- 1Key Laboratory of Marine Geology and Metallogeny, First Institute of Oceanography, Ministry of Natural Resources, Qingdao, China
- 2Laboratory for Marine Geology, Qingdao Marine Science and Technology Center, Qingdao, China
- 3Key Laboratory of Deep Sea Mineral Resources Development, Shandong (Preparatory), Qingdao, China
Biogeochemical mineralization is increasingly recognized as a significant factor in the formation of submarine hydrothermal sulfide deposits. While several mechanisms by which hydrothermal organisms may facilitate metal deposition have been documented in many seafloor hydrothermal deposits, the potential involvement of biogenic processes in the mineralization of hydrothermal deposits in the southern Mid-Atlantic Ridge (SMAR) has been largely overlooked until now. In this study, we investigate sulfide chimney sample from the volcanic-hosted Tongguan hydrothermal field on the SMAR and present several lines of evidence for biogeochemical mineralization. Mineralogical analysis infers four types of biogenic pyrite and chalcopyrite structures: macrobiotic-related tube structures, microbial-related quasi-stromatolite, quasi-oncolite and globular structures. These biogenic structures exhibit selective enrichment of elements such as Mn, Pb, and Cu in biogenic pyrite compared to abiotic pyrite. In-situ sulfur isotope studies indicate that biogenic minerals possess lower δ34S values than abiotic minerals. We identified three biomineralization mechanisms: an “active” mineralization process mediated by macro-organism, a “passive” mineralization process associated with microbial mats, and a microbial assimilatory sulfate reduction process. Our research suggests that the role of biogenic processes in SMAR hydrothermal mineralization should be given further consideration.
1 Introduction
Modern seafloor hydrothermal systems represent some of the most extreme environments on Earth, capable of supporting oases of life that flourish in high temperatures and perpetual darkness (e.g. Corliss et al., 1979; Humphris et al., 1995). Within these settings, a high diversity of microorganisms, encompassing both Bacteria and Archaea, derives energy from inorganic compounds through a process known as chemosynthesis, colonized on the hydrothermal vents (Corliss et al., 1979; Cavanaugh et al., 1981). Furthermore, numerous microorganisms support macrofaunal communities in a symbiotic relationship, populating the hydrothermal vent ecosystems (Cavanaugh et al., 1981).
Microorganisms associated with mid-ocean ridge vent systems have demonstrated the capacity to thrive within a broad temperature range from 2°C to 122°C (Dick, 2019; Früh-Green et al., 2022). The volcanic-hosted hydrothermal fields, characterized by their relatively high fluid temperatures and an abundance of metals and volatile compounds, support a more diverse microbial community compared to the ultramafic fields influenced by serpentinization, which are predominantly inhabited by methane-metabolizing archaea (Schrenk et al., 2004). In these volcanic environments, a variety of microorganisms thrive, including sulfate-reducing bacteria, sulfide-oxidizing bacteria, methanogens, iron-oxidizing bacteria, and others. This biodiversity underpins an even more varied array of vent fauna, contributing to the complex ecosystem dynamics of these deep-sea habitats (Amend et al., 2011; Früh-Green et al., 2022).
The role of micro- and macro-organisms in seafloor mineralization has been extensively explored in previous studies (Juniper and Fouquet, 1988; Zierenberg and Schiffman, 1990; Koski et al., 1994; Piercey, 2015). Firstly, the metabolic processes of microorganisms themselves involve the reduction of sulfates, which can directly lead to the production of H2S. This H2S can then react with iron ions to form framboidal pyrite at the micron scale (Canfield, 2001; Piercey, 2015; Piercey et al., 2018), and such microbial sulfate reduction is considered to play a significant role in the initial stages of seafloor sulfide mineralization (Nozaki et al., 2020). Beyond metabolism, microorganisms can also facilitate sulfide precipitation through “passive” nucleation processes (Jonasson and Walker, 1987; Juniper and Fouquet, 1988). For instance, the bacterial mats near low-temperature hydrothermal vents can provide nucleation surfaces for the precipitation of hydrothermal minerals. Additionally, microorganisms may induce local sulfide precipitation by altering the pH or sulfur fugacity (fS2) in the microenvironments of bacterial mats (Hannington et al., 1995). Meanwhile, macro-organism can promote sulfide precipitation through “active” mediation (Zierenberg and Schiffman, 1990). For instance, polychaete worms typically secrete mucous layers that can accumulate sulfur and trace elements (such as Cd, Cu), and inherently present in the mucus (Juniper et al., 1986). These mucous layers trap metals and precipitate them as sulfides through adsorption or complexation with soluble species in the organic matrix (Hannington et al., 1995).
These micro- and macro-organisms participate in the mineralization process through various forms, creating specific biogenically formed structures (Cook and Stakes, 1995; Hannington et al., 1995; Piercey, 2015; Nozaki et al., 2020). Mineralogical evidence for this includes the presence of biological structures or their remnants within sulfides (Hannington and Scott, 1988; Juniper et al., 1992; Koski et al., 1994), as well as mineral textures in sulfides that can be explained by microbial activity (Hannington et al., 1995). These phenomena indicate that micro- and macro-organisms play a significant role in the mineralization process of seafloor hydrothermal sulfides.
Compared to other mid-ocean ridges, the investigation and research on hydrothermal sulfides along the Southern Mid-Atlantic Ridge (SMAR) are relatively limited. As an important part of the global mid-ocean ridges, the SMAR is presumed to have a potentially diverse high-temperature hydrothermal fields (Fouquet et al., 2013). It is speculated that, like other hydrothermal fields, there is significant involvement of biomineralization in the hydrothermal mineralization process. However, prior to this, there have been no research reports on biogenic mineralization in the SMAR, and it is unclear whether biology is involved in the mineralization and what types of mineralization occur on SMAR.
In 2015, a hydrothermal field named Tongguan (27.1°S), characterized by the presence of hydrothermal sulfides and biota, was discovered on the SMAR (Wang et al., 2022). This is an active volcanic-hosted high-temperature hydrothermal field where hydrothermal deposits accumulate on the sediment-free basaltic seafloor. The chimney wall fragment was sampled during this expedition. In this study, we identified evidence of Biogeochemical mineralization in terms of mineralogy, mineral chemistry, and in-situ sulfur isotopes from the studied sample. The biological entities involved in the hydrothermal mineralization include both micro-organisms and macro-organisms. This further confirms the ubiquity of biogenic mineralization in seafloor hydrothermal systems.
2 Tongguan hydrothermal field
The Tongguan hydrothermal field (27.15°S, 13.45°W; water depth ~3300m) stands as the most southerly discovered hydrothermal field in the SMAR to date. The SMAR is a slow-spreading ridge, and its ridge axis features a prominent central rift valley. The rift valley is defined by a graben-like structure, with an inner valley delineated by a succession of normal bounding faults. The topographical data shows that the ridge exhibits symmetrical spreading, centered on the rift valley, with a series of symmetrical linear volcanic ridges on both flanks of the axis (Figure 1). The active Tongguan hydrothermal field is developed on the newly formed volcanic ridge in the middle of the rift valley, it is a typical sediment-free hydrothermal field.
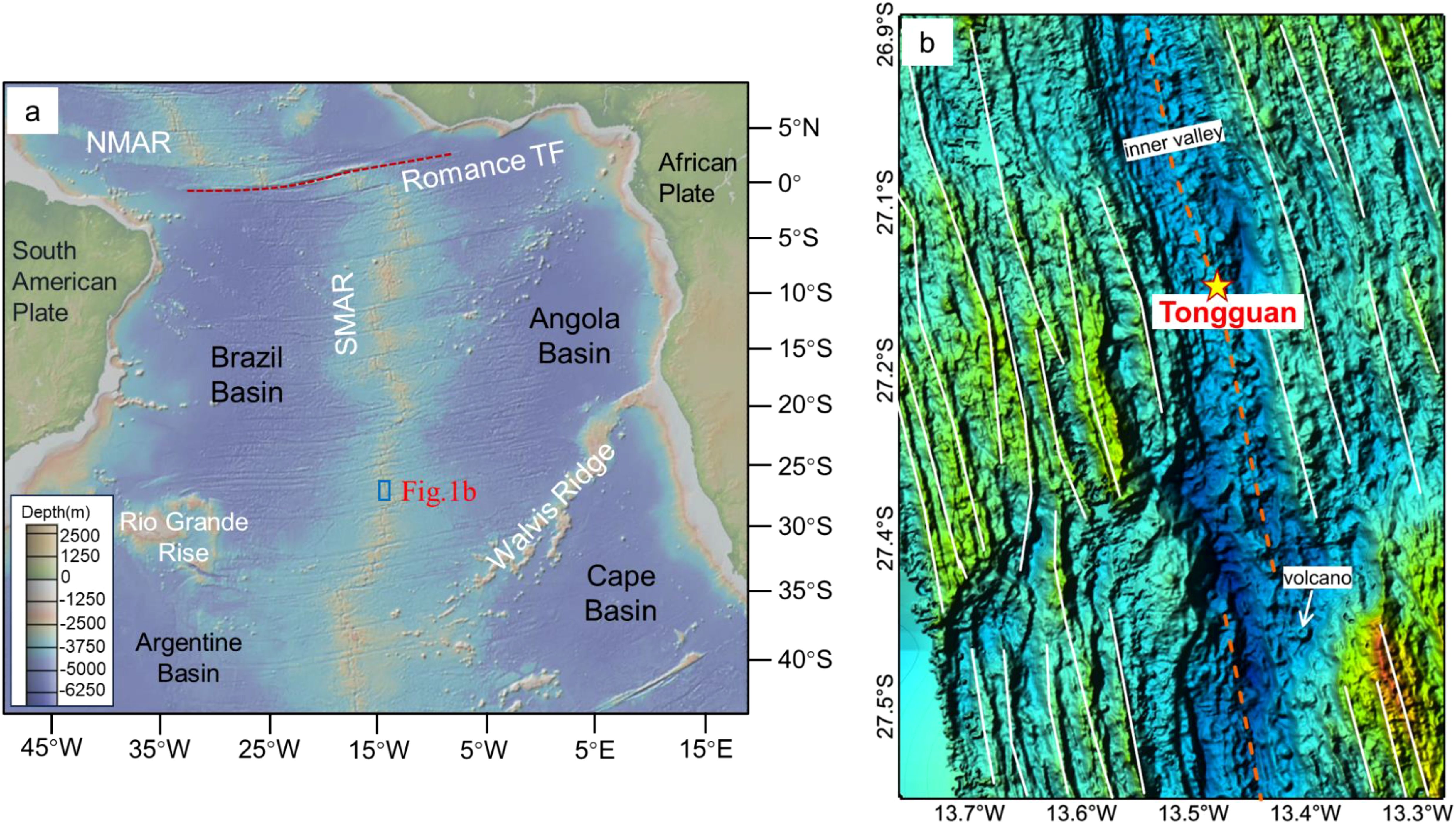
Figure 1. Tectonic boundaries and location of (b) along the southern Mid-Atlantic Ridge. (b) The topographic map of the ridge segment where the Tongguan (a) hydrothermal field is located, as measured by the shipborne multibeam survey.
Through the near-bottom observations by the manned submersible, the Tongguan hydrothermal field is directly developed on fresh pillow basalt (Figure 2a). Active black smoker vents can be seen (Figure 2b), and in their vicinity, numerous hydrothermal macro-organisms, mainly blind shrimp, crabs, and mussels, are visible. In addition, on the outer surface of the local hydrothermal edifice without enormous venting, a white bacterial mat can be seen (Figure 2c), which serves as a primary producer, providing a food source for large organisms like blind shrimp. Near the locally deceased chimney structures, a certain amount of biological remains can also be observed (Figure 2d).
