- 1Akvaplan-niva, Tromsø, Norway
- 2The Scottish Association for Marine Science (SAMS), The University of the Highlands and Islands (UHI) Oban, Argyll, United Kingdom
- 3Department of Arctic Technology, University Centre in Svalbard, Longyearbyen, Norway
This mini-review outlines major climate-change exacerbated sources of metal to the Arctic marine environment, leading to measured concentrations sometimes exceeding levels considered environmentally safe, and thus potentially impacting arctic marine zooplankton. We review the bioavailability of metals in Arctic marine environments and the current state of knowledge on metal toxicity in marine copepods. Toxicity response mechanisms to metals included oxidative stress as well as genetic processes of DNA damage and repair. We highlight species-specific differences in metal impacts within the diverse group of planktonic copepods. We summarize observed responses at multiple levels of biological organization, and note that studies on arctic species are scarce and need expansion, as results from temperate and tropical species may not be readily transferable to arctic counterparts. We further provide an updated view on impacts of metals in combination with other stressors in the Arctic marine system in light of increasing attention to multiple stressors of climate change and pollution. For arctic marine zooplankton, a number of research gaps are identified, including a need for integrating effects responses across levels of biological organization, for studies into mechanisms of heritable changes and long-term transgenerational impacts, and considering interspecific capacity for response and adaptation.
Introduction
The Arctic is strongly affected by global warming, resulting in altered environmental processes both on land and at sea that affect marine ecosystems and communities. Rising temperatures can impact the dynamics of metals in the arctic region, with subsequent consequences of their elevated availability for marine species. Metal transport rates to arctic coastal waters via riverine runoff are intensified by thawing permafrost, coastal erosion, and melting glaciers, while local human activities and long-range transport from distant anthropogenic sources add to these inputs. Metals can surpass environmental quality standards and toxicity levels under changing physical environments (e.g. warming), and some are toxic even at low concentrations (e.g. mercury, lead), posing risks for environmental and human health (Elnabi et al., 2023). Estimates of toxicity are largely based on studies of species with boreal, temperate, or tropical distributions, while examples from polar regions are scarce. Additionally, there is the need to integrate the knowledge on effects across different stressors and contaminants, providing a multi-stressor assessment of risk in exposed communities (Dinh et al., 2023; Orr et al., 2024).
Zooplankton are a diverse functional group of marine invertebrates exposed to multiple terrestrial contamination sources as well as large scale changing water conditions in the Arctic. Here, we summarize current knowledge on availability of metals for arctic marine zooplankton, examine species-specific metal toxicity in selected planktonic species, and summarize known ecological, physiological, and genetic responses of zooplankton to metal contamination. In addition, we examine the state of knowledge on their capacity to cope with additional stressors, such as climate change-related elevated temperature and ocean acidification, and chemical pollution, to highlight important knowledge gaps for combined and interactive effects of metal toxicity in a multiple stress context.
Metal sources to Arctic coastal marine systems
Metals occur naturally in the arctic environment as constituents of weathering bedrock, rock eroded by glaciers, tundra soil, and riverine runoff to coastal waters and marine sediments (Lu et al., 2013; Zaborska et al., 2017). Background levels of metals can be supplemented with metals transported by e.g. volcanic eruptions (Edmonds et al., 2022), and human activities can also add to the metal inventory, resulting in local variations in sediment and water concentrations. Metals from anthropogenic sources enter the Arctic Ocean from all around the Arctic, including Baffin Island (Zdanowicz et al., 2013), Greenland (Søndergaard et al., 2015), Svalbard (Zaborska, 2017; Zaborska et al., 2017, 2020), Alaska (Schuster et al., 2018; Perryman et al., 2020), and Siberia (Lim et al., 2020). Metals reach the Arctic marine environment through either autochthonous sources (locally derived) or allochthonous sources (long range transport pathways) (Figure 1). Climate-impacted changes in transport pathways include increased rates of permafrost thawing and coastal erosion (Kim et al., 2020; Nicu et al., 2021), glacial melt (Zaborska et al., 2017), and increased industrial and human activity (mining, aquaculture, shipping, tourism) enabled by retreating seasonal sea ice (Lasserre and Faury, 2019; Brockington, 2020; Emenike et al., 2022) (Figure 1).
