- 1Sarasota Dolphin Research Program, Brookfield Zoo Chicago, c/o Mote Marine Laboratory, Sarasota, FL, United States
- 2National Marine Fisheries Service, Southeast Fisheries Science Center, Beaufort, NC, United States
- 3Dolphin Biology Research Institute, San Diego, CA, United States
- 4Dolphin Quest, Inc., San Diego, CA, United States
- 5Auburn University College of Veterinary Medicine, Auburn, AL, United States
- 6Stranding Investigations Program, Mote Marine Laboratory, Sarasota, FL, United States
- 7Department of Biology, Portland State University, Portland, OR, United States
- 8National Marine Mammal Foundation, San Diego, CA, United States
- 9Dolphin Biology Research Institute, Eugene, OR, United States
Studies of the resident community of bottlenose dolphins (Tursiops truncatus) of Sarasota Bay, Florida, have been conducted for more than 50 years. Detailed histories have been collected for resident individuals through integrated observations, systematic photographic identification surveys, tagging and tracking, catch-and-release health assessments, remote biopsy sampling, and stranding response. This has produced a unique dataset documenting life history milestones and vital rates of a small cetacean. Analyses of data from 482 resident Sarasota Bay dolphins have revealed estimated maximum life spans of 67 years for females and 52 years for males. For females, predicted age at sexual maturation is 8.5 years, with a predicted age at first reproduction of 9.6 years. Females were observed to give birth when 6-48 years of age, and have been documented with as many as 12 calves, with 45% observed post-separation. Ten percent of females were considered to be reproductively senescent, having gone >13 years without producing a calf. For males, predicted age at sexual maturation is 10 years. Males 10-43 years old sired calves, producing up to 7 calves each. The average calving interval was 3.5 years, albeit with effects due to mother’s age, birth order, and calf survival. Seasonal reproduction was evident, with 81% of births occurring during May-July. Mean annual birth rate was 0.071. Mean annual fecundity was 0.182 births/adult female (defined as females 6 yrs or older). Recruitment rate through reproduction was estimated to be 0.050 based on calves surviving their first year. Immigration was infrequent, with an estimated annual rate of 0.003-0.013. Estimated mean annual maximum loss rate, from mortality, emigration, and changed identification characteristics, was 0.072. Periods of increased loss rates were related to environmental events, and factors that may be important to long-term population resilience were suggested.
Introduction
Understanding the population dynamics of small cetaceans requires knowledge of their life history, reproductive, and demographic parameters, and how these parameters are affected by the animals’ environment. Acquiring the requisite information on these parameters is challenging for animals that spend their entire lives, spanning decades, in the marine environment, where they often are visible to researchers for only brief periods during surfacing, and exhibit relatively long calving intervals and longevity. Two approaches have been used to obtain these kinds of information - cross-sectional studies, for example, single observations of life history parameters made at death – and longitudinal studies, which track individuals through their lifetimes.
Historically, data on life history and demographics have come from stranded carcasses or from fisheries. Necropsies allow for the collection of cross-sectional data for morphometrics, sex determination, genetics, determination of maturity, reproductive status and history, and age estimation. Such data have been obtained at the population level from compilation of individual stranding records over time [e.g., Hohn (1980); Stolen and Barlow (2003) for bottlenose dolphins (Tursiops spp.)], or from mass strandings, where entire groups come ashore [e.g., Mead et al. (1980) for spinner dolphins (Stenella longirostris), Irvine et al. (1979) for short-finned pilot whales (Globicephala macrorhynchus)]. For stranded animals, the opportunistic nature of these samples, the condition of the carcass, and the poorly understood circumstances that led them to strand means that it can be unclear where the animals originated or what stock they represent. It can also be unclear in some cases if the factors leading to stranding may have affected the parameters of interest, making the animal less-than-representative of healthy conspecifics.
For many decades, targeted fisheries and incidental bycatch of small cetaceans have provided a source to gather important life history and demographic data. Researchers have been able to learn a great deal when allowed to collect and/or examine carcasses of small cetaceans recovered from fisheries targeting fish that occur in the same habitat. For example, much of what is known about the life history and demographic parameters of several dolphin species, including spinner dolphins (Perrin et al., 1977), pantropical spotted dolphins (S. attenuata, Hohn et al., 1985), and short-beaked common dolphins (Delphinus delphis, Danil and Chivers, 2007), results from large bycatch in the tuna purse-seine fishery in the eastern tropical Pacific Ocean over decades. Similarly, bycatch from gillnets operating in the Gulf of Maine during 1989-1993 provided the basis for a detailed description of harbor porpoise (Phocoena phocoena) life history (Read and Hohn, 1995). Japanese drive fisheries for short-finned pilot whales yielded detailed information on life history and reproductive biology, based on hundreds of carcasses examined (e.g., Kasuya and Marsh, 1984).
While studies of dead animals can provide much information that cannot be easily obtained from living animals in their natural environment, they can be limited to a single and final, cross-sectional data point in what can be a multi-decade lifespan. Research on dolphins under professional care has provided an intermediate source of data, with opportunities for monitoring individuals over the course of their lives, sometimes followed by collection of measurements and samples at the end of the animal’s life. Such data have been compiled, for example, for killer whales (Duffield et al., 1995) and bottlenose dolphins (Sergeant et al., 1973; Duffield and Robeck, 2000). Studies of reproductive seasonality of dolphins managed under human care have contributed to understanding wild dolphins, for example, spinner dolphins in Hawaii (Wells, 1984) and bottlenose dolphins (Urian et al., 1996). One potential drawback of such studies is that it is not known if or how unnatural environmental conditions may affect life history and reproductive parameters.
Beginning in the 1970s, field researchers began to fill some of the data gaps for small cetacean life history, reproductive biology, and demographic parameters through long-term observational studies of identifiable individuals in resident populations. Among the longest-term studies are those of killer whales (Orcinus orca) in the inshore waters off Vancouver Island, Canada and Washington State, USA. Observations since the 1970s of killer whale pods for which all members are recognizable have provided one of the most complete datasets available for free-ranging small cetaceans (e.g., Olesiuk et al., 1993). Similarly, studies initiated in the 1980s in Shark Bay in Western Australia have provided long-term data on individually identifiable, resident Indo-Pacific bottlenose dolphins (Tursiops aduncus, Mann et al., 2000). These and other studies integrated observations with remote biological sampling to obtain information on sex, genetics, age, diet, and hormone concentrations from a very small sample of skin and blubber (Wenzel et al., 2010; Sinclair et al., 2015). Observational studies since 1985 from the surface and underwater of individually identifiable, resident Atlantic spotted dolphins (Stenella frontalis), on the Bahama Banks, have also provided insights into their reproduction and demography (Herzing, 1997).
Some long-term observational projects have increased our understanding of life history and reproductive biology through brief catch-and-release operations (e.g., Wells et al., 2004). Catch-and-release provides opportunities to collect data and samples to provide information on sex, genetics, age, morphometrics, mass, maturity status, reproductive hormone concentrations, pregnancy and lactation status, testis size, and health and environmental contaminant concentrations, and to mark individuals for unambiguous identification during subsequent observations over the course of their lives (Barratclough et al., 2019a). The combination of long-term observation research coupled with sampling is exemplified by bottlenose dolphin research conducted in Sarasota Bay, Florida since 1970 (Scott et al., 1990; Wells, 2009, 2020), and Amazon river dolphin (Inia geoffrensis) research conducted in the Brazilian Amazon River since 1994 (Martin and Da Silva, 2018). In contrast to the single snap-shot of an individual provided from carcasses, long-term observational studies, especially those coupled with catch-and-release, can provide longitudinal and varied data for describing the actual history of life for individuals, including estimation of milestones such as age at first birth, calf independence from mother, and calf survival.
The need for high-quality empirical data on small cetacean life history, reproductive, and demographic parameters goes beyond general biological interest. These data are needed for conservation, as they are fundamental to understanding the population processes that are assessed for evaluating the status of population units and development of management plans. Statistical modeling is an important conservation tool for understanding the impacts of natural or anthropogenic forces on projections for populations into the future (e.g., Schwacke et al., 2017). Such models require input parameters based on the biology of the animals, using empirical data from the stocks or species of interest where they are available, or otherwise basing assumptions on comparable taxa. Increased management consequences for stakeholders call for increased specificity and precision of parameters used for modeling.
Studies of the long-term resident bottlenose dolphins of Sarasota Bay, Florida, USA, have been conducted by scientists, students, and collaborators of the Sarasota Dolphin Research Program (SDRP) since 1970, making this the longest-running study of any population of small cetacean. Detailed longitudinal data collected for individually identifiable dolphins through integrated observational studies, systematic photographic identification (photo-ID) surveys, tagging and tracking, catch-and-release health assessments, remote biopsy dart sampling, and findings from stranding response efforts form a unique dataset for describing the biology of a small cetacean, and for refining life history, reproductive, and demographic parameters for bottlenose dolphins and comparable species. The purpose of the current study is to summarize results on life history, reproduction, and demographics of bottlenose dolphins from Sarasota Bay and make the unique data set available for comparative or other studies.
Methods
Study area
The long-term study area includes the inshore and coastal waters along the central west coast of Florida, from Tampa Bay southward through Charlotte Harbor and Pine Island Sound, and in the Gulf of Mexico up to about 5-10 km offshore (Figure 1). Most of the research effort, however, has been concentrated in Sarasota Bay and adjacent bays, sounds, and Gulf waters within about 1 km of the shore, due to initial findings of dolphin residency to these waters (Irvine and Wells, 1972). The shallow (< 4 m deep), sheltered bay and estuarine waters are separated from the Gulf of Mexico by a series of barrier islands, communicating with the Gulf through narrow, deeper (up to ~10 m deep) passes. The bays contain areas of shallow seagrass meadows and are fringed by mangroves, along with extensive manmade features such as bridges, piers, and seawalls. Natural or dredged channels 3-4 m deep run through seagrass meadows and sand or mud flats. A gently sloping, shallow sandy bottom extends offshore from the Gulf sides of the barrier islands.
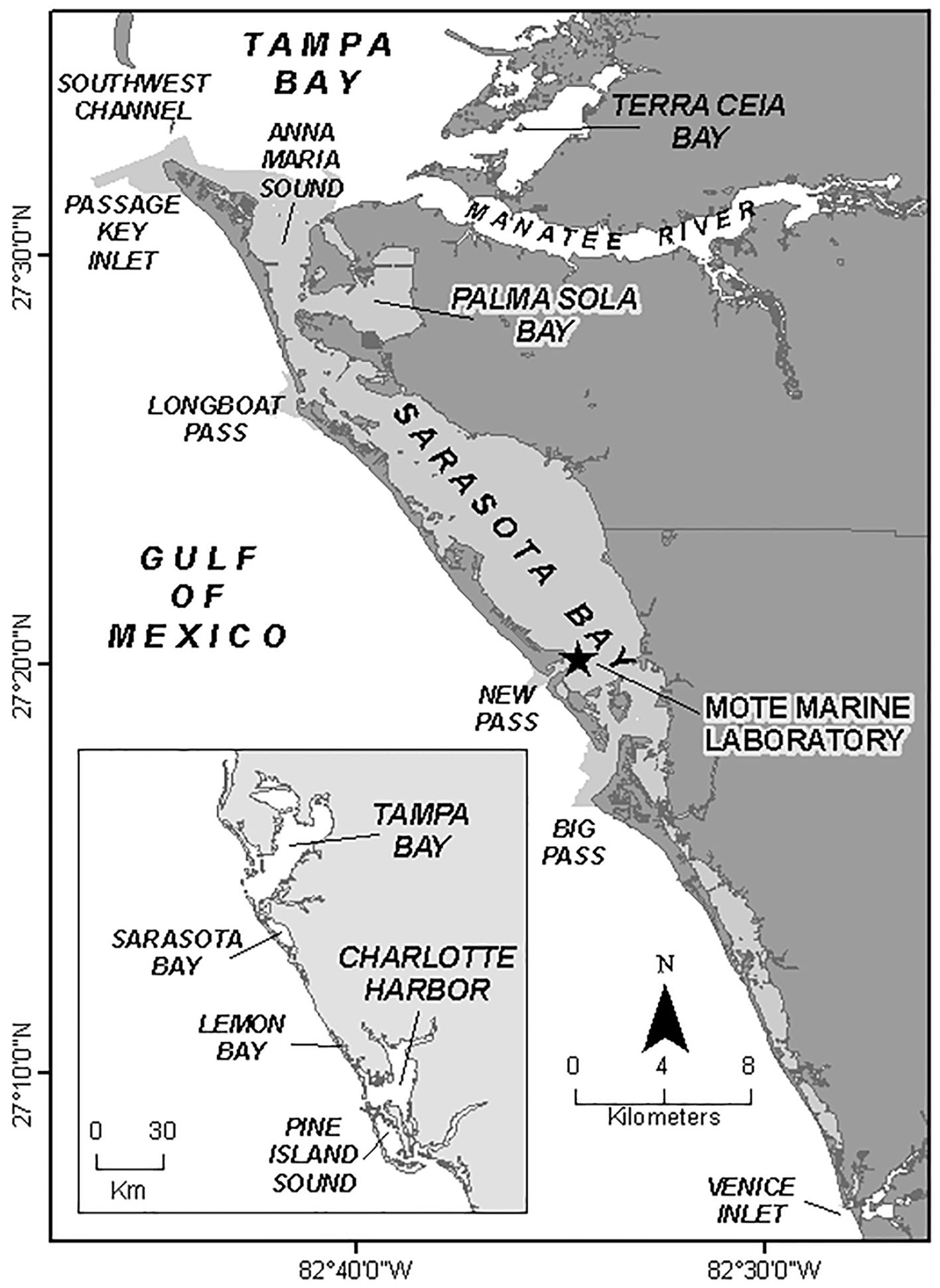
Figure 1. Sarasota study area, and core area (shaded) defining residency for life history and demographic analyses.
The long-term resident Sarasota dolphin community inhabits a home range extending from southern Tampa Bay to Venice Inlet (Figure 1; Wells, 2014). Sarasota and Manatee Counties, which encompass the Sarasota dolphin community’s home range, are heavily populated, with more than 900,000 people, and more than 46,000 registered vessels (as of 2023).
Data collection
Longitudinal data on individually identifiable bottlenose dolphins were collected through observations, radio-tracking, sampling and measurements during catch-and-release operations, remote biopsy dart sampling, and stranding response.
Observations and radio-tracking
As summarized by Scott et al. (1990) and Wells (2009), initial identifications of individuals occurred through tagging with plastic tags and freeze-brands on dorsal fins, with opportunistic resighting, during 1970-1972 (Irvine and Wells, 1972). During 1975-1976, additional tags were applied, including UHF radio-transmitters, and systematic surveys through the study area (based on radio-tracked movements) were initiated to locate tagged and naturally marked dolphins (Wells et al., 1980; Irvine et al., 1981). Opportunistic photo-ID surveys continued through the 1970s. In 1980, funded seasonal photo-ID surveys were initiated, and continued until 1992 (Wells and Scott, 1990; Wells, 2009). Since mid-1992, standardized photographic-identification surveys have been conducted on ten boat-days each month in and around Sarasota Bay (Wells, 2014). Data from the dedicated Sarasota photo-ID surveys have been supplemented by identification photographs collected during other field efforts in Sarasota Bay, and through photo-ID surveys through the adjacent or nearby waters of Tampa Bay (Wells et al., 1996a; Urian et al., 2009), Charlotte Harbor (Wells et al., 1996b; Bassos-Hull et al., 2013), Pine Island Sound (Wells et al., 1997), and the coastal Gulf of Mexico (Fazioli et al., 2006). Demographic analyses for this paper are based primarily on sighting data collected during the years involving the most consistent photo-ID effort in Sarasota Bay, 1993-2019, with additional data providing perspective on movements of identifiable individuals beyond Sarasota Bay.
Photo-ID surveys have been conducted typically from 6-7 m, outboard-powered research vessels that traveled at the minimum speed allowable to maintain a plane (i.e., 10 to 14 knots), during good sighting conditions (Beaufort Sea State ≤ 3) (Rosel et al., 2011; Urian et al., 2015). Research teams consisted of two to five researchers onboard each vessel. Surveys were designed to cover the entire study area each month, from Terra Ceia Bay, Manatee River, and southern Tampa Bay, southward to Venice Inlet, including all inshore waters and Gulf coastal waters to within about 1 km from shore (Figure 1). Specific daily routes were selected based on weather, seas, and tides.
When a group of dolphins was sighted, the boat slowly approached the animals and recorded their location, along with other environmental (e.g., depth, salinity, dissolved oxygen, sea state, weather), and biological data (e.g., numbers of dolphins, young-of-the-year and older calves, dolphin activities, associated organisms). Single-lens reflex cameras with 70- to 300-mm zoom telephoto lenses have been used to obtain photographs of the dorsal fin and other distinguishing features (e.g., peduncle) of every dolphin in the group. In the early years of photo-ID, images were primarily color slides, but since 2004 photographs have been digital. If the group was large, an attempt was made to obtain photographs of as many animals as possible, marked or unmarked (Rosel et al., 2011; Urian et al., 2015). Images were examined by experienced staff and compared to photo-ID catalogs to confirm identifications, and sighting data were entered into a relational database, FinBase (Adams et al., 2006), and double-checked, for further analyses.
Sampling and measurements during catch-and-release operations
Catch-and-release operations have been conducted periodically for a variety of purposes, including applying tags (1970-1976; Irvine and Wells, 1972; Irvine et al., 1981), obtaining life history samples and data and marking individuals for long-term identification (1984 – present; Wells et al., 1987; Wells and Scott, 1990), and obtaining information on health, physiology, and environmental contaminant concentrations (1988 – present; Wells et al., 2004, 2005). Small groups of dolphins were encircled by a 500-m long x 4-m deep seine net deployed by a fast boat in shallow water (e.g., Loughlin et al., 2010). Teams of experienced handlers and veterinarians were deployed around the net compass to ensure the safety of the dolphins. One at a time, dolphins were brought aboard a specially designed veterinary processing vessel, where they were weighed and placed on a foam-covered, shaded deck for measurements, examination, and sampling. In some cases, samples were collected while the dolphin was in the water. Upon completion of the work, including marking with a freeze-brand to facilitate subsequent identification (Wells, 2018), the dolphins were photographed and released on-site.
Sex was determined from examination of the genital slit of each dolphin handled, and standardized morphometrics were collected, including mass, lengths, and girths. Blood samples for health, genetic, and reproductive hormone analyses were collected by veterinarians from vasculature in the fluke (Wells et al., 2004; Barratclough et al., 2019a). Maternal relationships with presumed calves were confirmed via genetic analyses, and paternities were determined through exclusion analyses (Duffield and Wells, 1991, 2002, 2023). Concentrations of progesterone, testosterone, and estradiol were obtained from analyses by Cornell University’s Animal Health Diagnostic Center (see below). Diagnostic ultrasound examination provided information on pregnancies and testis dimensions (Wells et al., 2014; Barratclough et al., 2019a).
Age determination
Most dolphins were of known age because they were monitored from the time of birth to known resident mothers. Initially, if a dolphin was of unknown age, a tooth was obtained under local anesthesia for examination of growth layer groups (Hohn et al., 1989). In more recent years, new, less-invasive techniques became available and were applied. The few unknown ages were estimated from radiographic assessment of flipper bone fusion (Barratclough et al., 2019b), dental radiography (Herrman et al., 2020), and/or epigenetics (Beal et al., 2019; Barratclough et al., 2021). It should be noted that dolphin age estimation using any of the available techniques tends to become less accurate in older dolphins, particularly those more than 30 years old (Barratclough et al., 2023). The ages of most of the older dolphins used for this study were first estimated when they were in their mid-20s to mid-30s, and their old ages were derived from incrementing their ages annually after the first estimation. For example, the oldest female in the dataset, 67-yr-old FB15, was estimated from tooth growth layer groups to be 34 years old at her first sampling in 1984, and she was observed for another 33 years. The oldest male, 52-yr-old F154, was sampled when he was 28 years old, and he was observed for another 24 years. Of the other nine who reached 50 years or more during the study period, two were estimated to be 43 years old when first sampled, and reached 50 and 52 years. The remaining seven were estimated to be 24-36 years old when first sampled, reaching 50-62 years of age during the study period. Thus, while the actual error in age estimates is unknown, we anticipate that is it relatively small in the sample used in the current analyses, given that many of the older individuals were much younger when their age was first assigned, and age estimation is more accurate for younger dolphins. Therefore, the ages of most of the older dolphins should be considered more accurate than if they were first estimated at their oldest ages.
Remote biopsy dart sampling
Additional information on dolphin sex, genetic relationships, age, and reproductive hormones was obtained in some cases from small samples of skin and blubber collected through remote biopsy dart sampling (Sellas et al., 2005; Kellar et al., 2006, 2009; Beal et al., 2019). Using standard techniques (Kiszka et al., 2010; Wenzel et al., 2010; Sinclair et al., 2015), dolphins were selected and approached for sampling with darts delivered from a distance of 2-9 m, from either a modified rifle (e.g., John Geiges, South Carolina, USA) or a crossbow, targeting the region immediately below or behind the dorsal fin. Darts included stoppers to limit penetration depth, and held stainless-steel sampling heads that were typically 10 mm in bore diameter, and 25 mm long (e.g., Ceta-Dart, Copenhagen, Denmark).
Stranding response
Marine mammal stranding response network members along the central west coast of Florida, especially Mote Marine Laboratory’s Stranding Investigations Program, provided crucial life history samples and data for resident dolphins (e.g., Hazelkorn et al., 2020). Necropsies of recovered carcasses yielded information on sex, age, genetics, reproductive status, morphometrics, and cause of death or impacts of injuries (Wells et al., 2008; Wells, 2009). About one third of all Sarasota Bay resident dolphins that disappear are eventually recovered as carcasses (Wells et al., 2015).
Data selection
More than 5,000 bottlenose dolphins have been individually identified by the SDRP along the central and southwest coast of Florida over the decades, but these are from multiple population units, and not all have been observed with sufficient frequency to be of value for analyses of life history, reproductive, and demographic parameters (Wells, 2014). For the analyses reported here, a subset of dolphins, belonging to an intensively and systematically studied, biologically meaningful population unit was selected, the Sarasota dolphin community (Wells, 2014). A dolphin community has been defined as “a regional society of animals sharing ranges and social associates, but exhibiting genetic exchange with other similar units” (Wells et al., 1999). Dolphins were considered to be residents of the Sarasota dolphin community if they were seen at least ten times and more than half of their sighting records occurred within the region bounded by Tampa Bay to the north and Venice Inlet to the south, and the barrier island chain to the west. In addition to facilitating the practical considerations of observation frequency, the geographical criterion also ensures that common evolutionary selection pressures are in force across the dolphin unit, shaping the parameters of interest.
The dataset included long-term resident Sarasota Bay dolphins that were seen with sufficient frequency that it was unlikely that important milestones in their lives, such as births and deaths, would be missed. Along the west coast of Florida, bottlenose dolphins live in long-term resident communities that form a mosaic, with slightly overlapping ranges (Wells et al., 1987; Urian et al., 2009). While regular systematic surveys that include waters adjacent to Sarasota Bay, along with radio-tracking, have demonstrated that Sarasota Bay residents often range into adjacent waters shared with other communities, for example along the Gulf of Mexico beaches or into southern Tampa Bay, the vast majority of Sarasota Bay resident sightings are inshore of the barrier island chain that defines Sarasota and associated bays (Irvine et al., 1981; Wells et al., 1987, 2013; Wells, 2014). Wells (2014) reported that, on average, 89% (± 12% SD) of the sightings of dolphins considered to be Sarasota Bay residents occurred within Sarasota Bay. Of those dolphins known to be at least 15 years old, 96% had been observed in the area over a span of at least 15 years, and some had been observed for as many as 45 years.