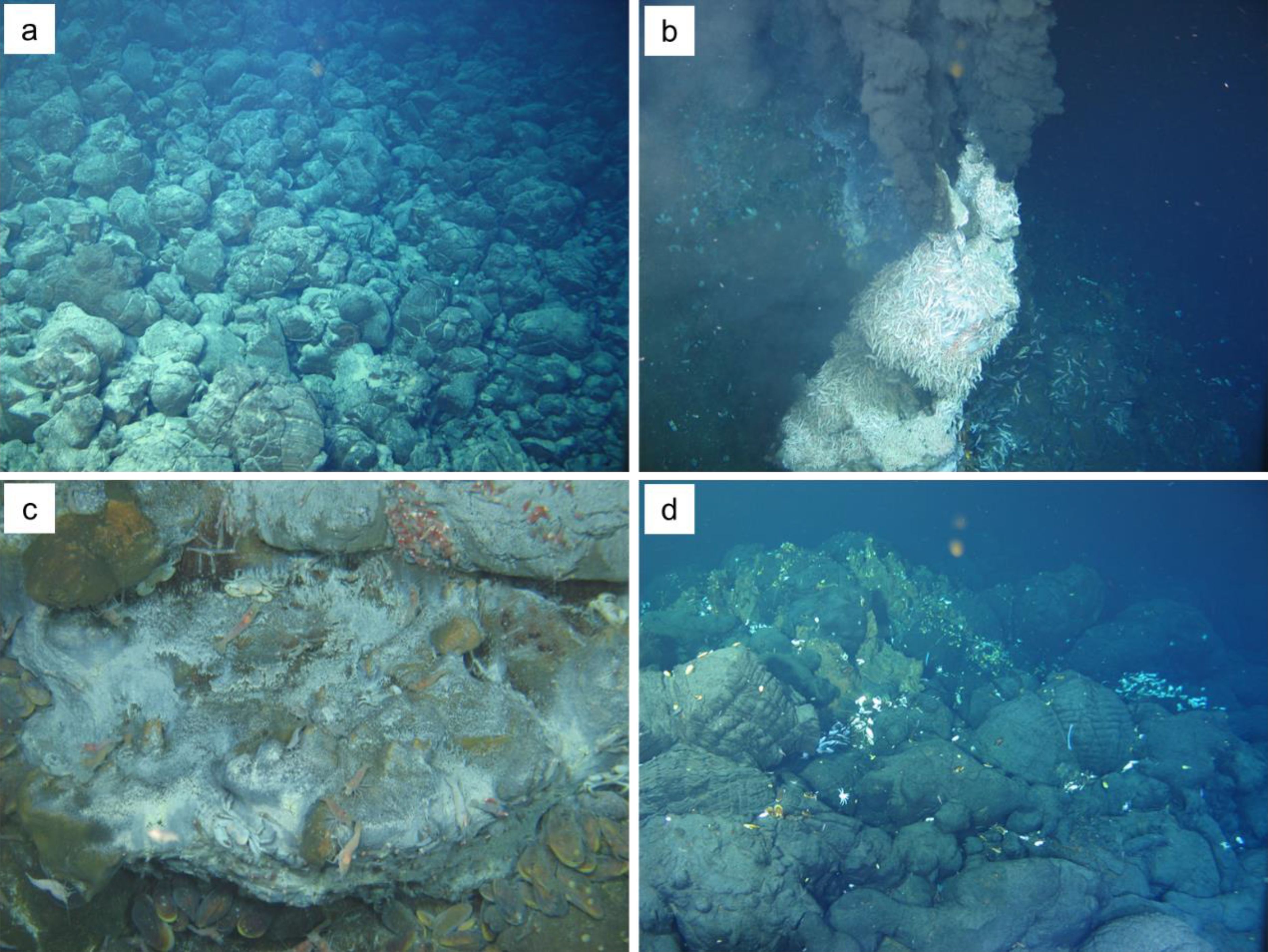
Figure 2. Photographs of the Tongguan hydrothermal vent field. (a) A large amount of fresh pillow basalt near the vent field. (b) An active hydrothermal vent edifice covered with countless hydrothermal blind shrimp. (c) White bacterial mats on the surface of sulfide edifices, along with microorganism such as blind shrimp, hydrothermal crabs, and mussels. (d) Small-scale inactive chimney structures and hydrothermal biological remains on the pillow basalt.
3 Sample and methods
The specimens examined in this research were gathered from the Chinese Ocean 33 expedition conducted in 2015. The collection of all specimens was carried out utilizing a TV grab. During the seaward operations, having pinpointed the site of the hydrothermal sulfide deposit through the use of towed cameras, we deployed a towed TV grab to harvest samples of hydrothermal sulfides.
The studied sample (Figure 3a) shows a fragment of a sulfide chimney wall characterized by mineral banded structures, such as the cross-section of a classic black smoker chimney (Haymon, 1983), exhibiting a sequence of various mineral aggregates that transition progressively from the interior to the exterior. The innermost layer is composed of coarse-grained chalcopyrite and quartz, while the outer layers are made up of fine-grained pyrite and sphalerite bands. Biogenic structures are primarily concentrated in the inner part.
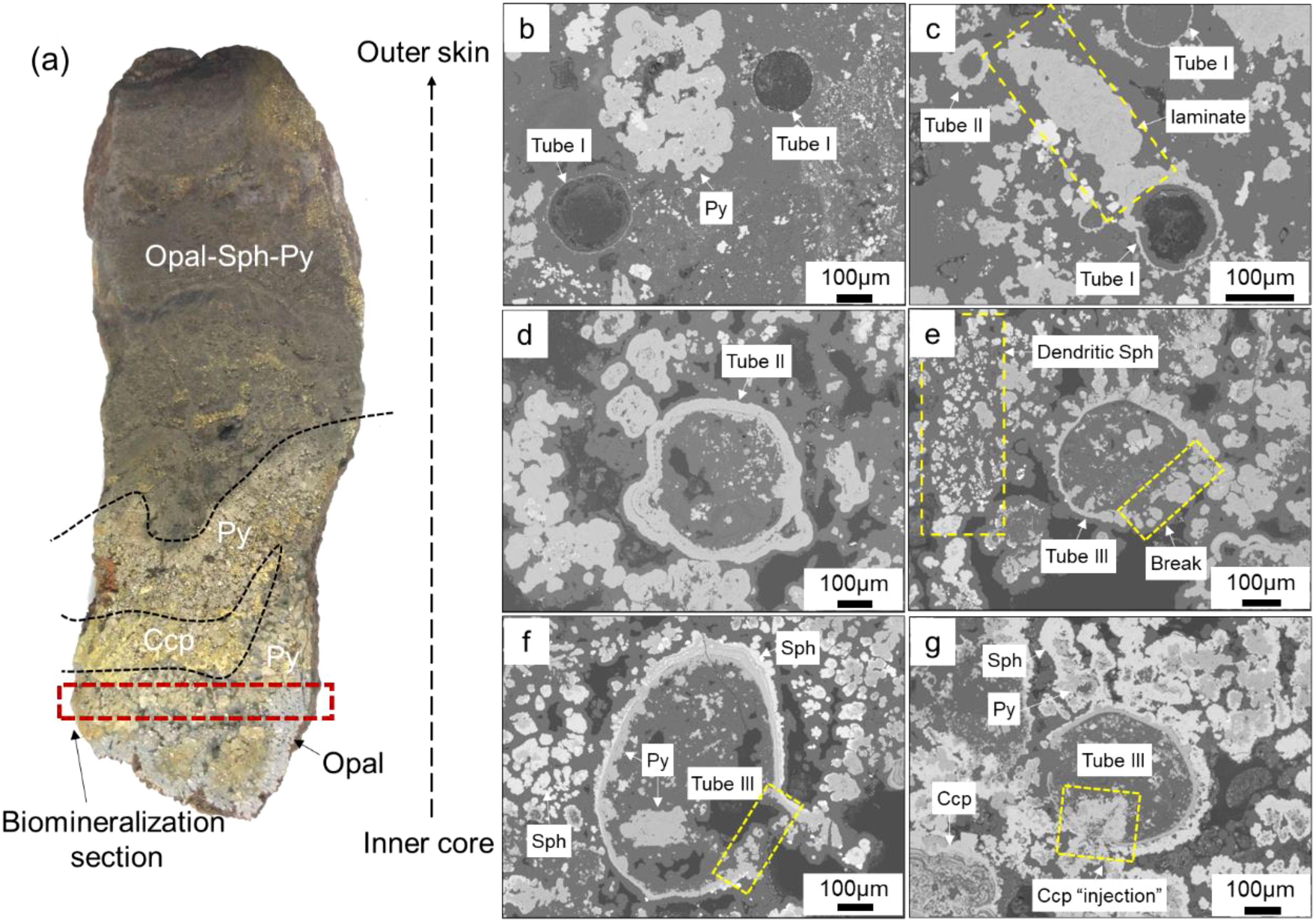
Figure 3. Backscatter electron microscopy of tube structures within Chimney wall. (a) Chimney wall exhibit layered mineralogical structures, representing fragments of chimneys. (b) Tube I: A thin layer of pyrite is adhered to the wall of the tube, while the interior remains unfilled by sulfides. (c) Part of the outer wall of Tube I is covered with directionally grown laminates. (d) Tube II: The outer wall has thickened, and the interior of the tube is filled with opal that contains a minor amount of sulfide crystals. (e) Tube III: Pyrite and sphalerite are attached to the outer wall of Tube II and extend outward in irregular “spiky” formations. Locally, pyrite also adheres to the inner wall and grows inward, revealing larger sphalerite and pyrite crystals within the tube. (f) The adherence of pyrite to the inner wall causes it to grow inward, while sphalerite adheres to the outer wall, prompting outward growth. Large pyrite crystals are visible inside the tube. (g) A distinct filling structure of chalcopyrite is observed, extending from the outside to the inside following a wall rupture. Py, Pyrite; Sph, Sphalerite; Ccp, Chalcopyrite.
The sample was first cut and polished into thin sections for further mineralogical and electron probe analysis. Optical reflection microscopy and Back Scattered Electron Imaging (BSE) were utilized in conjunction for the identification of minerals and the interpretation of textures. Microscopic observations were conducted using a Zeiss Axioskop 40 microscope, with magnifications ranging from 100 to 500 times.
For mineral chemical and BSE analysis, a JXA-8230 model Electron Probe X-ray Micro-Analyzer (EPMA) manufactured by JEOL (Japan Electron Optics Laboratory Co., Ltd.) was employed. The operating conditions were set as follows: an incident current of 2.0×10^-8 A, an acceleration voltage of 15 kV, and a beam spot size of 1.0μm. Simultaneous measurements were conducted for elements such as Fe, S, Cu, Zn, As, Pb, Mo, Co, Ni, Mn, Si, and Ag. The mineralogical and EPMA work was conducted at the Key Laboratory of Marine Geology and Metallogeny, FIO.
In-situ sulfur isotope analysis of pyrite and chalcopyrite was performed using the combination of a Teledyne Cetac Technologies Analyte Excite laser-ablation system (Bozeman, Montana, USA) and a Nu Instruments Nu Plasma II MC-ICP-MS (Wrexham, Wales, UK). During the experiment, the energy density of the laser beam used was 2.5 J/cm². Each acquisition included a 30-second background (gas blank) measurement, followed by a spot diameter of 33 µm (for pyrite) and 50 µm (for chalcopyrite) at a repetition rate of 5 Hz for 35 seconds. Helium (800 ml/min) was applied as a carrier gas to efficiently transport the aerosol out of the ablation cell and was mixed with argon (~0.9 L/min) via a T-connector before entering the ICP torch. Natural-pyrite Wenshan (δ34/32S= +1.1‰ V-CDT) and chalcopyrite GBW07268 (δ34/32S= -0.1‰, from the National Research Center for Geoanalysis, China) were used as external bracketing standards every fourth analysis. Fine-grained-sphalerite NBS 123 (δ34/32S= +17.1‰, from the National Institute of Standards and Technology, U.S.) and chalcopyrite TC1725 (δ34/32S= +12.8‰) were treated as quality controls. The long-term reproducibility of δ34S is better than 0.5‰ (1 Standard Deviation). The work was completed at Nanjing FocuMS Technology Co. Ltd.
4 Results
4.1 Biogenic mineralogy
Biogenic structures often exhibit regularly shaped, anhedral mineral aggregates. Based on the mineral and aggregate patterns, we suggest that there are four types of potential biogenic structures (Table 1): tube structure, quasi-oncolite structure, quasi-stromatolite structure and globular structure. These structures are confined to specific section in the core part of the wall.
4.1.1 Tube structure
A certain amount of tube structure has been identified in chimney wall, which developed in the opal matrix. Within chimney wall, tube structures are associated with the quasi-oncolite and quasi-stromatolite structures (see the text below), with a distribution width of several hundred micrometers.
Based on the mineral filling and wall rupture of the tubes, tube structures can be categorized into three types. Tube I is characterized by a thin-walled tube composed of iron sulfides, which is hollow inside or filled with opal but not with sulfides (Figures 3b, c). Some Tube I structures have locally thickened walls due to the attachment of outward-growing pyrite laminates (Figure 3c). Tube II is an extension of Tube I where the wall is further thickened by the attachment of sulfide minerals and partially filled with a small number of sulfide aggregates (Figure 3d). Tube III, building on Tube II, shows further growth of the tube wall either inward or outward, with a noticeable presence of large sulfide aggregates inside (~100μm, Figures 3f, g). In some cases, it is clearly visible that chalcopyrite fills the tube from the outside in following wall rupture (Figure 3g).