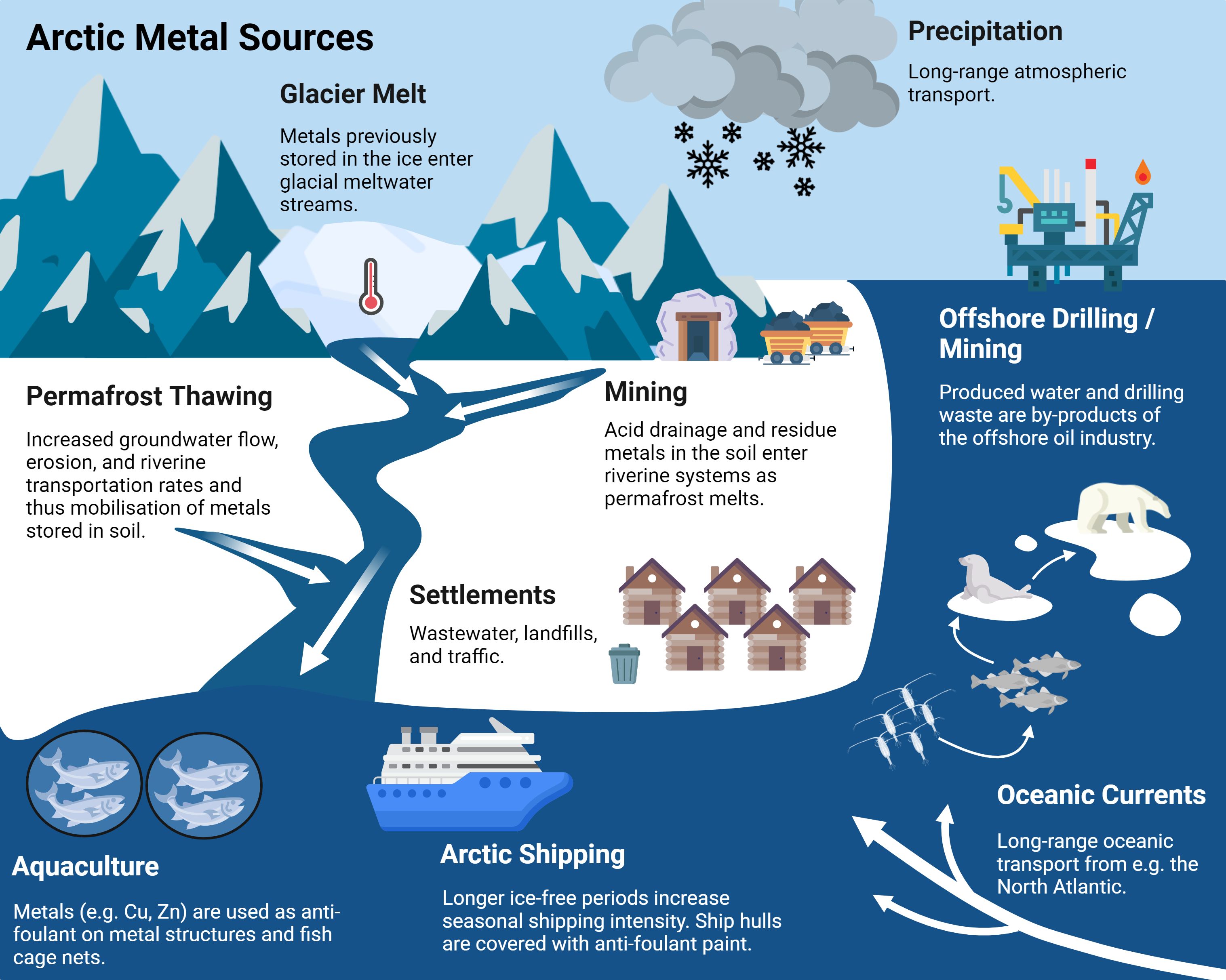
Figure 1. Major sources of metals to the Arctic marine ecosystem. White arrows depict movement through the environmental compartments. Created with BioRender.com.
Bioavailability of metals in arctic marine environments
Combined understanding of metal concentrations, availability, and toxicity is required to assess overall environmental risk. The degree to which metals present in the environment are available for interactions with organisms, i.e. their bioavailability, depends on their accessibility, lability, chemical activity, and metal absorption, uptake, and regulation mechanisms of organisms (Grotti et al., 2013). In water, free metal ions exist in equilibrium with metal ions complexed with inorganic or organic ligands, such as carbonate, chloride, humic and fulvic acids, and chelators, or adsorbed on particulates (Sigg and Xue, 1994). Metal concentrations are classified according to environmental quality standards and thresholds set by national and international organisations (e.g. the European Union through their Environmental Quality Standards EQS and the United States Environmental Protection Agency USEPA). These are selected according to the level of risk to the environment and biota, with risk defined according to standardised metrics such as the median concentration eliciting a 50% mortality response (LC50), or the concentration eliciting no detected effects (NEC).
The form in which metals are present determines their bioavailability to biota and thus their toxicity. Dissolved metals are subject to currents and vertical oceanographic processes within the water column and are more readily bioavailable for uptake in pelagic species than metals bound in sediments (Hansson et al., 2020). A high ratio of water-dissolved to sediment-bound particulates indicates overall increased risk of negative impact on marine biota (Zaborska et al., 2017, 2020). Elevated dissolved metal concentrations above background levels have been reported from Arctic fjords, with multiple sediment measurements exceeding safe environmental thresholds in fjords in Svalbard (Grotti et al., 2013; Lu et al., 2013; Zaborska et al., 2017), the Beaufort Sea (Sweeney and Naidu, 1989; Trefry et al., 2013), the Chukchi Sea (Lu and Kang, 2018), the Bering Strait (Lu and Kang, 2018), and the Barents Sea (Zaborska et al., 2017). In Hornsund, Svalbard, reported dissolved cadmium and copper concentrations of up to 4.99 and 6.28 µg/L, respectively, surpass the US EPA maximum concentration for acute exposure; resident Svalbard marine biota are thus at risk of metal toxicity (Zaborska et al., 2020).
