- 1Dept. de Biologia Evolutiva, Ecologia i Ciències Ambientals, Universitat de Barcelona, Barcelona, Spain
- 2Institut de Recerca de la Biodiversitat (IRBio), Universitat de Barcelona, Barcelona, Spain
- 3Dept. Biología Animal, Edafología y Geología, Universidad de La Laguna, Santa Cruz de Tenerife, Spain
Ocean acidification (OA) stands out as one of the main threats to marine ecosystems. OA leads to a reduction in the availability of carbonate ions, which are essential for marine calcifiers such as echinoderms. We aim to understand the physiological responses of two sea urchin species, Paracentrotus lividus and Arbacia lixula to low pH conditions and determine whether their responses result from phenotypic plasticity or local adaptation. The study is divided into two parts: plasticity response over time, measuring respiration rates of individuals from the Mediterranean Sea exposed to low pH over seven days, and adaptation and plasticity under changing pH, analyzing individuals inhabiting a pH gradient in a natural CO2 vent system located in La Palma Island, Spain. Over the seven days of low pH exposure, distinct patterns in respiration rates were revealed, with both species demonstrating potential for acclimatization. Notably, P. lividus and A. lixula displayed unsynchronized acidosis/alkalosis cycles, suggesting different physiological mechanisms. Additionally, environmental history seemed to influence adaptive capacity, as specimens from fluctuating pH environments exhibited respiration rates similar to those from stable environments with heightened phenotypic plasticity. Overall, our results suggest that both species possess the capacity for metabolic plasticity, which may enhance their resilience to future OA scenarios but likely involve energetic costs. Moreover, CO2 vent systems may serve as OA refugia, facilitating long-term survival. Understanding the plastic responses versus adaptations is crucial for predicting the effects of OA on species distribution and abundance of marine organisms in response to ongoing climate change.
Introduction
The anthropogenic rise in atmospheric CO2 leads to its absorption by the oceans resulting in the decreasing of seawater pH, known as ocean acidification (OA). Under current emissions, a drop in oceanic pH by 0.4 units is projected by the end of the 21st century compared to the current values (Allan et al., 2021). OA severely threatens marine ecosystems (Dupont and Pörtner, 2013), due to the reduction in the availability of carbonate ions, which affects marine calcifiers who use these minerals to synthesize carbonate biostructures. This vulnerability encompasses a broad range of taxa, including echinoderms (Byrne and Hernández, 2020). The consequences of vulnerable systems are disruptions to food webs, alteration of biodiversity on marine invertebrates, and impacts on the overall health of the oceans (Stumpp et al., 2013).
The sea urchins Paracentrotus lividus (Lamarck, 1816) and Arbacia lixula (Linnaeus, 1758) are calcifying species and key components in benthic communities of the Mediterranean Sea and northeast Atlantic due to their role in algae population regulation (Palacı́n et al., 1998). Within the Mediterranean, the combined grazing action at high densities promotes the formation of barren ground areas (Bonaviri et al., 2011; Agnetta et al., 2015), making changes in their abundance a concern for coastal conservation (Vergés et al., 2014). Studying their adaptability to overcome OA is essential to predict potential impacts on shallow Atlantic-Mediterranean waters.
Seawater pH affects the metabolism of the organisms by altering biochemical pathways (Dupont et al., 2010). External rising CO2 concentration can diffuse into body tissues causing hypercapnia and acidosis (a decreased internal pH) (Collard et al., 2013) which disrupt physiological processes such as calcification, nutrition, and metabolism (Melzner et al., 2009; Byrne and Fitzer, 2019; Pörtner, 2008). Understanding metabolic responses is necessary for assessing the species survival, health, and fitness under environmental challenges (Brown et al., 2004; Calosi et al., 2013). Stabilized metabolic rates can occur via adaptation (selecting genotypes), or acclimatization (phenotypic plasticity) (Norin and Metcalfe, 2019). However, physiological plasticity and adaptation to low pH in marine organisms remain poorly understood (Calosi et al., 2013; Di Giglio et al., 2020). Both P. lividus and A. lixula have a likely efficient pH compensation system, essential for survival in acidified areas. However, P. lividus is less effective in regulating extracellular acid-base balance than A. lixula (Calosi et al., 2013). Nevertheless, this buffering ability may involve an energy reallocation (Catarino et al., 2012), making it essential to study these hidden physiological responses for predicting their long-term viability and ecosystem dynamics.
Much of our knowledge of OA effects mainly comes from short to medium-term laboratory experiments (e.g. Stumpp et al., 2012; Spicer et al., 2011; Wood et al., 2008), but long-term exposure often reveals more complex responses. Long-term studies on tropical sea urchins, particularly those in the Echinometra genus, showed varied OA responses, depending on life stage and environmental context (Uthicke et al., 2020, 2021; Karelitz et al., 2019). Moreover, studies on natural CO2 emission points, also known as CO2 vents, such as those at Ischia, Papua New Guinea and Canary Island, show that some populations of sea urchins exhibit adaptations to high CO2 environments, with adults demonstrating resilience (Foo et al., 2022; Di Giglio et al., 2020; Uthicke et al., 2016, 2019; González-Delgado et al., 2024). In this sense, marine CO2 vents, which create natural pH gradients, offer valuable scenarios to study the long-term effects of OA on marine ecosystems (González-Delgado and Hernández, 2018; González-Delgado et al., 2023), allowing scientists to investigate the biological responses of organisms, as well as their adaptive potential and resilience (Hofmann et al., 2014).
The Canary Islands, situated in the northeastern Atlantic Ocean, are home to the only subtropical marine CO2 vent under investigation (Hernández et al., 2024). La Palma Island presents a continuous degassing of CO2 in the south, in Fuencaliente (Figure 1) (Carracedo et al., 2001; Padrón et al., 2015). The features of the natural CO2 vent include a pH gradient that decreases from 8.1 at open sea (150m from the vent center) to less than 7.4 at the source during low tide (González-Delgado et al., 2021), allowing us to test the effect of decreasing pH on marine organisms in a situation analogous to future oceans.