Dolphins were selected for analyses if they were observed during 1993-2019 and available data supported that they spent more time in the core portion of the Sarasota dolphin community range inside Sarasota and associated bays and sounds than outside of that region. The criteria included:
1. The animal must have a minimum of 10 sightings recorded since 1975 (not just during the 1993-2019 study period), or the animal is the calf of a female with at least 10 sightings. Sighting sources included all available data from the sighting database: surveys, focal-animal follows, and catch-and-release operations.
2. More than 50% of all sightings of an individual were inshore of the barrier islands, and included associated shallow bars bordering passes and between Tampa Bay and Anna Maria Sound (shaded area of Figure 1).
3. The individual must have been observed in the region during more than six different months of the year. These months can have occurred within one year or may have been spread across multiple years of the animal’s records.
Data analyses
Female sexual maturity
Female sexual maturity was estimated from 1) observations of females with first-born calves, 2) ultrasound exams, and 3) reproductive hormone concentrations in blood, as possible. Observational data confirmed maturity when a female was seen regularly (in at least three consecutive sightings) accompanied by a young dolphin believed to be its calf. In many cases, genetic analyses of samples from the calf and the presumed mother were possible, and in each case of close association, maternity was supported (Duffield and Wells, 2002). Presumed full reproductive histories were documented for females either observed from birth, or from an age when they were too young to produce a calf. In a few cases, estimates of age at first birth could be biased upward, as it was possible that a first-born calf died before it could be observed alongside its mother. In several cases, stranded calf carcasses have been matched to mothers genetically. However, based on the frequency of observations during the primary calving months during potential reproductive years, the presumed first birth of each of these females has likely been recorded. The latest possible age at sexual maturation for each mother was estimated by subtracting the mean gestation period of 12.5 months (O’Brien and Robeck, 2012) from the mother’s age at parturition.
Female sexual maturity status was also derived from measurement of serum progesterone and estradiol in blood samples collected during health assessments (Wells et al., 2004). Progesterone analysis was conducted by the Endocrinology Laboratory of the Animal Health Diagnostic Center of Cornell University’s College of Veterinary Medicine (AHDC). Progesterone was measured using a solid-phase radioimmunoassay (RIA), with Coat-A-Count (CAC) reagents manufactured by Siemens Healthcare Diagnostics. The assay was designed for quantification of progesterone directly in serum or plasma, using antibody-coated polypropylene tubes, [1251]-progesterone label, and the calibrators included with the kit. The assay calibration range was 0.1-40 ng/ml, and the analytical sensitivity (limit of detectability, LD) was about 0.02 ng/ml, depending on the year in which the analyses were performed. Concentrations returned as <LD were converted to values as one half of the indicated LD. Hormonal indications of sexual maturity included increases in serum progesterone above baseline. Progesterone values of >5.0 ng/ml were considered to be indicative of pregnancy (Steinman et al., 2016). Estradiol was measured using a solid-phase radioimmunoassay (RIA) which included a pre-assay sample extraction. Samples were extracted using ethyl ether and 3H-Estradiol (i.e., tritiated-Estradiol). The 3H-Estradiol was used for determining percent extraction efficiency for each sample, and this number was used in the calculation of the final result. The procedure used Coat-A-Count Estradiol antibody-coated polypropylene tubes and [1251)-estradiol reagents manufactured by Siemens Healthcare Diagnostics, and a standard curve prepared by the laboratory. The assay was designed for quantification of Estradiol in extracted serum or plasma samples. The assay calibration range was 15 to 1,000 pg/ml with an analytical sensitivity (LD) of about 6 pg/ml, depending on the year in which the analyses were performed. Concentrations returned as <LD were converted to values as one half of the indicated LD. In some cases, it was also possible to determine maturity from ultrasound examination, through visualization of follicular activity or a fetus (Wells et al., 2014).
A nonlinear mixed model was used to predict the average age, and length and mass at first birth based on repeated sampling of individuals, with individual dolphin used as a random effect in the model (SAS 9.4 Proc NLMIXED). For each sighting of each dolphin, the individual was identified as mature or immature. Only one sighting per year was included in the model, with the summer sighting chosen if available, as most of the other data were from summer. For length at maturation, length was truncated for convergence and to reduce the effects of a relatively large number of smaller individuals; the final model used lengths ≥200 cm. Results for females and males were compared. The model was also run to estimate age at sexual maturation in females after deducting 12.5 months from individual ages at the time of sighting, and that result compared to age at sexual maturation (ASM) in males.
Male sexual maturity
Male sexual maturity was also determined from multiple methods, primarily from data and samples obtained during health assessments, and included 1) presence of sperm in urine samples, 2) serum testosterone levels, 3) ultrasonic measurements of the testes, and 4) genetic paternity determinations. Serum testosterone was measured by the AHDC. Testosterone was measured through 2014 using a solid-phase radioimmunoassay (RIA), with Coat-A-Count reagents manufactured by Siemens Healthcare Diagnostics. The assay was designed for direct measurement of testosterone in serological samples using antibody-coated polypropylene tubes, [125I]-testosterone as tracer, and the calibrators included with the kit. The assay calibration range was 0.2 -10 ng/ml and the analytical sensitivity (limit of detectability, LD) was about 0.04 ng/ml, depending on the year in which the analyses were performed. Concentrations returned as <LD were converted to values as one half of the indicated LD. Testosterone spikes above approximately 5 ng/ml were considered to be indicative of maturity, as elevated testosterone concentrations beyond this level (up to 300 times immature levels, Sherman et al., 2021) co-occur with other indications of maturity, such as increased testis size, and/or siring of offspring.
During 2015-2019, following discontinuation of the Siemens assay, the ImmuChem Testosterone radioimmunoassay kit was used (MP Biomedicals, LLC, Solon, OH). Comparisons of the two assays by the Cornell lab found very close concordance (R2 = 0.995, B. Schanbacher, Animal Health Diagnostic Center Endocrinology Laboratory, pers. comm., 18 May 2021), so the data sets from both analyses have been combined for subsequent analyses. The nonlinear mixed model used for estimating female maturation parameters was also used for males.
Ultrasound was used to measure testis length and diameter (Wells et al., 2004). Male social maturity, the age at which sexually mature males successfully sired offspring, was determined from genetic paternity analyses (Duffield and Wells, 1991, 2002, 2023).
Calving intervals
Calving intervals (CI) were measured from the birthdate of one calf to the birthdate of the presumed next calf. The birthdate is considered to be the midpoint of the period from the last sighting of the mother alone to her first sighting with a new calf, if that period was <120 days. If the period was >120 days, then a birthdate was not identified and a CI was not calculated. A t-test was used to test for differences in CI when calves were successfully reared or not, summed across all mothers. The effects of mother’s age, calf rearing success, calf sex and birth sequence on CI were tested using a mixed model for repeated sampling of individual mothers, with individual dolphin as a random effect (SAS 9.4 GLIMMIX).
Calf rearing durations
Calf rearing durations were measured in two ways:
1. Maximum duration of association: This measure tries to account for the fact that some separations of mothers and calves are gradual rather than abrupt. Separation was considered to have occurred immediately after the last date that the rolling half-weight coefficient of association (COA, as per Wells et al., 1987) calculated over a period of one year was >0.5. The duration was measured from the estimated calf birthdate, as defined under Calving Intervals. While the COA calculation benefited from the more robust sighting sample size associated with a one-year criterion, this affected the sensitivity of the analysis and likely biased it toward longer durations of rearing association, as sufficient numbers of sightings of individuals alone must be included in the analysis to lower the COA below 0.5. As above, the effects of mother’s age, calf rearing success, calf sex and birth sequence on calf rearing duration were tested using a mixed model for repeated sampling of individual mothers, with individual dolphin as a random effect (SAS 9.4 GLIMMIX).
2. To characterize more-abrupt separation events, separation of a calf was also scored as occurring on the date of the first sighting of a series of at least five consecutive sightings of the calf without the mother. The duration was measured from the estimated calf birthdate, as described above.
Minimum abundance
Minimum abundance was measured as the number of dolphins that met our criteria for residency. These numbers represent a minimum count of identifiable individuals and current dependent calves present in the study area. This census does not take into account unidentifiable non-calf dolphins or dolphins previously considered to be residents, but not seen in the study area during a given year.
Age-sex distribution
Age-sex distribution histograms were created for each year. During the late 1960s-early 1970s, a number of young dolphins were commercially collected from Florida waters, including Sarasota and vicinity. The number of takes from the Sarasota area are not known as pre-Marine Mammal Protection Act of 1972 records were not available. The histograms were examined for gaps that may have coincided with these takes when projected through time. Kolmogorov-Smirnov tests were used to explore the possibility of differences among annual distributions for the first year (1993), the middle year (2006), and the last year (2019) of the study.
Birth rates and fecundity
The mean annual birth rate was calculated as the number of births relative to the number of dolphins present, not including calves born during the year. Fecundity was measured in several ways. The standard measure, of calculating the number of calves born each year per mature female, required knowledge of the maturity status of all of the resident females. While this detailed information was available for many individuals based on reproductive histories, ultrasound examinations, or hormone measurements as described above, it was not available for all. To calculate fecundity, the number of mature females was considered to be all females at least six years of age, the youngest age of parturition documented from this dolphin population. This included females of unknown age that had been observed with a calf presumed to be their own, or observed for at least six years. This approach may slightly overestimate fecundity, but likely not by much as the proportion of females in the youngest age classes is small. Age-specific fecundity was examined by dividing the number of births to females of a specific age by the number of available females of that age, summed over all 27 years of the analysis period.
Recruitment
Recruitment into the Sarasota dolphin community occurred through reproduction and, to a lesser extent, immigration. Recruitment through reproduction was scored when a calf born to a female considered to be resident during the 27-year analysis period was documented to have survived through at least its first year of life. The number of surviving calves was divided by the total population size for the year (minimum abundance, including surviving calves and those that did not survive). Non-reproductive recruitment was more difficult to define precisely, and included documented immigrants and possible immigrants. Immigration occurred when an individual was documented as having moved to Sarasota Bay after having been first documented outside Sarasota. Other dolphins were first documented as residents when they were non-calves. Within this category, it is difficult to distinguish dolphins new to Sarasota Bay coming from elsewhere from existing residents with changed identifying features. However, such identification feature changes are rare for Sarasota dolphins. The known immigrants provided a lower bound, for calculating the minimum annual immigration rate, and the combined documented and possible immigrants provided an upper bound, for calculating the maximum annual immigration rate, relative to the total population size each year.
Losses of individuals from the 27-year dataset included documented or presumed mortalities, emigration, changed identification characteristics, and disappearances (dolphins never identified again). Overall, 33% of disappearances are eventually recovered as carcasses (Wells et al., 2015) and scored as documented mortalities (see Lovewell et al., this volume). In addition, disappearances of calves within their first year of life are also scored as mortalities due to the low likelihood that such highly dependent calves could survive on their own.
The mean minimum annual mortality rate was based conservatively on only known and presumed deaths. The mean annual minimum mortality rate likely underestimates the true mortality rate, as 67% of dolphins disappear without being recovered as carcasses or identified as having emigrated. The mean annual maximum mortality rate includes known mortalities and disappearances. These values may include small numbers of continuing residents for which identifying features changed, but, as noted above, such changes are rare. Several emigration events were documented, when identifiable resident Sarasota dolphins were observed elsewhere after having left Sarasota (McHugh et al., 2011).
Reproductive success
Reproductive success was measured for females as the proportion of calves observed after separation from the mother, indicating successful rearing to independence. Male reproductive success was defined by the number of cases in which a male was identified in paternity tests as the sole possible sire (Duffield and Wells, 2023).
Results
Dataset description
In total, 482 identifiable dolphins met the data-selection criteria. Of these, 69% were of known sex (179 f: 152 m: 151 unknown). The year of birth was known or estimated for 411 dolphins (85%), including individuals ranging in age from young-of-the-year calves up to 67 years. Fifteen dolphins were observed across all 27 years of the dataset; on average, individuals appeared in the dataset for 8.5 years. The dataset included 130 known mothers and 453 of their calves (some of these calves also became mothers). Reproductive histories continuing from the birth of the presumed first calf are available for 54 of the mothers in the 1993-2019 dataset, and include as many as 11 calves for any given mother (since these analyses were completed, a Sarasota resident was observed with her 12th calf). For analyses involving full reproductive histories of mothers in the 1993-2019 dataset, calves born during 1973-2020 were included.
Reproduction: sexual maturity
Females
The youngest female observed with a new calf was 6 years old, indicating sexual maturation at ~5 years old or earlier. The youngest pregnancy detected during health assessment ultrasound exams involved a 7-yr-old female, whose calf was born 17 days later, indicating the female became sexually mature at 6 years of age or younger. Increases in serum progesterone above baseline were recorded for other females as young as 7 years of age (Figure 2A).
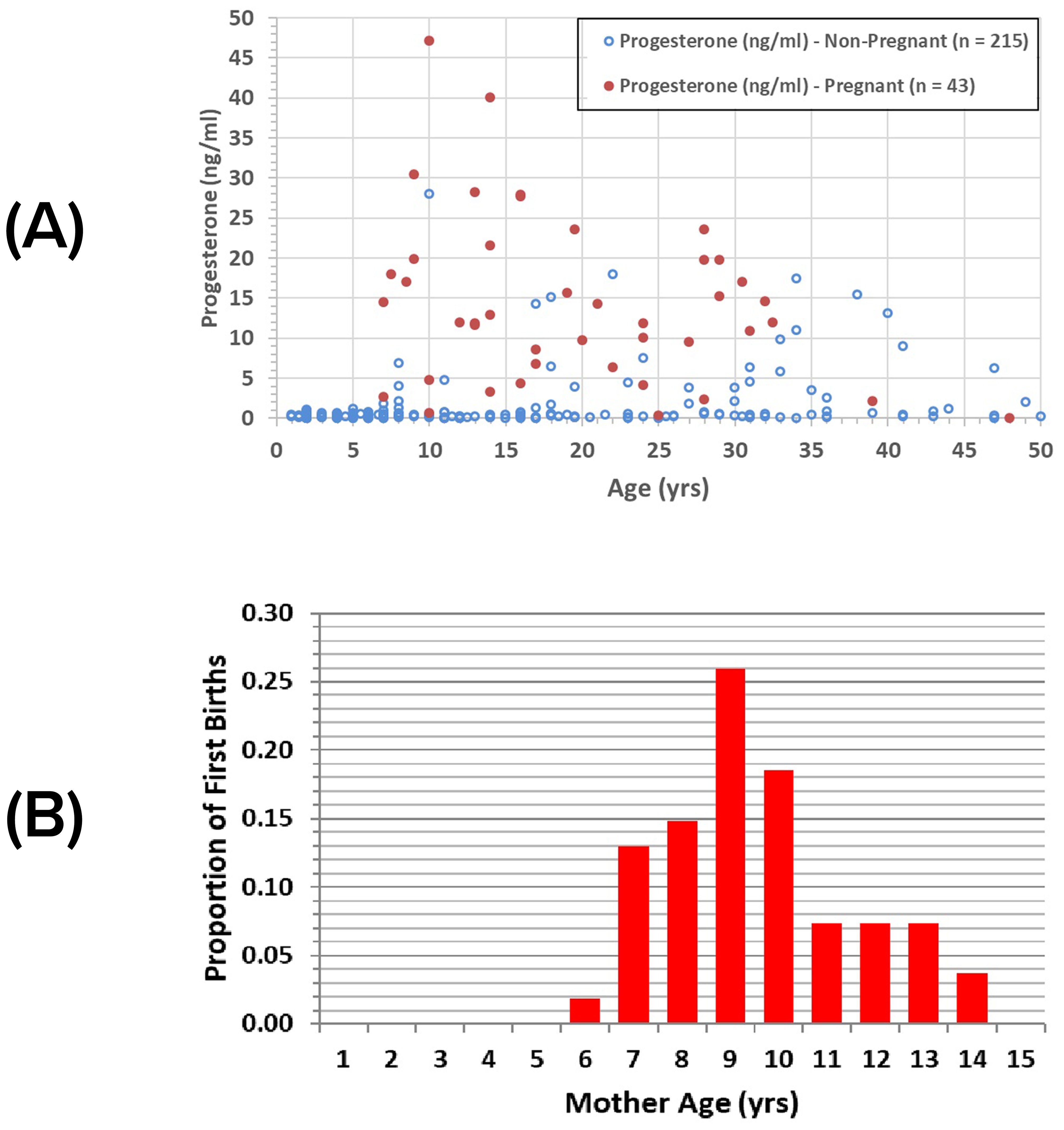
Figure 2. Sarasota female sexual maturity: (A) serum progesterone relative to age and pregnancy status for Sarasota female dolphins; (B) proportions of first births relative to mothers’ ages (n = 54).
We also examined the sighting data for those females who had not yet been observed with a calf, females that we considered as immature. Of the 183 immature females, all were 10 years old or younger except for two, ranging up to 13 years of age. Thus, age classes 5-13 years were considered indeterminate with regard to sexual maturation, i.e., age classes in which both immature and mature females occurred.
Presumed first-born calves have been observed with females up to 14 years old (Figure 2B). The mean age of females observed with their first calf was 9.6 years (± 1.96 sd, n=54), yielding a mean age at sexual maturation of 8.5 years, a value that is likely biased upward because it is based on the unlikely assumptions that conception occurred immediately upon reaching sexual maturity and all first calves were detected. The predicted age at first calving from the mixed model was 8.77 years, with a predicted age at sexual maturation of 7.84 years (Table 1).
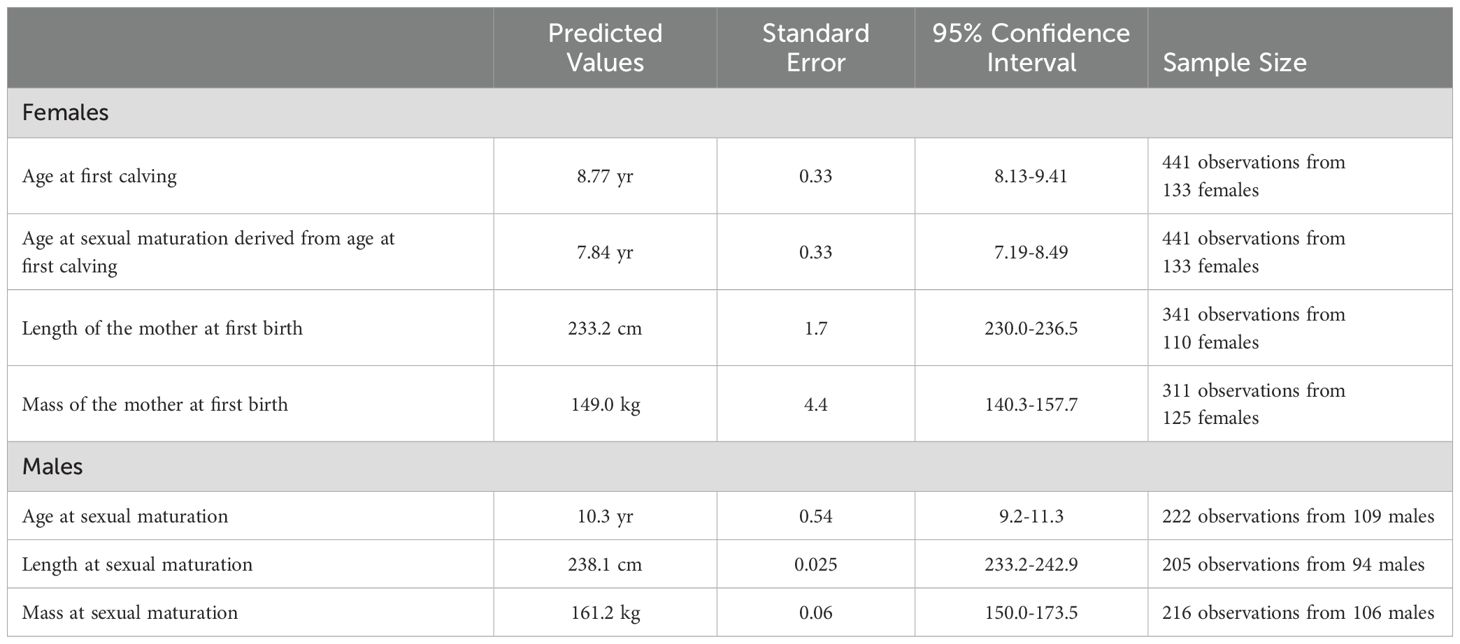
Table 1. Predicted age, length, and mass at first birth (mothers) and sexual maturation from general linear mixed models for bottlenose dolphin males and females from Sarasota Bay.
All but one of the females documented as mothers were at least 225 cm in length and weighed at least 138 kg. The one exception, FB99, exhibited congenital scoliosis, limiting her length to 215 cm and her mass to 121 kg (DeLynn et al., 2011). All but six observations from immature females were less than 250 cm; the six were 250-256 cm. The predicted maternal length at first birth was 233.2 cm (Table 1). All but two immature females weighed 177 kg or less; the two weighed 196 and 207 kg. The predicted maternal mass at first birth was 149.0 kg (Table 1).
The average length at first calving was reached at 93.7% of asymptotic length (Figure 3A, asymptotic values from Read et al., 1993). The average mass at first calving (Figure 3B) was reached at 76.8% of asymptotic mass. Data to estimate the average length and mass at maturation (as opposed to at first calving) are not available.
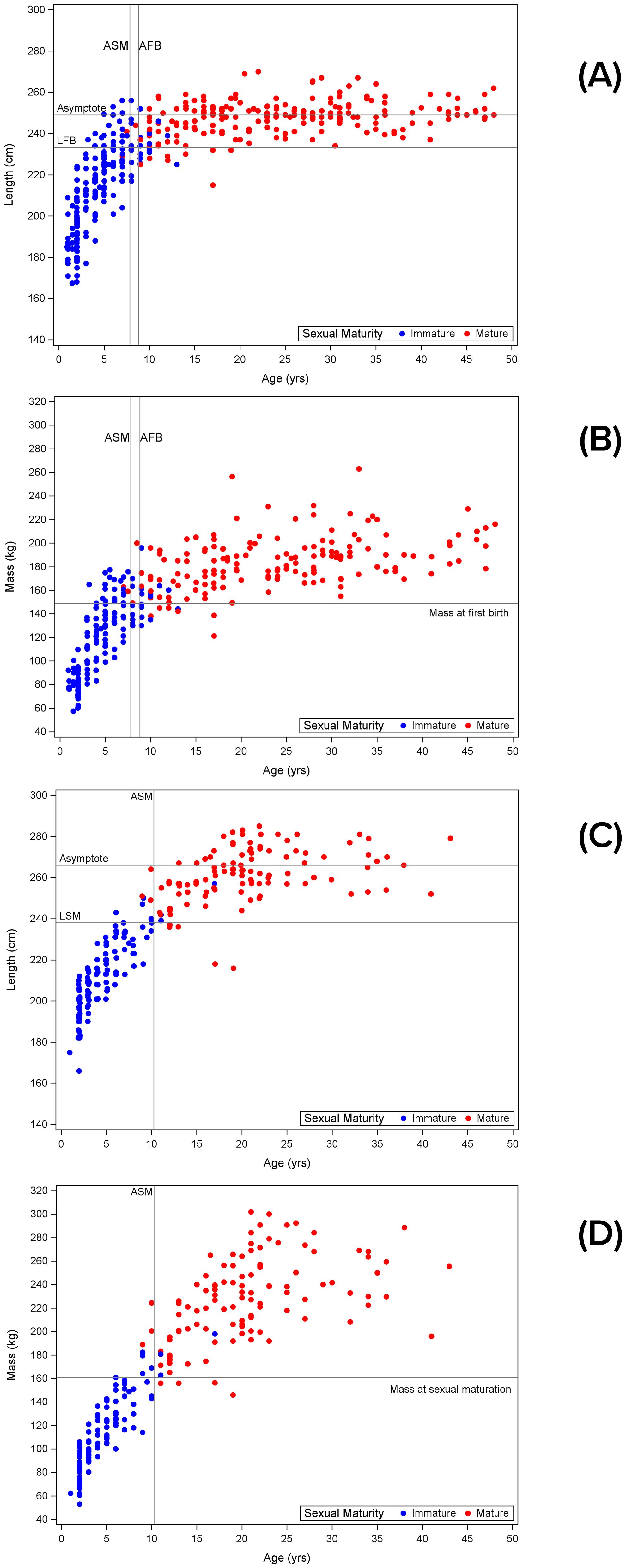
Figure 3. Length and mass relationships with female and male maturity: (A) female body length relative to maturity status based on whether females had been seen with a calf, and age; (B) female mass relative to maturity status based on whether females had been seen with a calf, and age; (C) male body length relative to maturity status and age; (D) male mass relative to maturity status and age. ASM, age at sexual maturation; AFB, age at first birth; LFB, length at first birth; LSM, length at sexual maturation.