4.1.2 Quasi-oncolite structure
Within the chimney wall, several quasi-oncolite structures was identified, exhibiting a close symbiotic relationship with tube structures. These quasi-oncolites bear a striking resemblance to oncolites found in carbonate rocks (Weiss, 1969), thus we refer to it as a quasi-oncolite structure. They are characterized by a sequence of bands that envelop an initial nucleus (Figure 4), facilitating a laminate growth pattern resembling microbially induced layered microbial rocks (Grotzinger and Al-Rawahi, 2014). The assemblage includes quasi-oncolites predominantly composed of pyrite (Figures 4a, b) as well as structures where chalcopyrite is the primary component (Figures 4c, d). At the heart of these quasi-oncolite structures lies a nucleus, which may consist of early-stage pyrite crystals (Figure 4a) or opal (Figures 4b–d). This nucleus is encased in a shell of irregular, concentrically banded layers, which alternate between light and dark layers. The light layers are chiefly composed of colloform sulfide minerals, whereas the dark layers are predominantly composed of amorphous silica with impurities. The resultant quasi-oncolite structures are notably large, with some reaching a maximum size more than 300μm.
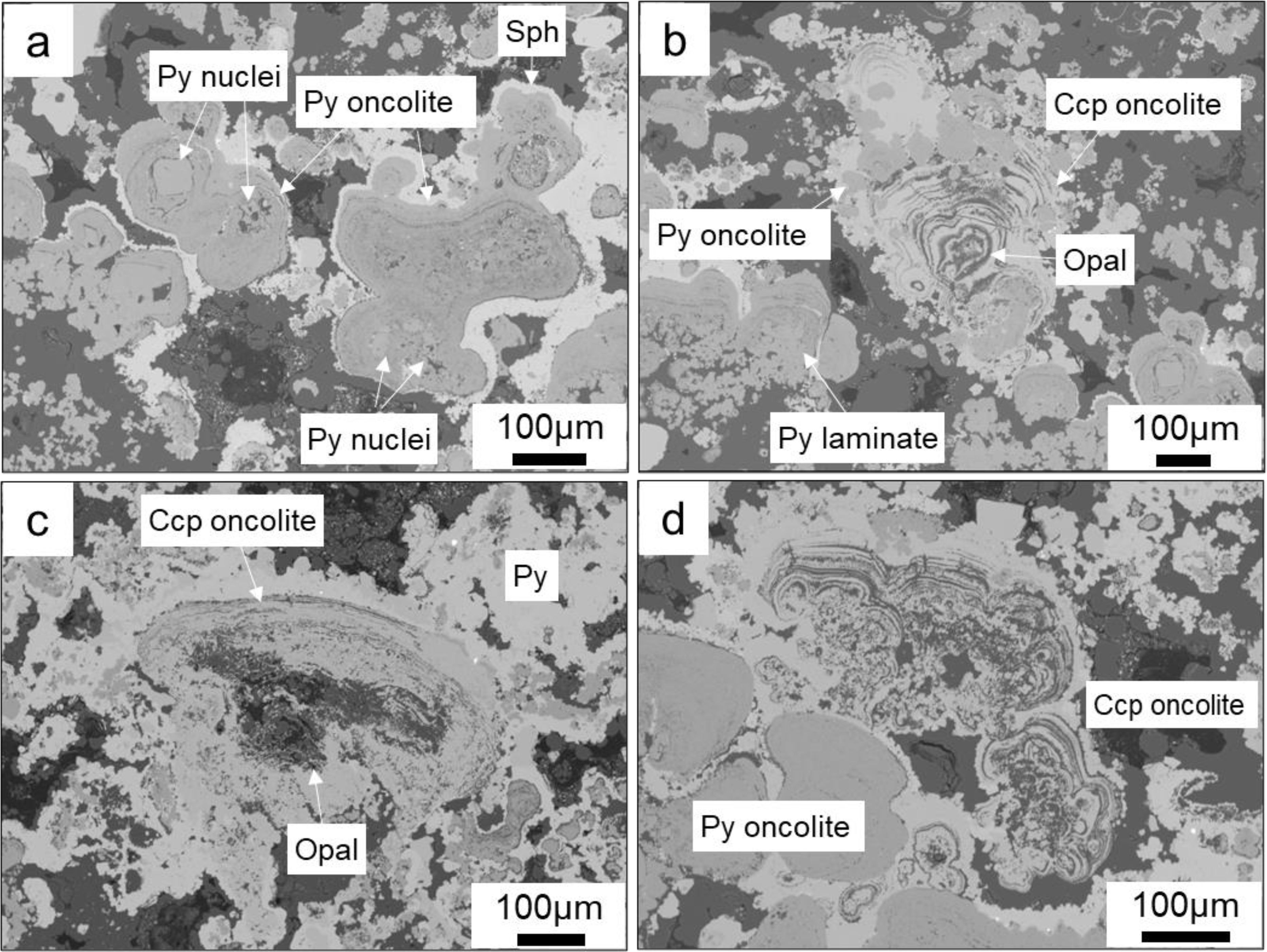
Figure 4. Backscatter electron microscopy of Quasi-oncolite structures within chimney wall. (a) Quasi-oncolite Structures with early pyrite crystals as the nuclei, generally formed by the fusion of multiple-nuclei closed pyrite laminates; (b–d). Quasi-oncolite structures with nuclei primarily composed of crystals containing amorphous silica. In (b), the chalcopyrite laminates encase a certain amount of small pyrite-dominated oncolites, while in (c, d), the closed laminates are more irregular in shape, and the oncolites are coated by later-stage pyrite.
4.1.3 Quasi-stromatolite structure
The quasi-stromatolite structure located within chimney wall exhibit a laminate like that of the quasi-oncolite structure, which is composed of alternating light and dark layers (Figure 5). The difference lies in the fact that the quasi-stromatolite structures develop on an early “basement”, growing along a pronounced dominant direction with varying heights of several tens of micrometers, rather than growing in concentric bands around a nucleus like the quasi-oncolite structure. This resembles the stromatolites associated with algal growth (Hohl and Viehmann, 2021), thus we refer to it as a quasi- stromatolite structure. The laminates are not closed. The “basement” colonized by the laminates consist of early-stage pyrite and chalcopyrite aggregates, or an early quasi-oncolite. The mineral composition of the laminates is the same as that of the quasi-oncolite structure.
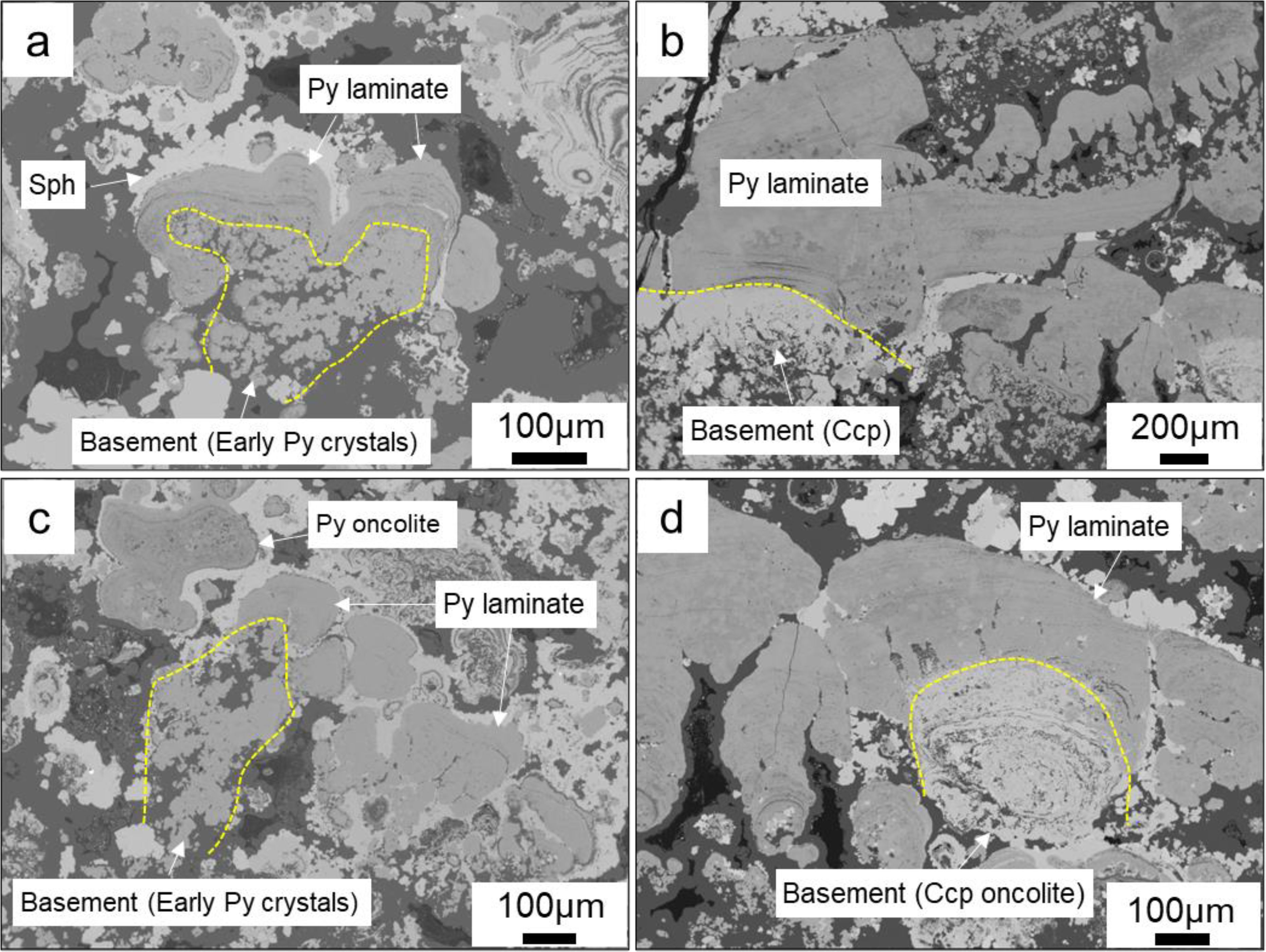
Figure 5. Backscatter electron microscopy of quasi-stromatolite structures within Chimney Wall. (a) Pyrite laminate growing on the basement of early subhedral pyrite aggregates, subsequently coated by colloform sphalerite; (b) Pyrite laminate stratifying growth on the basement of early anhedral chalcopyrite; (c) Pyrite laminate associated with pyrite oncolite; (d) Pyrite laminate growing on the basement of early chalcopyrite oncolite.
4.1.4 Globular structure
Based on the occurrence patterns, the globular pyrite can be classified into three types: (1) single globular, with diameters ranging from 10 to 40μm (Figures 6a–d), some of which appear relatively “dirty” due to the presence of impurities (Figures 6a–c); (2) serving as the nucleus for the growth of other mineral aggregates (Figures 6a, b), where later-stage colloform pyrite can grow in concentric bands around the early globular pyrite, forming central bands. Under the action of surface energy, multiple pyrite aggregates, each having globular pyrite at its nucleus, can combine to form larger aggregates (Sawlowicz, 1993). (Figure 6a). The later-stage colloform pyrite can also grow along a dominant direction to form a laminate (Figure 6b). Additionally, globular pyrite can be directly enveloped by later-stage sphalerite (Figure 6a); (3) globular pyrite aggregates (Figures 6c, d), formed by the direct aggregation of multiple globular pyrites (Figures 6c, d); locally, single globular pyrite can also adhere directly to the outer wall of the tubes (Figure 6d).
