-Healing assay assesses cell proliferation, actin cytoskeleton reorganization, dissolving/renewing of focal adhesion points, and migration. Here, cells are grown on the implant surface then an open gap is created by removing cells via scratching through the confluent cell monolayer. The time for the cells to close this “wound” by proliferation, spreading, and moving is monitored (Oprea et al., 2003; Bindschadler and McGrath, 2007).
Physiological Cell Function
As the implant surface properties can also influence osteoblast differentiation and, thus, ultimately their physiological function, more specialized investigations should be considered. Typical osteoblast-specific parameters include activity of alkaline phosphatase enzyme, secretion of collagen type I, osteopontin or osteocalcin, or formation of calcium phosphate deposits (Hempel et al., 2004; Meyer et al., 2005). Alkaline phosphatase activity can be determined, e.g., via histochemical staining and fluorescence microscopy. Monsees et al. (2005) noted regular alkaline phosphatase activity in SaOS-2 osteoblasts attached to smooth or structured titanium thin films. By contrast, surface composition greatly influenced osteopontin expression.
Biodegradation
Material degradation or corrosion within the body can lead to loosening and failure of the implant. The impact of body fluids on the degradation of metallic implants can be modeled using artificial saliva, blood serum, and salt solution (media) normally used for tissue culture at 37°C for longer periods of time (days or weeks) (ISO 10993 Part 15). Parameters investigated may include changes in weight or hardness of the implant material, electrochemical behavior, changes of the surface, by means of profilometry, scanning electron or atomic force microscopy, and nature and amount of metal ions leaching from the implant assessed by X-ray diffraction and atomic emission spectroscopy (Wetzel et al., 2008; Lesniewicz et al., 2010). It is worthwhile to also compare potential biodegradation with implants that have been in contact with cells for the same time and conditions. Depending on the surface coating and the underlying bulk material (metal alloy, ceramics, or polyurethane), the presence of cells may protect the surface, e.g., by secreting extracellular matrix proteins (Ozkucur et al., 2009a) or may accelerate especially polymer degradation by releasing hydrolytic enzymes (Ozkucur et al., 2009b).
Microbiology
Microorganisms often used in testing the anti-microbiological activity of implants include bacteria that can be present in the oral cavity, e.g., Staphylococcus aureus and Pseudomonas aeruginosa (Gram-negative), and Streptococcus sanguinis and Escherichia coli (Gram-positive) (Pye et al., 2009). Two often used tests are the Agar Disc Diffusion assay and the Direct Contact method. In the agar diffusion assay, the respective single strain of a microorganism is spread on solidified agar medium. Then the implant sample is gently pressed on the agar and incubated for 24 h at 37°C. Any antibacterial activity is measured as a zone of inhibition around the sample disk. A standard implant surface serves as “negative control,” whereas an appropriate antibiotic serves as positive control (Subramanian et al., 2015). For direct contact method, media containing the respective bacteria are placed onto round implant samples and then incubated at 37°C for 24 h. Alternatively, implant samples can be incubated on a shaker with the respective bacteria suspension. Adhered bacteria populations can be visualized after appropriate staining using a stereo-optical or epifluorescence microscope or processed for scanning electron microscopy (Chung et al., 2006; Patenge et al., 2012).
Conclusion
The aim of any biomaterial research is to improve the properties of the implant in certain ways. This may be faster and better attachment of osteoblasts or more effective deterring of bacteria. In vitro biocompatibility characterization is a means to test such potential improvements under precise conditions. Besides the mandatory cytotoxicity assay, further tests are recommended to investigate the influence of the novel surface modification on more complex cellular parameters such as adhesion, morphology, differentiation and physiological function. These more sophisticated tests will lead to a better understanding of the precise interaction between implant surface and surrounding cells or microorganisms. The outcome of the biocompatibility tests can then be used to steer the surface modification process into the desired direction.
Author Contributions
TM planned and wrote the manuscript of this mini review.
Conflict of Interest Statement
The author declares that this research was performed in the absence of any commercial or financial relationships that could be understood as a potential conflict of interest.