Males
The presence or absence of sperm in urine samples was recorded for 119 males. Sperm was reported from 39 urine samples collected from males ranging in age from 11 to 43 years. Of these, 38 were collected during May and June, and one was collected from a 28-yr-old male in November. Samples with no sperm were collected from 80 males ranging in age from 2 to 34 years, collected during February, May, June, and November. Of these, 63.8% were collected from males less than 11 years old.
Serum testosterone increased with age beginning at about 10 years, increasing by several orders of magnitude (Figure 4A). With the exception of one immature 17-yr-old (based on low testosterone and lack of sperm in urine during breeding season, and not yet a sire), the oldest immature males were 11 years old while the youngest mature males were 9 years old, resulting in just three indeterminate age classes, ages 9-11 years. By age class, one of six 9-yr-olds, two of five 10-yr-olds, and four of six 11-yr-olds were mature. The predicted age at sexual maturation from the mixed model was 10.3 years (Table 1). Breeding season testosterone concentrations peaked during about 12-28 years of age, and then declined.
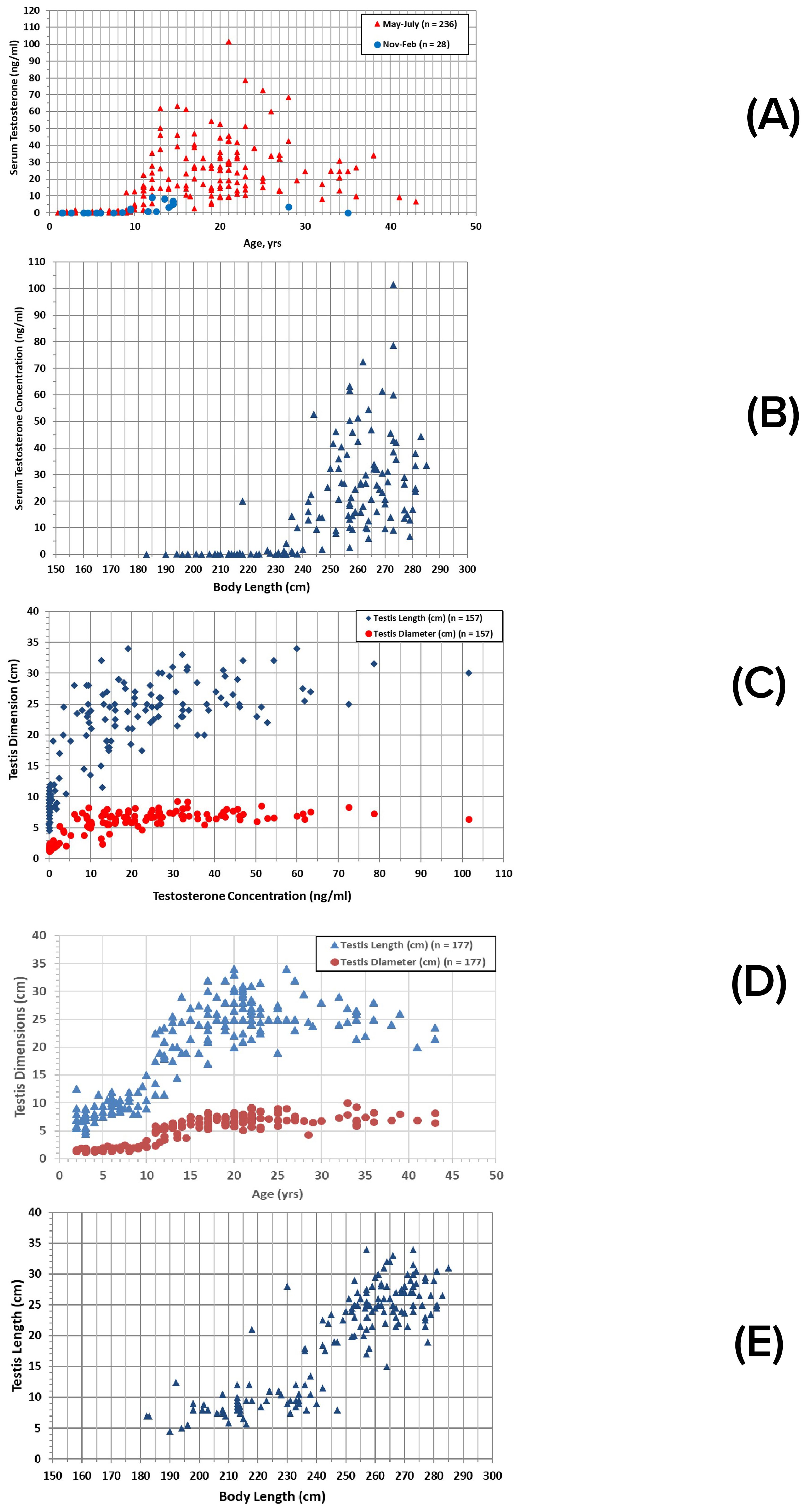
Figure 4. Male reproductive parameters: (A) serum testosterone concentrations relative to male age and sampling season; (B) relationships between testosterone concentrations and total body length during spring/summer (n = 143); (C) relationships between testosterone concentrations and testis dimensions; (D) ultrasonic measurements of testis dimensions relative to age; (E) ultrasonic measurements of testis dimensions relative to total body length, including only spring/summer measurements (n = 177).
Increased testosterone concentrations were associated with increased body length (Figure 4B) and testis dimensions (Figure 4C). All but six (of 107) measurements of immature males were less than 240 cm; the six were 240-257 cm long, with the largest being the 17-yr-old immature male. All but one mature male were 236 cm or longer, although the majority (98 of 115 observations) were 250 cm or greater. The smallest mature male was 216-218 cm at 17-19 years of age. The predicted length at sexual maturation was 238.1 cm (Table 1).
Ultrasonic measurements of testis length and diameter were in accord with the testosterone measures. Both dimensions began to increase rapidly at about 10 years of age (Figure 4D), and at about 235 cm in total body length (Figure 4E).
All but four of 104 observations of immature males weighed less than 170 kg; the four ranged from 179 to 198 kg. All but four of 113 observations of mature males weighed more than 179 kg; the four weighed 146-156 kg. The predicted mass at sexual maturation was 161.2 kg (Table 1). The predicted length at sexual maturation was reached at 89.5% of asymptotic length (Figure 3C, asymptotic values from Read et al., 1993). The predicted mass at sexual maturation (Figure 3D) was reached at 62.2% of asymptotic mass.
Male maturation was also assessed from paternity determinations of calves born to known mothers. Genetic analyses narrowed the identity of the sire to a single resident male candidate in 135 cases (n=129 known-age males) (Duffield and Wells, 2023). The youngest sire was 10 years old at conception (Figure 5A). Siring frequency increased from the mid-teens until the mid-twenties, and then declined, with the oldest sire being 43 years old. Individual males were responsible for siring up to 7 calves, over breeding tenures of up to 24 years. Data on testis size, along with testosterone concentration, were obtained for seven males sampled within one year of their first documented siring event: testis length ranged from 22 to 33 cm, with diameters from 5.7 to 8.3 cm, testosterone ranged from 24.6 to 72.5 ng/ml, and these seven males ranged in total body length from 262 to 277 cm. Overall, males averaged 263.5 cm (± 14.9 cm sd, n=26) in length by the time they were first documented to have sired calves. The shortest sire was 216-218 cm at 17-19 years of age; this individual did not grow beyond this length.
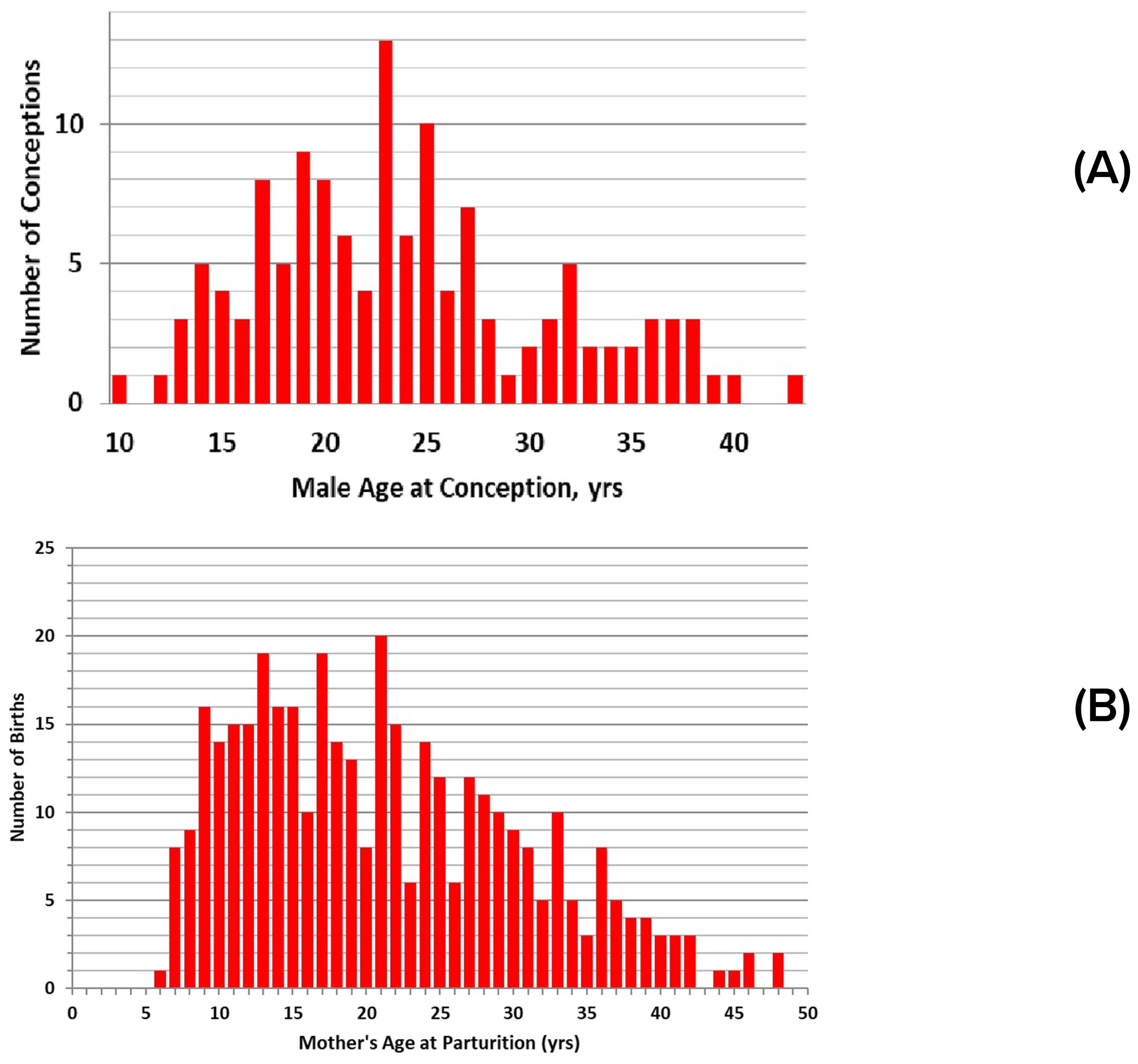
Figure 5. Calf production relative to parental age: (A) numbers of calves conceived relative to the age of the sire at the time of conception (n=129 events); (B) numbers of calves born relative to mother’s age at parturition (n = 375).
The predicted age at maturation was significantly different for males and females; males were older than females relative to both age at sexual maturation (p=0.003) and age at first calving (p=0.018). No difference was found in length (LSM) (p=0.101) or mass at sexual maturation (MSM) (p=0.086) for males relative to females at first birth. A difference might be expected if comparing LSM and MSM for females at sexual maturation, both of which would be expected to be lower than LSM and MSM at first calving.
Reproduction: seasonality
Dolphin reproduction is seasonal in Sarasota Bay, with most calves (81% of 353 assigned birthdates) born during May-July (Figure 6A). No births have been documented for January, and only one birth has been assigned to December. Spikes in reproductive hormones in blood, including testosterone for males and estradiol for females, occurred at the beginning of the peak of the calving season, consistent with a 12.5-month gestation period, although sampling did not occur during all months (Figure 6B). Seasonal changes in testis size consistent with the calving and hormone changes were also evident for adult males (≥10 years) (Figure 6C). Average testis length in winter was 72% of that in spring/summer (17.83 cm ± 4.13 cm sd, n = 6, vs. 24.66 ± 4.61 cm sd, n = 115; t-test, equal variance, 2-tailed, p = 0.0005). Four males were examined and sampled in both June and February. In all four cases, testosterone concentration, testis length, and testis diameter were greater in June. Declines in testosterone concentration and testis size appeared to occur in late autumn. Values were similar in both months for one male examined in June and October. A male examined in both June and November showed lower values for testosterone and testis diameter, but not testis length, in November.
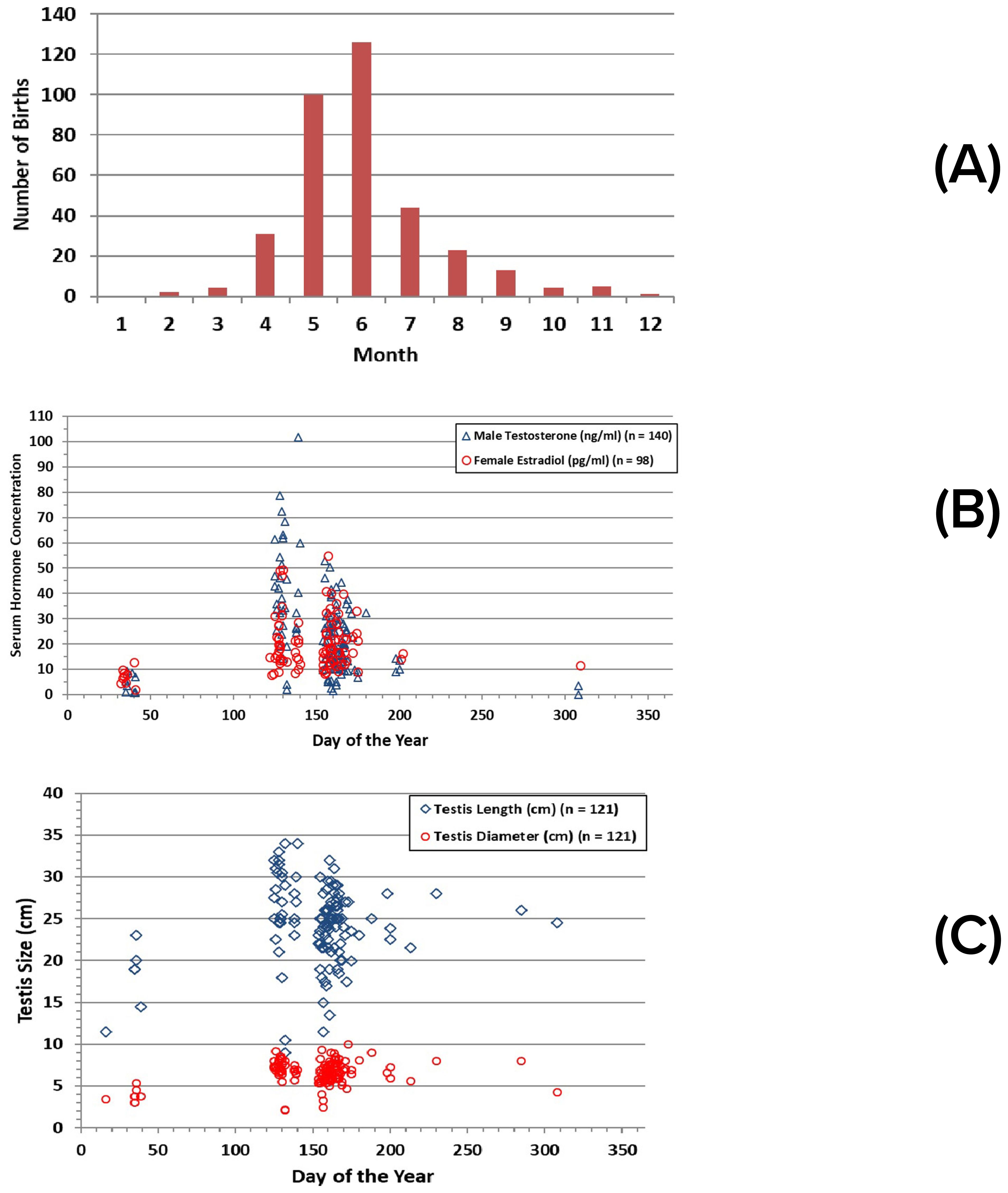
Figure 6. Reproductive seasonality indicated by: (A) number of calves born in Sarasota Bay, by month (n = 353); (B) estradiol concentrations in females, testosterone concentrations in males; (C) seasonal variation in testis dimensions for adult males (≥10 yrs).
Reproduction: calving intervals, duration of mother-calf associations
Calving intervals varied greatly, depending on maternal age, birth order, and whether a calf survived long enough to separate from its mother. The shortest calving interval when the calf survived to separation was 1.9 years. The mean calving interval, regardless of calf survival to separation from mother, was 3.52 years (± 1.47 years sd, n=229), with a range of 1.02 to 10.05 years. Mean calving intervals when calves survived (n=105, 3.84 yr, CI 3.6-4.1) were significantly longer than when calves did not survive (n=124, 3.24 yrs, CI 3.0-3.5) (t-test for unequal variances, p=0.002). Mean calving intervals for successful cases preceding the birth of a male calf (mean = 3.67 ± 1.33 yrs sd, n = 28) were not significantly different from those preceding the birth of a female calf (mean = 4.18 ± 1.38 yrs sd, n = 35; t-test, 2 tails, p=0.14). Considering individual mothers, calving interval significantly increased with mother’s age (Figure 7A), whether a calf was successfully reared or not, and with birth order (Figure 7B) (Table 2). No significant effect was found for calf sex. Testing each of those parameters individually to take advantage of larger sample sizes, significant effects were found for mother’s age and whether calves were successfully reared but not for birth order or calf sex (Table 2).
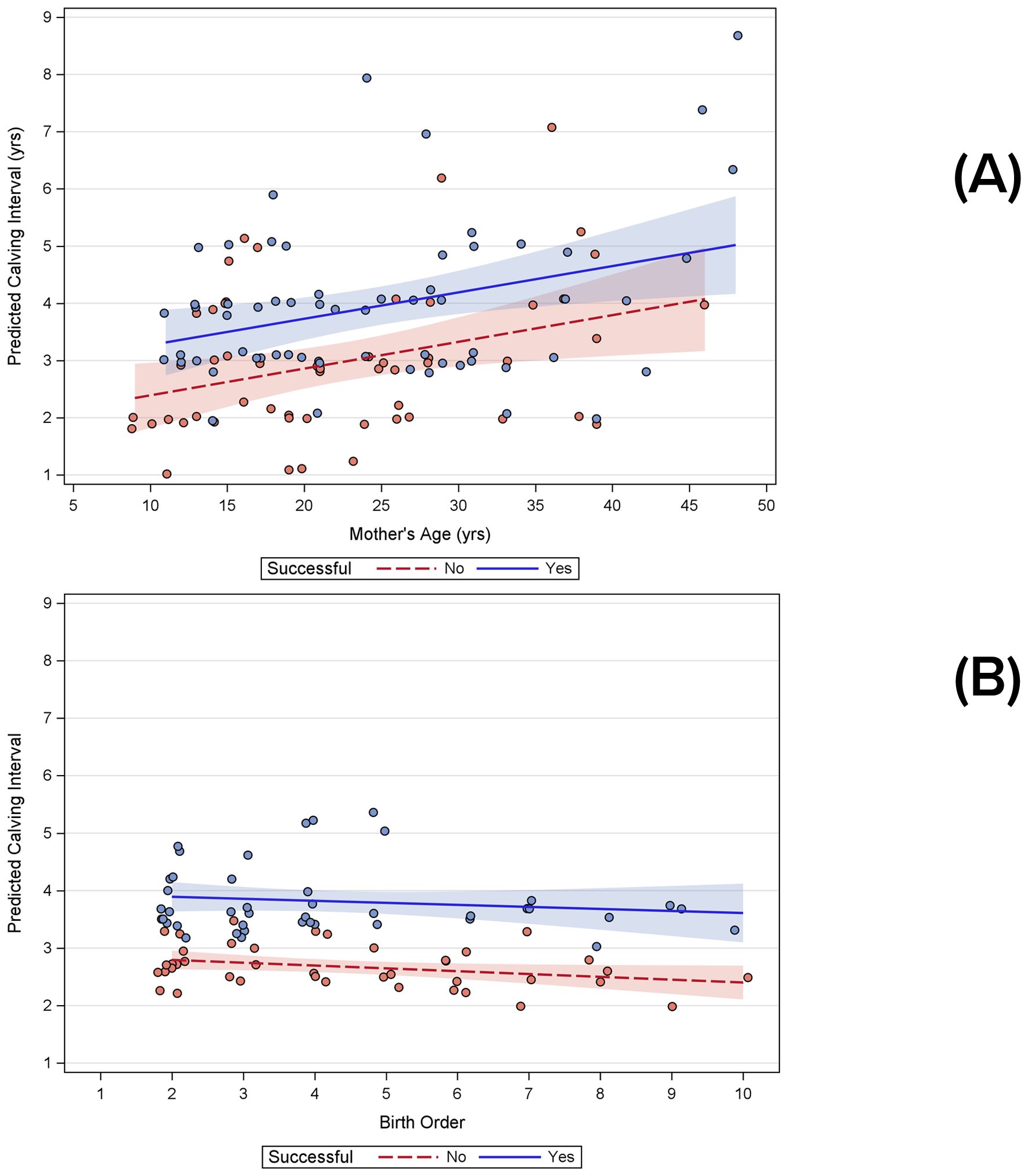
Figure 7. Results of general linear models showing significant influences on calving intervals both when calves were successfully reared or not: (A) increasing calving intervals as a function of age; (B) decreasing calving intervals with birth order as a function of birth order.