Metal toxicity in arctic marine zooplankton
Not all metals are toxic and some are essential, such as iron (essential in blood) and magnesium (chlorophyll) (Soetan et al., 2010). They can be toxic in high concentrations or when in a certain chemical form, e.g. as free ions, soluble compounds or organometallic molecules (George, 2018)), whilst others such as lead, cadmium, and mercury are harmful at all concentrations (Ansari et al., 2004). In seawater, most metals are complexed with organic ligands; organic matter interacts with metal molecules and the resulting complexes determine both bioavailability, uptake, and toxic effects on marine animals (Ansari et al., 2004). Toxicity of metals varies 3-4 orders of magnitude governed by seawater residence times (Dong et al., 2015). The degree of toxicity varies both among metals and between species (Ansari et al., 2004), described through quantification of standardized LC50´s to provide a basis for risk assessments across contaminant groups and impacted environments.
Zooplankton are a useful bioindicator group for understanding impacts of metals in marine systems (Battuello et al., 2016; Bat et al., 2016). A review of toxicity effects of metals on marine invertebrates (Chiarelli and Roccheri, 2014) suggests meroplanktonic sea urchin larvae are sensitive bioindicators, and zooplankton species diversity can change along gradients of types and concentrations of metals (Cardoso et al., 2013). Copepods are the most abundant and widely distributed zooplankton group, and published LC50 values for copepods exposed to metals are compiled in Table 1. LC50 values for copepods can vary immensely depending on metal, species, and biogeography. The temperate copepod A. tonsa showed no significant change in mortality when exposed to copper but considerable reduction in reproductive rates (Bielmeyer et al., 2006). Data for arctic species are virtually absent, except for one study that included C. glacialis exposed to mercury, highlighting an urgent knowledge gap for Arctic taxa.
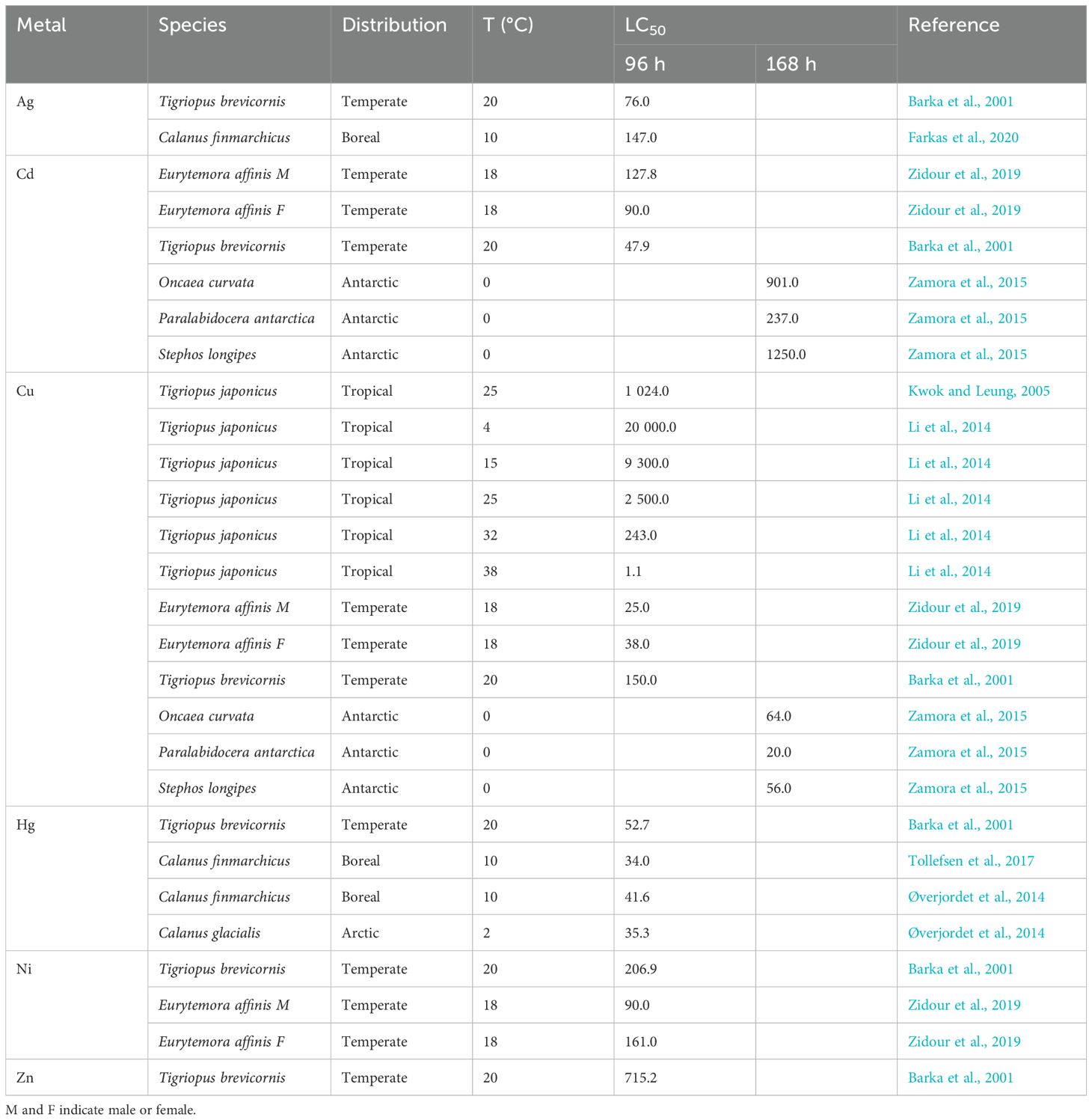
Table 1. Lethal concentrations (LC50s) for marine copepods from various climates exposed to metals (µg/L) for 4 (96 hours) or 7 days (168 hours) at a given temperature (T).