References
Abraham, C. M. (2014). A brief historical perspective on dental implants, their surface coatings and treatments. Open Dent. J. 8, 50–55. doi:10.2174/1874210601408010050
Actis, L., Gaviria, L., Guda, T., and Ong, J. L. (2013). Antimicrobial surfaces for craniofacial implants: state of the art. J. Korean Assoc. Oral Maxillofac. Surg. 39, 43–54. doi:10.5125/jkaoms.2013.39.2.43
Anil, S., Anand, P. S., Alghamdi, H., and Jansen, J. A. (2011). “Dental implant surface enhancement and osseointegration,” in Implant Chemistry – A Rapidly Evolving Practice, ed. I. Turkyilmaz (Rijeka: InTech), 83–108.
Avila, G., Misch, K., Galindo-Moreno, P., and Wang, H. L. (2009). Implant surface treatment using biomimetic agents. Implant Dent. 18, 17–26. doi:10.1097/ID.0b013e318192cb7d
Bierbaum, S., Douglas, T., Hanke, T., Scharnweber, D., Tippelt, S., Monsees, T. K., et al. (2006). Collagenous matrix coatings on titanium implants modified with decorin and chondroitin sulfate: characterization and influence on osteoblastic cells. J. Biomed. Mater. Res. A 77, 551–562. doi:10.1002/jbm.a.30572
Bindschadler, M., and McGrath, J. L. (2007). Sheet migration by wounded monolayers as an emergent property of single-cell dynamics. J. Cell. Sci. 120, 876–884. doi:10.1242/jcs.03395
Campoccia, D., Montanaro, L., and Arciola, C. R. (2013). A review of the biomaterials technologies for infection-resistant surfaces. Biomaterials 32, 8533–8554. doi:10.1016/j.biomaterials.2013.07.089
Chung, R. J., Hsieh, M. F., Huang, C. W., Perng, L. H., Wen, H. W., and Chin, T. S. (2006). Antimicrobial effects and human gingival biocompatibility of hydroxyapatite sol-gel coatings. J. Biomed. Mater. Res. B Appl. Biomater. 76, 169–178. doi:10.1002/jbm.b.30365
Cohen, M., Joester, D., Geiger, B., and Addadi, L. (2004). Spatial and temporal sequence of events in cell adhesion: from molecular recognition to focal adhesion assembly. Chembiochem 5, 1393–1399. doi:10.1002/cbic.200400162
Dalby, M. J., Andar, A., Nag, A., Affrossman, S., Tare, R., McFarlane, S., et al. (2008). Genomic expression of mesenchymal stem cells to altered nanoscale topographies. J R Soc Interface 5, 1055–1065. doi:10.1098/rsif.2008.0016
Dohan Ehrenfest, D. M., Coelho, P. G., Kang, B. S., Sul, Y. T., and Albrektsson, T. (2010). Classification of osseointegrated implant surfaces: materials, chemistry and topography. Trends Biotechnol. 28, 198–206. doi:10.1016/j.tibtech.2009.12.003
Garcia, A. J., and Reyes, C. D. (2005). Bio-adhesive surfaces to promote osteoblast differentiation and bone formation. J. Dent. Res. 84, 407–413. doi:10.1177/154405910508400502
Gumbiner, B. M. (1996). Cell adhesion: the molecular basis of tissue architecture and morphogenesis. Cell 84, 345–357. doi:10.1016/S0092-8674(00)81279-9
Hasan, J., Crawford, R. J., and Ivanova, E. P. (2013). Antibacterial surfaces: the quest for a new generation of biomaterials. Trends Biotechnol. 31, 295–304. doi:10.1016/j.tibtech.2013.01.017
Hempel, U., Reinstorf, A., Poppe, M., Fisher, U., Gelinsky, M., Pompe, W., et al. (2004). Proliferation and differentiation of osteoblasts on biocement D modified with collagen type I and citric acid. J. Biomed. Mater. Res. B Appl. Biomater. 71, 143. doi:10.1002/jbm.b.30082
Huang, Q., Elkhooly, T. A., Liu, X., Zhang, R., Yang, X., Shen, Z., et al. (2016). SaOS-2 cell response to macro-porous boron-incorporated TiO2 coating prepared by micro-arc oxidation on titanium. Mater. Sci. Eng. C 67, 195–204. doi:10.1016/j.msec.2016.05.051