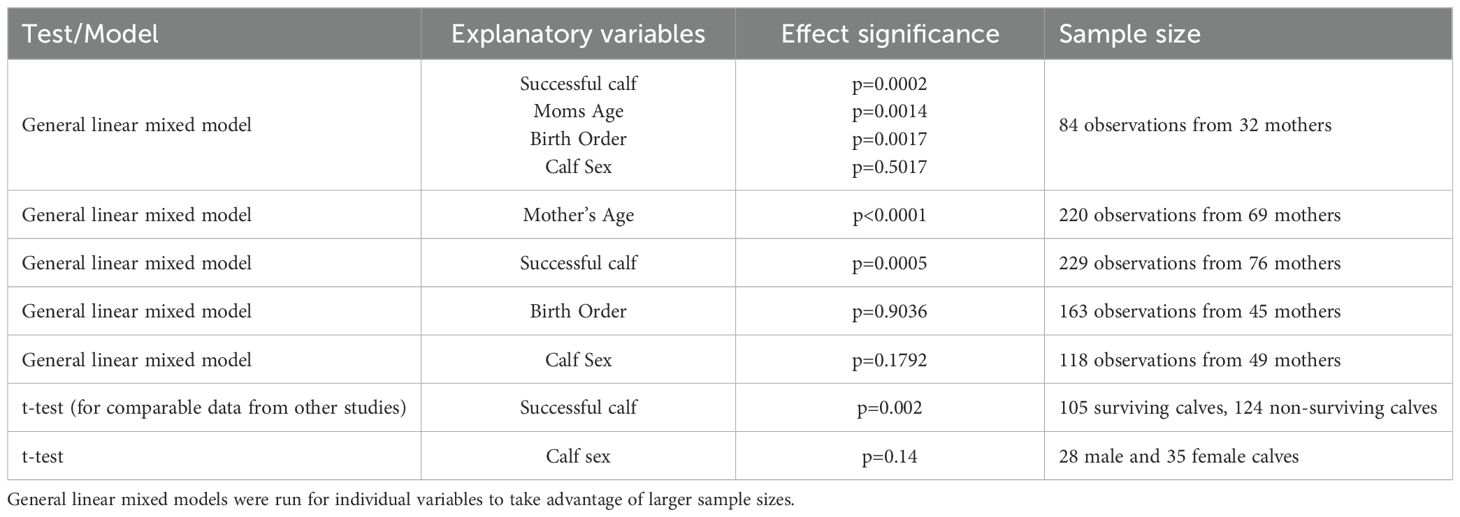
Table 2. Possible explanatory variables affecting calving intervals in bottlenose dolphins from Sarasota Bay, FL.
The mean length of time that mothers invested in successfully raising a calf was 4.38 years (± 1.75 years sd, n = 143) for the maximum duration of association based on the COA and 4.23 years (± 1.72 years sd, n = 142) for abrupt separations. These estimates were not significantly different, however the variability surrounding both of these means was high. This variability reflects different calf-rearing approaches both among different mothers and for an individual mother. In the 159 cases for which post-separation associations with mothers could be examined over the remainder of the separation year, separation was complete for 88 calves (55.3%), indicating abrupt separation rather than gradual. These calves were from 78 mothers, of which 33 (42.3%) were only observed to engage in abrupt separations from their calves.
In 51% of the cases for which it was possible to determine, separation of a previous calf occurred prior to birth of the next calf. On average, prior separations (based on COAs) occurred 1.72 years (± 1.29 years sd, n=126) before the next birth. Post-birth separations of previous calves occurred on average 0.72 years (± 1.08 years sd, n=121) after the birth. Individual mothers did not consistently engage in one pattern or the other across calves.
There were no sex-related differences with regard to whether calves separated from their mothers before or after the birth of the next calf. Successful male calves remained with their mothers, on average, 4.42 years (± 1.56 years sd, n = 64), while successful females remained, on average, 4.43 years (± 2.01 years sd, n = 58). Differences by sex were not found in calf length or calf mass obtained within ±1 year of separation (both sexes combined: mean length = 211.5 cm ± 16.7 cm sd, n = 70; mean mass = 114.5 kg ± 28.5 kg sd, n = 66) (Table 3).
Although there is much variability, the duration of association between mother and calf was most variable for younger mothers (the shortest and longest durations), with duration tending to increase with mother’s age, regardless of whether the separation was defined by COA values, or the timing of abrupt separation (Figures 8A, B). The pattern was similar for relative durations of association as a function of birth order, with the first births more variable (Figures 8C, D). Generally, this pattern appears to be manifested in calf sizes at separation, with calves being longer and heavier for younger and older mothers by the time they separate, and calves tending to be smaller at separation for mothers of intermediate age (Figures 9A, B). A similar pattern is evident for calf size at separation relative to birth order (Figures 9C, D).
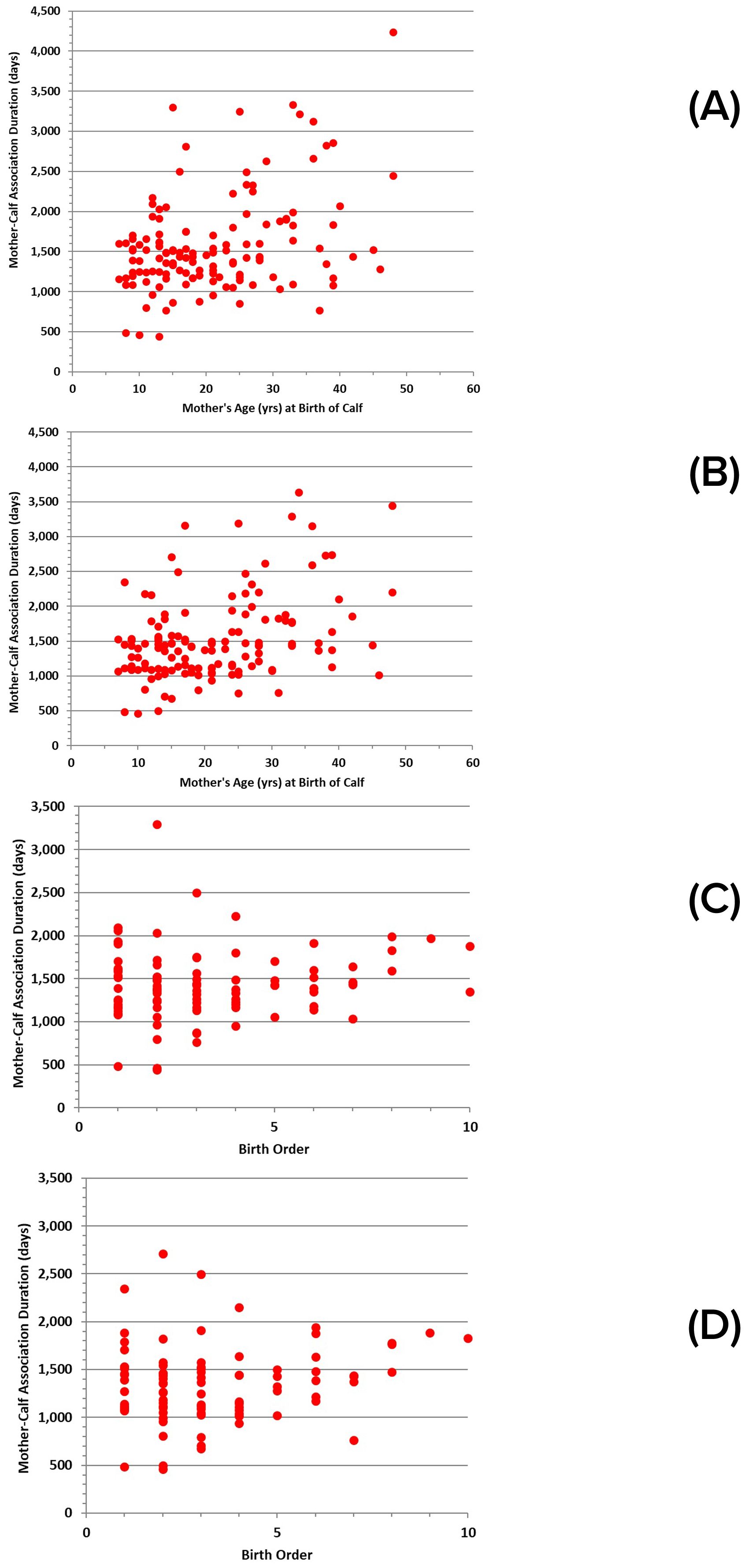
Figure 8. Duration of mother-calf associations for successful calves relative to: (A) mother’s age at the time of birth, based on COA measures (n = 135); (B) mother’s age at the time of birth, based on measures of abrupt separation (n = 134); (C) calf birth order, based on COA measures (n = 94); (D) calf birth order, based on measures of abrupt separation (n = 92).
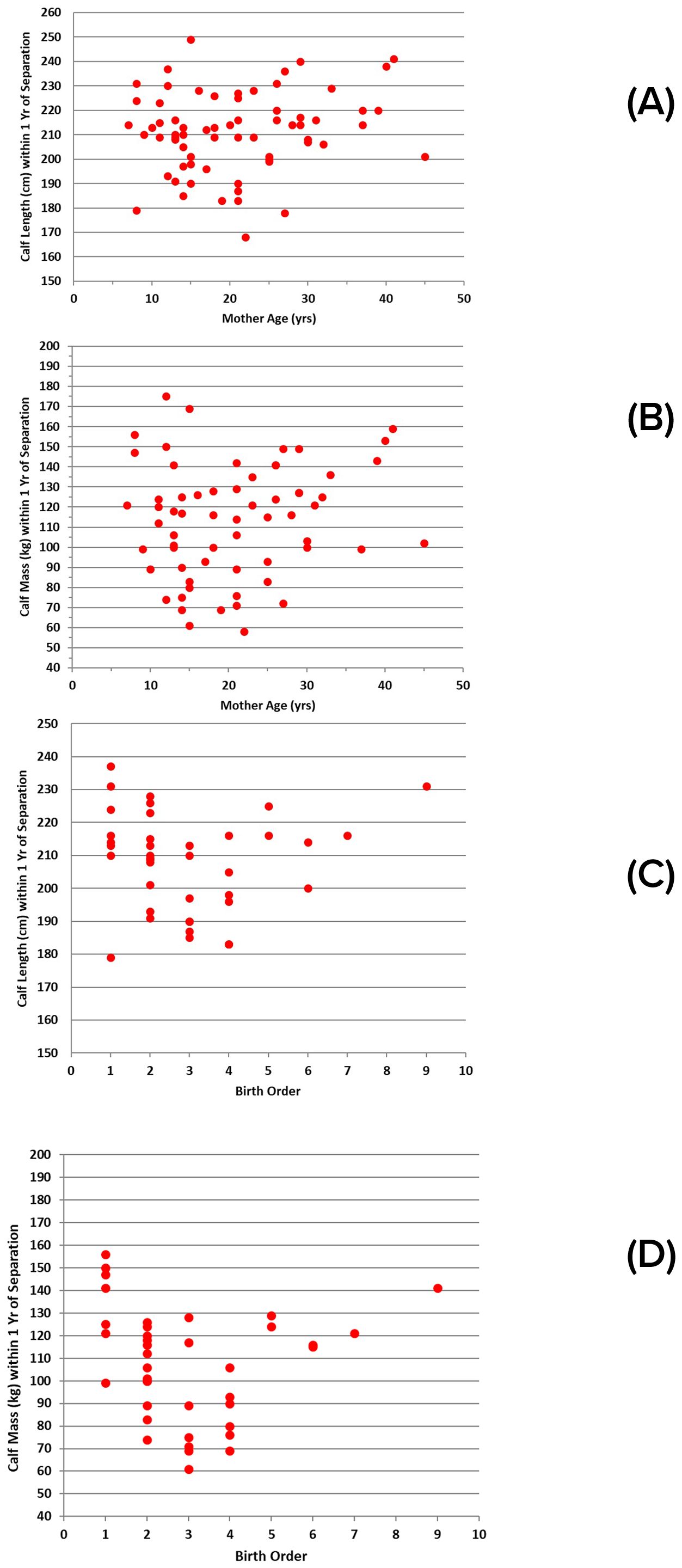
Figure 9. Sizes of successful calves within ±1 year of separation: (A) length relative to mother’s age (n = 67); (B) mass relative to mother’s age (n = 63); (C) length relative to birth order (n = 40); (D) mass relative to birth order (n = 39).
Calves were observed after the death of their mothers, but before indications of separation, in nine cases. The durations of association with their mother for calves that survived beyond separation ranged from 1.2 to 8.9 years. The fact that a third of the orphaned calves survived on their own after a period of association with mother of less than 1.4 years provides an indication that nutritional support is of less importance to the prolonged mother-calf bond than other factors.
Lactation was noted for 81 females during health assessments. They were accompanied by calves up to nine years in age. Two of the lactating mothers had calves >6 yrs old, and 16% of females had calves >3 years old. In each case, the period of association between mother and calf continued beyond the date that lactation was recorded. Thus, considering that calves remained with their mothers for more than 4 years, on average, many were likely remaining with their mothers well beyond the time when they were receiving milk.
Reproduction: reproductive lifespan
Females
Females as old as 48 years have been documented as mothers of newborn calves and successfully reared their calves (Figure 5B). Females from 9 yrs of age to their early 30s are responsible for most of the births. The birth frequency tapers off in later years due to fewer females remaining, extended calving intervals, and increased investment in each calf by older females (Figures 8A, B). The maximum number of calves produced by any given female within the selected dataset is 11, achieved by two mothers. They were 36 and 41 years old at the birth of their 11th calves. Subsequent to this study period, the older of these two mothers had a 12th calf, at 44 years of age. In two cases, females have had at least 6 of their calves survive beyond the point of separation from the mother.
Apparent reproductive senescence has been observed for 10% of all known-age mothers in Sarasota Bay. Senescence was assumed when a female’s calving interval exceeded 12.99 years, the maximum documented calving interval (10.05 yrs) plus two standard deviations (2.94 yrs). Nine females met this criterion, having lived 13 to 22 years past their last parturition.
Males
Males up to their mid-20s were most often identified as sires, in part due to the loss of males with age (Figure 5A). The oldest male documented as a sire was 43 years of age at the time of conception. The decrease in siring with age corresponded to a decrease in testosterone concentrations, from highs of 60-100 ng/ml in males up to 28 years of age, to less than 40 ng/ml after reaching 30 years of age, and in testis length, from a maximum of 30-35 cm up to about 27 years (n=156), to 20-30 cm for males 30 years and older (n=16) (Figure 4D).
Demographics: minimum abundance
Over the 27 years considered for these analyses, the number of individuals present in any given year ranged from 108 in 1993 to 184 in 2018 (Figure 10A). On average, during any given year (1993-2019), 77.71% (± 12.37 sd) of groups were completely identified, and 87.86% (± 7.82 sd) of dolphins seen in the study area were identified. During the most recent decade, the percentage of identified dolphins increased to 95.28%. The catalog of resident dolphins was well-established at the beginning of the analysis period in 1993, with new individuals added at a relatively stable average annual rate of 14.4 dolphins/year (± 4.99 sd) thereafter, through reproduction, immigration, or fins that became distinctive (Figure 10B).
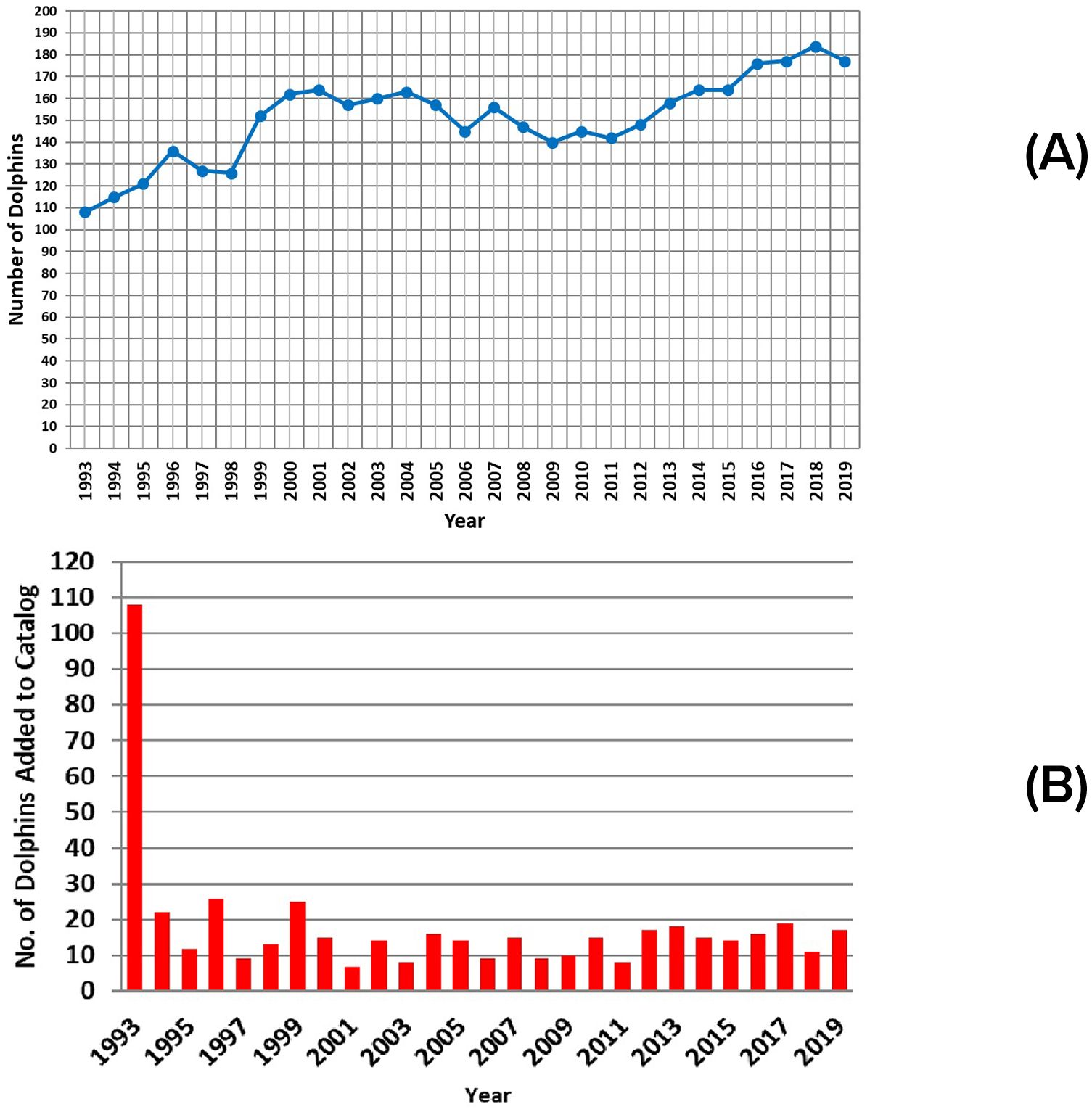
Figure 10. Annual changes in: (A) abundance; (B) numbers of identifiable resident dolphins present each year.
Demographics: Age-sex distribution
The age-sex distributions for 1993 and 2019 are shown in Figures 11A, B. The age-sex distribution of members of the community appears to be stable over time. A series of Kolmogorov-Smirnov tests found no significant differences between one year and the next, and no differences among the first year (1993), the middle year (2006), and the last year (2019) for which appropriate data were available.
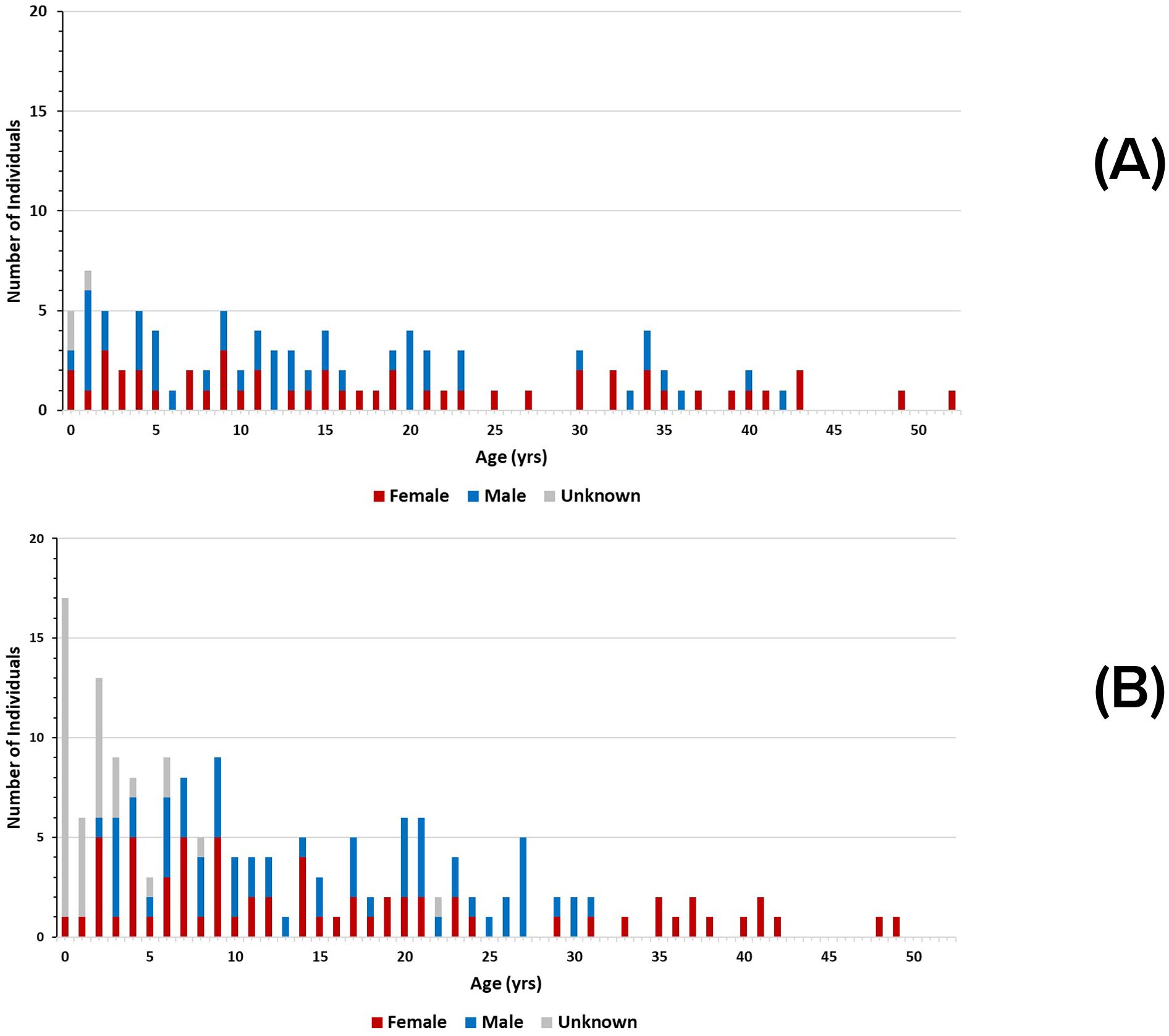
Figure 11. Age-sex histograms for: (A) 1993, and (B) 2019 for known-age dolphins in the Sarasota bottlenose dolphin community. The difference in the total number of individuals between the two years is due to a higher proportion of known dolphins in later years. There is an apparent gap in the 1993 distribution between the ages of 24 and 29. That gap, if projected backwards in time, coincides with removals of an unknown number of young dolphins from the population in the late 1960’s- early 1970’s for public display, research, and military applications. Note also in the distribution in 2019, the most recent year in the study, that there is a high number of young animals whose sex is yet to be determined. It often takes years to identify individuals to sex.
Demographics: birth rates and fecundity
In total, 268 births were documented during the 27-year study period. On average, 9.93 (± 4.39 sd, range 2-18/yr) calves were born each year. The mean annual birth rate was 0.071 births/dolphin (± 0.031 sd). It should be noted that, subsequent to compilation of the dataset used for these analyses, a record 22 calves were born to Sarasota residents in 2021.