Toxicity response mechanisms to metals
How organisms respond to toxic exposure to metals can differ across species and metals, with differences according to the level of biological effects measured. Biological effects can be scaled by factors of time and concentration, and can be ordered according to the continuum from lethal to sublethal effects (Van Leeuwen, 1995), with high concentrations leading to mortality and lesser exposure conditions affecting lower levels of biological organisation such as reproduction, development, behaviour, physiology, molecular processes, and genetic integrity and regulation (Schuijt et al., 2021). In zooplankton, exposure to copper can lead to increased mortality (Dinh et al., 2021), reduced developmental rates (Kwok et al., 2008), maturation delays (Lode et al., 2018), and reduced clutch size and hatching success (Dinh et al., 2021). Egg (embryo) quality is lowered when exposed to high copper concentrations, caused by downregulation in the vitellogenin gene (Ki et al., 2009; Dinh et al., 2021), a primary yolk protein that is a vital nutrient for embryonic copepods (Lauritano et al., 2021). Some copepod females have been reported to abort their egg sacs when exposed to high concentrations of copper (Biandolino et al., 2018), which may exacerbate reproductive failure. Mercury has been shown to inactivate cellular enzymes in C. finmarchicus, with overexcitation of neurotoxicity receptors and uncoupling of oxidative phosphorylation (Tollefsen et al., 2017). Upregulation of genes from the GST family typically involved in general stress responses, detoxification, and defence against oxidative stress (Hayes et al., 2005) has been observed in many copepod species following acute sublethal metal exposure (Lee et al., 2008; Ki et al., 2009; Wang and Wang, 2009; Øverjordet et al., 2014; Farkas et al., 2020); however, the regulatory mechanism behind the induction of GST in different copepod species is not well understood. Detoxification and stress response systems differ between species and organism groups; therefore, effects are the net result from a combination of toxic impact as well as response. Inclusion of mechanistic toxicity and response effects in experimental zooplankton studies could shed light on why extent and impacts of exposure can differ between species, metals, and exposure situations, as previously shown for sea urchin larvae (Reinardy and Bodnar, 2015).
Dissolved metals have been linked to structural damage of DNA, lipids, enzymes, and proteins through enhanced intracellular production of reactive oxygen species (ROS), and resulting oxidative stress (e.g. Reinardy et al., 2013). Genome integrity is fundamental for maintaining cellular, physiological, and organismal functions, and genetic damage from exposure to environmental metal contaminants can disrupt all levels of biological functions (Reinardy and Bodnar, 2015). The mechanism by which individual effects link to longer-term population and even species-level impacts is through heritable changes in either the genome or epigenome: changes can be beneficial for adaptation or negative through inherited genome instability. DNA repair is the main mechanism to mitigate damaged DNA, and DNA repair pathways are largely conserved across a wide range of phyla, reflecting the fundamental importance of maintaining genome integrity (El-Bibany et al., 2014). Zooplankton are susceptible to DNA damage from genotoxic exposure (Halsband et al., 2021); however, studies on DNA repair in zooplankton are limited (Reinardy and Bodnar, 2015; Bailey et al., 2017; Park et al., 2023). This approach of comparative susceptibility and toxicity response is of importance when considering realistic, complex, and fluctuating changing environmental conditions and presence of contaminants, and should be further developed for ecologically essential zooplankton.
There is evidence for metal toxicity through oxidative stress response and genotoxicity in zooplankton. Copper can induce oxidative stress, antioxidant defence systems, and genotoxicity in copepods. Copper caused oxidative stress in harpacticoid T. japonicus indicated by significant upregulation of the catalase gene (Rhee et al., 2013) and reduced the body load of the antioxidant astaxanthin (Weaver et al., 2016). DNA damage, hypothesized through DNA cross-links, measured in C. finmarchicus and Acartia longiremis exposed to 20 µg/L copper was correlated with induction of oxidative stress genes and suggested that the mechanism of toxicity leading to mortality was a ROS-induced oxidative stress DNA damage response (Thomsen et al., in review1). However, lethal responses in Temora longicornis, T. brevicornis, and Acartia tonsa exposed to 6 and 60 µg/L copper were not linked to detected DNA damage as measured by the DNA strand break comet assay (Sahlmann et al., 2019). The mechanisms of sublethal toxicity from copper, and the link to different forms and levels of DNA damage, are therefore still unclear. The arctic congener C. glacialis more rapidly induced transcription of GST when exposed to mercury compared with boreal C. finmarchicus, and the species differences likely do not lie in metabolic differences as both species were kept at their metabolic optimum (Øverjordet et al., 2014). This result would be in line with the hypothesis that toxicity should decrease from the tropics to the poles (Chapman and Riddle, 2005). Arctic copepods could be more resilient to metal contamination than their boreal and tropical counterparts, possibly due to rapid and effective initiation of antioxidant and stress response systems (Øverjordet et al., 2014). Energy reserves in the form of storage lipids are an important component of Arctic copepod survival (Falk-Petersen et al., 2009) and may play an alleviating role in metal toxicity. Phenotypic plasticity (Sasaki and Dam, 2021) and cellular and genetic stress response variability (Collins et al., 2023) may also underpin inter- and intra-specific variability in zooplankton stress responses, but are little studied in relation to metal contamination.