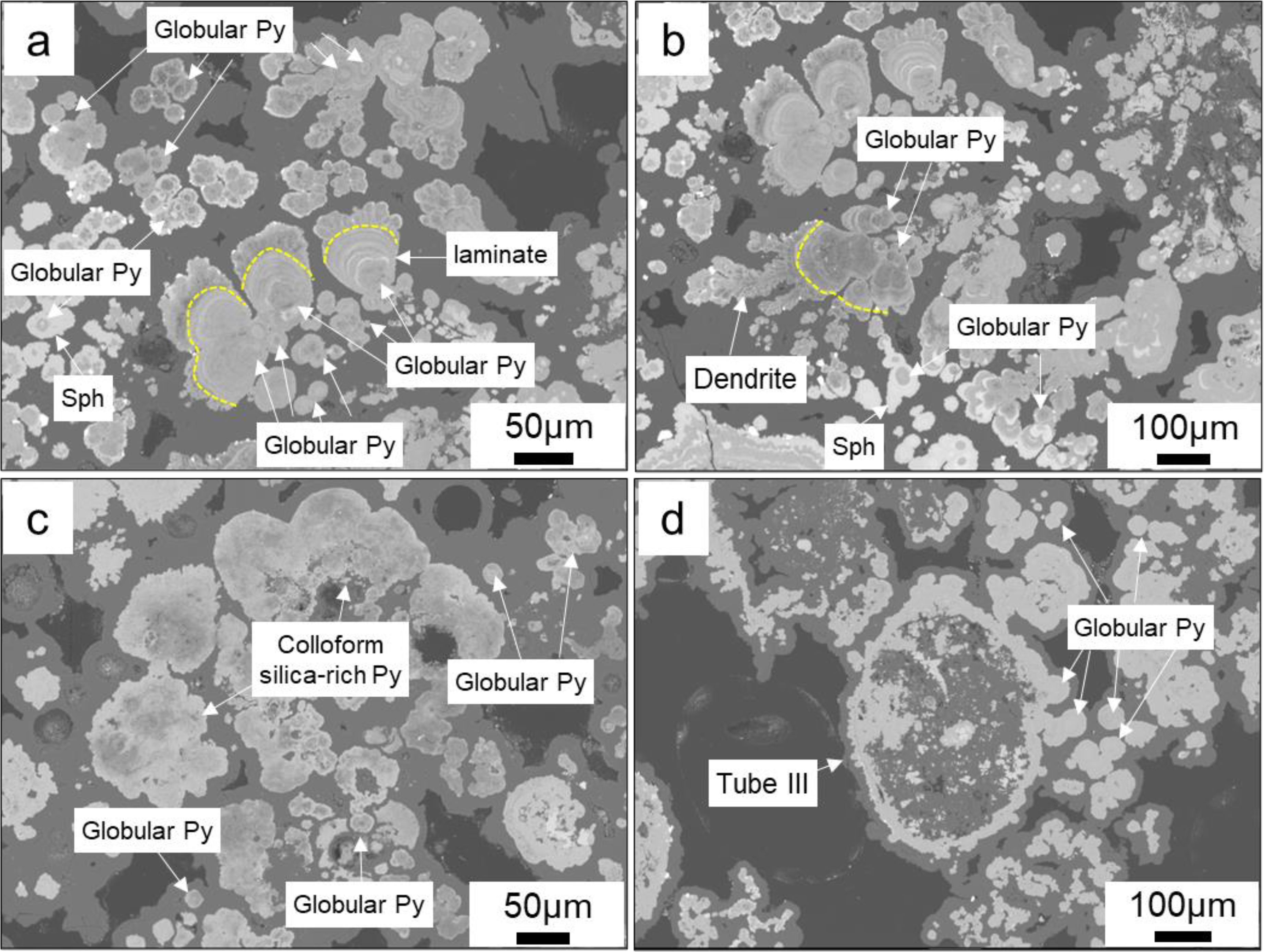
Figure 6. Backscatter electron microscopy of globular pyrites. (a, b) Globular pyrite acts as a nucleus for the subsequent growth of minerals, including pyrite laminate, aggregates of globular pyrite, and colloform sphalerite; (c) Individual globular pyrite and its aggregates, associated with colloform silica-rich pyrite; (d) Multiple larger globular pyrites (~30-40μm) form aggregates, and several globular pyrites can be seen adhering to the outer wall of Tube III.
4.2 Mineral chemistry
The chemical compositions of biogenic pyrite and chalcopyrite, as well as the compositions of abiotic pyrite and chalcopyrite that developed adjacent to those biogenic minerals, as determined by EPMA, are presented in Supplementary Tables 1-6. Their statistical values are summarized in Table 2.
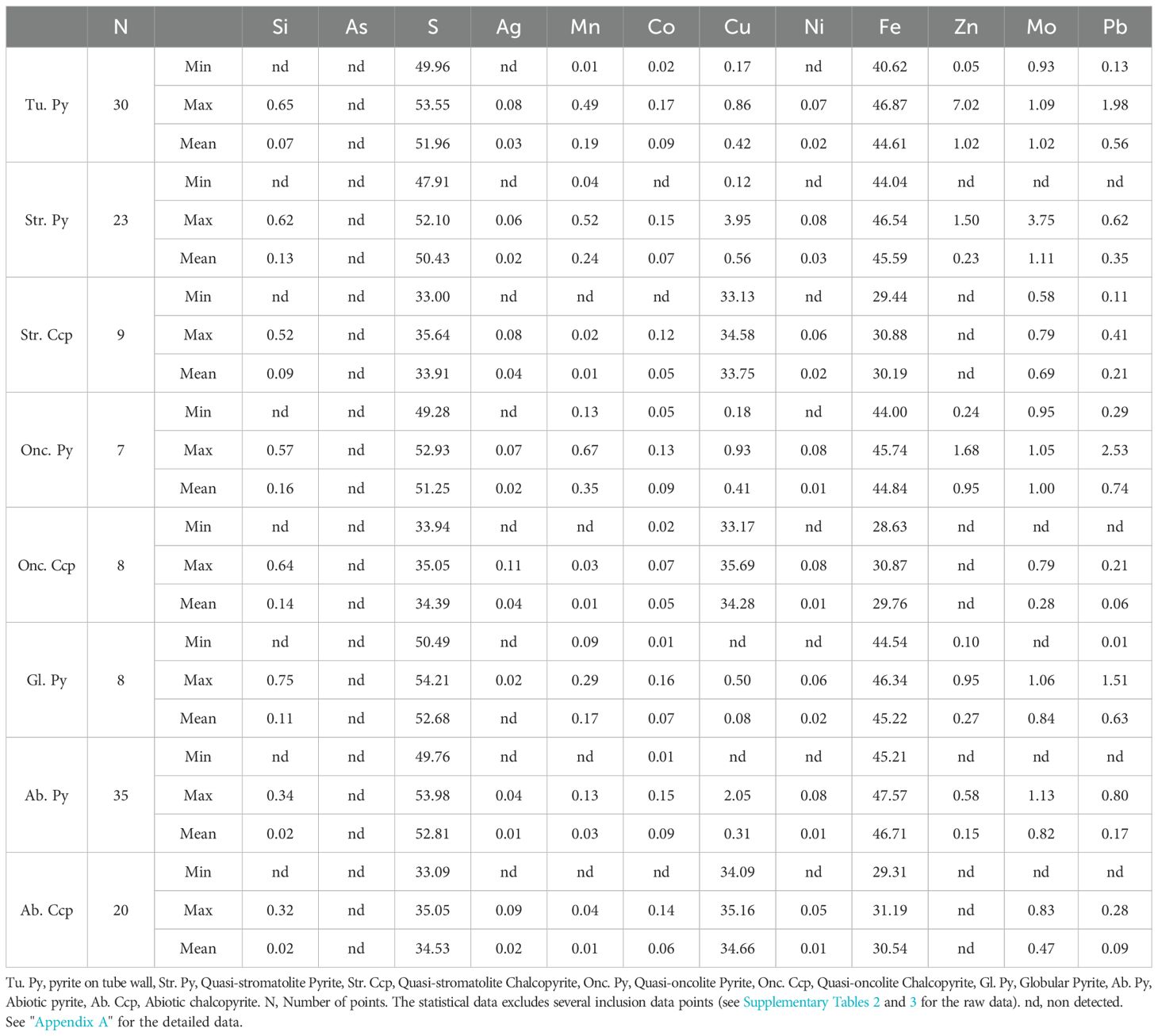
Table 2. Chemical compositions of four types of biogenic pyrite, 2 types of biogenic chalcopyrite, and abiotic pyrite and chalcopyrite as determined by EPMA analysis.
By comparing the four types of biogenic pyrite with abiotic pyrite, several significant differences in mineral element composition can be observed (Table 2; Figure 7): compared to the abiotic pyrite, which has a more concentrated content of Fe (46-48 wt%) and S (52-54 wt%), the major elements Fe and S in the four types of biogenic pyrite are all lower, and the content of Fe and S has a greater range of variation (Figure 7a). Although the major element contents are low, the trace element contents of Mn (with an average value ranging between 0.17-0.35 wt%) and Pb (with an average value ranging between 0.35-0.74 wt%) in the four types of biogenic pyrite are all higher than those in abiotic pyrite (Mn average 0.03 wt%, Pb average 0.17 wt%), and they have a larger range of variation, whereas the trace element variation range in abiotic pyrite is smaller (Figures 7b, c).
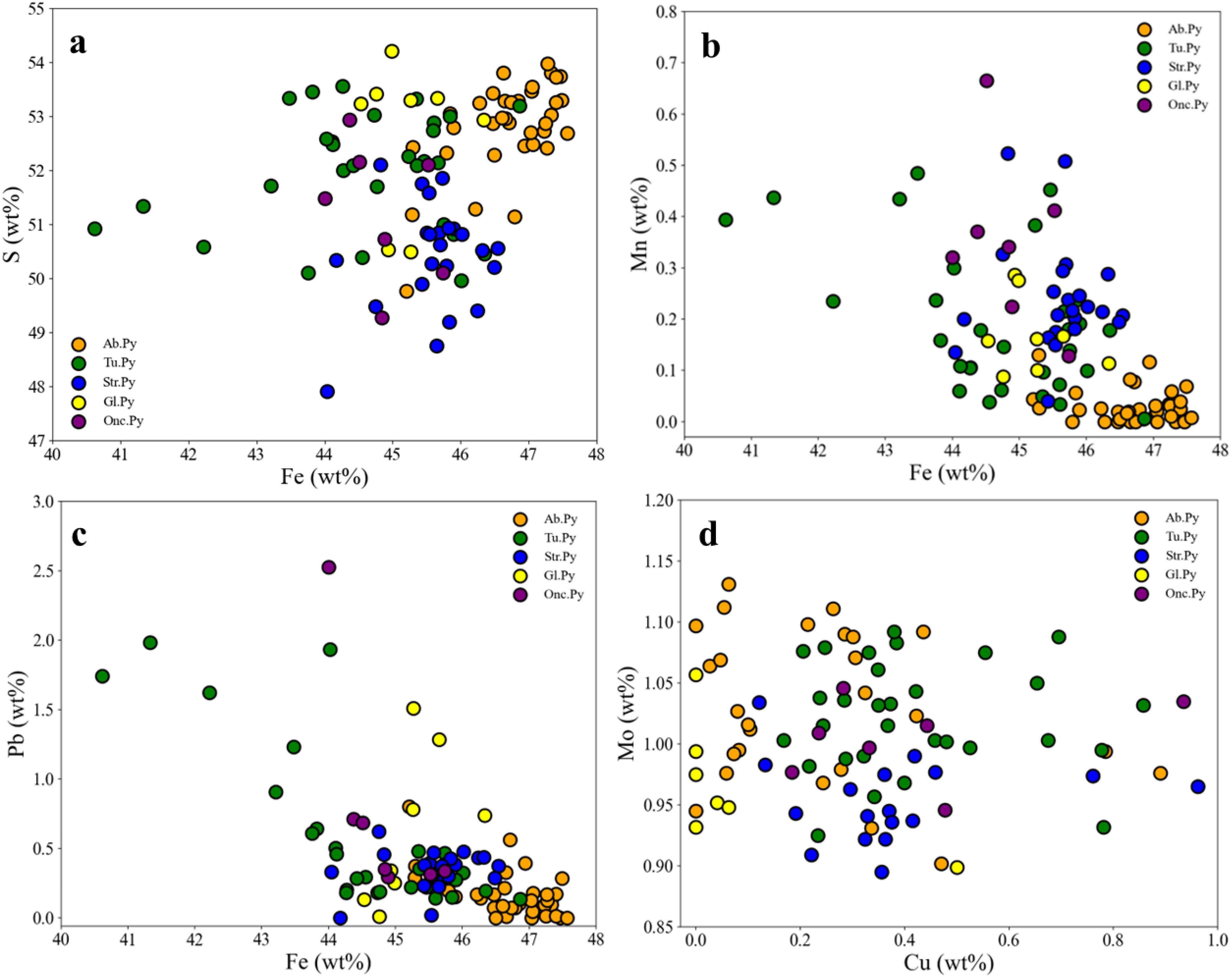
Figure 7. EPMA element correlations for (a) Fe-S, (b) Fe-Mn, (c) Fe-Pb, and (d) Cu-Mo in 5 types of pyrite, comprising 4 types of biogenic pyrite and 1 type of abiotic pyrite. The data for 2 inclusions within (Str. Py) are excluded from the d plot (see Supplementary Table 2). Tu. Py, pyrite on tube wall, Str. Py, Quasi-stromatolite Pyrite, Onc. Py, Quasi-oncolite Pyrite, Gl. Py, Globular Pyrite, Ab. Py, Abiotic pyrite.