The estimated number of mature females (6 years old and above) included in fecundity analyses in any given year ranged from 42 to 63. Mean annual fecundity was 0.182 (± 0.79 sd), ranging from 0.033 to 0.316. This value may be downward-biased by the low threshold for assigning maturity of 6 years, thereby likely including some females that were not yet mature, and by including females older than 48 years, beyond reproductive age. Removing females known to be older than 48 years of age yielded an estimated number of mature females (6-48 years old) in any given year ranging from 40 to 61, with a mean annual fecundity of 0.189 (± 0.81 sd), ranging from 0.036 to 0.321. It is possible that the dataset still included females of undetermined age older than 48 years.
Age-specific fecundity was examined for 241 calves born to known-age females (Figure 12A). In general, fecundity increases steadily from 7 years of age until females reach about 12 years of age, continues at a higher rate with no clear trend into the mid-20s, and then declines after about 25 years of age (Lacy et al., 2021).
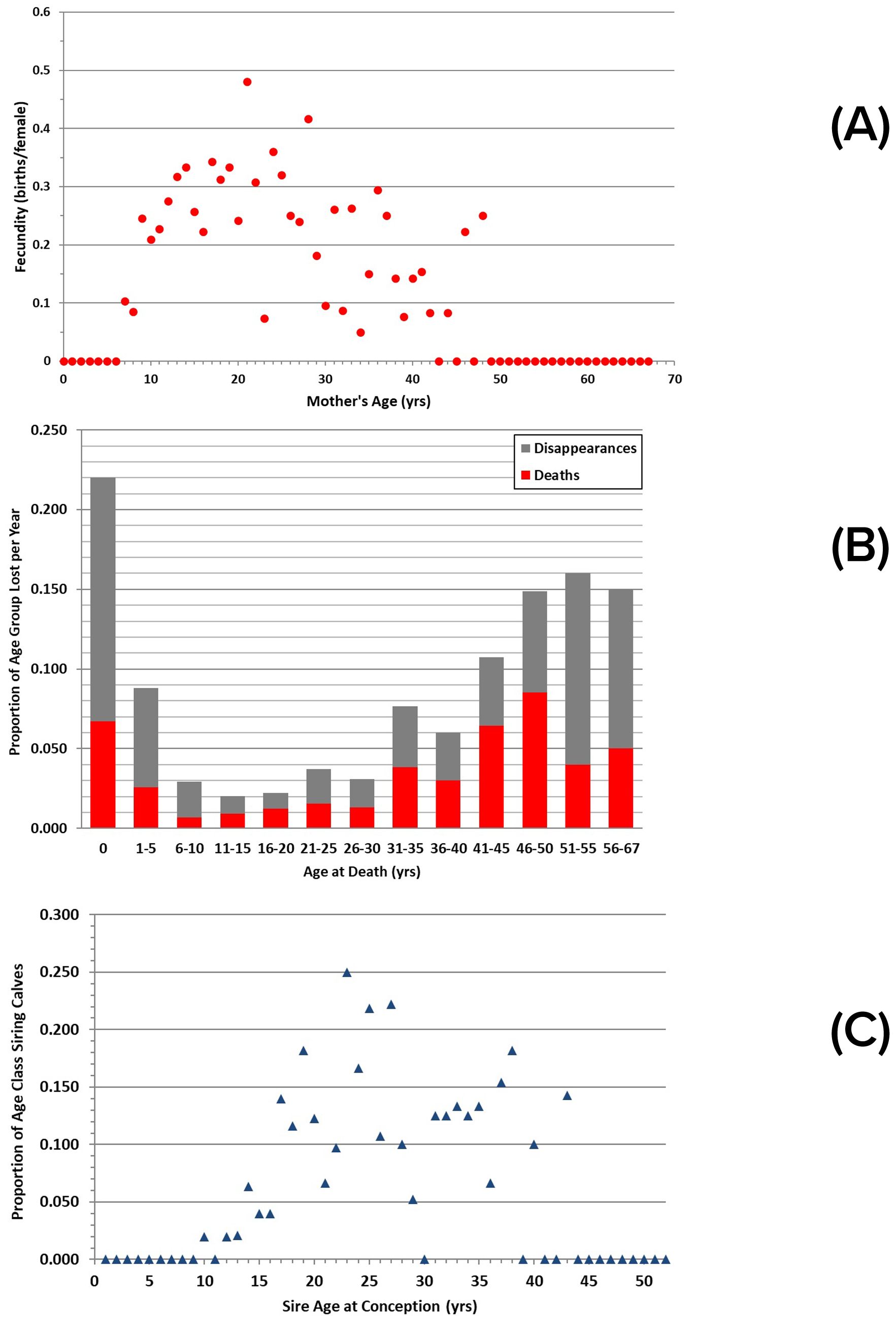
Figure 12. Age-specific parameters for Sarasota dolphins: (A) fecundity, measured as the summed number of births per female of a specified age (n = 68); (B) losses, measured as the number of documented deaths and disappearances at a specified age per dolphin of that age group, summed across the 27 years of analyses. Note unequal sizes of terminal age classes; (C) siring events, measured as summed number of calves per male of a specified age.
Demographics: recruitment
Recruitment through reproduction was calculated based on 268 calves born to females considered to be resident during the 27-year analysis period. Of these, 205 (77%) were documented to have survived through at least their first year of life. The mean annual recruitment rate through reproduction was 0.050 (± 0.023 sd), ranging from annual rates of 0.006 to 0.097.
Non-reproductive recruitment was more difficult to define precisely, and included documented immigrants and possible immigrants, involving 10.8% of the residents. Over the 27-year analysis period, 11 of 482 resident dolphins (2.3%) were documented as having moved to Sarasota Bay after having been first documented outside Sarasota. Another 41 dolphins (8.5%) were first documented as residents when they were non-calves. Using the known immigrants to provide a lower bound, and the combined documented and possible immigrants as an upper bound, the minimum annual immigration rate was 0.003 (± 0.005 sd) dolphins per year, ranging up to a maximum annual rate of 0.013 (± 0.011 sd).
Demographics: losses
Of the 482 dolphins in the 27-year dataset, 129 were documented as mortalities, including 66 individuals greater than one year old and 63 younger calves. Of the 129, 78 were of known age and sex. The average age at death for the 43 females was 17.7 years (± 18.5 sd), with a median of 12 years. For the 35 males, the average age at death was 18.3 years (± 17.2 sd), with a median of 16 years. The oldest female in the confirmed death dataset was 58 years; the oldest male was 50 years. For both sexes, dolphins with older age estimates have been documented in Sarasota, but they disappeared (female = 67 years, male = 52 years).
The mean annual minimum mortality (i.e., known and presumed deaths) of all dolphins was 4.82 (± 2.32 sd) deaths/year, including 2.33 (± 1.82 sd) deaths/year for first-year calves, and 2.44 (± 1.63 sd) deaths/year for all others. The mean annual minimum mortality rate over all dolphins was 0.032 (± 0.014 sd), ranging from 0.006 to 0.051 in any given year. The mean annual minimum mortality rate likely underestimates the true mortality rate, as 67% of dolphins disappear without being recovered as carcasses or identified as having emigrated. Of the 482 dolphins in the dataset, 162 disappeared without further record over the 27 years of the analysis period. Combined with the known mortalities, the mean annual maximum mortality rate was 0.072 (± 0.025 sd), ranging from 0.034 to 0.132 in any given year.
Loss rates vary with dolphin age, with the highest rates occurring among the youngest and oldest age classes (Figure 12B). The rate of confirmed mortality from recovered carcasses for young-of-the-year calves was 0.067, but the actual value is likely 0.22, as disappearances of such young calves are also considered mortalities. Mortalities declined and remained low, at ≤ 1.5%, from the 6-10 year class through the 26-30 year class, then increased. The apparent decline in loss rate after 51-55 years is likely an artifact of small sample size for individuals reaching those estimated ages. Disappearance rates by age class track known mortalities well, suggesting that many of the disappearances with no other explanation could reasonably be considered to be mortalities.
For four of the 482 residents (0.8%, 3 males, 1 female), it was possible to document range shifts indicative of probable emigration. Three of these cases involved shifting to estuaries to the north, and one to the south. The mean annual minimum emigration rate, based solely on documented cases, was 0.001 (± 0.003 sd), ranging from 0.000 to 0.012 for any given year. Combining known emigrations with all disappearances of unknown cause as possible emigration events, the mean annual maximum emigration rate was 0.041 (± 0.022 sd), ranging from 0.000 to 0.088 in any given year.
Reproductive success
Female reproductive success, measured as the proportion of calves seen post-separation, varies from none to all of their calves, with a mean of 0.45 (± 0.37 sd) for the 125 females for which information on calf presence post-separation was available. No surviving calves were identified for 28% of the 125 mothers, while all calves are known to have survived for 22% of the mothers. Two Sarasota females have successfully reared at least six calves each.
Overall, of 426 calves with documented birth year and known or presumed fates, including calves born prior to 1993, 78.2% survived their first year, 61.1% survived their first three years, and 48.4% were identified post-separation. Maternal experience appeared to play a role in reproductive success, as exemplified by analyses of the fates of 224 calves of known birth order. Only 62.3% of first-born calves survived their first year as compared to 79.5% of subsequent calves (Fisher Exact Test, chisq p=0.0168). Calves of multiparous females also showed higher, but not significant, survival at three years (60.5% multiparous, 54.7% primiparous, Fisher Exact Test chisq p=0.5205), and was near parity at post-separation (54.8% and 50%, Fisher Exact Test chisq p=0.8753).
Male reproductive success could be assessed for 52 cases where males were identified as the only possible sire for calves born to resident Sarasota females. The maximum number of calves sired was seven, documented for two males. Most of the calves were sired by males in their twenties to mid-thirties, with no males older than 43 years identified as sires (Figure 12C).
Discussion
The primary intent of this study is to make broadly available empirical data collected during more than 50 years of field studies on one community of bottlenose dolphins. We present an overview of how these data contribute to understanding the scope and range of life-history parameter estimates as determined from long-term observational or catch-and-release studies. While not attempting a comparison to life-history studies using carcasses only, we provide some perspective on differences between estimating parameters from long-term studies and those from dead animals as the results may not be comparable.
A distinctive characteristic of the study of common bottlenose dolphins in Sarasota Bay is that the duration of the study exceeds relevant time scales for observing difficult-to-study population characteristics. For bottlenose dolphins, estimating age at sexual maturation, lifetime age-specific reproductive output, or longevity requires multiple decades and generations. The results from Sarasota Bay offer a unique opportunity to understand dolphin populations. The more than 50-year span of this study matches the long lifespan of bottlenose dolphins, and the collection of large sample sizes and decades-long histories of individual dolphins have allowed the estimation of these variables. These estimates are relatively unbiased compared to estimates from examination of carcasses from strandings or fishery mortalities (e.g., Peltier et al., 2012; Barlow and Hohn, 1984). In addition, the scale of sampling, i.e., duration and sample size, allowed for documentation of the natural range of variability among individuals, reflecting the variability inherent in the population and the environment. These results, then, may provide a benchmark for comparison of results from studies of species with similar life histories.
In addition to the duration of longitudinal studies, knowledge of age is key to developing meaningful life-history and demographic parameters, and for interpreting health. Estimation of age of Sarasota Bay dolphins has been accomplished in several ways. The ages of most of the residents are known because individuals have been monitored since birth to well-known resident mothers (Scott et al., 1990; Wells, 2014). The ages of many others (only three after 2011) were determined from examination of growth layer groups in a tooth extracted under local anesthesia by a veterinarian (Hohn et al., 1989). Sarasota Bay has provided opportunities to test and refine this technique with animals of known age. Recently, Sarasota Bay has provided opportunities to test less-invasive methods to obtain age estimates, from development of radiographic examination of pectoral flipper epiphyseal closure (Barratclough et al., 2019b) or from growth layer groups of in situ teeth (Herrman et al., 2020), and from epigenetic analyses of skin or blood samples (Beal et al., 2019; Barratclough et al., 2021). These newer methods have the potential for increasing the sample size of known-age individuals in long-term studies (Barratclough et al., 2023).
Females
Some results from the Sarasota Bay study are not available from other dolphin longitudinal studies, e.g., lifetime reproductive success and longevity. However, other long-term studies have provided observational data to estimate, or place bounds on, age-specific demographic parameters that require long time-series as well as ages of known individuals. Age at first birth, or the related parameter, age at sexual maturation (ASM), is a parameter often estimated. For 11 female Indo-Pacific bottlenose dolphins observed in western Australia during 16 years, first birth for 4 females occurred at 12-15 years of age, while 7 females of the same age had not yet given birth (Mann et al., 2000). Similarly, in a 16-year study of common bottlenose dolphins in Doubtful Sound, NZ, 3 females of known age gave birth at 10.8-11.8 years of age, while 4 females over the age of 12 years had not yet calved (Henderson et al., 2014). In a 20-yr study of common bottlenose dolphins in Scotland, of 16 females known since birth, 11 gave birth at ages 8-9, while the youngest and oldest primiparous females were 6 years (n=2) and 13 years (n=1), respectively; with the assumption that no earlier births by the older female were missed, the mean was 8.2 years (Robinson et al., 2017). In a 16-year study in eastern Scotland, of 13 females sighted every year since birth, age at first birth ranged from 6-14 years (median=9) (Cheney et al., 2019). Indo-Pacific bottlenose dolphins under human care were determined to be mature on the basis of a detected ovulation or hormone levels as early as 6 years of age at one facility (Zhang et al., 2021; Brook, 1997), while first conception occurred at 11-13 years in a 10-year study at different facility, with age at maturation unknown (Cheal and Gales, 1991).
Although a different genus, for 22 female Amazon River dolphins observed during a 24-year study, first birth occurred at 7.2-12.4 years (mean=9.7), with 3 of the 76 mature females never having been seen with a calf (Martin and Da Silva, 2018). These results are similar to those from Sarasota Bay (for 54 resident females observed over 38 years, first birth ranged from 6 to 14 years, mean=9.6, with four older females never having been seen with a calf), resulting in Martin and Da Silva (2018) suggesting that some life-history traits are conserved; this similarity maybe be correlated with body size (Calder, 1996; Peters, 1986). The two longer-term studies (current study and Martin and Da Silva, 2018) were able to put upper bounds on age at first birth, while the shorter-term studies described above had not yet identified that bound, illustrating the length of study time needed to estimate age at first birth in late-maturing, long-lived species. They also illustrate the late age at which dolphins of this size become mature, on average, and the variation in age at first birth, with a range for Inia of 5 years and, to date, 8 years for Tursiops. These studies also illustrate the older ages at which some females have not yet given birth.
More data, and increased sample sizes, are available for parameters requiring a shorter time frame to observe and ages known only for calves. Two related parameters often estimated are calving interval (CI) (or inter-birth interval), and age at weaning. The CI is reported differently among studies, e.g., including or excluding intervals when a calf did not survive to weaning, limiting some direct comparisons and introducing different biases (Arso Civil et al., 2017; Cheney et al., 2019). Nonetheless, patterns emerge among studies. The CI ranges from as low as one year, when a calf was lost shortly after birth to as high as 10 years (current study, Mann et al., 2000; Henderson et al., 2014; Robinson et al., 2017) and is influenced by calf survival, mother’s age, birth sequence, timing of birth, group size, prior successful births, and other factors (current study, Henderson et al., 2014; Blasi et al., 2020; Robinson et al., 2017; Bezamat et al., 2020). Direct observations of a CI of 2 years for females successfully weaning calves are rare but have been reported (current study, Arso Civil et al., 2017; Bezamat et al., 2020; Cheney et al., 2019; Steiner and Bossley, 2008), as were CIs greater than seven years (current study, Mann et al., 2000; Arso Civil et al., 2017; Henderson et al., 2014; Robinson et al., 2017), all of the latter being recorded in field studies of greater than 17 years. A shorter range of observed CI was reported for shorter-term studies (Indo-Pacific bottlenose dolphins, range 1-6 years over an 8-yr period, Kogi et al., 2004; common bottlenose dolphins, range 1-2 years over two disjointed periods for a total of six years, Bezamat et al., 2020; common bottlenose dolphins, range 2-7 years over an 8-yr period, Baker et al., 2018), which may reflect actual differences in range of CI for those populations or be biased downward given the length of the studies (Barlow and Clapham, 1997; Arso Civil et al., 2017; Cheney et al., 2019).
Excluding the shorter-term, potentially negatively biased, studies noted above, the mean CI was similar among studies of bottlenose dolphins, ranging from 3.3 to 4.7 yrs (current study, Mann et al., 2000; Arso Civil et al., 2017; Henderson et al., 2014; Blasi et al., 2020; Robinson et al., 2017; Fruet et al., 2015). The lowest mean CI was 1.7 years, estimated from all birth intervals including lost calves, for a sample of five reproductive females observed during a 16-yr study, while CI for surviving calves of nine females was 3.8 years (Steiner and Bossley, 2008). When provided, the mean CI differed when estimated from all calving intervals or from intervals only when a calf survived, although the direction of difference varied among studies. The studies recognized that the mean CI will be positively biased if non-surviving calves were missed, which will be affected by the intersection of survey frequency and calving seasonality. The average age at weaning, when estimated, was the same as, or close to, CI for all of the studies. It should be recognized, however, that age at nutritional weaning and age at separation are likely to not be the same, as the period of association between Sarasota Bay mothers and calves continued post-lactation, a difference often not distinguished, and possibly not known, in most published studies.
The CI has been estimated for other small-cetacean species from long-term studies, as well. In a 24-yr study of Inia, the CI ranged from 2 to 7.7 years with a mean of 4.6 when including all calves sighted (Martin and Da Silva, 2018). Duration of nursing ranged from 1.5 to 5.8 years (mean=2.8 years). In an 11-year study of Stenella frontalis, the CI ranged from 1 to 5 years with a mean of 3.6 (all calves included) or 4.3 years (including only when a calf successfully weaned) (Herzing, 1997). In a 13-year study of beluga whales (Delphinapterus leucas), CI ranged from 2 to 13 years (McGuire et al., 2020). Zeng et al. (2021) estimated a range of 3.5 to 5.8 years for Sousa chinensis in a study lasting six years, suggesting the possibility of a downward bias in CI due to the relative length of the study.
Many studies report data pertaining to pregnancies or births, including crude or mean annual birth rate (the number of calves relative to the total number of individuals less the number of births) (e.g., Steiner and Bossley, 2008; Baker et al., 2018), fecundity, annual fertility rate (e.g., Pleslić et al., 2019; Cheney et al., 2019), and annualized birth rate when fecundity is corrected for length of gestation (e.g., Martin and Da Silva, 2018). Fecundity has variously been defined as the proportion of calves surviving to age 1 year relative to the number of mature females (also called annual recruitment rate) (e.g., Herzing, 1997; Zeng et al., 2021), annual proportion of females giving birth (e.g., Arso Civil et al., 2017; Baker et al., 2018), and the number of female offspring relative to the number of mature females (e.g., Fruet et al., 2015; Robinson et al., 2017). Direct comparison across studies is not possible because the parameters estimated and reported are non-standardized. However, given an unbiased estimate of CI (see Arso Civil et al., 2017), fecundity is the reciprocal of the CI and may be more readily estimated as such. For the various long-term studies, the reciprocal of the mean CI results in estimates of fecundity of 0.21 to 0.30, which is somewhat higher than the estimates of fecundity for the Sarasota Bay community (range 0.12-0.19, depending on estimation method). However, among studies, when both fecundity and CI are reported, the reciprocal of CI is often higher than the estimates of fecundity (current study, Kogi et al., 2004; Herzing, 1997), and can be incomparable (e.g., Bezamat et al., 2020; Fruet et al., 2015; Martin and Da Silva, 2018), which likely reflects the various ways the parameters are estimated or is due to undetected fetal or neonatal loss. Martin and Da Silva (2018) report a large difference in CI and pregnancy rate in Inia; however, if every detected pregnancy (via ultrasound) resulted in a detected calf, then the two parameters would have been comparable. For useful comparisons among populations, estimation methods for CI and reproductive parameters would need to be better standardized. That standardization would be especially helpful for parameters that can be estimated from shorter-term studies and that are used in population models, such as the use of age-specific reproductive rates in population viability analysis (PVA) (e.g., Lacy et al., 2021) or for correcting for data-driven biases (Arso Civil et al., 2017).
Reproductive success and output vary within communities, albeit with some commonalities among studies. Variation occurs in the number of calves per mature female and the survival of those calves, with some mature females not seen to successfully raise a calf to separation (full independence) while other mature females raise all of their calves to separation (e.g., current study, Henderson et al., 2014; Fruet et al., 2015). Further, primiparous females have been found to be less successful at raising calves to separation and multiparous females more successful, in general, although some primiparous females are more successful than others (current study, Reddy et al., 2001; Wells, 2000; Schwacke et al., 2002; Baker et al., 2018; Henderson et al., 2014; Blasi et al., 2020; Robinson et al., 2017; Cheney et al., 2019; Mann et al., 2000; Arso Civil et al., 2017). Most longitudinal studies report females having three or fewer calves, while the longer-term observations have resulted in a higher number (Robinson et al., 2017; Arso Civil et al., 2017), including in Sarasota Bay, where females have been observed through as many as 12 calves. While data on age-specific fecundity are limited due to the duration of longitudinal studies and knowledge of individual ages, reproductive output has been reported to increase initially, stabilize, then decline in older females (current study, Lacy et al., 2021; Robinson et al., 2017, and Perrin et al., 1977 from carcass studies). Adjustment of results from shorter-term studies, e.g., that extrapolate from primarily younger females, may not capture the full variation in the population nor age-specific differences in calving success or survival of mature females giving birth, nor account for reproductive senescence in older females. A population viability analysis (PVA) for Sarasota Bay dolphins found that population growth was most influenced by reproduction in peak breeding-age females (ages 12-25 years), along with calf and juvenile survival (Lacy et al., 2021), illustrating the importance of robust estimates of age-specific reproduction.
Examination of carcasses can provide data on some reproductive parameters, although such results are not necessarily comparable to data from observational or catch-and-release studies. For large and presumably unbiased samples, pregnancy rates can be estimated on the basis of the relative number of fetuses (e.g., Perrin and Donovan, 1984; Murphy et al., 2009). Otherwise, counts of ovarian scars from (presumed) pregnancies (corpora lutea or albicantia) have been used to estimate the number of possible births (see Perrin and Donovan, 1984; Murphy et al., 2009), although the interpretation may be complicated with species differences (Dabin et al., 2008). A valuable opportunity to compare counts of ovarian corpora to observed births in three free-ranging female Indo-Pacific bottlenose dolphins with known life histories resulted in an equal number of corpora and births (Kemper et al., 2019), and for a female Indo-Pacific bottlenose dolphin under human care and undergoing frequent ultrasound examinations the number of corpora albicantia equaled the number of calves born (n=3) and not the number of ovulations (n=18) (Brook et al., 2002). There was no opportunity to evaluate corpora persistence following fetal loss. However, caution is needed in translating pregnancy rates from carcasses to successful reproduction. For example, Martin and Da Silva (2018) found that the pregnancy rate, as detected using ultrasound, was twice the annual birth rate (0.22) based on the sighting of calves. While some calves may have been missed during visual surveys, the difference between the two parameters suggests significant fetal loss. In Sarasota Bay, 83% of fetuses observed in ultrasound exams were subsequently observed as living calves, suggesting a fetal loss of up to 17% (Wells et al., 2014). Further, fetal loss, as well as loss of the first calf, is amplified by high contaminant burdens in mothers, including exposure to chemicals from oil spills (e.g., Wells et al., 2005; Schwacke et al., 2002; Reddy et al., 2001; Lane et al., 2015) and likely would vary among geographic areas. For example, in Barataria Bay, Louisiana, where bottlenose dolphins were exposed to the Deepwater Horizon oil spill, only 20% of fetuses were subsequently observed as calves (Lane et al., 2015). As a result, calving rates may be overestimated, and age at first birth may be underestimated in studies from carcasses, as lost fetuses from early pregnancies would delay successful births, thus decreasing calving rates and increasing the actual age at first birth. While data-based biases occur for both observational or catch-and-release studies and for studies using carcasses, the biases are different, and studies from carcasses may suggest higher population growth rates or potentials than are likely.