Jemat, A., Ghazali, M. J., Razali, M., and Otsuka, Y. (2015). Surface modifications and their effects on titanium dental implants. Biomed. Res. 2015, 791725. doi:10.1155/2015/791725
Kargupta, R., Bok, S., Darr, C. M., Crist, B. D., Gangopadhyay, K., Gangopadhyay, S., et al. (2014). Coatings and surface modifications imparting antimicrobial activity to orthopedic implants. Wiley Interdiscip. Rev. Nanomed. Nanobiotechnol. 6, 475–495. doi:10.1002/wnan.1273
Karlsson, J., Sundell, G., Thuvander, M., and Andersson, M. (2014). Atomically resolved tissue integration. Nano Lett. 14, 4220–4223. doi:10.1021/nl501564f
Kikuchi, M. (2013). Hydroxyapatite/Collagen bone-like nanocomposite. Biol. Pharm. Bull. 36, 1666–1669. doi:10.1248/bpb.b13-00460
Kim, J., Magnos, M. H. R., Ortiz, O., McBride, S., Darr, A., Kohn, J., et al. (2015). Next generation resorbable polymer scaffolds with surface-precipitated calcium phosphate coatings. Regen. Biomater. 2, 1–8. doi:10.1093/rb/rbu019
Le Guéhennec, L., Layrolle, P., and Daculsi, G. (2004). A review of bioceramics and fibrin sealant. Eur. Cells Mater. 8, 1–11.
Lesniewicz, A., Gackiewcz, L., and Zyrnicki, W. (2010). Biodegradation of metallic surgical implants investigated using an ultrasound-assisted process combined with ICP-OES and XRD. Int. Biodeterior. Biodegradation 64, 81–85. doi:10.1016/j.ibiod.2009.09.012
Lu, T., Qiao, Y., and Liu, X. (2012). Surface modification of biomaterials using plasma immersion ion implantation and deposition. Interface Focus 2, 325–336. doi:10.1098/rsfs.2012.0003
Meyer, U., Buechter, A., Wiesmann, H. P., Joos, U., and Jones, D. B. (2005). Basic reactions of osteoblasts on structured material surfaces. Eur. Cells Mater. 9, 39–49.
Monsees, T. K., Barth, K., Tippelt, S., Heidel, K., Gorbunov, A., Pompe, W., et al. (2005). Effects of different titanium alloys and nanosize surface patterning on adhesion, differentiation and orientation of osteoblast-like cells. Cells Tissues Organs 180, 81–95. doi:10.1159/000086749
Moon, K. S., Choi, E. J., Oh, S., and Kim, S. (2015). The effect of covalently immobilized FGF-2 on biphasic calcium phosphate bone substitute on enhanced biological compatibility and activity. Biomed Res. Int. 2015, 742192. doi:10.1155/2015/742192
Novaez, A. B., de Souza, S. L. S., de Barros, R. R. M., Pereira, K. K. Y., Iezzi, G., and Piattelli, A. (2010). Influence of implant surfaces on osseointegration. Braz. Dent. J. 21, 471–481. doi:10.1590/S0103-64402010000600001
Oprea, W. E., Karp, J. M., Hosseini, M. M., and Davies, J. E. (2003). Effect of platelet release on bone cell migration and recruitment in vitro. J. Craniofac. Surg. 14, 292–300. doi:10.1097/00001665-200305000-00006
Ozkucur, N., Wetzel, C., Hollstein, F., Richter, E., Funk, R. H. W., and Monsees, T. K. (2009a). Physical vapor deposition of zirconium or titanium thin films on flexible polyurethane highly support adhesion and physiology of human endothelial cells. J. Biomed. Mater. Res. A 89, 57–67. doi:10.1002/jbm.a.32003
Ozkucur, N., Richter, R., Wetzel, C., Funk, R. H. W., and Monsees, T. K. (2009b). Biological relevance of ion energy in performance of human endothelial cells on ion-implanted flexible polyurethane surfaces. J. Biomed. Mater. Res. A 93, 258–268. doi:10.1002/jbm.a.32541