The difference in Mo content between biogenic and abiotic pyrites is not significant (Figure 7d), mostly ranging between 0.9-1.1 wt%. The average Mo content in biogenic pyrite increases, which is related to inclusions reaching up to 4 wt% locally (Supplementary Table 2). In addition, although the Zn content in biogenic pyrite is also higher than that in abiotic pyrite, this increase is related to the replacement of biogenic pyrite by later sphalerite (Figures 3f, 5a, 6a, b). Moreover, except for globular pyrite, the Cu content in other biogenic pyrites is also significantly higher than that in abiotic pyrite.
Compared to abiotic chalcopyrite, the two types of biogenic chalcopyrite (quasi-stromatolite and quasi-oncolite chalcopyrite) both have relatively slightly lower contents of Fe, Cu, and S (Table 2), and they exhibit a relatively larger range of variation (Figures 8a, b). In terms of trace elements, individual biogenic chalcopyrite crystals have higher Zn contents due to the presence of sphalerite inclusions (Supplementary Tables 2&3), while the rest of the biogenic chalcopyrite crystals and abiotic chalcopyrite do not contain Zn. Additionally, except for the quasi-stromatolite chalcopyrite, which has a relatively slightly higher Pb content (Figure 8c), there are no significant differences in other trace elements.
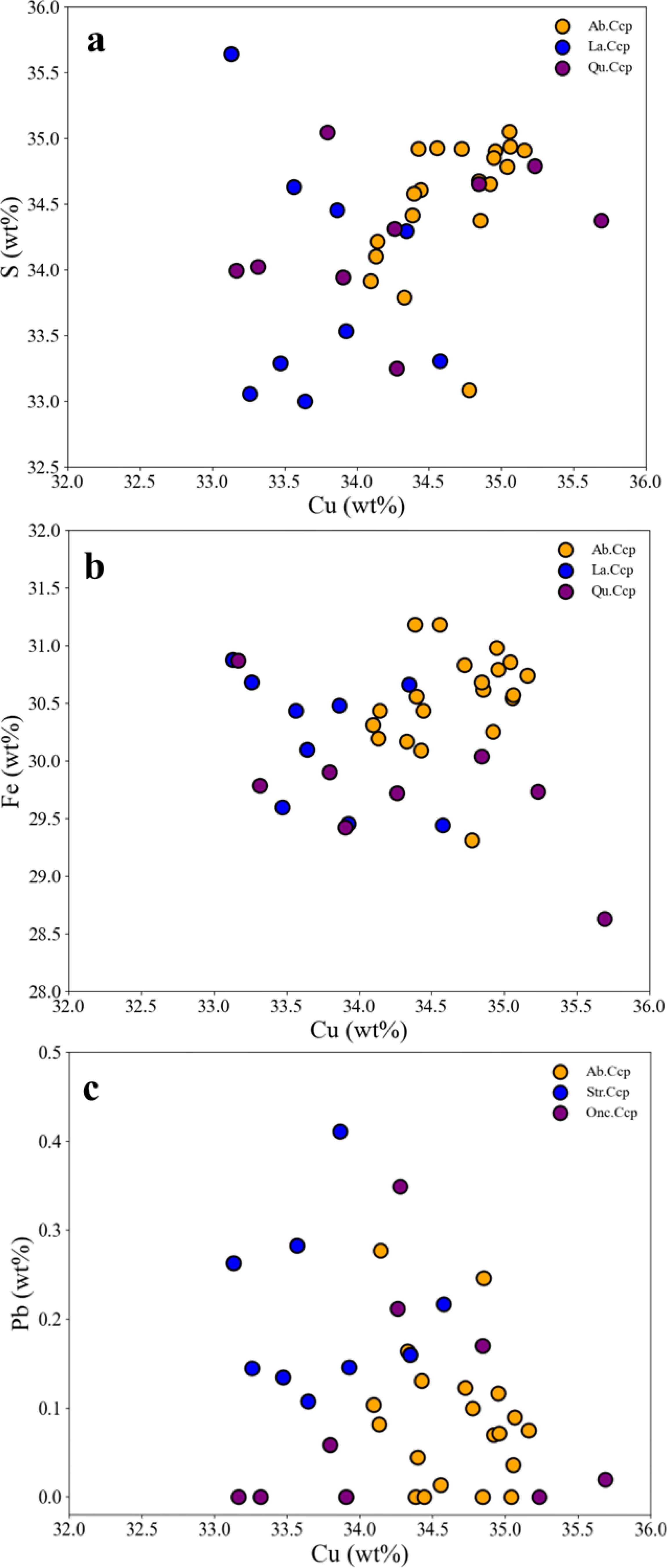
Figure 8. EPMA element correlations for Cu-S, Cu-Fe, Cu-Pb in 3 types of chalcopyrite, comprising 2 types of biogenic chalcopyrite and 1 type of abiotic chalcopyrite. In biogenic chalcopyrite, data for several inclusions containing sphalerite are excluded. Str. Ccp, Quasi-stromatolite Chalcopyrite, Onc. Ccp, Quasi-oncolite Chalcopyrite, Ab. Ccp, Abiotic Chalcopyrite.
4.3 In-situ sulfur isotope
A total of 37 in-situ sulfur isotopes were conducted on sulfide minerals, including 29 samples of biogenic/abiotic pyrite, as well as 8 samples of biogenic/abiotic chalcopyrite. The results are presented in Table 3 and illustrated in Figure 9. For the biogenic minerals, the δ34S values of 3 pyrite spots on the tube wall range from 2.8-4.1‰, those of 4 pyrite spots from the laminate of the quasi-oncolite structure range from 2.0-3.5‰, and the δ34S values of 2 pyrite spots from the laminate of the quasi-stromatolite structure are 3.4‰ and 3.7‰, respectively. Additionally, the δ34S values of 13 globular pyrite spots range from 1.3-2.8‰, and those of 6 chalcopyrite spots from the Quasi-oncolite structure range from 2.6-3.6‰. For the abiotic minerals, the δ34S values of 7 granular pyrite spots range from 4.0-5.3‰, and both granular chalcopyrite spots have a δ34S value of 4.2‰.
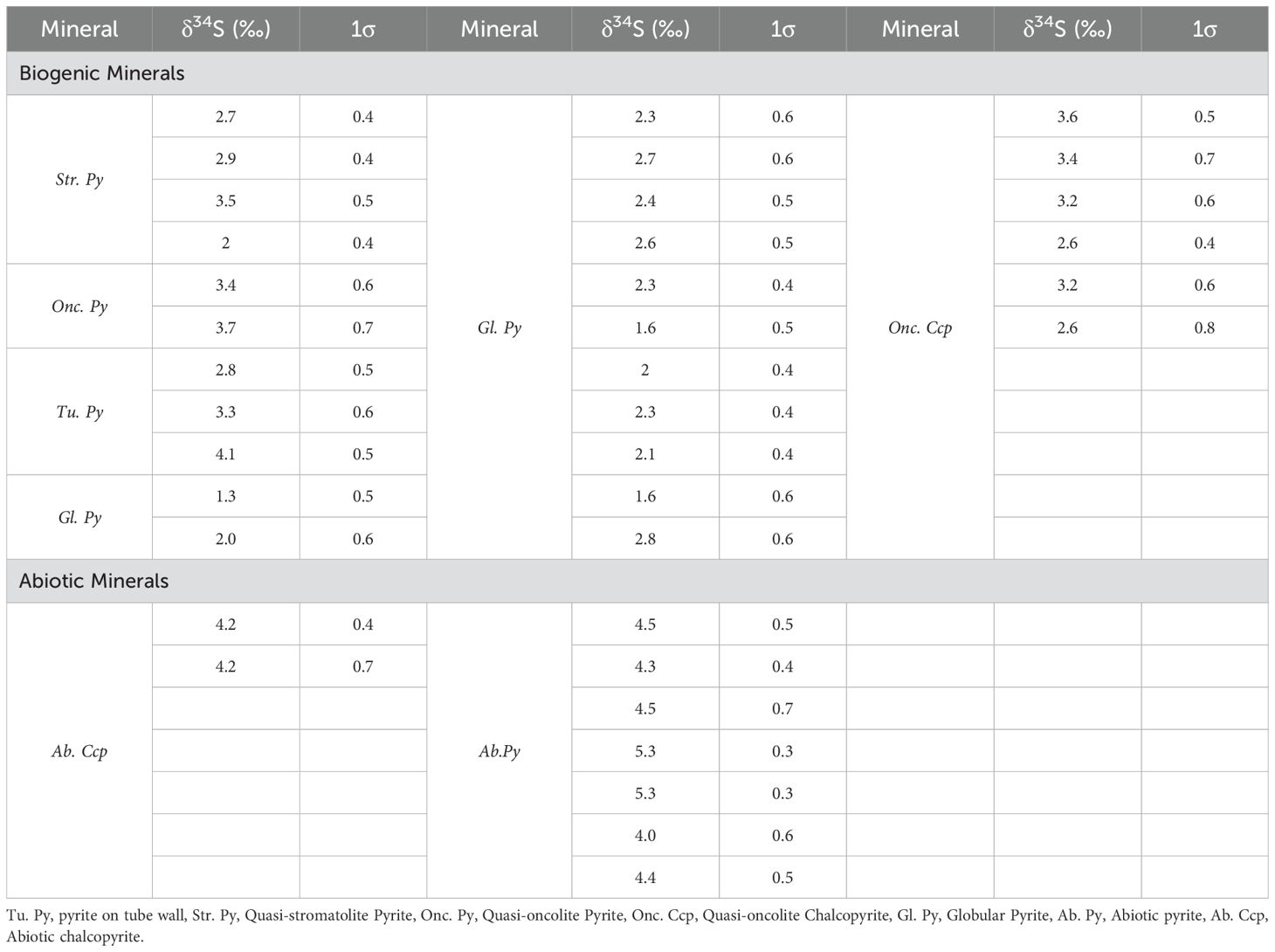
Table 3. In-situ sulfur isotopic compositions of sulfide biogenic/abiotic minerals in the Tongguan hydrothermal field.