While age at first birth is more important for estimating population growth, for populations with short-term or no longitudinal studies, which is most populations of cetaceans, the ability to estimate ASM from carcasses may be useful. ASM is more conservative and less subject to the range of environmental influences than age at first calving (Millar and Zammuto, 1983; Stearns, 1983; Ferguson and Higdon, 2013; Peters, 1986; Calder, 1996; 1996, Sibly and Brown, 2007). In a representative sample, this estimate may be more accurate than the estimate from observational studies, which would be age at first birth minus the gestation time, given the evidence of fetal and neonatal losses in younger females. However, given actual or potential biases in carcass samples, results from longitudinal studies may provide guidance for when a sample is adequate for estimating a robust ASM from carcass samples. In particular, one result from estimates of age at first birth from observational studies is that, within a population, there is a protracted range of ages over which first birth (and resulting ASM estimated from age at first birth) occurs. This results in a number of indeterminate age classes, which are age classes for which maturity cannot be assigned by age alone. Samples not including the full range of indeterminate ages are likely biased, with many sample age distributions tending toward younger animals or smaller size (e.g., Barlow and Hohn, 1984; Moore and Read, 2008; Venuto et al., 2020; Berner and Blanckenhorn, 2007), which would result in a downward bias. In these cases, the sample is simply inadequate to obtain a robust estimate of ASM.
As has been reported for several species of small cetaceans, apparent reproductive senescence was documented for 10% of all known-age mothers in Sarasota Bay, with the onset ranging from 25 to 48 years of age. In short-finned pilot whales (Globicephala macrorhynchus), females live many years beyond the age of last reproduction (Kasuya and Marsh, 1984). In resident killer whales (Orcinus orca), reproductive senescence can last more than 50 years, starting at 40 years of age (Foster et al., 2012). Post-reproductive females can serve as repositories of important information gleaned from decades of experience, including such things as knowledge of resources and how to acquire them, predators and how to defend against them, and social knowledge that can help in navigating social interactions (grandmother hypothesis, Johnstone and Cant, 2010; Croft et al., 2017). Further, the existence of post-reproductive females may increase cooperation behavior (Ross et al., 2015) and stabilize population dynamics (Mitteldorf and Goodnight, 2012). It has been demonstrated that post-reproductive female resident killer whales increase the fitness of their kin through transfer of ecological knowledge (Ward et al., 2009; Brent et al., 2015). In resident killer whales, direct application of this information to the benefit of long-term, related swimming associates is possible. The evolutionary advantages derived from the continued existence of Sarasota Bay females in their fluid society well beyond their reproductive years remain to be determined.
Males
Adult male Sarasota bottlenose dolphins can be easily distinguished from immature males based on testosterone concentrations in serum and blubber, with adult males having 300 times greater mean serum concentration than immatures, and 100 times greater in blubber (Sherman et al., 2021). Testis volume was highly correlated with blubber testosterone, with mature males having 60 times greater volume than immatures (Sherman et al., 2021). The short period of time over which maturation occurs means that few males would be expected to have been indeterminate with respect to maturation.
Estimates of age and length at maturation in male dolphins from long-term observations are rare. Over 10 years of observations, three male Indo-Pacific bottlenose dolphins under human care were estimated to be 11 to 12 years old when they first sired calves (Cheal and Gales, 1991). In contrast, using the first presence of sperm in semen to define sexual maturity, five Indo-Pacific bottlenose dolphins monitored for 3 to 5 years matured at ages 6.6 to 7.3 years (Yuen, 2007). Estimates of age at first siring and age at first sperm production for a population are quite different parameters given the delay in siring for males, and, as noted earlier for females, neither is likely to be directly comparable to the ASM estimated from carcasses. Although a mean age at first siring is not available, age at maturation for Sarasota Bay males would be less than age at siring (i.e., social maturation), given that the youngest sires are 10 years of age at conception and most siring occurs when males are in the mid-teens and older.
Sarasota males matured later than females, both for age at first reproduction and ASM. Length and mass at maturation in males were not different from age at first calving and first birth in females, although these values are not equivalent as females would have an additional year of growth, and thus, it would be expected that they were smaller one year prior. Male-biased sexual dimorphism in asymptotic size occurs in most delphinids (Caspar and Begall, 2022; Ralls and Mesnick, 2009; Tolley et al., 1995; Moller et al., 2007; Möller, 2012) and does occur in the Sarasota Bay dolphins (Read et al., 1993). Most of the data supporting dimorphism in age or length at maturation are from carcass studies (e.g., Murphy et al., 2009; Betty et al., 2022; Ngqulana et al., 2017) rather than studies of free-ranging individuals; in carcass studies, direct comparisons of dimorphism would be comparable provided the sample size is sufficient.
Law (1956) proposed that sexual maturation in male delphinids is attained at 93% of total length. Sergeant et al. (1973) proposed that it occurs at 90% of total length in bottlenose dolphins. Cheal and Gales (1991) documented maturation to occur in male Indo-Pacific dolphins under human care coincident with the secondary growth spurt. The value from Sarasota Bay dolphins is similar to other published values, with predicted LSM occurring at 89% of asymptotic length. Body size and age at maturation are linked (Stamps et al., 1998; Sibly and Brown, 2007) so using a robust growth curve may be helpful to evaluate whether an ASM estimate is reasonable.
After sexual maturation, there is significant variability in serum testosterone levels and testis dimensions relative to age and body size in Sarasota Bay males. Variability in these parameters has also been documented in dolphins under human care (Yuen, 2007; Yuen et al., 2009; Brook, 1997; Schroeder and Keller, 1989). In three of four “elderly” Indo-Pacific bottlenose dolphins under human care ranging from 47 to 50 years of age, Koga et al. (2019) found testosterone levels and sperm concentrations to still be relatively high and suggested that even older dolphins retained their ability to breed. The results from mature males in Sarasota Bay were similar; however, there is an apparent decline in testosterone after about age 30, as well as a decline in siring frequency a few years earlier. The oldest documented actively breeding male in Sarasota was 43 years old, 9 years younger than the oldest documented male, suggesting that successful breeding does not continue through a male’s entire adult life. Whether the decline in siring is due to social competition or insufficient production of sperm or semen is unknown. The potential role of accumulated environmental contaminants in the decline is also unknown, as concentrations of some of these are known to exceed thresholds for health and reproductive impacts in some older Sarasota males (Wells et al., 2005).
Seasonality in reproductive parameters of male dolphins is common among sampled populations (Plön and Bernard, 2007). Studies on Tursiops spp. under human care have found elevations in testosterone levels, sperm counts or density, and/or other metrics over periods as brief as a couple of months during the year (Schroeder and Keller, 1989; Mingramm et al., 2019; Katsumata et al., 2017; Koga et al., 2019; Yuen, 2007; Wells, 1984; Funasaka et al., 2011). Schroeder and Keller (1989) have shown that peak testosterone levels preceded peak sperm density in bottlenose dolphins under human care. In Indo-Pacific bottlenose dolphins under human care, Brook (1997) and Yuen (2007) found a correlation between testosterone levels and testis volume that was cyclical, coinciding with ovulation in females, although Yuen (2007) found no annual pattern in sperm density. Seasonal changes in testosterone levels in blubber samples have been demonstrated from free-ranging bottlenose and short-beaked common dolphins (Sherman et al., 2021; Kellar et al., 2009; Boggs et al., 2019). While only limited sampling in Sarasota Bay occurred outside of the breeding season, the results are consistent with a seasonal increase in testosterone, coincident with breeding season (Sherman et al., 2021). The use of ultrasound also allowed detection of a decrease in testis size with lower testosterone levels outside of the breeding season, as was reported for dolphins under human care.
Determining maturation and seasonality of reproduction in small cetaceans has predominantly been based on carcass studies. Studies with an adequate sample size, especially in indeterminate age classes for sexual maturation, have confirmed the dimorphism in average ASM and LSM between males and females. Two differences are relevant with regard to defining ASM and LSM in males and females, however. First, while a female is considered to be clearly mature when at least one corpus of ovulation is present on at least one ovary, the precise timing of maturity in males is ill-defined with no single criterion widely applied (Perrin and Henderson, 1984, Plön and Bernard, 2007; Yuen, 2007). Applying models that estimate or predict ASM or LSM using the proportion mature in each age class based on criteria generally known to indicate maturity, such as testosterone levels, testis size, or sperm presence, should still provide reasonable estimates for males if the sample is sufficient. Second, for longitudinal studies such as in Sarasota Bay, ASM or LSM will not be known for females based on first ovulation and will need to be estimated from age at first birth, which, as discussed above, is likely to overestimate ASM. In contrast, estimates for males are likely to be comparable to those from carcass studies. Thus, dimorphism in average ASM and LSM may be underestimated and not directly comparable to estimates determined for males and females from carcasses.
Seasonal changes in testis dimensions, hormone levels, or histological features have been shown for a number of small-cetacean species from studies using carcasses (Hohn et al., 1985; Read, 1990; Slooten, 1991; Van Waerebeek and Read, 1994; Hohn et al., 1996; Fisher and Harrison, 1970; Collet and Saint Girons, 1984; Neimanis et al., 2000; Desportes et al., 1994). In addition, studies from carcasses of long-finned (Globicephala melas) and short-finned pilot whales, allowing for morphometric and histological examination as well as measurement of testosterone levels, showed that testosterone levels were not correlated with testis mass (Kita et al., 1999) or body length or age after sexual maturation (Desportes et al., 1994). Results from a large sample of pantropical spotted dolphin carcasses showed that maximum testis size peaked prior to the predicted mating season, while spermatozoa levels were elevated during the breeding season (Hohn et al., 1985). These estimates are more straight-forward than estimating reproductive parameters for females, as testis size and spermatogenesis should provide a non-biased snapshot for males if the sample size and seasonal distribution of samples are adequate, and given the constraint that maturation in males is not uniformly defined. For those parameters measured in longitudinal studies and from carcasses, however, the results on seasonality should be generally comparable.
Sarasota males were determined through paternity testing to be sires of up to seven calves each within the Sarasota dolphin community (and they could have sired more beyond Sarasota; Duffield and Wells, 2023). Members of strongly bonded male pairs sired 75% of calves, and individual males within pairs were closely matched for age, length, mass, and association history with the females (Owen et al., 2002; Duffield and Wells, 2023). Duffield and Wells (2023) examined characteristics of males seen in association with females during periods of presumed female receptivity to identify features associated with breeding success. Breeding males were characterized by age, in that 77% of sires were older than the mothers, on average exceeding mother’s age by more than five years, in spite of the fact that females can still produce calves at 48 years of age, and very few males live that long (Lacy et al., 2021; Wells, 2000). Successful sires were significantly older and heavier than non-sire associates during the period of female receptivity (Duffield and Wells, 2023). Taken together, these observations suggest that some form of male-male competition and male dominance may be in play, within a polygynandric system that involves mate-guarding.
Demography
The demographic structure and dynamics of the Sarasota community can serve as a model for other cetacean communities. The number of Sarasota dolphin community members identified in any given year has been variable, ranging from 108 in 1993 up to 184 in 2018 (Figure 10A). This increase occurred in large part after a 1995 banning of fishing nets in inshore Florida waters that captured many of the dolphins’ prey fish species and occasionally killed dolphins. Declines from one year to the next often coincided with or followed major environmental perturbations, such as severe harmful algal blooms (Karenia brevis red tides) that depleted prey and/or increased predation pressure or increased adverse human interactions (Gannon et al., 2009; Berens McCabe et al., 2021; Wells et al., 2019). In recent years, the increase in numbers of identifiable Sarasota dolphin community members appears to have slowed, suggesting that the community may be reaching its carrying capacity (Lacy et al., 2021). The current community size of up to about 200 individuals including both identifiable and unidentifiable dolphins is comparable to sizes identified for a number of other bay, sound, and estuary regions along the Gulf coast of Florida. For example, the estimated 524 dolphins of Tampa Bay were spread among four different communities (Urian et al., 2009). Multiple communities likely exist in the Charlotte Harbor and Pine Island Sound region, where about 826 dolphins were estimated to occur (Bassos-Hull et al., 2013). A resident community of 78-152 dolphins was identified in St. Joseph Bay (Balmer et al., 2008).
Some vital rates, such as immigration and emigration, are difficult to measure in the absence of long-term study and data collection beyond the geographic range of the focal community, so data for comparison to Sarasota are lacking. The low estimated mean rates of immigration (0.003-0.013), emigration (0.001-0.041), and occasional movements of breeding males among communities are sufficient to maintain genetic exchange with neighboring communities (Duffield and Wells, 2023). Data on parameters such as estimated birth rates and mortalities are generated from within a community, providing more opportunities for comparisons. The Sarasota mean annual birth rate of 0.071 falls within the range of previously reported bottlenose dolphin values of 0.012 to 0.156 (Leatherwood and Reeves, 1982; Perrin and Reilly, 1984). For Sarasota dolphins across all age classes, mean annual mortality rates were estimated as 0.032-0.072, recognizing that the upper limit is biased upward due to including losses from all causes, including undocumented emigration events and changes in identifying characteristics. Comparable or higher loss rates have been reported from other sites. Off Charleston, South Carolina, the estimated annual loss rate is 0.049, without being able to distinguish between mortality and permanent emigration (Speakman et al., 2010). Ludwig et al. (2021) estimated an annual loss rate of 0.06 for the Shannon River estuary in Ireland. Stolen and Barlow (2003) estimated an overall annual mortality rate of 0.098, based on stranding data. In the southwestern Gulf of Mexico, estimated annual loss rate was about 0.12 (Bolaños-Jiménez et al., 2021). Around Reunion Island, the apparent annual loss rate was 0.17 (Estrade and Dulau, 2020). For perspective, a loss rate of 0.132 was estimated for the Barataria Bay, Louisiana, population of bottlenose dolphins impacted by the Deepwater Horizon oil spill (Lane et al., 2015).
Calf losses varied with maternal experience, and from site to site, with young-of-the-year typically suffering the highest losses. Overall, of 426 Sarasota calves with documented birth year and known or presumed fates, 78.2% survived their first year, 61.1% survived their first three years, and 48.4% were identified post-separation. Only 62.3% of first-born Sarasota calves survived their first year, as compared to 79.5% of subsequent calves. Along the eastern Adriatic coast, 87.5% of calves survived their first year (Pleslić et al., 2021). In Doubtful Sound, New Zealand, 67% of calves survived their first year, and 40% survived through their first three years (Henderson et al., 2014). In Bay of Islands, New Zealand, first-year mortality was 0.34-0.52 (Tezanos-Pinto et al., 2015). First-year Lahille’s bottlenose dolphin calves (T. t. gephyreus) were lost at a rate of 0.16 (Fruet et al., 2015). Environmental contaminants have been suggested as a potential contributor to elevated losses of first-born calves (e.g., Schwacke et al., 2002; Wells et al., 2005).
Population growth rates have been estimated for a few bottlenose dolphin populations. Lacy et al. (2021) estimated a long-term (27 years) mean annual population growth rate of 0.021 (sd = 0.031%) for the Sarasota dolphins, noting that population growth was most sensitive to uncertainty and annual variation in reproduction of peak breeding-age females and in calf and juvenile mortality, with little variation in adult survival over time. Stolen and Barlow (2003) estimated a rate between 0.00 and 0.046 for Tamanend’s bottlenose dolphin (T. erebennus) from a life table based on stranding data in the Indian River Lagoon of Florida. A growth rate of -0.007 was reported for the 145-dolphin population in the Shannon River Estuary of Ireland (Blázquez et al., 2020). Given the slow rates of population growth for bottlenose dolphin populations, it is estimated that the population of dolphins in Barataria Bay, Louisiana, will require 35 years to recover from the Deepwater Horizon oil spill (Schwacke et al., 2022). It was estimated that a resident estuarine population of Lahille’s bottlenose dolphin in southern Brazil would grow at a rate of about 0.03 annually in the absence of bycatch (Fruet et al., 2021). Most measured growth rates are less than the predicted growth rate of 4% commonly used for delphinid population growth (Reilly and Barlow, 1986).
In the late 1960s-early 1970s, bottlenose dolphins, typically young females, were collected from many parts of the southeast U.S., including Sarasota waters, for public display, research, and military applications (Leatherwood and Reeves, 1982). There was little appreciation at the time that these populations of bottlenose dolphins were relatively discrete, small and resident to the coastal bays and estuaries. As such, removals that targeted specific bays and specific age cohorts had the potential to create a long-lasting gap in the age distributions. Gaps were apparent in the age distribution (see Figure 11 for the year 1993) that coincided with the collections, projected through time. It might be expected that this gap, as it projected through the prime female breeding ages, could have had effects on calf production. No such effect was observed, however, likely due to the confounding effects of high annual variability in calf production, a changing carrying capacity after an inshore gillnet fishing ban was enacted in Florida waters in 1995, and developing climate change.
Conclusions
The long-term observational and catch-and-release research on bottlenose dolphins in Sarasota Bay has produced foundational information for understanding the life history, reproduction, and demography of bottlenose dolphins and similar species (due to allometric relationships). These findings are important for informing management actions and strategies for conservation. They can help illuminate the basis for social structure. In concert with other very long-term studies of dolphins, the results identify the scale of life-history parameters, along with variability, and provide benchmarks for comparisons with other populations, whether from observational or carcass studies.
In summary:
● Females younger than 5 years old were immature, those older than 13 were mature, and age classes 5-13 years were indeterminate, containing both immature and mature females. The average age when females were observed with their first calf was 9.6 years, yielding a mean age at sexual maturation of 8.5 years. Model predictions estimated the age at first calving of 8.8 years and the age at sexual maturation of 7.8 years.
● Males younger than 9 years old were immature, those older than 11 years old were typically mature, and the age classes 9-11 were indeterminate. Results from testosterone concentrations, occurrence of sperm, genetics, and modeling predictions all indicate an age of maturation of 10 years.
● Sarasota dolphin reproduction is seasonal with most calves being born during May-July. Reproductive hormones in blood peaked at the beginning of the breeding season, consistent with a 12.5-month gestation period.
● The average calving interval was 3.5 years with a range of 1-10 years, which varied with mother’s age and whether calves were successfully reared or not. For calves that were successfully reared, two different methods showed that the average time that mothers invested in these calves exceeded 4 years.
● Calf mass, but not calf length, significantly increased with birth order.
● On average, 45% of a female’s calves reached the age of separation from mother.
● Females as old as 48 years have successfully given birth and raised calves. However, apparent reproductive senescence has been observed for 10% of all known-age mothers that ranged in age from 38-61 years old.
● Males up to their mid-20s were most often identified as sires, with the oldest sire being 43 years of age at the time of conception. Individual males, on average, were each responsible for 2.62 of the known paternities and the maximum number of calves sired was seven. At about 30 years of age, testis size and testosterone concentrations decreased.
● The community size has slowly increased, from 108 in 1993 to 184 in 2018. This increase occurred in large part after a 1995 banning of fishing nets in inshore Florida waters that captured many of the dolphins’ prey fish species and occasionally killed dolphins.
● The mean annual birth rate was 0.071 births/dolphin. Fecundity estimates ranged from 0.182 to 0.189 calves born per mature female, depending on assumptions about ages of maturity and senescence.
● The average annual recruitment rate through reproduction was 0.050 dolphins. The minimum annual immigration rate was 0.003 dolphins, and the maximum was 0.013 dolphins.
● The mean minimum annual mortality rate was 0.032 and the mean maximum annual mortality rate was 0.072. The mean annual mortality rate for young-of-the-year calves is 0.22. The mean annual minimum emigration rate was 0.001. Combining known emigrations with all disappearances of unknown cause as possible emigration events, the mean annual maximum emigration rate was 0.041.
Author contributions
RW: Methodology, Project administration, Resources, Supervision, Visualization, Writing – original draft, Writing – review & editing, Conceptualization, Data curation, Formal analysis, Funding acquisition, Investigation. AH: Conceptualization, Formal analysis, Investigation, Methodology, Visualization, Writing – original draft, Writing – review & editing. MS: Conceptualization, Formal analysis, Funding acquisition, Investigation, Methodology, Resources, Visualization, Writing – original draft, Writing – review & editing. JS: Funding acquisition, Investigation, Methodology, Resources, Writing – review & editing. FT: Investigation, Methodology, Writing – review & editing. JA: Data curation, Investigation, Writing – review & editing. AB: Investigation, Resources, Writing – review & editing. KM: Investigation, Writing – review & editing. KB: Investigation, Writing – review & editing. GL: Data curation, Formal analysis, Investigation, Methodology, Resources, Writing – review & editing. DD: Data curation, Formal analysis, Investigation, Methodology, Writing – review & editing. CS: Data curation, Formal analysis, Investigation, Methodology, Resources, Writing – review & editing. AI: Conceptualization, Funding acquisition, Investigation, Methodology, Resources, Writing – review & editing.
Funding
The author(s) declare that financial support was received for the research, authorship, and/or publication of this article. We are grateful to NOAA’s National Marine Fisheries Service, Earthwatch Institute, Dolphin Quest, Inc., the Charles and Margery Barancik Foundation, The National Marine Mammal Foundation, Don and Lee Hamilton, Edward McCormick Blair, Jr., Elizabeth Moore, Rick Moskovitz, and Disney for significant, long-term, repeated, and/or ongoing support. Disney was not involved in the study design, collection, analysis, interpretation of data, the writing of this article or the decision to submit it for publication. The Irvine family provided support specifically for the analyses in this paper.
Acknowledgments
We would like to dedicate this paper to Dr. William Perrin; we thought of him reading over our shoulders as we wrote the paper and we think he would have enjoyed it. We stand on the shoulders of giants… Research spanning more than 50 years has required and benefitted from the involvement of many people, too numerous to include as co-authors or to acknowledge individually here. Co-authorship was decided on the basis of having: 1) conceptualized and/or designed and/or found funding for the research, 2) been instrumental in essential data/sample collection, archiving, processing, and/or analyses for 15 years or more, 3) had crucial and unique roles in providing data on genetics, life history, and/or mortality, and/or 4) done the majority of the writing. We would like to thank the more than 1,000 staff, students, interns, volunteers, dolphin handlers, veterinarians, citizen scientists, and collaborators of the Sarasota Dolphin Research Program (SDRP) for their contributions to the fieldwork and analyses. Nearly every member of SDRP staff over the decades collected photo-identification data in Sarasota Bay, and many also participated in photo-analysis. Kim Urian, Sue Hofmann, and Stephanie Nowacek contributed greatly to the development of the early databases for the SDRP. Andy Read provided important guidance and encouragement during the early development of this research. Larry Fulford secured dolphins for life history research and health assessments during 1984-2019. We are grateful to Deb Fauquier for wrangling the multitudes of veterinarians assisting with health assessment sessions, and to Howard Rhinehart, Brian Balmer, Sunnie Brenneman, Christina Toms, and Amanda Moors for wrangling the resulting samples and sample processors. We appreciate the assistance of Susan Kruse, Megan Stolen, Wayne McFee, Andria Beal, and Ashley Barratclough with age estimation. We are grateful to the superb team of National Marine Mammal Foundation veterinarians for bringing standardized, systematic ultrasound examinations to our efforts. Thanks go to Carolyn Cush, Allison Honaker, Kylee DiMaggio, Krystan Wilkinson, Shauna McBride, and Jonathan Crossman, and many others before them for their help with photo-identification and data processing. Mote Marine Laboratory’s Stranding Investigations Program, led during early years by Geoff Patton, Jay Gorzelany, Nélio Barros, and Greg Early, provided important information from carcasses of resident dolphins. We thank Kylee DiMaggio and Nadine Slimak for help with preparation of the manuscript. We are grateful to Perry Gilbert, the director of Mote Marine Laboratory (1970-72), and the leaders of the Chicago Zoological Society/Brookfield Zoo Chicago (George Rabb, 1989-2003, Stuart Strahl, 2003-2021, and Michael Adkesson, 2021-present), for facilitating the development and continuation of this research. The research was conducted under a series of National Marine Fisheries Service Scientific Research Permits, with annual approvals by Mote Marine Laboratory’s Institutional Animal Care and Use Committee. We greatly appreciate the helpful reviews by Andy Read and Aimee Lang.