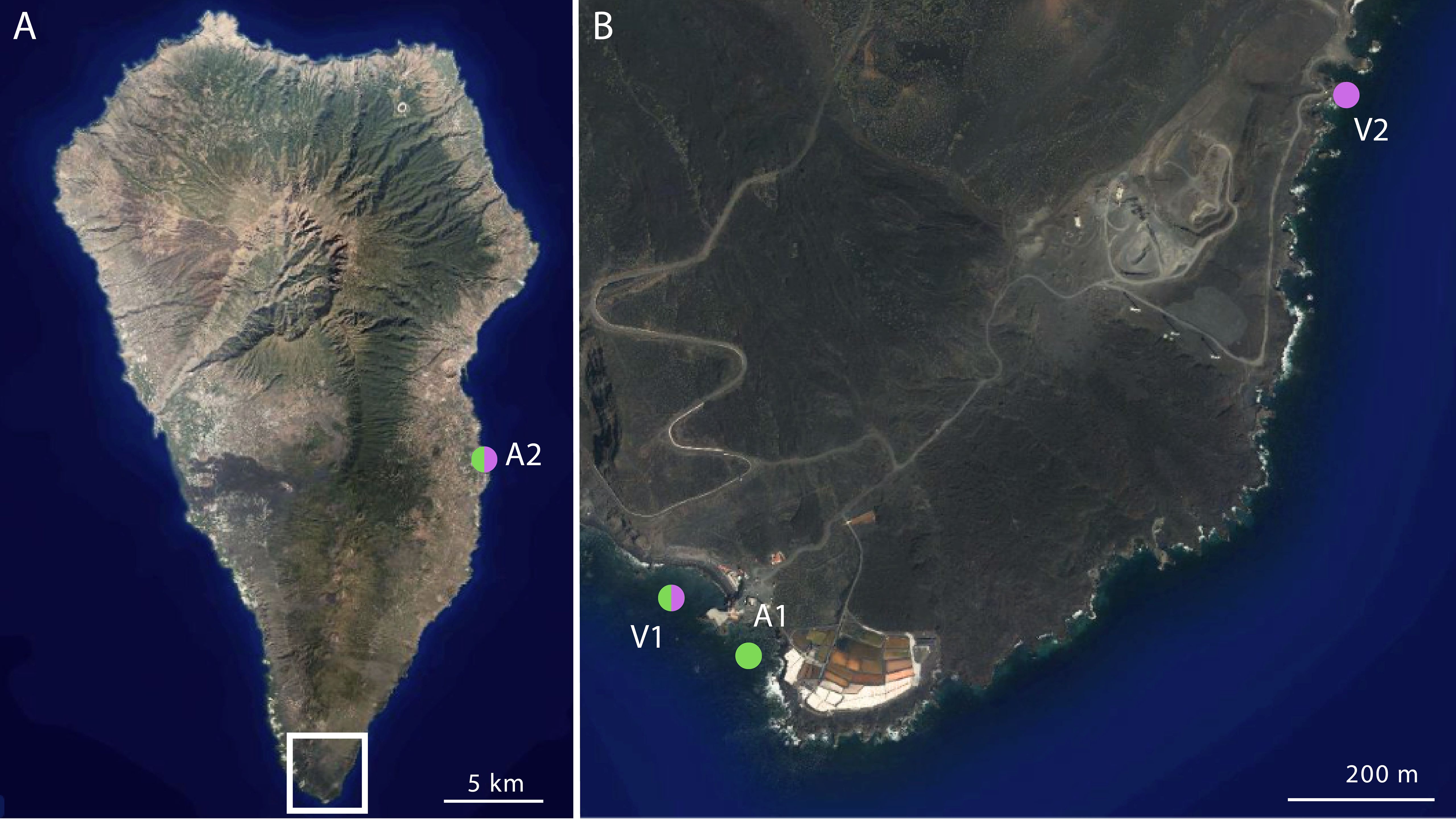
Figure 1. (A, B) Sampling sites at La Palma (Canary Islands, north-eastern Atlantic Ocean). Ambient sampling sites: El Muelle del Faro (A1), and La Bajita (A2) with pH ~8.1. Vent sampling sites: Playa del Faro (V1), and Las Cabras (V2) with pH ~7.5-7.8. Green dots are the sampling sites for Paracentrotus lividus, and purple dots are the sampling sites for Arbacia lixula. The maps used are distributed in the public domain (https://www.grafcan.es/, last accessed: 30 January 2024).
This study investigates how the metabolic rates vary in two calcifying echinoderms, the sea urchins P. lividus and A. lixula, under different pH conditions during experiments in a laboratory and along a naturally acidified area. The main goal is to better understand their physiological responses to OA conditions predicted for the near future and whether those responses are a result of large phenotypic plasticity or local adaptation to long-term exposure to low pH. Specifically, we measured metabolic responses by analyzing respiration rates (VO2 consumption) of organisms under experimental pH changes to assess their innate plasticity to pH shifts. Additionally, we compared organisms from natural CO2 vent systems (pH ~7.5) and ambient sites (pH ~8.1) to identify potential adaptive differences between them. We expected organisms from more stable environments to be more affected by environmental changes, while those accustomed to greater environmental variability may respond more effectively, having adapted to wider fluctuations in abiotic factors (Asnicar et al., 2021). It is critical to have a clear understanding of when plastic responses occur instead of genetic adaptations and vice versa, as this knowledge is essential for predicting how OA affects the distribution and abundance of species and thus predicting the responses of marine organisms to ongoing climate change.
Materials and methods
Our study is divided into two different parts. For investigating the plasticity response over time experiments were performed with individuals collected from ambient sites at pH 8.1 to measure their plasticity responses to low pH conditions over seven–days. Adaptation and plasticity under changing pH was analyzed using individuals living along a pH gradient from a natural CO2 vent system. Each part of the study was performed in a different laboratory and locality due to infrastructure and field requirements.
Plasticity response over time
To measure metabolic responses to experimental changes of pH, we collected individuals by freediving at 0.5–5 m depth at Cala Sant Francesc in Blanes (NW Mediterranean) (see Supplementary Table S1). All specimens collected were adults with volumes between 16.72–38.6 mL in P. lividus and 8.55–27.76 mL in A. lixula. Twelve individuals of P. lividus were collected in February 2024, six specimens were used for control conditions (individuals maintained at the same collection chemical parameters) and six specimens for experimental conditions (individuals collected and exposed to low pH)(Supplementary Table S2 for chemical parameters). For A.lixula six specimens were collected in December 2023 for control conditions and six in May 2024 for experimental conditions (Supplementary Table S3 for chemical parameters). The individuals maintained at the collected parameters (control condition) were used to control the housing effect. All experiments were conducted at the University of Barcelona. Seawater pH at the sampling point was measured with the multiparametric sensor AAQ-RINKO by JFE Advantech Co., Ltd. Experiments were conducted separately for both species, following the same protocol.
Once in the laboratory, animals were acclimatized in a 25L settling tank. After 48 hours, they were transferred to experimental tanks (125L), containing individual 10L tanks for each replicate. Then, they were exposed to either control or experimental low-pH conditions for seven days as explained above. Oxygen consumption (VO2 consumption) of each specimen was measured before placing it in the experimental tank (Day 0) and after 24h (Day 1), 48h (Day 2), 72h (Day 3), and 168h (Day 7) of the experiment. Animals were allowed to feed over the rocks placed at the bottom of the tank throughout the experiments. However, before each VO2 measurement of the corresponding days, animals were starved for 12h to ensure a similar physiological status for all of them (Grosjean, 1998; Shpigel et al., 2004). Values of pH were achieved using a pH-controlling setup (Milwaukee PRO Digital controller) connected to a pH electrode which regulated pH by bubbling CO2 gas into the seawater to maintain or reach the desired pH 7.5. Dissolved oxygen (DO) levels were maintained by the simultaneously bubbling air. Alkalinity was also checked using a SERA KH test to keep it constant at 11–14 dKH by adding Reef Carbonate (4,000 meq/L) from Seachem when necessary. Temperature was experimentally maintained at the temperature of the collection day (16°C±1°C) using a cooler (HAILEA HS28A).
Respiration rate (VO2; mg/kg/h) was independently measured per specimen using an intermittent-flow respirometry system (Q-box mini-AQUA aquatic respirometer, Qubit Systems) with the Logger Pro 3.16.1 software from Vernier Software Technology. A respirometer chamber was placed in a 20L aquarium equipped with a pH-controlling setup connected to a pH electrode to control the desired pH and a cooler to maintain a constant temperature. The total volume of the respirometry setup was 465ml. Once introduced into the chamber, the specimens were allowed 5 minutes to acclimate as performed by Christensen et al. (2011). The decreasing DO levels were measured with three cycles of oxygen consumption consisting of a 5-minute flush phase, 7-minute rest phase, and 10-minutes of closed circulation. A run without specimen (blank run) was performed before each condition to control the background VO2 consumption from microorganisms. Each specimen was weighed (g), and the height (mm) and the width (mm) were measured to calculate its volume (mL) using the Elliott et al. (2012) formula:
The respiration rate for each specimen was calculated with the difference between final VO2 and background VO2 (blank run).