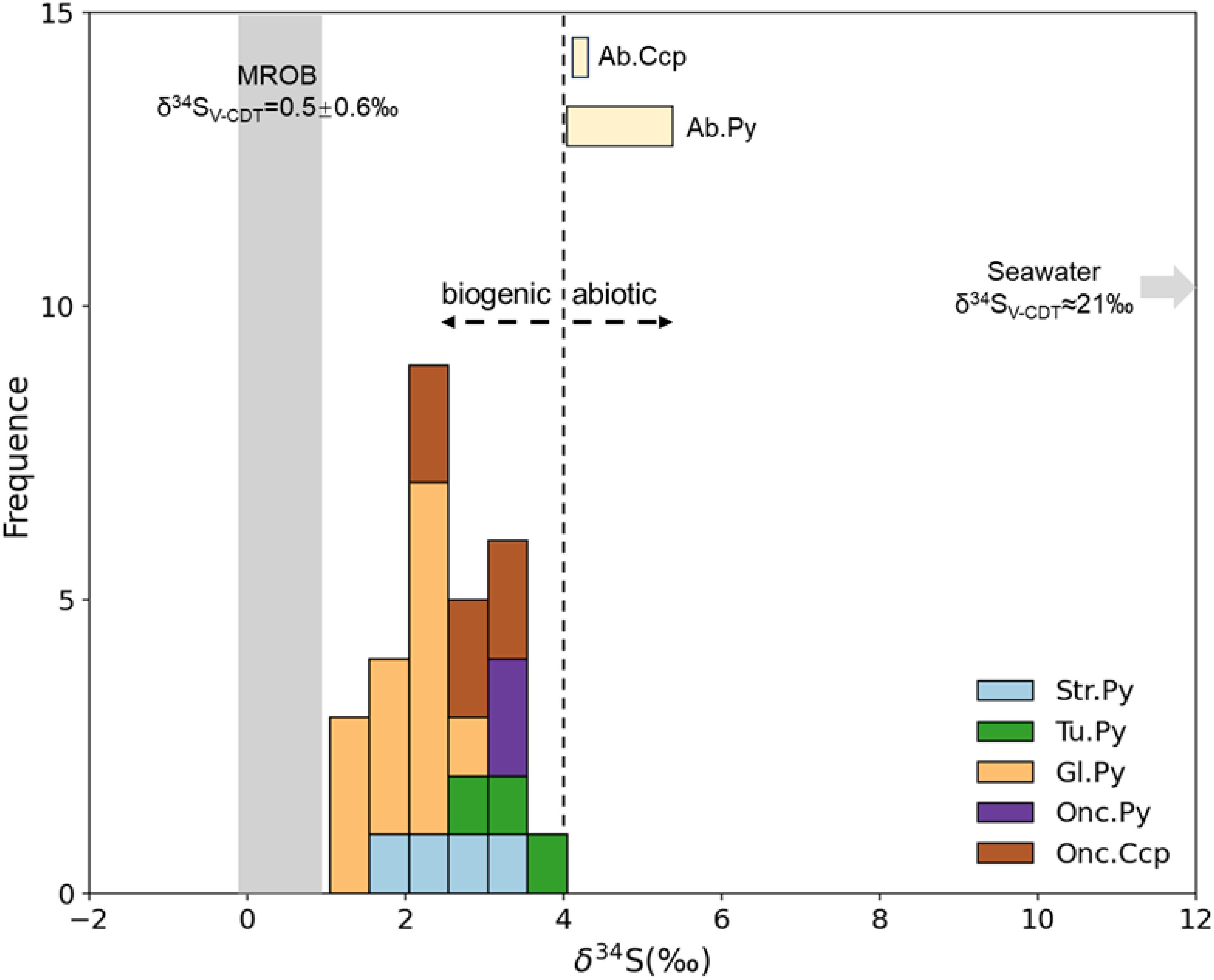
Figure 9. Frequency distribution of δ34S values for different sulfides from the Tongguan hydrothermal field. Tu. Py, pyrite on tube wall, Str. Py, Quasi-stromatolite Pyrite, Onc. Py, Quasi-oncolite Pyrite, Onc. Ccp, Quasi-oncolite Chalcopyrite, Gl. Py, Globular Pyrite, Ab. Py, Abiotic pyrite, Ab. Ccp, Abiotic chalcopyrite. Data sources: Seawater (Rees et al., 1978), sulfides in MORB (Sakai et al., 1984).
5 Discussion
5.1 Macrobial sulfide mineralization
The tube structures developed in the chimney wall are regarded as biogenic structures related to the activities of macro-organisms in hydrothermal fields, specifically annelida, including vestimentiferans, annelid worms, and polychaete worms (Juniper et al., 1986; Cook and Stakes, 1995), reflecting the participation of macro-organisms in the mineralization process. The presence of tube structures in different ridge hydrothermal sulfide deposits and ancient Volcanic Hosted Massive Sulfide (VHMS) has been commonly reported previously (e.g., Hannington and Scott, 1988; Cook and Stakes, 1995; Little et al., 1997; Georgieva et al., 2018).
Annelida are widely found in various seafloor hydrothermal systems (Black et al., 1998; Pedersen et al., 2010; Bright et al., 2024) and their presence is closely associated with chemosynthetic bacteria in hydrothermal fields as primary producers (Georgieva et al., 2018). Annelida form external coatings by secreting mucus layers, which passively adsorb and capture metal ions, precipitating on the outer wall to form metal layers, representing an important type of macrobial biomineralization (Hannington et al., 1995; Park and Damien, 2021). Mineralogical chemical analysis shows that the pyrite in the tube walls of this field has significantly higher Mn, Pb and Cu contents than abiotic pyrite, indicating that the pyrite precipitated during the macrobial biomineralization process selectively enriches elements.
Cook and Stakes (1995) proposed the petrological evolution of tube structures, which is divided into five different sequences, starting with low-temperature minerals (such as barite) and gradually transitioning to high-temperature minerals (such as chalcopyrite), representing the evolutionary process of the tube structures. The three evolutionary sequences of tubular structures identified in the Tongguan field (Tube I to Tube III) are consistent with the evolution proposed by Cook and Stakes (1995), which is from an initial low-temperature mineral stage to a subsequent thickening of the tube walls inward and outward, and gradual replacement by high-temperature minerals and the filling of the tube lumen with high-temperature phases. However, according to the evolutionary sequence of Cook and Stakes (1995), with further infilling and recrystallization, the tubes will eventually lose all or most of their layers. But in this field, such structures have not been observed. This indicates that the evolution of the tube structures in this field is incomplete, suggesting that the high- temperature stage in the later period of tube structure evolution was relatively short - lived. Instead, most tube structures in this field are eventually filled with abiotic low-temperature opal precipitates. Additionally, there are laminate structures locally adhering to the tube wall in Tube I (Figure 6b). The laminate structure is closely associated with the activities of microbes, indicating the coexistence of annelids and microorganisms. In addition, the conditions during the early stage of tube mineralization were also conducive to microbial activity.
5.2 Microbial sulfide mineralization
Bacteria and archaea are an essential part of modern hydrothermal vent communities and are the primary producers in these ecosystems, with their biomass far exceeding that of macro-organisms (Reysenbach et al., 2000; Sievert and Vetriani, 2012). In this field, the outer wall of the hydrothermal vent structure shows development of bacterial mats (Figure 2c). These microorganisms can participate in the hydrothermal mineralization process through various mechanisms (Zierenberg and Schiffman, 1990; Georgieva et al., 2018; Park and Damien, 2021). Unlike macrobial mineralization, which preserves the remains of individual organisms, microbial mineralization exhibits more complex structures. Similar to microbial rocks such as stromatolites and oncolites in sedimentary rocks (Reid et al., 2000; Grotzinger and Al-Rawahi, 2014; Nutman et al., 2016), as well as seabed biogenic polymetallic nodules and ferromanganese crusts with similar structural features (e.g. Wang and Müller, 2009; Zhao et al., 2014; Jiang et al., 2017), we propose that the quasi-stromatolite and quasi-oncolite structures in this field are of microbial origin. However, their formation mechanisms differ.
5.2.1 Laminate pyrite/chalcopyrite
Comparing the quasi-stromatolite and quasi-oncolite structures, apart from the difference between one having a “basement” and the other having a nucleus, they are highly similar in terms of mineral type and composition: both exhibit laminate structures composed of light and dark layers, with the thickness of individual laminae being essentially uniform. Additionally, the pyrite composition that forms the laminate and their δ34S values are also quite similar. The morphological characteristics of this laminate are strikingly like those found in carbonate stromatolites, oncolites and seabed ferromanganese crusts (e.g. Reid et al., 2000; Jiang et al., 2017).
For stromatolites and oncolites, the laminate is formed through the growth and metabolic activities of microbial mats, with the secretion of metabolic by-products by microbial communities inducing mineral precipitation, which is a type of microbially-induced mechanism (Park and Damien, 2021). In seafloor hydrothermal fields, especially those involving low-temperature diffuse fluids, extensive biofilm-forming bacterial mats composed of single-celled and filamentous bacteria are developed, utilizing dissolved reduced minerals (primarily sulfur compounds) or solid metal sulfide deposits emitted from the vents as their chemoautotrophic electron or energy source (Corliss et al., 1979). The bacterial mat resembles the microbial mat that grows in stromatolites, and the mineral precipitation induced by bacterial mats has also been reported in other hydrothermal fields (Corliss et al., 1979; Zierenberg and Schiffman, 1990).
From a mineral chemistry perspective, the laminate pyrite in this field is enriched in Mn, Pb, and Cu (Table 2 and Figure 7). Generally, when abiotic pyrite is enriched in Mn and Pb, it typically indicates a moderate to low-temperature, weakly reducing, or even slightly oxidizing environment. Conversely, when abiotic pyrite is enriched in Cu, it indicates a slightly higher temperature reducing environment (Maslennikov et al., 2009; Keith et al., 2016). The simultaneous enrichment of Mn, Pb, and Cu in pyrite is difficult to explain from an abiotic origin. Furthermore, other granular pyrite crystals in the same analyzed sample location, which share the same physicochemical conditions for precipitation as the laminate, do not enrich in Mn, Pb, and Cu, suggesting that the enrichment of trace elements in the laminate pyrite is not temperature-dependent. We suggest this is due to a bacterial mat-mediated process that selectively precipitates specific metals, as previous studies have shown that bacterial mats in certain hydrothermal fields can selectively enrich in Cu (Zierenberg and Schiffman, 1990).
Both the stromatolite-like and oncolite-like structures are rarely reported in previous studies of seafloor hydrothermal sulfides. From a mineralogical perspective, both structures coexist with the macrobial structure-tube structure in specific part of the chimney wall, indicating similar survival environments. Bacterial mats are likely to provide a food source for Annelida (Georgieva et al., 2018). The presence of a certain amount of laminate adhering to some tubes suggests that there is still bacterial mat activity on the already mineralized tube I, further indicating that the bacterial mats in this area have more extensive survival conditions than Annelida.
5.2.2 Globular pyrite
Despite the observation of abiotic globular pyrite formation under certain experimental conditions (Ohfuji and Rickard, 2005; Duverger et al., 2021), the prevailing view is still that globular pyrite under natural conditions is primarily associated with microbial activity (Piercey, 2015; Wacey et al., 2015; Nozaki et al., 2020; Truong et al., 2024). The evidence supporting a biogenic origin includes the selective enrichment of certain elements in pyrite, the presence of a certain amount of organic matter, possible microbial fossils and so on.
In this study, globular pyrite exhibits a relatively “dirty” characteristic (Figure 6), indicating the presence of organic matter. Given that organic matter is necessarily a product of either microbial metabolism or the microbial itself, the association of globular pyrite with organic matter strongly suggests a biogenic origin. As discussed by Duverger et al. (2021), the formation of globular pyrite is closely linked to the presence of organic matter. Biogenic globular pyrite biofilms contain a wealth of organic compounds that may facilitate the formation of these pyrite aggregates. This is reminiscent of framboidal pyrite, which is also biogenic but forms through the aggregation of numerous small pyrite crystals (Sawlowicz, 1993). In comparison to abiotic pyrite, globular pyrite is enriched in Mn and Pb, but not Cu relative to laminar pyrite, suggesting a selective enrichment of certain elements. However, this selective enrichment does not entirely correspond to that observed in bacterial mats within laminar pyrite.
Unlike framboidal pyrite, globular pyrite does not exhibit highly negative δ34S values (Sim et al., 2011; Nozaki et al., 2020, down to ~-60‰), but rather has slightly lower δ34S values compared to other biogenic pyrite types and a smaller sulfur isotope fractionation of ~4‰ compared to abiotic pyrite. This indicates that the pyrite biofilm in globular pyrite has not undergone significant microbial sulfate reduction. According to Canfield (2001), lower sulfur isotope fractionation generally corresponds to assimilatory sulfate reduction, where microorganisms convert seawater sulfate (SO42-) into sulfide (S2-) and incorporate it into cellular organic sulfur compounds. In this process, because the sulfate transport is unidirectional, no significant sulfur isotope fractionation is observed. Therefore, we believe that the microbial metabolic process related to assimilatory sulfate reduction is the key factor leading to the formation of globular pyrite in this field.
5.3 Models
For decades, biological activities have been considered to play a non-negligible role in the formation of submarine hydrothermal sulfide deposits (Juniper and Fouquet, 1988; Hannington et al., 1995; Piercey, 2015; Nozaki et al., 2020). Our research further confirms this perspective. However, biogenic minerals are confined to specific locations within chimney structure, rather than throughout the entire sample, indicating that biological activities are subject to strict environmental constraints. From the perspective of mineral paragenesis (Figures 3–6), the laminate structure exhibits characteristics of coexistence with both the tube and globular structures, and the globular structure also shows coexistence with the Tube structure. The bacterial mats associated with the laminate generally require low-temperature hydrothermal conditions (Winn et al., 1986; Zierenberg and Schiffman, 1990), indicating that microbial mineralization in this area primarily occurs at low temperatures.