Conflict of interest
Author JS is an owner of Dolphin Quest, Inc.
The remaining authors declare that the research was conducted in the absence of any commercial or financial relationships that could be construed as a potential conflict of interest.
The authors declare that this study received funding from Dolphin Quest, Inc. The funder had the following involvement in the study: Dolphin Quest also provided veterinary and dolphin handler staff to help collect samples and data.
Generative AI statement
The author(s) declare that no Generative AI was used in the creation of this manuscript.
Publisher’s note
All claims expressed in this article are solely those of the authors and do not necessarily represent those of their affiliated organizations, or those of the publisher, the editors and the reviewers. Any product that may be evaluated in this article, or claim that may be made by its manufacturer, is not guaranteed or endorsed by the publisher.
References
Adams J. D., Speakman T., Zolman E., Schwacke L. H. (2006). Automating image matching, cataloguing, and analysis for photo-identification research. Aquat. Mammals 32, 374–384. doi: 10.1578/AM.32.3.2006.374
Arso Civil M., Cheney B., Quick N. J., Thompson P. M., Hammond P. S. (2017). A new approach to estimate fecundity rate from inter-birth intervals. Ecosphere 8 (4), e01796. doi: 10.1002/ecs2.1796
Baker I., O’Brien J., McHugh K., Berrow S. (2018). Female reproductive parameters and population demographics of bottlenose dolphins (Tursiops truncatus) in the Shannon Estuary, Ireland. Mar. Biol. 165, 15. doi: 10.1007/s00227-017-3265-z
Balmer B. C., Wells R. S., Nowacek S. M., Nowacek D. P., Schwacke L. H., McLellan W. A., et al. (2008). Seasonal abundance and distribution patterns of common bottlenose dolphins (Tursiops truncatus) near st. joseph bay, florida, USA. J. Cetacean Res. Manag 10, 157–167.
Barlow J., Clapham P. J. (1997). A new birth-interval approach to estimating demographic parameters of humpback whales. Ecology 78, 535–546. doi: 10.2307/2266028
Barlow J., Hohn A. A. (1984). Interpreting spotted dolphin age distributions (La Jolla, CA: NOAA Technical Memorandum NMFS-SWFC-48), 1–22. Available online at: https://swfsc-publications.fisheries.noaa.gov/publications/TM/SWFSC/NOAA-TM-NMFS-SWFC-48.pdf.
Barratclough A., McFee W. E., Stolen M., Hohn A. A., Lovewell G. N., Gomez F. M., et al. (2023). How to estimate age of old bottlenose dolphins (Tursiops truncatus); by tooth or pectoral flipper? Front. Mar. Sci. 10. doi: 10.3389/fmars.2023.1135521
Barratclough A., Sanz-Requena R., Marti-Bonmati L., Schmitt T. L., Jensen E., Garcı́a-Párraga D. (2019b). Radiographic assessment of pectoral flipper bone maturation in bottlenose dolphins (Tursiops truncatus), as a novel technique to accurately estimate chronological age. PloS One 14, e0222722. doi: 10.1371/journal.pone.0222722
Barratclough A., Smith C. R., Gomez F. M., Photopoulou T., Takeshita R., Pirotta E., et al. (2021). Accurate epigenetic aging in bottlenose dolphins (Tursiops truncatus), an essential step in the conservation of at-risk dolphins. J. Zool. Bot. Gard. 2, 416–420. doi: 10.3390/jzbg2030030
Barratclough A., Wells R. S., Schwacke L. H., Rowles T. K., Gomez F. M., Fauquier D. A., et al. (2019a). Health assessments of common bottlenose dolphins (Tursiops truncatus): Past, present, and potential conservation applications. Front. Vet. Sci. 6. doi: 10.3389/fvets.2019.00444
Bassos-Hull K., Perrtree R., Shepard C., Schilling S., Barleycorn A., Allen J., et al. (2013). Long-term site fidelity and seasonal abundance estimates of common bottlenose dolphins (Tursiops truncatus) along the southwest coast of Florida and responses to natural perturbations. J. Cetacean Res. Manage. 13, 19–30. doi: 10.47536/jcrm.v13i1.551
Beal A., Kiszka J. J., Wells R. S., Eirin-Lopez J. M. (2019). The Bottlenose dolphin Epigenetic Aging Tool (BEAT): a molecular age estimation tool for small cetaceans. Front. Mar. Sci. 6. doi: 10.3389/fmars.2019.00561
Berens McCabe E. J., Wells R. S., Toms C., Barleycorn A., Wilkinson K. A., Palubok V. (2021). Effects of multiple Karenia brevis red tide blooms on a common bottlenose dolphin (Tursiops truncatus) prey assemblage: Patterns of resistance and resilience in sarasota bay, florida. Front. Mar. Science Mar. Conserv. Sustainability Special Topic: dolphins Sarasota Bay: lessons 50 years Res. Conserv. 8, 711114. doi: 10.3389/fmars.2021.711114
Berner D., Blanckenhorn W. U. (2007). An ontogenetic perspective on the relationship between age and size at maturity. Funct. Ecol. 21, 505–512. doi: 10.1111/j.1365-2435.2007.01253.x
Betty E. L., Stockin K. A., Hinton B., Bollard B. A., Smith A. N., Orams M. B., et al. (2022). Age, growth, and sexual dimorphism of the Southern Hemisphere long-finned pilot whale (Globicephala melas edwardii). J. Mammal. 103, 560–575. doi: 10.1093/jmammal/gyab165
Bezamat C., Castilho P. V., Simões-Lopes P. C., Ingram S. N., Daura-Jorge F. G. (2020). Reproductive parameters and factors influencing calf survival of bottlenose dolphins that engage in a unique foraging cooperation with fishermen. Mar. Biol. 167, 1–12. doi: 10.1007/s00227-019-3611-4
Blasi M. F., Bruno C., Boitani L. (2020). Female reproductive output in a Mediterranean bottlenose dolphin Tursiops truncatus population. Aquat. Biol. 29, 123–136. doi: 10.3354/ab00732
Blázquez M., Baker I., O’Brien J. M., Berrow S. D. (2020). Population Viability Analysis and comparison of two monitoring strategies for bottlenose dolphins (Tursiops truncatus) in the Shannon Estuary, Ireland, to inform management. Aquat. Mammals 46, 307–325. doi: 10.1578/AM.46.3.2020.307
Boggs A. S. P., Ragland J. M., Zolman E. S., Schock T. B., Morey J. S., Galligan T. M., et al. (2019). Remote blubber sampling paired with liquid chromatography tandem mass spectrometry for steroidal endocrinology in free-ranging bottlenose dolphins (Tursiops truncatus). Gen. Comp. Endocrinol. 281, 164–172. doi: 10.1016/j.ygcen.2019.06.006
Bolaños-Jiménez J., Morteo E., Delfín-Alfonso C. A., Fruet P. F., Secchi E. R., Bello-Pineda J. (2021). Population dynamics reveal a core community of the common bottlenose dolphin (Tursiops truncatus) in open waters of the southwestern Gulf of Mexico. Front. Mar. Sci. 8. doi: 10.3389/fmars.2021.753484
Brent L. J. N., Franks D. W., Foster E. A., Balcomb K. C., Cant M. A., Croft D. P. (2015). Ecological knowledge, leadership, and the evolution of menopause in killer whales. Curr. Biol. 25, 746–750. doi: 10.1016/j.cub.2015.01.037
Brook F. (1997). The use of diagnostic ultrasound in assessment of the reproductive status of the bottlenose dolphin, tursiops aduncas, in capacity & applications in management of a controlled breeding programme (Hung Hom, Kowloon (Hong Kong: The Hong Kong Polytechnic University).
Brook F. M., Kinoshita R., Benirschke K. (2002). Histology of the ovaries of a bottlenose dolphin, Tursiops aduncus, of known reproductive history. Mar. Mammal Sci. 18, 540–544. doi: 10.1111/j.1748-7692.2002.tb01054.x
Caspar K. R., Begall S. (2022). Sexual dimorphism in toothed whales (Odontoceti) follows Rensch’s rule. Mamm. Biol. 102, 523–529. doi: 10.1007/s42991-022-00239-1
Cheal A. J., Gales N. J. (1991). Body mass and food intake in captive, breeding bottlenose dolphins, Tursiops truncatus. Zoo Biol. 10, 451–456. doi: 10.1002/zoo.1430100603
Cheney B. J., Thompson P. M., Cordes L. S. (2019). Increasing trends in fecundity and calf survival of bottlenose dolphins in a marine protected area. Sci. Rep. 9, 1767. doi: 10.1038/s41598-018-38278-9
Collet A., Saint Girons H. (1984).“Preliminary study of the male reproductive cycle in common dolphins, Delphinus delphis, in the eastern North Atlantic” in Reproduction in Whales, Dolphins and Porpoises. Eds. Perrin W. F., Brownell R. L., DeMaster D. P. (Cambridge: Reports of the International Whaling Commission. Special Issue 6), 355–360.
Croft D. P., Johnstone R. A., Ellis S., Nattrass S., Franks D. W., Brent L. J. N., et al. (2017). Reproductive conflict and the evolution of menopause in killer whales. Curr. Biol. 27, 298–304. doi: 10.1016/j.cub.2016.12.015
Dabin W., Cossais F., Pierce G. J., Ridoux V. (2008). Do ovarian scars persist with age in all Cetaceans: new insight from the short-beaked common dolphin (Delphinus delphis Linnaeus 1758). Mar. Biol. 156, 127–139. doi: 10.1007/s00227-008-1070-4
Danil K., Chivers S. J. (2007). Growth and reproduction of female short-beaked common dolphins, Delphinus delphis, in the eastern tropical Pacific. Can. J. Zool. 85, 108–121. doi: 10.1139/z06-188
DeLynn R. E., Lovewell G., Wells R. S., Early G. (2011). Congenital scoliosis of a bottlenose dolphin. J. Wildlife Disease. 47, 979–983. doi: 10.7589/0090-3558-47.4.979
Desportes G., Saboureau M., Lacroix A. (1994). Growth-related changes in testicular mass and plasma testosterone concentrations in long-finned pilot whales, Globicephala melas. J. Reprod. fertility 102, 237–244. doi: 10.1530/jrf.0.1020237
Duffield D. A., Odell D. K., McBain J. F., Andrews B. (1995). Killer whale (Orcinus orca) reproduction at Sea World. Zoo Biol. 14, 417–430. doi: 10.1002/zoo.1430140504
Duffield D. A., Robeck T. (2000). Bottlenose Dolphin Reproduction Workshop Report (Silver Springs, MD: AZA Mar. Mammal Taxon Advisory Group).
Duffield D. A., Wells R. S. (1991). “The combined application of chromosome, protein and molecular data for the investigation of social unit structure and dynamics in Tursiops truncatus,” in Genetic Ecology of Whales and Dolphins. Ed. Hoelzel A. R. (Report of the International Whaling Commission, Cambridge, U.K), 155–169. Special Issue 13.
Duffield D. A., Wells R. S. (2002). “The molecular profile of a resident community of bottlenose dolphins, Tursiops truncatus,” in Molecular and Cell Biology of Marine Mammals. Ed. Pfeiffer C. J. (Krieger Publishing Company, Melbourne, FL), 3–11.
Duffield D. A., Wells R. S. (2023). Paternity patterns in a long-term resident bottlenose dolphin community. Front. Mar. Sci. 10. doi: 10.3389/fmars.2023.1076715
Estrade V., Dulau V. (2020). Abundance and site fidelity of bottlenose dolphins off a remote oceanic island (Reunion Island, southwest Indian Ocean). Mar. Mammal Sci. 36, 871–896. doi: 10.1111/mms.12693
Fazioli K. L., Hofmann S., Wells R. S. (2006). Use of coastal Gulf of Mexico waters by distinct assemblages of bottlenose dolphins Tursiops truncatus. Aquat. Mammals 32, 212–222. doi: 10.1578/AM.32.2.2006.212
Ferguson S. H., Higdon J. W. (2013). Grouping world cetaceans according to life-history characteristics indicates two divergent strategies. Can. Wildlife Biol. Manage. 2, 51–66.
Fisher H. D., Harrison R. J. (1970). Reproduction in the common porpoise (Phocoena phocoena) of the North Atlantic. J. Zool. 161, 471–486. doi: 10.1111/j.1469-7998.1970.tb02050.x
Foster E. A., Franks D. W., Mazzi S., Darden S. K., Balcomb K. C., Ford J. K. B., et al. (2012). Adaptive prolonged post-reproductive life span in killer whales. Science 337, 1313. doi: 10.1126/science.1224198
Fruet P. F., Genoves R. C., Möller L. M., Botta S., Secchi E. R. (2015). Using mark-recapture and stranding data to estimate reproductive traits in female bottlenose dolphins (Tursiops truncatus) of the Southwestern Atlantic Ocean. Mar. Biol. 162, 661–673. doi: 10.1007/s00227-015-2613-0
Fruet P. F., Möller L. M., Secchi E. R. (2021). Dynamics and viability of a small, estuarine-resident population of Lahille’s bottlenose dolphins from southern Brazil. Front. Mar. Sci. 7. doi: 10.3389/fmars.2020.593474
Funasaka N., Yoshioka M., Suzuki M., Ueda K., Miyahara H., Uchida S. (2011). Seasonal difference of diurnal variations in serum melatonin, cortisol, testosterone, and rectal temperature in Indo-Pacific bottlenose dolphins (Tursiops aduncus). Aquat. Mammals 37, 433–442. doi: 10.1578/AM.37.4.2011.433
Gannon D. P., Berens E. J., Camilleri S. A., Gannon J. G., Brueggen M. K., Barleycorn A., et al. (2009). Effects of Karenia brevis harmful algal blooms on nearshore fish communities in southwest Florida. Mar. Ecol. Prog. Ser. 378, 171–186. doi: 10.3390/toxins14070439
Hazelkorn R. A., Wells R. S., Siders Z. A., DeLynn R., Lovewell G. N. (2020). Physical maturity in common bottlenose dolphins (Tursiops truncatus) from Sarasota Bay, FL. Mar. Mammal Sci. 36 (4), 1309–1321. doi: 10.1111/mms.12733
Henderson S. D., Dawson S. M., Currey R. J. C., Lusseau D., Schneider K. (2014). Reproduction, birth seasonality, and calf survival of bottlenose dolphins in Doubtful Sound, New Zealand. Mar. Mammal Sci. 30, 1067–1080. doi: 10.1111/mms.12109
Herrman J., Morey J. S., Takeshita R., De Guise S., Wells R. S., McFee W., et al. (2020). Age determination of common bottlenose dolphins (Tursiops truncatus) using dental radiography pulp:tooth area ratio measurements. PloS One 15, e0242273. doi: 10.1371/journal.pone.0242273
Herzing D. L. (1997). The life history of free-ranging Atlantic spotted dolphins (Stenella frontalis): age classes, color phases, and female reproduction. Mar. Mammal Sci. 13, 576–595. doi: 10.1111/j.1748-7692.1997.tb00085.x
Hohn A. A. (1980). Age determination and age related factors in the teeth of western north atlantic bottlenose dolphins. Sci. Rep. Whales Res. Institute 32, 39–66.
Hohn A. A., Chivers S. J., Barlow J. (1985). Reproductive maturity and seasonality of male spotted dolphins, Stenella attenuata, in the eastern tropical Pacific. Mar. Mammal Sci. 1, 273–293. doi: 10.1111/j.1748-7692.1985.tb00016.x
Hohn A. A., Read A. J., Fernandez S., Vidal O., Findley L. T. (1996). Life history of the vaquita, Phocoena sinus (Phocoenidae, cetacea). J. Zoology 239 (2), 235–251. doi: 10.1111/j.1469-7998.1996.tb05450.x
Hohn A. A., Scott M. D., Wells R. S., Sweeney J. C., Irvine A. B. (1989). Growth layers in teeth from known-age, free-ranging bottlenose dolphins. Mar. Mammal Sci. 5, 315–342. doi: 10.1111/j.1748-7692.1989.tb00346.x
Irvine A. B., Scott M. D., Wells R. S., Kaufmann J. H. (1981). Movements and activities of the Atlantic bottlenose dolphin, Tursiops truncatus, near Sarasota, Florida. Fish. Bull. U.S. 79, 671–688.
Irvine A. B., Scott M. D., Wells R. S., Mead J. G. (1979). Stranding of the pilot whale, Globicephala macrorhynchus, in Florida and South Carolina. Fish. Bull. U.S. 77, 511–513.
Irvine B., Wells R. S. (1972). Results of attempts to tag Atlantic bottlenosed dolphins (Tursiops truncatus). Cetology 13, 1–5.
Johnstone R. A., Cant M. A. (2010). The evolution of menopause in cetaceans and humans: the role of demography. Proc. R. Soc B. 277, 3765–3771. doi: 10.1098/rspb.2010.0988
Kasuya T., Marsh H. (1984). “Life history and reproductive biology of the short-finned pilot whale, Globicephala macrorhynchus, off the Pacific coast of Japan” in Reproduction in Whales, Dolphins and Porpoises. Eds. Perrin W. F., Brownell R. L., DeMaster D. P. (Cambridge: Reports of the International Whaling Commission, Special Issue 6), 259–310.
Katsumata E., Jaroenporn S., Ueda Y., Arai K., Katsumata H., Watanabe G., et al. (2017). Circulating gonadotropins and testicular hormones during sexual maturation and annual changes in male bottlenose dolphins (Tursiops truncatus). J. Vet. Med. Sci. 79, 1899–1905. doi: 10.1292/jvms.16-0544
Kellar N. M., Trego M. L., Marks C. I., Chivers S. J., Danil K., Archer F. I. (2009). Blubber testosterone: A potential marker of male reproductive status in short-beaked common dolphins. Mar. Mammal Sci. 25, 507–522. doi: 10.1111/j.1748-7692.2009.00291.x
Kellar N. M., Trego M. L., Marks C. I., Dizon A. E. (2006). Determining pregnancy from blubber in three species of delphinids. Mar. Mammal Sci. 22, 1–16. doi: 10.1111/j.1748-7692.2006.00001.x
Kemper C., Talamonti M., Bossley M., Steiner A. (2019). Sexual maturity and estimated fecundity in female Indo-Pacific bottlenose dolphins (Tursiops aduncus) from South Australia: Combining field observations and postmortem results. Mar. Mammal Sci. 35, 40–57. doi: 10.1111/mms.12509
Kiszka J. J., Simon-Bouhet B., Charlier F., Pusineri C., Ridoux V. (2010). Individual and group behavioural reactions of small delphinids to remote biopsy sampling. Anim. Welfare 19, 411–417. doi: 10.1017/S0962728600001895
Kita S., Yoshioka M., Kashiwagi M. (1999). Relationship between sexual maturity and testis testosterone concentration in the two forms of short-finned pilot whales, Globicephala macrorhynchus. Fish. Sci. 65, 878–883. doi: 10.2331/fishsci.65.878
Koga S., Yanagisawa M., Koga H., Ueda K., Kawazu I., Tokutake K., et al. (2019). Reproductive ability of elderly male Indo-Pacific bottlenose dolphins (Tursiops aduncus) in captivity. Mammal Study 44, 135–139. doi: 10.3106/ms2018-0044
Kogi K., Hishii T., Imamura A., Iwatani T., Dudzinski K. M. (2004). Demographic parameters of Indo-Pacific bottlenose dolphins (Tursiops aduncus) around Mikura Island, Japan. Mar. Mammal Sci. 20, 510–526. doi: 10.1111/j.1748-7692.2004.tb01176.x
Lacy R. C., Wells R. S., Scott M. D., Allen J. B., Barleycorn A. A., Urian K. W., et al. (2021). Assessing the viability of the Sarasota Bay community of bottlenose dolphins. Front. Mar. Sci. 8. doi: 10.3389/fmars.2021.788086
Lane S. M., Smith C. R., Balmer B. C., Barry K. P., McDonald T., Mitchell J., et al. (2015). Survival and reproductive outcome of bottlenose dolphins sampled in Barataria Bay, Louisiana, USA following the Deepwater Horizon oil spill. Proc. R. Soc. B. 282, 20151944. doi: 10.1098/rspb.2015.1944
Leatherwood S., Reeves R. R. (1982). “Bottlenose dolphin (Tursiops truncatus) and other toothed cetaceans,” in Wild Mammals of North America: Biology, Management, Economics. Eds. Chapman J. A., Feldhamer G. A. (Baltimore: Johns Hopkins University Press), 369–414.
Loughlin T., Cunningham L., Gales N., Wells R. S., Boyd I. (2010). “Marking and capturing,” in Marine Mammal Ecology and Conservation: A Handbook of Techniques. Eds. Boyd I., Bowen D., Iverson S. (Oxford, U.K.: Oxford University Press), 16–41.
Ludwig K. E., Daly M., Levesque S., Berrow S. D. (2021). Survival rates and capture heterogeneity of bottlenose dolphins (Tursiops truncatus) in the Shannon Estuary, Ireland. Front. Mar. Sci. 8. doi: 10.3389/fmars.2021.611219
Mann J., Connor R. C., Barre L. M., Heithaus M. R. (2000). Female reproductive success in bottlenose dolphins (Tursiops sp.): life history, habitat, provisioning, and group size effects. Behav. Ecol. 11, 210–219. doi: 10.1093/beheco/11.2.210
Martin A. R., Da Silva V. M. F. (2018). Reproductive parameters of the Amazon river dolphin or boto, Inia geoffrensis (Cetacea: Iniidae); an evolutionary outlier bucks no trends. Biol. J. Linn. Soc. 123, 666–676. doi: 10.1093/biolinnean/bly005
McGuire T. L., Stephens A. D., McClung J. R., Garner C. D., Shelden K. E. W., Boor G. K. H., et al. (2020). Reproductive natural history of endangered Cook Inlet Beluga whales: insights from a long-term photo-identification study. Polar Biol. 43, 1851–1871. doi: 10.1007/s00300-020-02750-y
McHugh K. A., Allen J. B., Barleycorn A., Wells R. S. (2011). Natal philopatry, ranging behavior, and habitat selection of juvenile bottlenose dolphins in Sarasota Bay, FL. J. Mammal. 92, 1298–1313. doi: 10.1644/11-MAMM-A-026.1
Mead J. G., Odell D. K., Wells R. S., Scott M. D. (1980). Observations on a mass stranding of spinner dolphins, Stenella longirostris, from the west coast of Florida. Fish. Bull. U.S. 78, 353–360.