Adaptation and rapid response under natural pH gradients
To determine potential differences in metabolic rates for individuals that naturally live under different pH values, we collected adult individuals of both species in La Palma (Canary Islands, Spain) in four different places in January 2024 (see Supplementary Table S1). We sampled at two “Ambient” sites (pH 8.1) at Muelle del Faro (A1) for P. lividus and La Bajita (A2) for both species, and two CO2 “Vent” sites at Playa del Faro (V1) for both species with a pH fluctuation between 8.01–7.4, and Las Cabras (V2) for A. lixula, with a pH fluctuation of 8.0–7.7 (See Figure 1, Supplementary Table S1) (González-Delgado et al., 2021; Hernández et al., 2016). Specimens were collected following the same criteria applied before. There were some differences in the sampling scheme and experimental design between species due to their different abundances at the sampling points. The analyses were conducted at the Observatorio Marino de Cambio Climático (OMACC) in Fuencaliente, La Palma Island.
The experiments were conducted using freshly collected seawater from the sampling points keeping the variables salinity and temperature constant (see Supplementary Tables S2, S3). The temperature, salinity, and pH were measured at each sampling point using a multiparametric sensor (AAQ-RINKO by JFE Advantech Co., Ltd.) and specimens were acclimatized at the same seawater parameters as the collection site. Unfortunately, due to infrastructure limitations, experiments at the OMACC had to be limited to a 24-hour acclimatization. After acclimatization in the settling tank (25L), the specimens were transferred to an experimental tank (125L), with individual tanks (10L) inside for each replicate. Following a 24-hour exposure period to the conditions described below, the VO2 consumption was measured for each specimen after 12h of starvation and following the same protocol previously detailed.
To investigate if metabolic rates vary due to local adaptation to pH, we tested individuals from the sampling sites under their natural conditions, hereafter basal conditions. For P. lividus (N=24), we collected eight samples from each of the three locations: Ambient 1 (A1A, pH = 8.1), Ambient 2 (A2A, pH = 8.1), and Vent 1 (V1V, pH = 7.5). For A. lixula (N=24), we took eight samples from three locations as well: Ambient 2 (A2A, pH = 8.1), Vent 1 (V1V, pH = 7.5), and Vent 2 (V2V, pH = 7.8). A. lixula was not present in the Ambient 1 site, thus we collected only ambient specimens from La Bajita (A2). Therefore, achieving equivalent sampling points for both species was not feasible, although the design was maintained as initially planned.
Finally, to determine whether specimens which are geographically closely related but living under different pH conditions do display different immediate responses to pH changes, we experimentally varied pH values in the laboratory, hereafter experimental conditions. In P. lividus, eight specimens from the Vent 1 were exposed to ambient pH (V1A: pH=8.1), and eight specimens from Ambient 2 were exposed to low pH (A2V: pH=7.5). In A. lixula, eight specimens from Ambient 2 were exposed to low pH (A2V: pH= 7.5). The abundance of individuals of A. lixula in both vent sites was low, which prevented us from conducting the exposure of specimens from the vent to ambient pH.
Statistical analysis
For the plasticity response over time experiments, we fitted mixed-effects models (Harrison et al., 2018) to evaluate the interaction between TREAT (Control vs pH 7.5) and DAY (Day 0, Day 1, Day 2, Day 3 and Day 7) on VO2 consumption, incorporating random effects for individuals, thereby accounting for repeated measures. The analyses were conducted in RStudio version 4.2.2 (2022-10-31; R Core Team, 2022) and using lme4 package (Bates et al., 2015). Different distributions (Normal and Gamma) and log transformations were tested. Models were selected based on the lowest AIC and convergence (Akaike, 1998). To further explore the interaction effects, post-hoc and pairwise comparisons were performed using emmeans package (Lenth, 2024). To illustrate the results, box plots were created using the ggplot2 package (Wickham, 2016). The normality of the residuals of the models was tested with the Shapiro-Wilk test.
For investigating the adaptation and rapid response under natural pH gradients, we compared the VO2 consumption among individuals in their basal and experimental conditions. We normalized the VO2 values of A. lixula using a log transformation whereas the transformation was not needed for P. lividus data. Initially, normality of the residuals was checked using the Shapiro-Wilk test, while the homogeneity of variances was evaluated for the variables ‘meanVO2’ (dependent variable) and ‘pH’ (independent variable) using the Levene test through rstatix package (Kassambara, 2023). An analysis of variance (ANOVA) was performed in RStudio followed by the post-hoc Tukey test through the function TukeyHSD (R Core Team, 2022) to determine differences between the respiration rates from vent and ambient specimens and at different pH conditions. Boxplots were created for data visualization using the ggplot2 package.
Results
Plasticity response over time
The VO2 consumption of both species was log-transformed to normalize the data for subsequent analyses. A linear mixed model was then employed for P. lividus, as its residuals were normally distributed, whereas a generalized mixed model with gamma distribution was applied for A. lixula, to account for its non-normal distribution. The best model in both species followed the formula: VO2~DAY*TREAT+(1|IND). The Shapiro-Wilk test confirmed the normal distribution of residuals for both species after applying the respective models (P. lividus, p = 0.053; A. lixula, p = 0.07).
We assessed mean VO2 across days under control conditions for each species to account for potential housing effects, we assessed mean VO2 across days under control conditions for each species. In P. lividus, the VO2 consumption under control conditions ranged from 7.70 to 24.65 mg/kg/h over the seven days, showing minor variations in respiration rates over time (Figure 2, Supplementary Table S4). Although comparisons among some days were significantly different (Supplementary Tables S5), the overall pattern remained stable, indicating no major housing effect. For A. lixula, there was no evidence of housing effect, as VO2 consumption range of 8.00 to 18.13 mg/kg/h and showed no significant differences among days under control conditions (Figure 2, Supplementary Tables S4, S5).