Multistressor metal toxicity
There is growing attention on combined stressor impacts driven by the need for more ecologically relevant toxicity scenarios which better match the stressor profiles in environments and ecosystems (Collins et al., 2023; Dinh et al., 2023; Orr et al., 2024). Metal toxicity can be altered depending on the trajectory of climate change, especially temperature and pH, and presence of other pollutants. The solubility of metals in water increases as temperature rises, enhancing their bioavailability (Banaee et al., 2024). Elevated temperatures can increase metal toxicity in marine zooplankton (Kwok and Leung, 2005; Bai and Wang, 2020) primarily due to higher rates of uptake, increased oxidative stress, and decreased rates of intracellular detoxification processes (Sokolova and Lannig, 2008; Dinh et al., 2020). Thermal tolerance ranges of species narrow with increasing latitude (Sasaki and Dam, 2021), and species inhabiting regions at the extreme ends of their thermal tolerance are more susceptible to metal toxicity (Heuschele et al., 2022). In the tropical copepod Pseudodiaptomus incisus, survival, reproduction, grazing habits, and body size were negatively affected by individual exposures to copper and elevated temperatures, and impacts were exacerbated when stressors were combined (Dinh et al., 2021). An additive interaction was also evident in a lower 96-h LC50 for copper with increasing temperatures in T. japonicus (Li et al., 2014, Table 1). Elevated temperature increases energetic demands and inactivates cellular enzymes (Rhee et al., 2009) which might especially hold true for Arctic copepods not adapted to large variations in their thermal range. Additionally, increased tolerance against copper pollution has been observed in tropical copepods after entering a dormant phase under extremely low temperature conditions (Kwok and Leung, 2005; Li et al., 2014), possibly due to improved energy supplies at the start of dormancy (Raisuddin et al., 2007). Li et al. (2014) suggested that a dormant phase lessens copper toxicity as copepods accumulate less metals due to decreased metabolism. Thomsen et al. (in review)1 observed higher DNA damage levels from copper under elevated temperature in C. finmarchicus compared with the smaller A. longiremis, possibly due to differences in energy demands for different body sizes and thermal regimes of the two species. How trade-offs in energy demands, thermal tolerance ranges, and subsequent altered capacity for mounting detoxification and response mechanisms impact arctic zooplankton undergoing diapause is not well understood especially in extreme Arctic winters (Dinh et al., 2023).
In addition to elevated temperatures, metal contamination will often be accompanied by other climate stressors (ocean acidification, altered salinity or oxygen profiles), toxic chemicals, or biological factors (predators, parasites, health status) which can alter the metal exposure effects. Presence of additional contaminants such as persistent organic pollutants, polycyclic aromatic hydrocarbons from oil contamination (Hansen et al., 2011), or leaching chemicals (anti-fouling agents, plastic litter additives), can combine for a complex ‘cocktail’ of stressors (Esbaugh et al., 2018). Combined stressors may not only have negative effects, but also contribute to increased resilience through seesaw effects, cross-tolerance, and memory effects (Zhou and Wang, 2023). Integration of positive and negative effects from multiple stressors may be assisted by modelling frameworks able to quantify synergies between the effects at ecosystem level (Bailey and van der Grient, 2020).
Transgenerational effect studies consider impacts on offspring from exposed parental generations. A combined copper and elevated temperature exposure of the tropical Pseudodiaptomus annandalei caused reduced parent survival and nauplii production, and increased metabolism indicated by higher faecal pellet production; additionally, females showed higher mortality than males with the suggestion that the higher food demands from reproduction fuelled higher metal intake (Dinh et al., 2020). A similar combination of metal and elevated temperature exposure of P. incises demonstrated negative fertility and fecundity, and reduced overall fitness in offspring, which suggests that exposure effects can cascade through multiple generations with possible long-term population and food web impacts (Dinh et al., 2021).
Research gaps
Polar species sensitivity
The duration of exposure to toxic metals might greatly affect interpretation of ecological and long-term impacts in the Arctic due to lower rates of metabolism and greater longevity in Arctic species. Contamination events with metal concentrations above legislation limits might not trigger effects in Arctic copepods as they might take longer to react due to their slower metabolism (Chapman and Riddle, 2005; Hansen et al., 2011; Zamora et al., 2015). Pulse exposure events have been investigated in temperate species (Bielmeyer et al., 2006; Hook and Fisher, 2001) but similar studies are missing for polar species. Standard 96-h acute exposure experiments generally used for toxicity testing in copepods from lower latitudes do not necessarily hold true for polar regions where stress responses to cadmium and copper have only been observed after 7 days in three Antarctic copepod species (Zamora et al., 2015; Table 1). The multitude of metal sources in the Arctic region presents an opportunity to target hotspots of multi-stressor and metal exposure as well as stable and less-exposed control sites for closely linked comparative toxicity responses, including long-term toxicity effects in Arctic populations and species.