Millar J. S., Zammuto R. M. (1983). Life histories of mammals: an analysis of life tables. Ecology 64, 631–635. doi: 10.2307/1937181
Mingramm F., Dunlop R., Blyde D., Whitworth D., Keeley T. (2019). Evaluation of respiratory vapour and blubber samples for use in endocrine assessments of bottlenose dolphins (Tursiops spp.). Gen. Comp. Endocrinol. 274, 37–49. doi: 10.1016/j.ygcen.2018.12.015
Mitteldorf J., Goodnight C. (2012). Post-reproductive life span and demographic stability. Oikos 121, 1370–1378. doi: 10.1134/S0006297913090071
Möller L. M. (2012). Sociogenetic structure, kin associations and bonding in delphinids. Mol. Ecol. 21, 745–764. doi: 10.1111/j.1365-294X.2011.05405.x
Möller L. M., Wiszniewski J., Allen S. J., Beheregaray L. B. (2007). Habitat type promotes rapid and extremely localised genetic differentiation in dolphins. Mar. Freshw. Res. 58, 640–648. doi: 10.1071/MF06218
Moore J. E., Read A. J. (2008). A Bayesian uncertainty analysis of cetacean demography and bycatch mortality using age-at-death data. Ecol. Appl. 18, 1914–1931. doi: 10.1890/07-0862.1
Murphy S., Winship A., Dabin W., Jepson P. D., Deaville R., Reid R. J., et al. (2009). Importance of biological parameters in assessing the status of Delphinus delphis. Mar. Ecol. Prog. Ser. 388, 273–291. doi: 10.3354/meps08129
Neimanis A. S., Read A. J., Foster R. A., Gaskin D. E. (2000). Seasonal regression in testicular size and histology in harbour porpoises (Phocoena phocoena, L.) from the Bay of Fundy and Gulf of Maine. J. Zool. 250, 221–229. doi: 10.1111/j.1469-7998.2000.tb01072.x
Ngqulana S. G., Hofmeyr G. G., Plön S. (2017). Sexual dimorphism in long-beaked common dolphins (Delphinus capensis) from KwaZulu-Natal, South Africa. J. Mammal. 98, 1389–1399. doi: 10.1093/jmammal/gyx086
O’Brien J. K., Robeck T. R. (2012). The relationship of maternal characteristics and circulating progesterone concentrations with reproductive outcome in the bottlenose dolphin (Tursiops truncatus) after artificial insemination, with and without ovulation induction, and natural breeding. Theriogenology 78, 469–482. doi: 10.1016/j.theriogenology.2012.02.011
Olesiuk P. F., Bigg M. A., Ellis G. M. (1993). Life history and population dynamics of resident killer whales (Orcinus orca) in the coastal waters of british columbia and washington state (Cambridge: Reports of the International Whaling Commission Special Issue 12), 209–243. Available at: https://archive.iwc.int/pages/view.php?search=%21collection34&k=&modal=&display=list&order_by=title&offset=0&per_page=240&archive=&sort=DESC&restypes=&recentdaylimit=&foredit=&noreload=true&access=&ref=472.
Owen E. C. G., Hofmann S., Wells R. S. (2002). Ranging and social association patterns of paired and unpaired adult male bottlenose dolphins, Tursiops truncatus, in sarasota, florida, provide no evidence for alternative male strategies. Can. J. Zoology. 80, 2072–2089.
Peltier H., Dabin W., Daniel P., Van Canneyt O., Dorémus G., Huon M., et al. (2012). The significance of stranding data as indicators of cetacean populations at sea: modelling the drift of cetacean carcasses. Ecol. Indic. 18, 278–290. doi: 10.1016/j.ecolind.2011.11.014
Perrin W. F., Henderson J. R. (1984). “Growth and reproductive rates in two populations of spinner dolphins, stenella longirostris, with different histories of exploitation,” in Reproduction in whales, dolphins and porpoises. Eds. Perrin W. F., Brownell R. L. Jr., DeMaster D. P. (Cambridge: Reports of the International Whaling Commission Special Issue 6), 417–430.
Perrin W. F., Donovan G. (1984). “Report of the workshop” in Reproduction in Whales, Dolphins and Porpoises. Eds. Perrin W. F., Brownell R. L. Jr., DeMaster D. P. (Cambridge: Reports of the International Whaling Commission Special Issue 6), 1–24.
Perrin W. F., Holts D. B., Miller R. B. (1977). Growth and reproduction of the eastern spinner dolphin, a geographical form of Stenella longirostris in the eastern tropical Pacific. Fish. Bull. U.S. 75, 725–750. doi: 10.1139/Z09-038
Perrin W. F., Reilly S. B. (1984). “Reproductive parameters of dolphins and small whales of the family Delphinidae” in Reproduction in Whales, Dolphins and Porpoises. Eds. Perrin W. F., Brownell R. L. Jr., DeMaster D. P. (Cambridge: Reports of the International Whaling Commission Special Issue 6), 97–133.
Peters R. H. (1986). The ecological implications of body size (Cambridge: Cambridge University Press).
Pleslić G., Rako-Gospić N., Holcer D. (2021). Bottlenose dolphins (Tursiops truncatus) in North Dalmatia, Croatia: Occurrence and demographic parameters. Mar. Mam. Sci. 37, 142–161. doi: 10.1111/mms.12735
Pleslić G., Rako-Gospić N., Miočić-Stošić J., Blazinić Vučur T., Radulović M., Mackelworth P., et al. (2019). Social structure and spatial distribution of bottlenose dolphins (Tursiops truncatus) along the Croatian Adriatic coast. Aquat. Conserv.: Mar. Freshw. Ecosyst. 29, 2116–2132. doi: 10.1002/aqc.3213
Plön S., Bernard R. (2007). “Testis, spermatogenesis, and testicular cycles,” in Reproductive biology and phylogeny of Cetacea: whales, porpoises and dolphins. Ed. Miller D. L. (CRC Press, New Hampshire, USA), 215–244.
Ralls K., Mesnick S. (2009). “Sexual dimorphism,” in Encyclopedia of marine mammals (San Diego: Academic Press), 1005–1011.
Read A. J. (1990). Age at sexual maturity and pregnancy rates of harbour porpoises Phocoena phocoena from the Bay of Fundy. Can. J. Fish. Aquat. Sci. 47, 561–565. doi: 10.1139/f90-064
Read A. J., Hohn A. A. (1995). Life in the fast lane: The life history of harbor porpoises from the Gulf of Maine. Mar. Mammal Sci. 11, 423–440. doi: 10.1111/j.1748-7692.1995.tb00667.x
Read A. J., Wells R. S., Hohn A. A., Scott M. D. (1993). Patterns of growth in wild bottlenose dolphins, Tursiops truncatus. J. Zoology 231, 107–123. doi: 10.1111/j.1469-7998.1993.tb05356.x
Reddy M. L., Reif J. S., Bachand A., Ridgway S. H. (2001). Opportunities for using Navy marine mammals to explore associations between organochlorine contaminants and unfavorable effects on reproduction. Sci. Total Environ. 274, 171–182. doi: 10.1016/S0048-9697(01)00741-0
Reilly S., Barlow J. (1986). Rates of increase in dolphin population size. Fishery Bull. 84, 527–533.
Robinson K. P., Sim T. M., Culloch R. M., Bean T. S., Cordoba Aguilar I., Eisfeld S. M., et al. (2017). Female reproductive success and calf survival in a North Sea coastal bottlenose dolphin (Tursiops truncatus) population. PloS One 12, e0185000. doi: 10.1371/journal.pone.0185000
Rosel P. E., Mullin K. D., Garrison L., Schwacke L., Adams J., Balmer B., et al. (2011). Photo-identification Capture-Mark-Recapture Techniques for Estimating Abundance of Bay, Sound and Estuary Populations of Bottlenose Dolphins along the U.S. East Coast and Gulf of Mexico: A Workshop Report (Miami, FL: NOAA Technical Memorandum NMFS-SEFSC-621), 30pp. Available online at: https://repository.library.noaa.gov/view/noaa/4014.
Ross C., Rychtář J., Rueppell O. (2015). A structured population model suggests that long life and post-reproductive lifespan promote the evolution of cooperation. J. Theor. Biol. 369, 85–94. doi: 10.1016/j.jtbi.2015.01.020
Schroeder J. P., Keller K. V. (1989). Seasonality of serum testosterone levels and sperm density in Tursiops truncatus. J. Exp. Zool. 249, 316–321. doi: 10.1002/jez.1402490310
Schwacke L., Marques T., Thomas L., Booth C., Balmer B., Barratclough A., et al. (2022). Modeling population effects of the Deepwater Horizon oil spill on a long-lived species. Conserv. Biol. 36, e13878. doi: 10.1111/cobi.13878
Schwacke L. H., Thomas L., Wells R. S., McFee W. E., Hohn A. A., Mullin K. D., et al. (2017). Quantifying injury to common bottlenose dolphins from the Deepwater Horizon oil spill using an age-, sex- and class-structured population model. Endangered Species Res. 33, 265–279. doi: 10.3354/esr00777
Schwacke L. H., Voit E. O., Hansen L. J., Wells R. S., Mitchum G. B., Hohn A. A., et al. (2002). Probabilistic risk assessment of reproductive effects of polychorinated biphenyls on bottlenose dolphins (Tursiops truncatus) from the southeast United States coast. Environ. Toxicol. Chem. 21, 2752–2764. doi: 10.1002/etc.5620211232
Scott M. D., Wells R. S., Irvine A. B. (1990). “A long-term study of bottlenose dolphins on the west coast of Florida,” in The Bottlenose Dolphin. Eds. Leatherwood S., Reeves R. R. (Academic Press, San Diego, CA), 235–244.
Sellas A. B., Wells R. S., Rosel P. E. (2005). Mitochondrial and nuclear DNA analyses reveal fine scale geographic structure in bottlenose dolphins (Tursiops truncatus) in the Gulf of Mexico. Conserv. Genet. 6, 715–728. doi: 10.1007/s10592-005-9031-7
Sergeant D., Caldwell D. K., Caldwell M. C. (1973). Age, growth, and maturity of bottlenosed dolphin (Tursiops truncatus) from northeast Florida. J. Fish. Board Canada 30, 1009–1011. doi: 10.1139/f73-165
Sherman K. K., Beaulieu-McCoy N. E., Wurster E. L., Wells R. S., Smith C. R., Barleycorn A. A., et al. (2021). Serum correlation, demographic differentiation, and seasonality in common bottlenose dolphins, Tursiops truncatus, in Sarasota Bay, FL. Sci. Rep. 11, 8941. doi: 10.1038/s41598-021-88602-z
Sibly R. M., Brown L. H. (2007). Effects of body size and lifestyle on evolution of mammal life histories. Proc. Natl. Acad. Sci. United States America 104, 17707. doi: 10.1073/pnas.0707725104
Sinclair C., Sinclair J., Zolman E. S., Martinez A., Balmer B., Barry K. P. (2015). Remote biopsy field sampling procedures for cetaceans used during the Natural Resource Damage Assessment of the MSC252 Deepwater Horizon Oil Spill. (Miami, FL: NOAA Technical Memorandum NMFS-SEFSC-670), 28pp. Available online at: https://repository.library.noaa.gov/view/noaa/4846.
Slooten E. (1991). Age, growth, and reproduction in Hector’s dolphins. Can. J. Zool. 69, 1689–1700. doi: 10.1139/z91-234
Speakman T. R., Lane S. M., Schwacke L. H., Fair P. A., Zolman E. S. (2010). Mark-recapture estimates of seasonal abundance and survivorship for bottlenose dolphins (Tursiops truncatus) near charleston, south carolina, USA. J. Cetacean Res. Manage 11 (2), 153–162.
Stamps J. A., Mangel M., Phillips J. A. (1998). A new look at relationships between size at maturity and asymptotic size. Am. Nat. 152, 470–479. doi: 10.1086/286183
Stearns S. C. (1983). The influence of size and phylogeny on patterns of covariation among life-history traits in the mammals. Oikos 41, 173–187. doi: 10.2307/3544261
Steiner A., Bossley M. (2008). Some reproductive parameters of an estuarine population of Indo-pacific bottlenose dolphins (Tursiops aduncus). Aquat. Mammals 34, 84–88,90-92. doi: 10.1578/AM.34.1.2008.84
Steinman K. J., Robeck T. R., O’Brien J. K. (2016). Characterization of estrogens, testosterone, and cortisol in normal bottlenose dolphin (Tursiops truncatus) pregnancy. Gen. Comp. Endocrinol. 226, 102–112. doi: 10.1016/j.ygcen.2015.12.019
Stolen M. K., Barlow J. (2003). A model life table for bottlenose dolphins (Tursiops truncatus) from the Indian River Lagoon system of East Florida. Mar. Mammal Sci. 19, 630–649. doi: 10.1111/j.1748-7692.2003.tb01121.x
Tezanos-Pinto G., Constantine R., Mourao F., Berghan J., Baker C. S. (2015). High calf mortality in bottlenose dolphins in the Bay of Islands, New Zealand. Mar. Mammal Sci. 31, 540–559. doi: 10.1111/mms.12174
Tolley K., Read A., Wells R., Urian K., Scott M., Irvine A., et al. (1995). Sexual dimorphism in wild bottlenose dolphins (Tursiops truncatus) from Sarasota, Florida. J. Mammal. 76, 1190–1198. doi: 10.2307/1382611
Urian K. W., Duffield D. A., Read A. J., Wells R. S., Shell D. D. (1996). Seasonality of reproduction in bottlenose dolphins, Tursiops truncatus. J. Mammal. 77, 394–403. doi: 10.2307/1382814
Urian K., Gorgone A., Read A., Balmer B., Wells R. S., Berggren P., et al. (2015). Recommendations for photo-identification methods used in capture-recapture models with cetaceans. Mar. Mammal Sci. 31 (1), 298–321. doi: 10.1111/mms.12141
Urian K. W., Hofmann S., Wells R. S., Read A. J. (2009). Fine-scale population structure of bottlenose dolphins, Tursiops truncatus, in Tampa Bay, Florida. Mar. Mammal Sci. 25, 619–638. doi: 10.1111/j.1748-7692.2009.00284.x
Van Waerebeek K., Read A. J. (1994). Reproduction of dusky dolphins, Lagenorhynchus obscurus, from coastal Peru. J. Mammal. 75, 1054–1062. doi: 10.2307/1382489
Venuto R., Botta S., Barreto A. S., Secchi E. R., Fruet P. F. (2020). Age structure of strandings and growth of Lahille’s bottlenose dolphin (Tursiops truncatus gephyreus). Mar. Mammal Sci. 36, 813–827. doi: 10.1111/mms.12683
Ward E. J., Parsons K., Holmes E. E., Balcomb K. C., Ford J. K. (2009). The role of menopause and reproductive senescence in a long-lived social mammal. Front. Zool. 6, 1–10. doi: 10.1186/1742-9994-6-4
Wells R. S. (1984). “Reproductive behavior and hormonal correlates in Hawaiian spinner dolphins, Stenella longirostris,” in Reproduction in Whales, Dolphins, and Porpoises. Eds. Perrin W. F., Brownell R. L. Jr., DeMaster D. P. (Reports of the International Whaling Commission, Special Issue 6, Cambridge), 465–472.
Wells R. S. (2000). “Reproduction in wild bottlenose dolphins: Overview of patterns observed during a long-term study,” in Bottlenose Dolphin Reproduction Workshop Report. Eds. Duffield D., Robeck T. (AZA Mar. Mammal Taxon Advisory Group, Silver Springs, MD), 57–74.
Wells R. S. (2009). Learning from nature: Bottlenose dolphin care and husbandry. Zoo Biol. 28, 1–17. doi: 10.1002/zoo.20252
Wells R. S. (2014). “Social structure and life history of common bottlenose dolphins near Sarasota Bay, Florida: Insights from four decades and five generations,” in Primates and Cetaceans: Field Research and Conservation of Complex Mammalian Societies, Primatology Monographs. Eds. Yamagiwa J., Karczmarski L. (Springer, Tokyo, Japan), 149–172. doi: 10.1007/978-4-431-54523-1_8
Wells R. S. (2018). “Identification methods,” in Encyclopedia of Marine Mammals, 3rd Ed. Eds. Würsig B., Thewissen J. G. M., Kovacs K. (Academic Press/Elsevier, San Diego, CA), 503–509.
Wells R. S. (2020). The Sarasota Dolphin Research Program in 2020: Celebrating 50 years of research, conservation, and education. Aquat. Mammals 25, 502–503. doi: 10.1578/AM.46.5.2020.502
Wells R. S., Allen J. B., Hofmann S., Bassos-Hull K., Fauquier D. A., Barros N. B., et al. (2008). Consequences of injuries on survival and reproduction of common bottlenose dolphins (Tursiops truncatus) along the west coast of Florida. Mar. Mammal Sci. 24, 774–794. doi: 10.1111/j.1748-7692.2008.00212.x
Wells R. S., Allen J. B., Lovewell G. N., Gorzelany J., DeLynn R. E., Fauquier D. A., et al. (2015). Carcass-recovery rates for resident bottlenose dolphins in Sarasota Bay, Florida. Mar. Mammal Sci. 31, 355–368. doi: 10.1111/mms.12142
Wells R. S., Bassos M. K., Urian K. W., Carr W. J., Scott M. D. (1996a). Low-level monitoring of bottlenose dolphins, Tursiops truncatus, in Charlotte Harbor, Florida: 1990-1994. (Miami, FL: NOAA Tech. Mem. NMFS-SEFSC-384), 36 pp. Available online at: https://repository.library.noaa.gov/view/noaa/8530/noaa_8530_DS1.pdf.
Wells R. S., Bassos M. K., Urian K. W., Shane S. H., Owen E. C. G., Weiss C. F., et al. (1997). Low-level monitoring of bottlenose dolphins, Tursiops truncatus, in Pine Island Sound, Florida: 1996. Final Contract Report to National Marine Fisheries Service, Southeast Fisheries Science Center, Miami, FL (Contr. No. 40-WCNF601958), 86 pp.
Wells R. S., Boness D. J., Rathbun G. B. (1999). “Behavior,” in Biology of Marine Mammals. Eds. Reynolds J. E. III, Rommel S. A. (Smithsonian Institution Press, Washington, DC), 324–422.
Wells R. S., Irvine A. B., Scott M. D. (1980). “The social ecology of inshore odontocetes,” in Cetacean Behavior: Mechanisms and Functions. Ed. Herman L. M. (J. Wiley & Sons, New York), 263–317.
Wells R. S., McHugh K. A., Douglas D. C., Shippee S., Berens McCabe E., Barros N. B., et al. (2013). Evaluation of potential protective factors against metabolic syndrome in bottlenose dolphins: Feeding and activity patterns of dolphins in Sarasota Bay, Florida. Front. Endocrinol. 4. doi: 10.3389/fendo.2013.00139
Wells R. S., Natoli A., Braulik G. (2019). Tursiops truncatus (errata version published in 2019). The IUCN Red List of Threatened Species 2019: e.T22563A156932432. doi: 10.2305/IUCN.UK.2019-1.RLTS.T22563A156932432.en
Wells R. S., Rhinehart H. L., Hansen L. J., Sweeney J. C., Townsend F. I., Stone R., et al. (2004). Bottlenose dolphins as marine ecosystem sentinels: Developing a health monitoring system. EcoHealth 1, 246–254. doi: 10.1007/s10393-004-0094-6
Wells R. S., Scott M. D. (1990). “Estimating bottlenose dolphin population parameters from individual identification and capture-release techniques,” in Individual Recognition of Cetaceans: Use of Photo-Identification and Other Techniques to Estimate Population Parameters. Eds. Hammond P. S., Mizroch S. A., Donovan G. P. (Reports of the International Whaling Commission, Special Issue 12, Cambridge, U.K), 407–415.
Wells R. S., Scott M. D., Irvine A. B. (1987). “The social structure of free-ranging bottlenose dolphins,” in Current Mammalogy, Vol. 1. Ed. Genoways H. (Plenum Press, New York), 247–305.
Wells R. S., Smith C. R., Sweeney J. C., Townsend F. I., Fauquier D. A., Stone R., et al. (2014). Fetal survival of common bottlenose dolphins (Tursiops truncatus) in Sarasota Bay, Florida. Aquat. Mammals 40, 252–259. doi: 10.1578/AM.40.3.2014.252
Wells R. S., Tornero V., Borrell A., Aguilar A., Rowles T. K., Rhinehart H. L., et al. (2005). Integrating life history and reproductive success data to examine potential relationships with organochlorine compounds for bottlenose dolphins (Tursiops truncatus) in Sarasota Bay, Florida. Sci. Total Environ. 349, 106–119. doi: 10.1016/j.scitotenv.2005.01.010
Wells R. S., Urian K. W., Read A. J., Bassos M. K., Carr W. J., Scott M. D. (1996b). Low-level monitoring of bottlenose dolphins, Tursiops truncatus, in Tampa Bay, Florida: 1988-1993. (Miami, FL: NOAA Tech. Mem. NMFS-SEFSC-385), 25 pp. Available online at: https://library.oarcloud.noaa.gov/noaa_documents.lib/NMFS/SEFSC/TM_NMFS_SEFSC/NMFS_SEFSC_TM_385.pdf.
Wenzel F., Nicolas J., Larsen F., Pace R. M. III (2010). Northeast Fisheries Science Center Cetacean Biopsy Training Manual (US Dept Commerce, Northeast Fish Science Center Ref Doc.10-11), 18 p. Available online at: http://www.nefsc.noaa.gov/nefsc/publications/.
Yuen W.-h. Q. (2007). An assessment of reproductive development of the male indo-Pacific bottlenose dolphin, Tursiops aduncus, in captivity. [dissertation]. Hung Hom, Kowloon (Hong Kong): The Hong Kong Polytechnic University.
Yuen Q. W., Ying M. T., Brook F. M., Kinoshita R. E. (2009). Reliability of 2D ultrasound measurements of testis size in dolphins taken under voluntary behavior. Ultrasound Med. Biol. 35 (6), 1005–1009. doi: 10.1016/j.ultrasmedbio.2008.12.005
Zeng Q., Wang X., Zhu Q. (2021). Preliminary study on the reproductive ecology of a threatened Indo-Pacific humpback dolphin (Sousa chinensis) population in Xiamen Bay, China. Aquat. Mammals 47, 43–52. doi: 10.1578/AM.47.1.2021.43
Keywords: bottlenose dolphin, Tursiops truncatus, life history, demographics, reproduction, sexual maturation, reproductive senescence, calving interval
Citation: Wells RS, Hohn AA, Scott MD, Sweeney JC, Townsend Jr. FI, Allen JB, Barleycorn AA, McHugh KA, Bassos-Hull K, Lovewell GN, Duffield DA, Smith CR and Irvine AB (2025) Life history, reproductive, and demographic parameters for bottlenose dolphins (Tursiops truncatus) in Sarasota Bay, Florida. Front. Mar. Sci. 12:1531528. doi: 10.3389/fmars.2025.1531528
Received: 20 November 2024; Accepted: 17 January 2025;
Published: 10 February 2025.
Edited by:
Jeffrey C. Mangel, Prodelphinus, PeruReviewed by:
Aimee R. Lang, National Oceanic and Atmospheric Administration, United StatesAndrew Read, Duke University, United States
Copyright © 2025 Wells, Hohn, Scott, Sweeney, Townsend, Allen, Barleycorn, McHugh, Bassos-Hull, Lovewell, Duffield, Smith and Irvine. This is an open-access article distributed under the terms of the Creative Commons Attribution License (CC BY). The use, distribution or reproduction in other forums is permitted, provided the original author(s) and the copyright owner(s) are credited and that the original publication in this journal is cited, in accordance with accepted academic practice. No use, distribution or reproduction is permitted which does not comply with these terms.
*Correspondence: Randall S. Wells, cndlbGxzQG1vdGUub3Jn