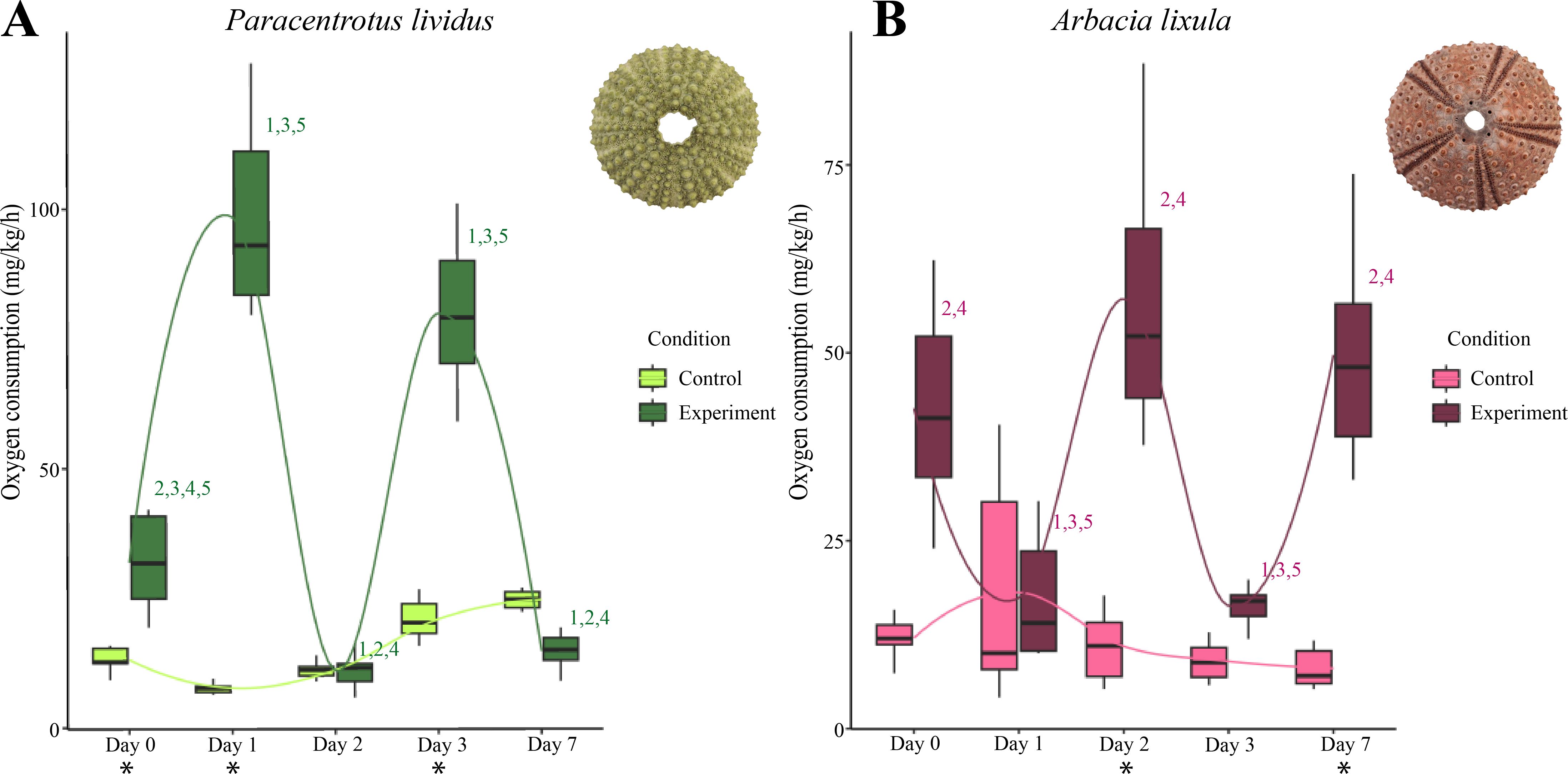
Figure 2. Plasticity responses over time. Means of VO2 consumption from all the specimens on different experimental conditions of pH in Paracentrotus lividus (A) and Arbacia lixula (B). For control conditions the pH was 8.1 and for experiment conditions the pH was 7.5 in both species. The pH during the Day 0 was 8.1 in both species and conditions. The numbers above the bars indicate the significance of pairwise comparisons (padj<0.05) among days in experimental conditions. Asterisks below pH treatment of each day indicate (padj<0.05) between control and experimental conditions.
In P. lividus, VO2 consumption during the experimental condition was consistent on Day 0, Day 2, and Day 7 (11.00–31.90 mg/kg/h) but spiked on Day 1 and Day 3 (98.50– 79.80 mg/kg/h). In A. lixula the VO2 consumption was stable on Day 0, Day 2, and Day 7 (42.60–57.20 mg/kg/h), but dropped significantly on Day 1, and Day 3 (17.40–16.31 mg/kg/h) (Figure 2, Supplementary Material Tables S4, S5). Interestingly, for each species the VO2 consumption before low pH exposure (Day 0) and the last day of experiment at low pH 7.5 (Day 7) showed similar values. However, only A. lixula showed no significant difference among those values (Supplementary Table S5).
Adaptation and rapid response under natural pH gradients
The Shapiro-Wilk test confirmed normal distribution of the residuals for both, P. lividus (p = 0.68), and A. lixula (p = 0.73). The Levene test proved homogeneity of variances for both, P. lividus (p = 0.29), and A. lixula (p = 0.13).
The ANOVA analysis indicated significant differences among the measured respiration rates in both species (P. lividus: F= 20.94, p <0.05; A. lixula: F= 25.17, p < 0.05) across all conditions, indicating differences in the VO2 consumption after the 24 hours of the experiment. Pairwise comparisons (Tukey tests) found no significant differences in basal VO2 consumption between specimens from the Vent and Ambient sites in both species (Figure 3, Supplementary Tables S6–8). Basal conditions in P. lividus, both ambient (A1 the closest to the Vent site and A2 the furthest to the Vent site) and the Vent showed a similar VO2 consumption (29.00 mg/kg/h). Moreover, in A. lixula, both Vents and the Ambient ranged from 27.50 to 53.20 mg/kg/h. Significant differences were found between the experimental and the basal conditions in both species (Figure 3, Supplementary Tables S6–8). P. lividus from ambient sites exposed to pH 7.5 showed a mean VO2 consumption (A2V = 150.90 mg/kg/h) twice as high as the individuals from the Vent exposed to ambient pH (V1A = 74.20 mg/kg/h) (Figure 3). A. lixula from the Ambient exposed to pH 7.5 (A2V = 276.41 mg/kg/h) increased 10 times the VO2 consumption compared to the basal levels (27.50–53.20 mg/kg/h).
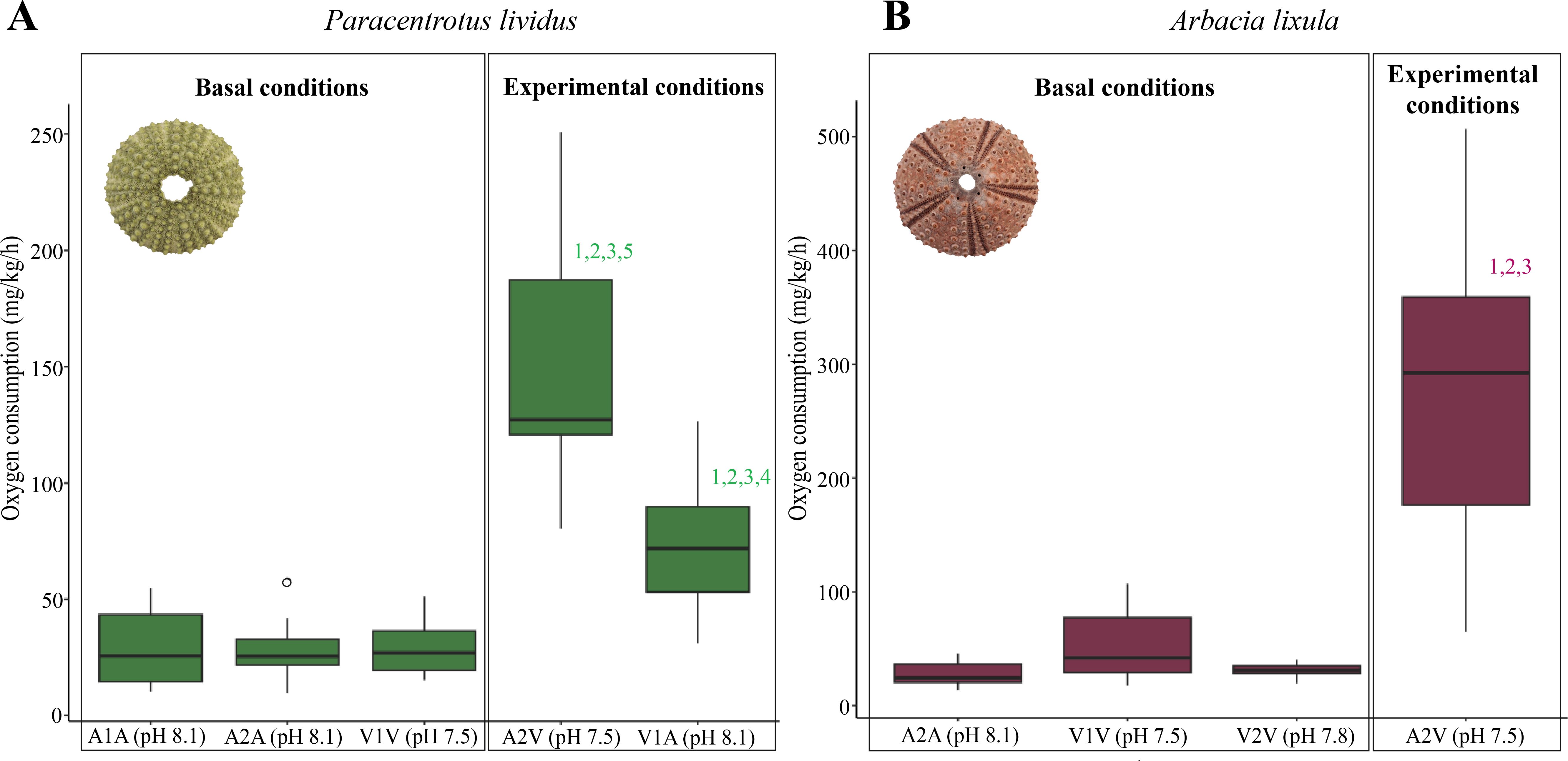
Figure 3. Adaptation and rapid responses to pH changes. Means of VO2 consumption from all the specimens on different experimental conditions of pH in Paracentrotus lividus (A) and Arbacia lixula (B). The exposure to each condition lasted 24 hours in both species. The numbers above the bars indicate the significance of pairwise comparisons (padj<0.05) among days in experimental conditions.