Intra- and inter-specific toxicity differences
The interspecific differences in metal toxicity reviewed above demonstrate that more acute stress response studies on polar species are required. The overwhelming majority of studies focused on copepods, while other planktonic taxa have been rarely considered. Copper can be more toxic than cadmium in Antarctic (Zamora et al., 2015) and some temperate copepods (Verriopoulos and Moraitou-Apostolopoulou, 1982; Hutchinson et al., 1994; Zidour et al., 2019), while the opposite is true for the temperate T. brevicornis (Barka et al., 2001). Larval, pre-adult, and adult life stages can also differ in their sensitivity and capacity to respond. In addition, regional and local adaptive variations may cause intra-specific plasticity, resulting in wide ranges of LC50’s across different populations. Different metal toxicities among closely related species highlight the need for further understanding of underlying inter-specific mechanistic differences in stressor response (Rocha-Olivares et al., 2004). Phenotypic plasticity of populations experiencing different environmental conditions and resulting cellular and molecular stress response variability require further study to disentangle inter- and intra-specific variability in zooplankton stress responses.
Genotoxic and molecular control of toxicity response
While there might be no phenotypic difference between observed LC50´s and metabolic rates for any given stressor between two different copepod species, their genetic responses may vary (Øverjordet et al., 2014; Thirunavukkarasu and Hwang, 2024). If Arctic populations and food chain dynamics are more sensitive to increasing metal concentrations might be answered by comparing genotoxic responses of Arctic copepods to those of congeneric temperate or tropical counterparts. Genetic responses are, however, not as straightforward to observe as morphological changes or metabolism rates, where results can be drawn from observations of food intake and respiration rates. Differences in levels and types of sublethal genotoxicity of copper in A. longiremis and Calanus spp. indicate species-specific differences in genetic sensitivity, DNA repair responses, and downstream control of reproduction (Thomsen et al., in review1). Individual survival and reproduction are ultimately controlled by complex genetic and molecular responses, and the fundamental mechanisms for effects beyond the individual level is through inheritable genetic and epigenetic pathways, yet studies on these pathways in Arctic zooplankton require much more expansion.
Conclusions
The review demonstrates that metal concentrations are increasing due to accelerated transport from natural and anthropogenic sources into coastal arctic systems. Hotspots with concentrations exceeding environmental quality standards pose an increasing risk in the Arctic, with implications for food web structure and function. Bioavailability of metals in Arctic marine environments is evident from some studies, however more data is required across different Arctic regions to directly assess marine concentrations in bioavailable forms to link metal sources to dissolved concentrations and uptake into zooplankton. New experimental and molecular approaches to the study of metal toxicity in a multi-stressor context can provide mechanistic understanding of a species’ resilience or vulnerability, and differential capacity for adaptive evolution in a changing Arctic Ocean. The review uncovers knowledge gaps concerning species-specific and population-wide impacts and we call for more Arctic-focused studies on zooplankton as a key group of species in the marine trophic web. The question of sensitivity versus resilience to changing conditions, including increasing metal concentrations, and multi-stressor environment is still open for most arctic species, and mechanistic studies can illuminate different response and coping strategies in different species under future ocean conditions to better predict and alleviate environmental risks from metal contamination in the Arctic.
Author contributions
CH: Writing – review & editing, Writing – original draft, Visualization, Supervision, Funding acquisition, Conceptualization. NT: Writing – review & editing, Writing – original draft, Visualization. HR: Writing – review & editing, Writing – original draft, Visualization, Supervision, Conceptualization.
Funding
The author(s) declare financial support was received for the research, authorship, and/or publication of this article. CH was supported by the Fram Centre program ‘Cumulative impact of multiple stressors in High North ecosystems – CLEAN’.
Conflict of interest
The authors declare that the research was conducted in the absence of any commercial or financial relationships that could be construed as a potential conflict of interest.
Generative AI statement
The author(s) declare that no Generative AI was used in the creation of this manuscript.
Publisher’s note
All claims expressed in this article are solely those of the authors and do not necessarily represent those of their affiliated organizations, or those of the publisher, the editors and the reviewers. Any product that may be evaluated in this article, or claim that may be made by its manufacturer, is not guaranteed or endorsed by the publisher.
Footnotes
- ^ Thomsen, N., Halsband, C., Hopland-Sperre, K., and Reinardy, H. C. Multi-stress effects of copper and elevated temperature in arctic calanoid copepods.