Patenge, N., Arndt, K., Eggert, T., Ziets, C., Kreikemeyer, B., Bader, R., et al. (2012). Evaluation of antimicrobial effects of novel implant materials by testing the prevention of biofilm formation using a simple scale medium-throughput growth inhibition assay. Biofouling 28, 267–277. doi:10.1080/08927014.2012.671305
Pye, A. D., Lockhart, D. E. A., Dawson, M. P., Murray, C. A., and Smith, A. J. (2009). A review of dental implants and infection. J. Hosp. Infect. 72, 104–110. doi:10.1016/j.jhin.2009.02.010
Rimondini, L., Fare, S., Brambilla, E., Felloni, A., Consonni, C., Brossa, F., et al. (1997). The effect of surface roughness on early in vivo plaque colonization on titanium. J. Periodontol. 68, 556–562. doi:10.1902/jop.1997.68.6.556
Subramanian, B., Maruthamuthu, S., and Rajan, S. T. (2015). Biocompatibility evaluation of sputtered zirconium-based thin film metallic glass-coated steels. Int. J. Nanomedicine 10, 17–29. doi:10.2147/IJN.S79977
Sun, W., Zhang, G., Tan, L., Yang, K., and Ai, H. (2016). The fluoride coated AZ31B magnesium alloy improves corrosion resistance and stimulates bone formation in rabbit model. Mater. Sci. Eng. C Mater. Biol. Appl. 63, 506–511. doi:10.1016/j.msec.2016.03.016
Van Oirschot, B. A. J. A., Bronkhorst, E. M., van den Beucken, J. J. J. P., Meijer, G. J., Jansen, J. A., and Junker, R. (2016). A systematic review on the long-term success of calcium phosphate plasma-spray-coated dental implants. Odontology. doi:10.1007/s10266-015-0230-5
Wetzel, C., Hollstein, F., Funk, R. H. W., Ozkucur, N., and Monsees, T. K. (2008). Investigation on zirconium-coated polyurethane surfaces with regard to biocompatibility. Surf. Coat. Technol. 202, 5728–5732. doi:10.1016/j.surfcoat.2008.06.134
Williams, D. F. (2008). On the mechanism of biocompatibility. Biomaterials 29, 2941–2953. doi:10.1016/j.biomaterials.2008.04.023
Williams, D. F. (2009). On the nature of biomaterials. Biomaterials 30, 5897–5909. doi:10.1016/j.biomaterials.2009.07.027
Wood, M. R., Vermilyea, S. G., Committee on Research in Fixed Prosthodontics of the Academy of Fixed Prosthodontics. (2004). A review of selected dental literature on evidence-based treatment planning for dental implants: report of the committee on research in fixed prosthodontics of the academy of fixed prosthodontics. J. Prosthet. Dent. 92, 447–462. doi:10.1016/j.prosdent.2004.08.003
Keywords: medical devices, biomaterial, biocompatibility, implants, coatings, cell adhesion, microorganisms
Citation: Monsees TK (2016) Biocompatibility and Anti-Microbiological Activity Characterization of Novel Coatings for Dental Implants: A Primer for Non-Biologists. Front. Mater. 3:40. doi: 10.3389/fmats.2016.00040
Received: 11 June 2016; Accepted: 03 August 2016;
Published: 18 August 2016
Edited by:
Alina Vladescu, National Institute for Research and Development in Optoelectronics, RomaniaReviewed by:
Ancuta Zazgyva, University of Medicine and Pharmacy of Tîrgu Mureş, RomaniaNemecz Andreea Miruna, Institute of Cellular Biology and Pathology “Nicolae Simionescu”, Romania
Copyright: © 2016 Monsees. This is an open-access article distributed under the terms of the Creative Commons Attribution License (CC BY). The use, distribution or reproduction in other forums is permitted, provided the original author(s) or licensor are credited and that the original publication in this journal is cited, in accordance with accepted academic practice. No use, distribution or reproduction is permitted which does not comply with these terms.
*Correspondence: Thomas K. Monsees, dG1vbnNlZXNAdXdjLmFjLnph