Discussion
Echinoderms, as marine calcifiers, are among the most sensitive organisms to future OA conditions (Byrne and Hernández, 2020; Byrne and Fitzer, 2019). Studies that combine laboratory experiments and OA natural analogues are useful for assessing the stress response and long-term effects of OA (Hernández et al., 2024). In the present study, we combined the study of short, medium, and long-term metabolic responses to OA conditions in two keystone species of sea urchins.
The ability of sea urchins such as P. lividus and A. lixula to cope with pH fluctuations stems from their coelomic fluid’s buffering capacity (Calosi et al., 2013; Di Giglio et al., 2020). Moreover, other physiological processes such as osmoregulation, and excretion, are also interrelated for maintaining metabolic activities (Catarino et al., 2012). After seven days of experiments at acidified conditions (pH=7.5), respiration rates of both species reached similar VO2 levels to Day 0 (pH=8.1) before the low pH exposure, indicating a potential recovery of the basal respiration rates. However, A. lixula showed significant differences when compared to the respiration rates found in the controls, possibly related to the sampling season. The specimens for the control conditions were collected at the end of December of 2023 while the specimens used for the experimental conditions were collected at the beginning of May of 2024. Although similar seawater temperature (16°C ± 1°C), other internal physiological (such as reproductive season) and external ecological (such as food supply) processes might be influencing the respiration rate of collected specimens (Burton et al., 2011).
During the seven days of low pH exposure, VO2 consumption alternate between increases and decreases in both species, following opposite patterns. In P. lividus, elevated respiration rates may be explained through metabolic upregulation to compensate for tissue acidosis (Stumpp et al., 2012). This finding aligns with Calosi et al. (2013), who reported that P. lividus adjusts to low pH by accumulating bicarbonates, a buffering mechanism likely requiring increased metabolic activity. In contrast, A. lixula exhibited an initial decrease in VO2 consumption, likely due to its non-bicarbonate buffering mechanisms requiring less metabolic adjustment (Calosi et al., 2013). These distinct physiological responses underline the species-specific strategies for coping with low pH.
Both species show potential for adaptation and/or long-term acclimatization in physiological responses since the basal respiration rates showed no differences between populations from the ambient sites (pH=8.1) and the CO2 vent sites (pH=7.5 and 7.8) at La Palma. Migliaccio et al. (2019) also found a lack of differences in P. lividus respiration rates between specimens from a CO2 vent and ambient sites, suggesting physiological mechanisms to survive under OA. Acclimatization to OA over time is not rare in sea urchins since several studies have already found no differences in respiration rates between pH treatments in the long-term, for instance, P. lividus (Catarino et al., 2012; Cohen-Rengifo et al., 2019), Echinometra sp (Asnicar et al., 2021; Moulin et al., 2014; Uthicke et al., 2020, 2021), Hemicentrotus pulcherrimus (Kurihara et al., 2013), Strongylocentrotus droebachiensis (Stumpp et al., 2012), and Sterechinus neumayeri (Suckling et al., 2015). However, studies such as Holtmann (2013) and Marčeta et al. (2020), indicate that maintaining coelomic fluid pH may involve metabolic trade-offs impacting growth, reproduction and immune functions. In this study, the sex of the individuals was not recorded to avoid sacrificing them. Marčeta et al. (2020) found that females exposed to acidified conditions showed reduced gonad quality and impaired oocyte development. These findings suggests that sex-specific differences could exist in the responses of P. lividus and A. lixula to OA, with females potentially being more sensitive due to the energetic demands of reproduction. While maintaining acid-base homeostasis is energetically demanding and can reduce the energy available for other essential processes (Catarino et al., 2012; Collard et al., 2013) it may not fully explain the shifts in energy budgets observed in OA environments. Indeed, other cofounding factors could contribute to a shift in energy budgets and VO2 consumption rates, such as biomineralization (Kurihara et al., 2012). These trade-offs further highlight the metabolic challenges associated with ocean acidification (Dupont and Thorndyke, 2012; Stumpp et al., 2012; Hernroth et al., 2011).
To assess the phenotypic plasticity, individuals from ambient sites in La Palma of both species were placed under short-term exposure to low pH conditions (pH=7.5). VO2 consumption increased significantly, especially in A. lixula, indicating a stress response to the sudden change. Interestingly, P. lividus showed a higher increase in VO2 consumption when ambient individuals were exposed to low pH conditions (A-V) compared to vent specimens exposed to ambient pH conditions (V-A). This might be related to the natural exposure to fluctuating pH of individuals living at vent sites, ranging from pH=8.1 to pH=7.4 during tides (González-Delgado et al., 2021) while organisms from ambient sites live under constant pH values (pH=8.1). Differences in food consumption at varying pH levels may have influenced the observed VO2 patterns, as sea urchins might adjust feeding to cope with acidification stress, aligning with other studies (e.g., Dupont et al., 2010). However, food consumption was not directly measured in this study. Populations exposed to high environmental variability may develop tolerance to environmental stressors (Asnicar et al., 2021; Teixidó et al., 2020) as well as maternal anticipatory effects (Palombo et al., 2023; Karelitz et al., 2019; Thor and Dupont, 2015). Studies have shown that echinoderms exhibit reduced sensitivity to OA when exposed to a highly variable environmental history (Asnicar et al., 2022; Kapsenberg and Cyronak, 2019) indicating higher phenotypic plasticity in specimens from more fluctuating environments, such as the CO2 vent sites studied here. In those lines, high pH variability areas have been proposed as OA refugia, offering adaptive advantages for marine organisms (Bednaršek et al., 2022; García et al., 2018; Kapsenberg and Cyronak, 2019). Thus, CO2 vent systems in particular, may serve as significant OA refugia to consider.
While P. lividus and A. lixula may acclimate their respiration rates to low pH over time, understanding their long-term physiological responses is key to predicting viability and ecosystem dynamics. This study suggests that adult sea urchins of both species can potentially acclimate to low pH conditions over the long-term, supporting previous findings (Calosi et al., 2013; Collard et al., 2013; Di Giglio et al., 2020; and Migliaccio et al., 2019). Incorporating sex identification and measuring feeding rates could reveal sex-specific differences and clarify dietary roles in metabolic homeostasis. Future research combining physiological data with population genomics and transcriptomics could uncover adaptive mechanisms and their inheritance.
Data availability statement
The original contributions presented in the study are included in the article/supplementary material. Further inquiries can be directed to the corresponding authors.