References
Ansari T. M., Marr I. L., Tariq N. (2004). Heavy metals in marine pollution perspective–A mini review. J. Appl. Sci. 4, 1–20. doi: 0.3923/jas.2004.1.20
Bai Z., Wang M. (2020). Warmer temperature increases mercury toxicity in a marine copepod. Ecotoxicol. Environ. Safe. 201, 110861. doi: 10.1016/j.ecoenv.2020.110861
Bailey A., De Wit P., Thor P., Browman H. I., Bjelland R., Shema S., et al. (2017). Regulation of gene expression is associated with tolerance of the Arctic copepod Calanus glacialis to CO2-acidified sea water. Ecol. Evol. 7, 7145–7160. doi: 10.1002/ece3.3063
Bailey R. M., van der Grient J. M. A. (2020). OSIRIS: A model for integrating the effects of multiple stressors on marine ecosystems. J. Theor. Bio. 493, 110211. doi: 10.1016/j.jtbi.2020.110211
Banaee M., Zeidi A., Mikušková N., Faggio C. (2024). Assessing metal toxicity on crustaceans in aquatic ecosystems: a comprehensive review. Biol. Trace Elem. Res. 202, 5743–5761. doi: 10.1007/s12011-024-04122-7
Barka S., Pavillon J.-F., Amiard J.-C. (2001). Influence of different essential and non-essential metals on MTLP levels in the Copepod Tigriopus brevicornis. Comp. Biochem. Phys. C 128, 479–493. doi: 10.1016/S1532-0456(00)00198-8
Bat L., Üstün F., Öztekin H. C. (2016). Heavy metal concentrations in zooplankton of Sinop coasts of the Black Sea, Turkey. Mar. Biol. J. 1, 5–13. doi: 10.21072/mbj.2016.01.1.01
Battuello M., Brizio P., Sartor R. M., Nurra N., Pessani D., Abete M. C., et al. (2016). Zooplankton from a northwestern Mediterranean area as a model of metal transfer in a marine environment. Ecol. Indic. 66, 440–451. doi: 10.1016/j.ecolind.2016.02.018
Biandolino F., Parlapiano I., Faraponova O., Prato E. (2018). Effects of short-and long-term exposures to copper on lethal and reproductive endpoints of the harpacticoid copepod Tigriopus fulvus. Ecotox. Environ. Safe 147, 327–333. doi: 10.1016/j.ecoenv.2017.08.041
Bielmeyer G. K., Grosell M., Brix K. V. (2006). Toxicity of silver, zinc, copper, and nickel to the copepod Acartia tonsa exposed via a phytoplankton diet. Environ. Sci. Technol. 40, 2063–2068. doi: 10.1021/es051589a
Brockington M. (2020). Offshore development contamination in Canada’s arctic: A threat to food quality and safety? Glob. Health: Annu. Rev. 1, 3–3.
Cardoso P. G., Marques S. C., D’Ambrosio M., Pereira E., Duarte A. C., Azeiteiro U. M., et al. (2013). Changes in zooplankton communities along a mercury contamination gradient in a coastal lagoon (Ria de Aveiro, Portugal). Mar. Pollut. Bull. 76, 170–177. doi: 10.1016/j.marpolbul.2013.09.007
Chapman P. M., Riddle M. J. (2005). Polar marine toxicology - Future research needs. Mar. pollut. Bull. 50, 905–908. doi: 10.1016/j.marpolbul.2005.06.001
Chiarelli R., Roccheri M. C. (2014). Marine invertebrates as bioindicators of heavy metal pollution. Open J. Met. 4, 93–106. doi: 10.4236/ojmetal.2014.44011
Collins M., Clark M. S., Truebano M. (2023). The environmental cellular stress response: the intertidal as a multistressor model. Cell Stress Chaperon. 28, 467–475. doi: 10.1007/s12192-023-01348-7
Dinh K. V., Albini D., Orr J. A., Macauley S. J., Rillig M., Borgå K., et al. (2023). Winter is coming: interactions of multiple stressors in winter and implications for the natural world. Glob. Change Biol. 29, 6834–6845. doi: 10.1111/gcb.16956
Dinh K. V., Doan K. L. U., Doan N. X., Pham H. Q., Le T. H. O., Le M. H., et al. (2021). Parental exposures increase the vulnerability of copepod offspring to copper and a simulated marine heatwave. Environ. pollut. 287, 117603. doi: 10.1016/j.envpol.2021.117603
Dinh K. V., Nguyen Q. T. T., Vo T. M. C., Bui T. B., Dao T. S., Tran D. M., et al. (2020). Interactive effects of extreme temperature and a widespread coastal metal contaminant reduce the fitness of a common tropical copepod across generations. Mar. pollut. Bull. 159, 111509. doi: 10.1016/j.marpolbul.2020.111509
Dong Y., Rosenbaum R. K., Hauschild M. Z. (2015). Assessment of metal toxicity in marine ecosystems: comparative toxicity potentials for nine cationic metals in coastal seawater. Environ. Sci. Technol. 50, 269–278. doi: 10.1021/acs.est.5b01625
Edmonds M., Mason E., Hogg O. (2022). Volcanic outgassing of volatile trace metals. Annu. Rev. Earth Pl. Sc. 50, 79–98. doi: 10.1146/annurev-earth-070921-062047
El-Bibany A. H., Bodnar A. G., Reinardy H. C. (2014). Comparative DNA damage and repair in echinoderm coelomocytes exposed to genotoxicants. PloS One 9, e107815. doi: 10.1371/journal.pone.0107815
Elnabi M. K., Elkaliny N. E., Elyazied M. M., Azab S. H., Elkhalifa S. A., Elmasry S., et al. (2023). Toxicity of heavy metals and recent advances in their removal: a review. Toxics. 11, 580. doi: 10.3390/toxics11070580