In this study, three mechanisms by which hydrothermal organisms may facilitate mineral precipitation have been identified (Figure 10). For the Tube structure, as previously revealed by research (Cook and Stakes, 1995; Hannington et al., 1995), this is an “active” mineralization process mediated by large organisms such as polychaete worms. These organisms secrete mucous layers, which, through adsorption or complexation with soluble species in the organic matrix, are subsequently replaced and mineralized to form sulfide precipitation layers. For the laminate structure, as discussed in other hydrothermal fields (e.g., Lilliput, Dekov et al., 2010; PACMANUS, Zeng et al., 2012), we propose that this is a “passive” mineralization process associated with biofilm-forming microbial mats. The metabolic products of these mats provide nucleation surfaces for hydrothermal minerals and promote their precipitation, forming amorphous silica and sulfide mineral layers covering the bacterial layer. The globular pyrite is generally considered to be characteristic of biological sulfate reduction products (Piercey, 2015). Although the globular pyrite having lower δ34S values than abiotic pyrite, exhibits much less sulfur isotope fractionation compared to other biogenically reduced pyrites (e.g. Nozaki et al., 2020; Ding et al., 2021). We suggest that this is a microbial assimilatory sulfate reduction process rather than dissimilatory sulfate reduction (Canfield, 2001), which results in only moderate sulfur isotope fractionation. Moreover, the microbial biofilm may be key to the aggregation of pyrite into a regular globular shape.
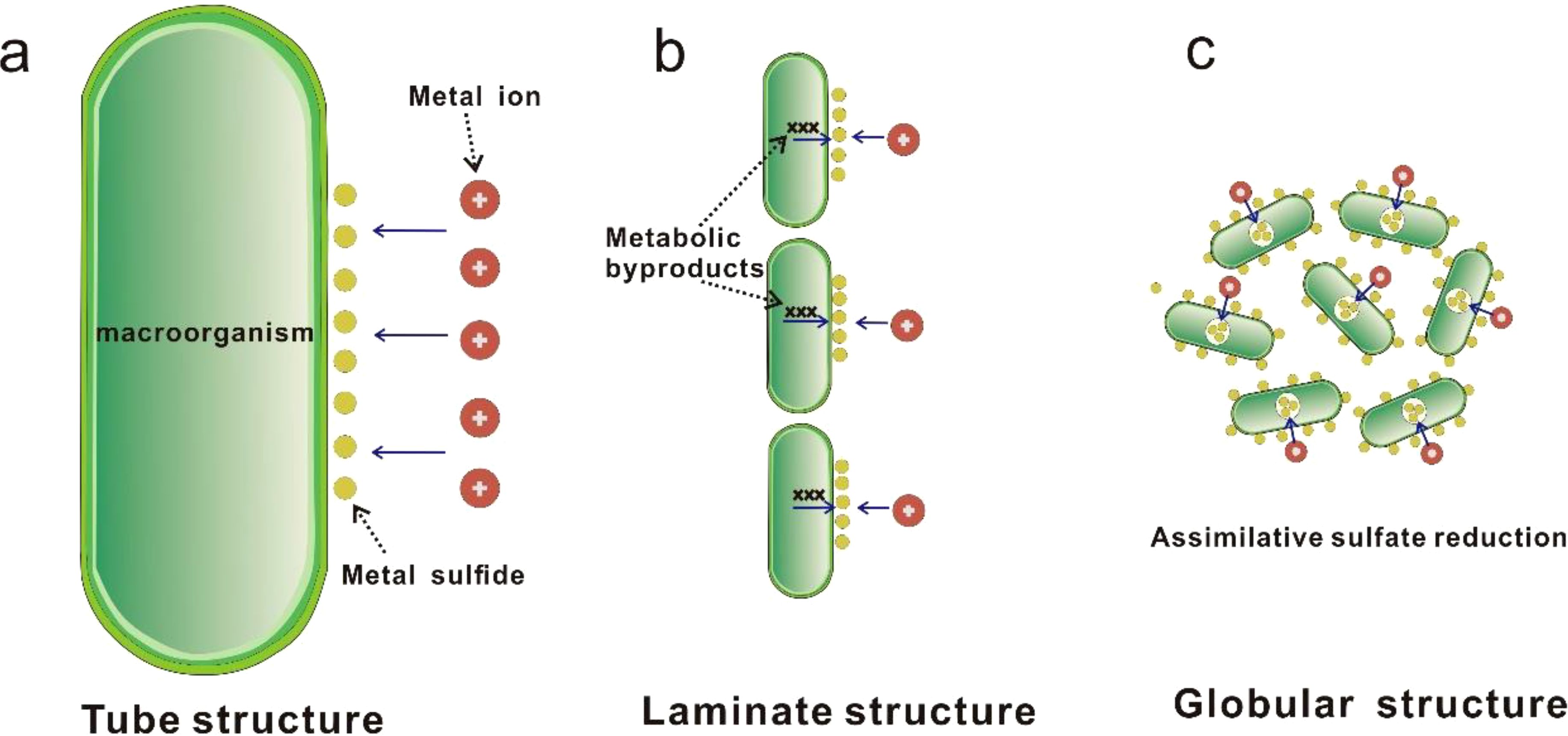
Figure 10. Schematic illustration of three types of biomineralization in the studied Tongguan sulfide samples (modified after Park and Damien, 2021). (a) The "active" mediation by macro-organisms, represented by polychaete worms, which involves the secretion of a mucous layer to capture metals from environment and subsequently precipitate them in the form of sulfides. (b) The "active" mediation by micro-organisms, where microorganism metabolic byproducts excreted by microorganisms provide nucleation surfaces for hydrothermal minerals and promote their precipitation, forming a laminate structure along the surface of a microbial mat composed of numerous microorganisms. (c) Formation of pyrite spherules through mixing of biogenic pyrite derived from biotic assimilative sulfate reduction reactions and adsorption of biofilms.
Whether it is macrobial or microbial mineralization, the selective adsorption of specific elements is a common feature of biogenic mineralization in this field, particularly in the case of pyrite. This widespread mediation by biofilms has led to increased concentrations of elements such as Mn and Pb. We speculate that the potential hydrothermal biotic mediation process selectively precipitates specific elements, which may contribute to the enrichment of certain metals in certain submarine hydrothermal base metal sulfide deposits.
6 Conclusions
This study investigated chimney sample from the volcanic-hosted Tongguan hydrothermal field on the SMAR and presented several lines of evidence for Biogeochemical mineralization. Four types of biogenic structures were proposed: macrobiotic tube structure, microbial mat-related stromatolite- and oncolite-like structures, and globular structure. The pyrite within these biogenic structures exhibited selective enrichment of elements such as Mn, Pb, and Cu compared to abiogenic pyrite. In-situ sulfur isotope studies revealed that biogenic minerals possess lower δ34S values than abiogenic minerals.
Both macro-organisms and microorganisms are involved in the mineralization process, facilitating mineral precipitation through different mechanisms. Three mechanisms by which hydrothermal organisms may facilitate mineral precipitation were identified: an “active” mineralization process mediated by large organisms like polychaete worms, a “passive” mineralization process associated with microbial mats, and a microbial assimilatory sulfate reduction process. Macrobial mineralization can coexist with microbial mineralization, which also needs attention.
Data availability statement
The original contributions presented in the study are included in the article/Supplementary Material. Further inquiries can be directed to the corresponding authors.
Author contributions
BL: Data curation, Formal Analysis, Investigation, Methodology, Writing – original draft. XS: Funding acquisition, Project administration, Resources, Supervision, Writing – review & editing. CL: Funding acquisition, Project administration, Resources, Writing – review & editing. SW: Methodology, Writing – review & editing. JY: Writing – review & editing. QY: Funding acquisition, Writing – review & editing. YD: Methodology, Writing – review & editing. XF: Methodology, Writing – review & editing.
Funding
The author(s) declare that financial support was received for the research and/or publication of this article. This paper is financially supported by the National Key Research and Development Program of China (No.2024YFC2815200 and No.2022YFC2803800), the China Ocean Mineral Resources R&D Association project (Grant No. DY135-S2-2-06), Laoshan Laboratory (Grant Nos. LSKJ202203601, LSKJ202204103) and Taishan Scholar Program of Shandong province.
Acknowledgments
We thank all the crews and scientists of the 33rd and 83rd ocean expedition of China.
Conflict of interest
The authors declare that the research was conducted in the absence of any commercial or financial relationships that could be construed as a potential conflict of interest.
Generative AI statement
The author(s) declare that no Generative AI was used in the creation of this manuscript.
Publisher’s note
All claims expressed in this article are solely those of the authors and do not necessarily represent those of their affiliated organizations, or those of the publisher, the editors and the reviewers. Any product that may be evaluated in this article, or claim that may be made by its manufacturer, is not guaranteed or endorsed by the publisher.
Supplementary material
The Supplementary Material for this article can be found online at: https://www.frontiersin.org/articles/10.3389/fmars.2025.1562763/full#supplementary-material
Supplementary Table 1 | Chemical compositions of pyrite on tube wall as determined by EPMA analysis. Tu. Py, pyrite on tube worm wall. nd, non detected.
Supplementary Table 2 | Chemical compositions of laminate pyrite and chalcopyrite as determined by EPMA analysis. La. Py, Laminate Pyrite; La. Ccp, Laminate Chalcopyrite. nd, non detected.
Supplementary Table 3 | Chemical compositions of quasi-oncolite pyrite and chalcopyrite as determined by EPMA analysis. Qu. Py, Quasi-oncolite Pyrite; Qu. Ccp, Quasi-oncolite Chalcopyrite. nd, non detected.
Supplementary Table 4 | Chemical compositions of framboidal pyrite as determined by EPMA analysis. Fr. Py, Framboidal Pyrite. nd, non detected.
Supplementary Table 5 | Chemical compositions of abiogenic pyrite in the vicinity of biogenic minerals as determined by EPMA analysis. Ab. Py, Abiogenic pyrite. nd, non detected.
Supplementary Table 6 | Chemical compositions of abiogenic chalcopyrite in the vicinity of biogenic minerals as determined by EPMA analysis Ab. Ccp, Abiogenic chalcopyrite. nd, non detected.
References
Amend J. P., McCollom T. M., Hentscher M., Bach W. (2011). Catabolic and anabolic energy for chemolithoautotrophs in deep-sea hydrothermal systems hosted in different rock types. Geochim. Cosmochim. Acta 75, 5736–5748. doi: 10.1016/j.gca.2011.07.041
Black M. B., Trivedi A., Maas P. A. Y., Vrijenhoek R. C. (1998). Population genetics and biogeography of vestimentiferan tube worms. Deep-Sea Res. 45, 365–382. doi: 10.1016/S0967-0645(97)00076-3
Bright M., Gollner S., de Oliveira A. L., Espada-Hinojosa S, Fulford A, Hughes IV, et al. (2024). Animal life in the shallow subseafloor crust at deep-sea hydrothermal vents. Nat. Commun. 15, 8466. doi: 10.1038/s41467-024-52631-9
Canfield D. E. (2001). Biogeochemistry of sulfur isotopes. Rev. Mineralogy Geochemistry. 43, 607–636. doi: 10.2138/gsrmg.43.1.607
Cavanaugh C. M., Gardiner S. L., Jones M. L., Jannasch H. W., Waterbury J. B. (1981). Prokaryotic cells in the hydrothermal vent tube worm Riftia pachyptila Jones: possible chemoautotrophic symbionts. Science 213, 340–342. doi: 10.1126/science.213.4505.340
Cook T. L., Stakes D. S. (1995). Biogeological mineralization in deep-sea hydrothermal deposits. Science 267 (5206), 1975–1979. doi: 10.1126/science.267.5206.1975
Corliss J. B., Dymond J., Gordon L. I., Edmond J. M., von Herzen R. P., Ballard R. D., et al. (1979). Submarine thermal springs on the galapagos rift. Science 203, 1073–1083. doi: 10.1126/science.203.4385.1073
Dekov V. M., Petersen S., Garbe-Schönberg C.-D., Kamenov G. D., Perner M., Kuzmann E., et al. (2010). Fe–si-oxyhydroxide deposits at a slow-spreading centre with thickened oceanic crust: The lilliput hydrothermal field (9°33′S, mid-atlantic ridge). Chem. Geology 278 (3–4), 186–200.