Author contributions
RF: Conceptualization, Data curation, Formal analysis, Investigation, Methodology, Writing – original draft, Writing – review & editing, Visualization. VA: Conceptualization, Formal analysis, Investigation, Methodology, Writing – original draft, Writing – review & editing, Data curation, Funding acquisition, Visualization. MM: Data curation, Formal analysis, Methodology, Writing – review & editing, Investigation, Visualization. JH: Conceptualization, Methodology, Resources, Supervision, Writing – review & editing, Funding acquisition. SG: Writing – review & editing, Investigation, Methodology. RP: Conceptualization, Funding acquisition, Methodology, Project administration, Resources, Supervision, Validation, Visualization, Writing – review & editing.
Funding
The author(s) declare financial support was received for the research, authorship, and/or publication of this article. This research was funded by the Spanish Government projects ACIDOMIC (CNS2022-135968 funded by MCIN/AEO/10.13039/501100011033 and, by the European Union NextGeneration EU/PRTR), ENVIOME (PID2021-128094NB-I00/MCIN/AEI/10.13039/501100011033/and FEDER una manera de hacer Europa), and ADAPTIVE (PGC2018-100735-B-I00/MCIU/AEI/FEDER, UE), and a “Ramón y Cajal” contract to RP (RYC2018-025070-I), the project “DIVERGEN- Ayudas Fundación BBVA a Proyectos Investigación Científica 2021”, and the Marie Sklodowska-Curie grant (agreement No 101105400 funded by the European Union’s Horizon Europe research and innovation program to VA). This paper is a contribution of the Consolidated Research Team: 2021 SGR 01271 Marine Biodiversity and Evolution (MBE).
Acknowledgments
Thanks to the Observatorio Marino de Cambio Climático (OMACC) from La Palma for letting us use their facilities at the Fuencaliente Lighthouse to carry out these experiments; to the QUIMA group of IOCAG, (ULPGC, Spain) for providing us with the chemical data of the sampling sites (Supplementary Table S2); to Dr. Quesada, from the Museum of Natural Science of Barcelona, for assisting us with the statistical analysis of our data; to the four reviewers for their valuable comments and suggestions, which have greatly improved the quality of this manuscript and its clarity. Also, thanks to Nancy and Adam for their help and support during our field trips to Fuencaliente, La Palma Island.
Conflict of interest
The authors declare that the research was conducted in the absence of any commercial or financial relationships that could be construed as a potential conflict of interest.
The author(s) declared that they were an editorial board member of Frontiers, at the time of submission. This had no impact on the peer review process and the final decision.
Publisher’s note
All claims expressed in this article are solely those of the authors and do not necessarily represent those of their affiliated organizations, or those of the publisher, the editors and the reviewers. Any product that may be evaluated in this article, or claim that may be made by its manufacturer, is not guaranteed or endorsed by the publisher.
Supplementary material
The Supplementary Material for this article can be found online at: https://www.frontiersin.org/articles/10.3389/fmars.2025.1500646/full#supplementary-material
References
Agnetta D., Badalamenti F., Ceccherelli G., Di Trapani F., Bonaviri C., Gianguzza P. (2015). Role of two co-occurring Mediterranean sea urchins in the formation of barren from Cystoseira canopy. Estuarine Coast. Shelf Sci. 152, 73–77. doi: 10.1016/j.ecss.2014.11.023
Akaike H. (1998). “Information theory and an extension of the maximum likelihood principle,” in Selected papers of hirotugu akaike (Springer New York, New York, NY), 199–213. doi: 10.1007/978-1-4612-1694-0_15
Allan R. P., Hawkins E., Bellouin N., Collins B. (2021). “Summary for policymakers,” in Climate Change 2021 – The Physical Science Basis (Cambridge, United Kingdom: Cambridge University Press), 3–32. doi: 10.1017/9781009157896.001
Asnicar D., Novoa-Abelleira A., Minichino R., Badocco D., Pastore P., Finos L., et al. (2021). When site matters: Metabolic and behavioural responses of adult sea urchins from different environments during long-term exposure to seawater acidification. Mar. Environ. Res. 169, 105372. doi: 10.1016/j.marenvres.2021.105372
Asnicar D., Zanovello L., Badocco D., Munari M., Marin M. G. (2022). Different ecological histories of sea urchins acclimated to reduced pH influence offspring response to multiple stressors. Environ. Res. 212, 113131. doi: 10.1016/j.envres.2022.113131
Bates D., Mächler M., Bolker B., Walker S. (2015). Fitting linear mixed-effects models using lme4. J. Stat. Software 67, 1–48. doi: 10.18637/jss.v067.i01
Bednaršek N., Beck M. W., Pelletier G., Applebaum S. L., Feely R. A., Butler R., et al. (2022). Natural Analogues in pH Variability and Predictability across the Coastal Pacific Estuaries: Extrapolation of the Increased Oyster Dissolution under Increased pH Amplitude and Low Predictability Related to Ocean Acidification. Environ. Sci. Technol. 56, 9015–9028. doi: 10.1021/acs.est.2c00010
Bonaviri C., Vega Fernández T., Fanelli G., Badalamenti F., Gianguzza P. (2011). Leading role of the sea urchin Arbacia lixula in maintaining the barren state in southwestern Mediterranean. Mar. Biol. 158, 2505–2513. doi: 10.1007/s00227-011-1751-2
Brown J. H., Gillooly J. F., Allen A. P., Savage V. M., West G. B. (2004). Toward a metabolic theory of ecology. Ecology 85, 1771–1789. doi: 10.1890/03-9000
Burton T., Killen S. S., Armstrong J. D., Metcalfe N. B. (2011). What causes intraspecific variation in resting metabolic rate and what are its ecological consequences? Proc. R. Soc. B: Biol. Sci. 278, 3465–3473. doi: 10.1098/rspb.2011.1778
Byrne M., Fitzer S. (2019). The impact of environmental acidification on the microstructure and mechanical integrity of marine invertebrate skeletons. Conserv. Physiol. 7, coz062. doi: 10.1093/conphys/coz062
Byrne M., Hernández J. C. (2020). “Sea urchins in a high CO2 world: Impacts of climate warming and ocean acidification across life history stages,” in Developments in Aquaculture and Fisheries Science, vol. 43. (Amsterdam, Netherlands: Elsevier B.V), 281–297. doi: 10.1016/B978-0-12-819570-3.00016-0
Calosi P., Rastrick S. P. S., Graziano M., Thomas S. C., Baggini C., Carter H. A., et al. (2013). Distribution of sea urchins living near shallow water CO2 vents is dependent upon species acid–base and ion-regulatory abilities. Mar. pollut. Bull. 73, 470–484. doi: 10.1016/j.marpolbul.2012.11.040
Carracedo J., Badiola E., Guillou H., de la Nuez J., Perez F., Perez Torrado F. (2001). “Geology and volcanology of La Palma and El Hierro, Western Canaries,” in Estudios Geologicos-Madrid (Madrid, Spain: Consejo Superior de Investigaciones Científicas (CSIC)), vol. 57. Available at: https://hal.science/hal-03323419 (Accessed January 27, 2025).
Catarino A. I., Bauwens M., Dubois P. (2012). Acid–base balance and metabolic response of the sea urchin Paracentrotus lividus to different seawater pH and temperatures. Environ. Sci. pollut. Res. 19, 2344–2353. doi: 10.1007/s11356-012-0743-1
Christensen A. B., Nguyen H. D., Byrne M. (2011). Thermotolerance and the effects of hypercapnia on the metabolic rate of the ophiuroid Ophionereis schayeri: Inferences for survivorship in a changing ocean. J. Exp. Mar. Biol. Ecol. 403, 31–38. doi: 10.1016/j.jembe.2011.04.002
Cohen-Rengifo M., Agüera A., Bouma T., M’Zoudi S., Flammang P., Dubois P. (2019). Ocean warming and acidification alter the behavioral response to flow of the sea urchin Paracentrotus lividus. Ecol. Evol. 9, 12128–12143. doi: 10.1002/ece3.5678
Collard M., Laitat K., Moulin L., Catarino A. I., Grosjean P., Dubois P. (2013). Buffer capacity of the coelomic fluid in echinoderms. Comp. Biochem. Physiol. Part A: Mol. Integr. Physiol. 166, 199–206. doi: 10.1016/j.cbpa.2013.06.002
Di Giglio S., Spatafora D., Milazzo M., M’Zoudi S., Zito F., Dubois P., et al. (2020). Are control of extracellular acid-base balance and regulation of skeleton genes linked to resistance to ocean acidification in adult sea urchins? Sci. Total Environ. 720, 137443. doi: 10.1016/j.scitotenv.2020.137443
Dupont S., Ortega-Martínez O., Thorndyke M. (2010). Impact of near-future ocean acidification on echinoderms. Ecotoxicology 19, 449–462. doi: 10.1007/s10646-010-0463-6
Dupont S., Pörtner H. (2013). Get ready for ocean acidification. Nature 498, 429–429. doi: 10.1038/498429a
Dupont S., Thorndyke M. (2012). Relationship between CO2-driven changes in extracellular acid–base balance and cellular immune response in two polar echinoderm species. J. Exp. Mar. Biol. Ecol. 424–425, 32–37. doi: 10.1016/j.jembe.2012.05.007
Elliott L., Russell M., Hernández J. (2012). “Estimating Echinoid test volume from height and diameter measurements,” in Echinoderms in a Changing World: Proceedings of the 13th International Echinoderm Conference (Boca Raton, Florida, USA: CRC Press), 105. University of Tasmania (Ed.).
Foo S. A., Munari M., Gambi M. C., Byrne M. (2022). Acclimation to low pH does not affect the thermal tolerance of Arbacia lixula progeny. Biol. Lett. 18, 20220087. doi: 10.1098/rsbl.2022.0087
García E., Clemente S., Hernández J. C. (2018). Effects of natural current pH variability on the sea urchin Paracentrotus lividus larvae development and settlement. Mar. Environ. Res. 139, 11–18. doi: 10.1016/j.marenvres.2018.04.012
González-Delgado S., González-Santana D., Santana-Casiano M., González-Dávila M., Hernández C. A., Sangil C., et al. (2021). Chemical characterization of the Punta de Fuencaliente CO2-enriched system (La Palma, NE Atlantic Ocean): a new natural laboratory for ocean acidification studies. Biogeosciences 18, 1673–1687. doi: 10.5194/bg-18-1673-2021
González-Delgado S., Hernández J. C. (2018). “The importance of natural acidified systems in the study of ocean acidification: what have we learned?,” in Advances in Marine Biology, vol. 80. (London, United Kingdom: Academic Press), 57–99. doi: 10.1016/bs.amb.2018.08.001
González-Delgado S., Pérez-Portela R., Ortega-Martínez O., Alfonso B., Pereyra R. T., Hernández J. C. (2024). Genomic signals of adaptation to a natural CO2 gradient over a striking microgeographic scale. Mar. pollut. Bull. 209, 117225. doi: 10.1016/j.marpolbul.2024.117225
González-Delgado S., Wangensteen O. S., Sangil C., Hernández C. A., Alfonso B., Soto A. Z., et al. (2023). High taxonomic diversity and miniaturization in benthic communities under persistent natural CO2 disturbances. Proc. R. Soc. B: Biol. Sci. 290, 20222417. doi: 10.1098/rspb.2022.2417
Grosjean P. (1998). Land-based, closed-cycle echiniculture of Paracentrotus lividus (Lamarck) (Echinoidea: Echinodermata): A long-term experiment at a pilot scale. Article J. Shellfish Res. 17, 1523–31.
Harrison X. A., Donaldson L., Correa-Cano M. E., Evans J., Fisher D. N., Goodwin C. E., et al. (2018). A brief introduction to mixed effects modelling and multi-model inference in ecology. PeerJ 6, e4794. doi: 10.7717/peerj.4794
Hernández J. C., González-Delgado S., Aliende-Hernández M., Alfonso B., Rufino-Navarro A., Hernández C. A. (2024). Natural acidified marine systems: Lessons and predictions. Adv. Mar. Biol. 100, 1–45. doi: 10.1016/BS.AMB.2024.08.002
Hernández C. A., Sangil C., Hernández J. C. (2016). A new CO2 vent for the study of ocean acidification in the Atlantic. Mar. pollut. Bull. 109, 419–426. doi: 10.1016/j.marpolbul.2016.05.040
Hernroth B., Baden S., Thorndyke M., Dupont S. (2011). Immune suppression of the echinoderm Asterias rubens (L.) following long-term ocean acidification. Aquat. Toxicol. 103, 222–224. doi: 10.1016/j.aquatox.2011.03.001
Hofmann G. E., Evans T. G., Kelly M. W., Padilla-Gamiño J. L., Blanchette C. A., Washburn L., et al. (2014). Exploring local adaptation and the ocean acidification seascape – studies in the California Current Large Marine Ecosystem. Biogeosciences 11, 1053–1064. doi: 10.5194/bg-11-1053-2014
Holtmann W. C. (2013). Maintenance of coelomic fluid pH in sea urchins exposed to elevated CO2: the role of body cavity epithelia and stereom dissolution. Mar. Biol. 160, 2631–2645. doi: 10.1007/s00227-013-2257-x
Kapsenberg L., Cyronak T. (2019). Ocean acidification refugia in variable environments. Global Change Biol. 25, 3201–3214. doi: 10.1111/gcb.14730
Karelitz S., Lamare M. D., Mos B., De Bari H., Dworjanyn S. A., Byrne M. (2019). Impact of growing up in a warmer, lower pH future on offspring performance: transgenerational plasticity in a pan-tropical sea urchin. Coral Reefs 38, 1085–1095. doi: 10.1007/s00338-019-01855-z
Kassambara A. (2023). rstatix: Pipe-friendly framework for basic statistical test. R Package Version 0.7.2. Available online at: https://CRAN.R-project.org/package=rstatix (Accessed January 27, 2025).
Kurihara H., Takano Y., Kurokawa D., Akasaka K. (2012). Ocean acidification reduces biomineralization-related gene expression in the sea urchin, Hemicentrotus pulcherrimus. Mar. Biol. 159, 2819–2826. doi: 10.1007/s00227-012-2043-1
Kurihara H., Yin R., Nishihara G., Soyano K., Ishimatsu A. (2013). Effect of ocean acidification on growth, gonad development and physiology of the sea urchin Hemicentrotus pulcherrimus. Aquat. Biol. 18, 281–292. doi: 10.3354/ab00510
Lenth R. V. (2024). emmeans: Estimated Marginal Means, aka Least-Squares Means (R package version 1.10.3). Available online at: https://CRAN.R-project.org/package=emmeans (Accessed January 27, 2025).