Dick G. J. (2019). The microbiomes of deep-sea hydrothermal vents: distributed globally, shaped locally. Nat. Rev. Microbiol. 17, 271–283. doi: 10.1038/s41579-019-0160-2
Ding T., Tao C., Dias Á.A., Liang J., Chen J., Wu B., et al. (2021). Sulfur isotopic compositions of sulfides along the Southwest Indian Ridge: implications for mineralization in ultramafic rocks. Miner Deposita 56, 991–1006. doi: 10.1007/s00126-020-01025-0
Duverger A., Bernard S., Viennet J.-C., Miot J., Busigny V. (2021). Formation of pyrite spherules from mixtures of biogenic FeS and organic compounds during experimental diagenesis. Geochemistry Geophysics Geosystems 22, e2021GC010056. doi: 10.1029/2021GC010056
Fouquet Y., Cambon P., Etoubleau J., Charlou J. L., Ondréas H., Barriga F. J. A. S., et al. (2013). Geodiversity of hydrothermal processes along the mid-atlantic ridge and ultramafic-hosted mineralization: A new type of oceanic Cu-Zn-Co-Au volcanogenic massive sulfide deposit. Geophysical Monograph Ser. 188, 321–367. doi: 10.1029/2008GM000746
Früh-Green G., Kelley D., Lilley M., Cannat M., Chavagnac V., Baross J., et al. (2022). Diversity of magmatism, hydrothermal processes and microbial interactions at mid-ocean ridges. Nat. Rev. Earth Environ. 3, 852–871. doi: 10.1038/s43017-022-00364-y
Georgieva M. N., Little C. T. S., Bailey R. J., Ball A. D., Glover A. G. (2018). Microbial-tubeworm associations in a 440 million year old hydrothermal vent community. Proc. Biol. Sci. 285, 20182004. doi: 10.1098/rspb.2018.2004
Grotzinger J., Al-Rawahi Z. (2014). Depositional facies and platformarchitecture of microbialite-dominated carbonate reservoirs, Ediacaran–Cambrian Ara Group, Sultanate of Oman. AAPG Bull. 98, 1453–1494. doi: 10.1306/02271412063
Hannington M. D., Jonasson I. R., Herzig P. M., Petersen S. (1995). “Physical and chemical processes of seafloor mineralization at mid-ocean ridges,” in Seafloor Hydrothermal Systems: Physical, Chemical, Biological, and Geological Interactions. Eds. Humphris S. E., Zierenberg R. A., Mullineaux L. S., Thomson R. E. (Washington, DC: American Geophysical Union), 115–157.
Hannington M. D., Scott S. D. (1988). Mineralogy and geochemistry of a hydrothermal silica-sulfide-sulfate spire in the caldera of Axial Seamount, Juan De Fuca Ridge. Can. Mineralogist 26, 603–625.
Haymon R. M. (1983). Growth history of black smoker hydrothermal chimneys. Nature 301, 695–698. doi: 10.1038/301695a0
Hohl S. V., Viehmann S. (2021). Stromatolites as geochemical archives to reconstruct microbial habitats through deep time: Potential and pitfalls of novel radiogenic and stable isotope systems. Earth-Science Rev. 218, 103683. doi: 10.1016/j.earscirev.2021.103683
Humphris S. E., Zierenberg R. A., Mullineaux L. S., Thomson R. E. Eds. (1995). Seafloor Hydrothermal Systems: Physical, Chemical, Biological, and Geological Interactions. In: Geophysical Monograph. (Washington, DC: AGU). doi: 10.1029/GM091
Jiang X. D., Sun X. M., Guan Y., Gong J. L., Lu Y., Lu R. F., et al. (2017). Biomineralisation of the ferromanganese crusts in the Western Pacific Ocean. J. Asian Earth Sci. 136, 58–67. doi: 10.1016/j.jseaes.2017.01.025
Jonasson I. R., Walker D. A. (1987). Micro-organisms and their debris as substrates for metal sulfide nucleation and accumulation in some mid-ocean ridge deposits (abstract) EOS, Trans. AGU 68, 1546. doi: 10.1029/93JB02871
Juniper S. K., Fouquet Y. (1988). Filamentous iron-silica deposits from modern and ancient hydrothermal sites, Can. Mineral. 26, 859–869.
Juniper S. K., Jonasson I. R., Tunnicliffe V., Southward A. J. (1992). Influence of a tube-building polychaete on hydrothermal chimney minerali7ation. Geology 20, 895–898.
Juniper S. K., Thompson J. A. J., Calvert S. E. (1986). Accumulation of minerals and trace elements in biogenic mucus at hydrothermal vents, Deep Sea Research Part A. Oceanographic Res. Papers 33, 339–347. doi: 10.1016/0198-0149(86)90095-6
Keith M., Häckel F., Haase K. M., Schwarz-Schampera U., Klemd R. (2016). Trace element systematics of pyrite from submarine hydrothermal vents. Ore Geology Rev. 72, 728–745. doi: 10.1016/j.oregeorev.2015.07.012
Koski R. A., Jonasson I. R., Kadko D. C., Smith V. K., Wong F. L. (1994). Compositions, growth mechanisms, and temporal relations of hydrothermal sulfide-sulfate-silica chimneys at the northern Cleft segment, Juan de Fuca Ridge. J. Geophys. Res. 99, 4813. doi: 10.1029/93JB02871
Little C. T. S., Herrington R. J., Maslennikov V. V., Morris N. J., Zaykov V. V. (1997). Silurian hydrothermal-vent community from the southern Urals, Russia. Nature 385, 146–148. doi: 10.1038/385146a0
Maslennikov V. V., Maslennikova S. P., Large R. R., Danyushevsky L. V. (2009). Study of trace element zonation in vent chimneys from the silurian yaman-kasy volcanic-hosted massive sulfide deposit (Southern urals, Russia) using laser ablation-inductively coupled plasma mass spectrometry (LA-ICPMS). Economic Geology. 104, 1111–1141. doi: 10.2113/gsecongeo.104.8.1111
Nozaki T., Nagase T., Ushikubo T., Shimizu K., Ishibashi J.-i., the D/V Chikyu Expedition 909 Scientists (2020). Microbial sulfate reduction plays an important role at the initial stage of subseafloor sulfide mineralization. Geology 49, 222–227. doi: 10.1130/GEOL.S.12964949.v1
Nutman A. P., Bennett V. C., Friend C. R. L., Kranendonk M. J. V., Chivas A. R. (2016). Rapid emergence of life shown by discovery of 3,700-million-year-old microbial structures. Nature 537, 535–538. doi: 10.1038/nature19355
Ohfuji H., Rickard D. (2005). Experimental syntheses of framboids–a review. Earth-Science Rev. 71 (3–4), 147–170.
Park Y., Damien F. (2021). Diversity of microbial metal sulfide biomineralization. ChemPlusChem 87, e202100457. doi: 10.1002/cplu.202100457
Pedersen R. B., Rapp H. T., Thorseth I. H., Lilley M. D., Barriga F. J. A. S, Baumberger T., et al. (2010). Discovery of a black smoker vent field and vent fauna at the Arctic Mid-Ocean Ridge. Nat. Commun. 1, 126. doi: 10.1038/ncomms1124
Piercey S. J. (2015). A semipermeable interface model for the genesis of subseafloor replacement-type volcanogenic massive sulfide (VMS) deposits. Economic Geology. 110, 1655–1660. doi: 10.2113/econgeo.110.7.1655
Piercey S. J., Squires G., Brace T. (2018). Geology and lithogeochemistry of hydrothermal mudstones from the upper block near the Duck Pond volcanogenic massive sulfide (VMS) deposit, Newfoundland, Canada: evidence for low-temperature venting into oxygenated mid-Cambrian seawater. Miner Deposita 53, 1167–1191. doi: 10.1007/s00126-018-0795-3
Rees C. E., Jenkins W. J., Jan M. (1978). The sulphur isotopic composition of ocean water sulphate. Geochimica Cosmochimica Acta 42 (4), 377–381.
Reid R. P., Visscher P. T., Decho A. W., Stolz J. F., Bebout B. M., Dupraz C., et al. (2000). The role of microbes in accretion, lamination and early lithification of modern marine stromatolites. Nature 406, 989–992. doi: 10.1038/35023158
Reysenbach A., Banta A. B., Boone D. R., Cary S. C., Luther G. W. (2000). Microbial essentials at hydrothermal vents. Nature 404, 835. doi: 10.1038/35009029
Sakai H., Marais D. J. D., Ueda A., Moore J. G. (1984). Concentrations and isotope ratios of carbon, nitrogen and sulfur in ocean-floor basalts. Geochimica Cosmochimica Acta 48 (12), 2433–2441.
Sawlowicz Z. (1993). Pyrite framboids and their development: a new conceptual mechanism. Geol Rundsch 82, 148–156. doi: 10.1007/BF00563277
Schrenk M. O., Kelley D. S., Bolton S. A., Baross J. A. (2004). Low archaeal diversity linked to subseafloor geochemical processes at the Lost City hydrothermal field, Mid-Atlantic Ridge. Environ. Microbiol. 6, 1086–1095. doi: 10.1111/j.1462-2920.2004.00650.x
Sievert Stefan M., Vetriani C. (2012). Chemoautotrophy at deep-sea vents : past, present, and future. Oceanography 25, 218–233.
Sim M. S., Bosak T., Ono S. (2011). Large sulfur isotope fractionation does not require disproportionation. Science 333, 74–77. doi: 10.1126/science.1205103
Truong C., Bernard S., Gorlas A., Guyot F. (2024). Abiotic syntheses of pyrite: clues to assess the biogenicity of pyrite spherules. Geochem. Persp. Let. 32, 27–33. doi: 10.7185/geochemlet.2438
Wacey D., Kilburn M. R., Saunders M., Cliff J. B., Kong C., Liu A. G., et al. (2015). Uncovering framboidal pyrite biogenicity using nano-scale CNorg mapping. Geology 43 (1), 27–30. doi: 10.1130/G36048.1
Wang S., Li C., Li B., Dang Y., Ye J., Zhu Z., et al. (2022). Constraints on fluid evolution and growth processes of black smoker chimneys by pyrite geochemistry: A case study of the Tongguan hydrothermal field, South Mid-Atlantic Ridge. Ore Geology Rev. 140, 104410. doi: 10.1016/j.oregeorev.2021.104410
Wang X., Müller W. E. G. (2009). Marine biominerals: perspectives and challenges for polymetallic nodules and crusts. Trends Biotechnol. 27, 375–383. doi: 10.1016/j.tibtech.2009.03.004
Weiss M. P. (1969). Oncolites, paleoecology, and laramide tectonics, central utah. AAPG Bulletin. 53, 1105–1120. doi: 10.1306/5D25C81F-16C1-11D7-8645000102C1865D
Winn C., Karl D., Massoth G. (1986). Microorganisms in deep-sea hydrothermal plumes. Nature 320, 744–746. doi: 10.1038/320744a0
Zeng Z., Ouyang H., Yin X., Chen S., Wang X., Wu L. (2012). Formation of Fe–Si–Mn oxyhydroxides at the PACMANUS hydrothermal field, Eastern Manus Basin: Mineralogical and geochemical evidence. J. Asian Earth Sci. 60, 130–146. doi: 10.1016/j.jseaes.2012.08.009
Zhao J., Zhang G. H., Wu G., Lu B., Pulyaeva I. A., Zhang H., et al. (2014). Biomineralization of organic matter in cobalt-rich crusts from the Marcus-Wake Seamounts of the western Pacific Ocean. Acta Oceanologica Sin. 33, 67–74. doi: 10.1007/s13131-014-0552-0
Zierenberg R. A., Schiffman P. (1990). Microbial control of silver mineralization at a sea-floor hydrothermal site on the northern Gorda Ridge. Nature 348, 155–157. doi: 10.1038/348155a0
Appendix A. Supplementary data
Chemical compositions of abiotic and biogenic pyrite and chalcopyrite in the studied Tongguan samples as determined by EPMA analysis are given in Supplementary Tables 1-6.
Keywords: biogeochemical mineralization, southern Mid-Atlantic Ridge, sulfide, biogenic structure, mineral chemistry, in-situ sulfur isotope
Citation: Li B, Shi X, Li C, Wang S, Ye J, Yan Q, Dang Y and Fang X (2025) Biogeochemical sulfide mineralization in the volcanic-hosted Tongguan hydrothermal field, southern Mid-Atlantic Ridge. Front. Mar. Sci. 12:1562763. doi: 10.3389/fmars.2025.1562763
Received: 18 January 2025; Accepted: 10 March 2025;
Published: 07 April 2025.
Edited by:
Marta Plavsic, Rudjer Boskovic Institute, CroatiaReviewed by:
Sotirios Karavoltsos, National and Kapodistrian University of Athens, GreeceXingwei Meng, Ministry of Natural Resources, China
Copyright © 2025 Li, Shi, Li, Wang, Ye, Yan, Dang and Fang. This is an open-access article distributed under the terms of the Creative Commons Attribution License (CC BY). The use, distribution or reproduction in other forums is permitted, provided the original author(s) and the copyright owner(s) are credited and that the original publication in this journal is cited, in accordance with accepted academic practice. No use, distribution or reproduction is permitted which does not comply with these terms.
*Correspondence: Xuefa Shi, eGZzaGlAZmlvLm9yZy5jbg==; Chuanshun Li, bGljaHVhbnNodW5AZmlvLm9yZy5jbg==