Marčeta T., Matozzo V., Alban S., Badocco D., Pastore P., Marin M. G. (2020). Do males and females respond differently to ocean acidification? An experimental study with the sea urchin Paracentrotus lividus. Environ. Sci. pollut. Res. 27, 39516–39530. doi: 10.1007/s11356-020-10040-7
Melzner F., Gutowska M. A., Langenbuch M., Dupont S., Lucassen M., Thorndyke M. C., et al. (2009). Physiological basis for high CO2; tolerance in marine ectothermic animals: pre-adaptation through lifestyle and ontogeny? Biogeosciences 6, 2313–2331. doi: 10.5194/bg-6-2313-2009
Migliaccio O., Pinsino A., Maffioli E., Smith A. M., Agnisola C., Matranga V., et al. (2019). Living in future ocean acidification, physiological adaptive responses of the immune system of sea urchins resident at a CO2 vent system. Sci. Total Environ. 672, 938–950. doi: 10.1016/j.scitotenv.2019.04.005
Moulin L., Grosjean P., Leblud J., Batigny A., Dubois P. (2014). Impact of elevated pCO2 on acid–base regulation of the sea urchin Echinometra mathaei and its relation to resistance to ocean acidification: A study in mesocosms. J. Exp. Mar. Biol. Ecol. 457, 97–104. doi: 10.1016/j.jembe.2014.04.007
Norin T., Metcalfe N. B. (2019). Ecological and evolutionary consequences of metabolic rate plasticity in response to environmental change. Philos. Trans. R. Soc. B: Biol. Sci. 374, 20180180. doi: 10.1098/rstb.2018.0180
Padrón E., Pérez N. M., Rodríguez F., Melián G., Hernández P. A., Sumino H., et al. (2015). Dynamics of diffuse carbon dioxide emissions from Cumbre Vieja volcano, La Palma, Canary Islands. Bull. Volcanology 77, 28. doi: 10.1007/s00445-015-0914-2
Palacı́n C., Giribet G., Carner S., Dantart L., Turon X. (1998). Low densities of sea urchins influence the structure of algal assemblages in the western Mediterranean. J. Sea Res. 39, 281–290. doi: 10.1016/S1385-1101(97)00061-0
Palombo C., Chiarore A., Ciscato M., Asnicar D., Mirasole A., Fabbrizzi E., et al. (2023). Thanks mum. Maternal effects in response to ocean acidification of sea urchin larvae at different ecologically relevant temperatures. Mar. pollut. Bull. 188, 114700. doi: 10.1016/j.marpolbul.2023.114700
Pörtner H. (2008). Ecosystem effects of ocean acidification in times of ocean warming: a physiologist’s view. Mar. Ecol. Prog. Ser. 373, 203–217. doi: 10.3354/meps07768
R Core Team (2022). “R: A language and environment for statistical computing,” in R Foundation for Statistical Computing (4.2.2) (Vienna, Austria: R Foundation for Statistical Computing). Available at: https://www.R-project.org/ (Accessed January 27, 2025).
Shpigel M., McBride S. C., Marciano S., Lupatsch I. (2004). The effect of photoperiod and temperature on the reproduction of European sea urchin Paracentrotus lividus. Aquaculture 232, 343–355. doi: 10.1016/S0044-8486(03)00539-8
Spicer J. I., Widdicombe S., Needham H. R., Berge J. A. (2011). Impact of CO2-acidified seawater on the extracellular acid–base balance of the northern sea urchin Strongylocentrotus dröebachiensis. J. Exp. Mar. Biol. Ecol. 407, 19–25. doi: 10.1016/j.jembe.2011.07.003
Stumpp M., Hu M., Casties I., Saborowski R., Bleich M., Melzner F., et al. (2013). Digestion in sea urchin larvae impaired under ocean acidification. Nat. Climate Change 3, 1044–1049. doi: 10.1038/nclimate2028
Stumpp M., Trübenbach K., Brennecke D., Hu M. Y., Melzner F. (2012). Resource allocation and extracellular acid–base status in the sea urchin Strongylocentrotus droebachiensis in response to CO2 induced seawater acidification. Aquat. Toxicol. 110, 194–207. doi: 10.1016/j.aquatox.2011.12.020
Suckling C. C., Clark M. S., Richard J., Morley S. A., Thorne M. A. S., Harper E. M., et al. (2015). Adult acclimation to combined temperature and pH stressors significantly enhances reproductive outcomes compared to short-term exposures. J. Anim. Ecol. 84, 773–784. doi: 10.1111/1365-2656.12316
Teixidó N., Caroselli E., Alliouane S., Ceccarelli C., Comeau S., Gattuso J., et al. (2020). Ocean acidification causes variable trait-shifts in a coral species. Global Change Biol. 26, 6813–6830. doi: 10.1111/gcb.15372
Thor P., Dupont S. (2015). Transgenerational effects alleviate severe fecundity loss during ocean acidification in a ubiquitous planktonic copepod. Global Change Biol. 21, 2261–2271. doi: 10.1111/gcb.12815
Uthicke S., Deshpande N. P., Liddy M., Patel F., Lamare M., Wilkins M. R. (2019). Little evidence of adaptation potential to ocean acidification in sea urchins living in “Future Ocean” conditions at a CO2 vent. Ecol. Evol. 9, 10004–10016. doi: 10.1002/ece3.v9.17
Uthicke S., Ebert T., Liddy M., Johansson C., Fabricius K., Lamare M. (2016). Echinometra sea urchins acclimatised to elevated pCO2 at volcanic vents outperform those under present- day pCO2 conditions. Global Change Biol. 22, 2451–2461. doi: 10.1111/gcb.13223
Uthicke S., Patel F., Karelitz S., Luter H. M., Webster N. S., Lamare M. (2020). Key biological responses over two generations of the sea urchin Echinometra sp. A under future ocean conditions. Mar. Ecol. Prog. Ser. 637, 87–101. doi: 10.3354/meps13236
Uthicke S., Patel F., Petrik C., Watson S. A., Karelitz S., Lamare M. (2021). Cross-generational response of a tropical sea urchin to global change and a selection event in a 43-month mesocosm study. Global Change Biol. 27, 3448–3462. doi: 10.1111/gcb.v27.15
Vergés A., Steinberg P. D., Hay M. E., Poore A. G. B., Campbell A. H., Ballesteros E., et al. (2014). The tropicalization of temperate marine ecosystems: climate-mediated changes in herbivory and community phase shifts. Proc. R. Soc. B: Biol. Sci. 281, 20140846. doi: 10.1098/rspb.2014.0846
Wickham H. (2016). ggplot2: Elegant Graphics for Data Analysis (New York, Usa: Springer-Verlag New York). Available at: https://ggplot2.tidyverse.org (Accessed January 27, 2025).
Keywords: echinoderms, aquatic respirometry, metabolism, climate change, CO2 vent
Citation: Fernández-Vilert R, Arranz V, Martín-Huete M, Hernández JC, González-Delgado S and Pérez-Portela R (2025) Effect of ocean acidification on the oxygen consumption of the sea urchins Paracentrotus lividus (Lamarck, 1816) and Arbacia lixula (Linnaeus, 1758) living in CO2 natural gradients. Front. Mar. Sci. 12:1500646. doi: 10.3389/fmars.2025.1500646
Received: 23 September 2024; Accepted: 17 January 2025;
Published: 04 February 2025.
Edited by:
Caitlin Blain, The University of Auckland, New ZealandReviewed by:
Nathalie Oulhen, Brown University, United StatesFederica Costantini, University of Bologna, Italy
Ian Dixon-Anderson, University of Otago, New Zealand
Copyright © 2025 Fernández-Vilert, Arranz, Martín-Huete, Hernández, González-Delgado and Pérez-Portela. This is an open-access article distributed under the terms of the Creative Commons Attribution License (CC BY). The use, distribution or reproduction in other forums is permitted, provided the original author(s) and the copyright owner(s) are credited and that the original publication in this journal is cited, in accordance with accepted academic practice. No use, distribution or reproduction is permitted which does not comply with these terms.
*Correspondence: Robert Fernández-Vilert, ci5mdmlsZXJ0QGdtYWlsLmNvbQ==; Vanessa Arranz, di5hcnJhbnpAdWIuZWR1
†These authors have contributed equally to this work and share first authorship