Emenike E. C., Iwuozor K. O., Anidiobi S. U. (2022). Heavy metal pollution in aquaculture: sources, impacts and mitigation techniques. Biol. Trace Elem. Res. 200, 4476–4492. doi: 10.1007/s12011-021-03037-x
Esbaugh A. J., Khursigara A., Johansen J. (2018). “Toxicity in aquatic environments: the cocktail effect,” in Development and environment. Eds. Burggren W., Dubansky B. (Springer, Cham), 203–234. doi: 10.1007/978-3-319-75935-7_9
Falk-Petersen S., Mayzaud P., Kattner G., Sargent J. R. (2009). Lipids and life strategy of Arctic Calanus. Mar. Biol. Res. 5, 18–39. doi: 10.1080/17451000802512267
Farkas J., Cappadona V., Olsen A. J., Hansen B. H., Posch W., Ciesielski T. M., et al. (2020). Combined effects of exposure to engineered silver nanoparticles and the water-soluble fraction of crude oil in the marine copepod Calanus finmarchicus. Aquat. Toxicol. 227, 105582. doi: 10.1016/j.aquatox.2020.105582
George S. G. (2018). “Biochemical and cytological assessments of metal toxicity in marine animals,” in Heavy metals in the marine environment (Boca Raton: CRC Press), 123–142. doi: 10.1201/9781351073158-8
Grotti M., Soggia F., Ianni C., Magi E., Udisti R. (2013). Bioavailability of trace elements in surface sediments from Kongsfjorden, Svalbard. Mar. pollut. Bull. 77, 367–374. doi: 10.1016/j.marpolbul.2013.10.010
Halsband C., Dix M. F., Sperre K. H., Reinardy H. C. (2021). Reduced pH increases mortality and genotoxicity in an Arctic coastal copepod, Acartia longiremis. Aquat. Toxicol. 239, 105961. doi: 10.1016/j.aquatox.2021.105961
Hansen B. H., Altin D., Rørvik S. F., Øverjordet I. B., Olsen A. J., Nordtug T. (2011). Comparative study on acute effects of water accommodated fractions of an artificially weathered crude oil on Calanus finmarchicus and Calanus glacialis (Crustacea: Copepoda). Sci. Total Environ. 409, 704–709. doi: 10.1016/j.scitotenv.2010.10.035
Hansson S. V., Desforges J. P., van Beest F. M., Bach L., Halden N. M., Sonne C., et al. (2020). Bioaccumulation of mining derived metals in blood, liver, muscle and otoliths of two Arctic predatory fish species (Gadus ogac and Myoxocephalus scorpius). Environ. Res. 183, 109194. doi: 10.1016/J.ENVRES.2020.109194
Hayes J. D., Flanagan J. U., Jowsey I. R. (2005). Glutathione transferases. Annu. Rev. Pharmacol. 45, 51–88. doi: 10.1146/annurev.pharmtox.45.120403.095857
Heuschele J., Lode T., Konestabo H. S., Titelman J., Andersen T., Borgå K. (2022). Drivers of copper sensitivity in copepods: A meta-analysis of LC50s. Ecotox. Environ. Safe. 242, 113907. doi: 10.1016/j.ecoenv.2022.113907
Hook S. E., Fisher N. S. (2001). Sublethal effects of silver in zooplankton: Importance of exposure pathways and implications for toxicity testing. Environ. Toxicol. Chem. 20, 568–574. doi: 10.1002/etc.5620200316
Hutchinson T. H., Williams T. D., Eales G. J. (1994). Toxicity of cadmium, hexavalent chromium and copper to marine fish larvae (Cyprinodon variegatus) and copepods (Tisbe battagliai). Mar. Environ. Res. 38, 275–290. doi: 10.1016/0141-1136(94)90028-0
Ki J. S., Raisuddin S., Lee K. W., Hwang D. S., Han J., Rhee J. S., et al. (2009). Gene expression profiling of copper-induced responses in the intertidal copepod Tigriopus japonicus using a 6K oligochip microarray. Aquat. Toxicol. 93, 177–187. doi: 10.1016/j.aquatox.2009.04.004
Kim H., Kwon S. Y., Lee K., Lim D., Han S., Kim T. W., et al. (2020). Input of terrestrial organic matter linked to deglaciation increased mercury transport to the Svalbard fjords. Sci. Rep. 10, 3446. doi: 10.1038/s41598-020-60261-6
Kwok K. W. H., Leung K. M. Y. (2005). Toxicity of antifouling biocides to the intertidal harpacticoid copepod Tigriopus japonicus (Crustacea, Copepoda): Effects of temperature and salinity. Mar. pollut. Bull. 51, 830–837. doi: 10.1016/j.marpolbul.2005.02.036
Kwok K. W. H., Leung K. M. Y., Bao V. W. W., Lee J.-S. (2008). Copper toxicity in the marine copepod Tigropus japonicus: Low variability and high reproducibility of repeated acute and life-cycle tests. Mar. pollut. Bull. 57, 632–636. doi: 10.1016/j.marpolbul.2008.03.026
Lasserre F., Faury O. (2019). Arctic shipping: Climate change, commercial traffic and port development (Routledge). Available at: https://www.routledge.com/Arctic-Shipping-Climate-Change-Commercial-Traffic-and-Port-Development/Lasserre-Faury/p/book/9780367777609?gad_source=1&gclid=EAIaIQobChMI6MWNre73iQMVhZNQBh2jeg11EAAYASAAEgJETvD_BwE
Lauritano C., Carotenuto Y., Roncalli V. (2021). Glutathione s-transferases in marine copepods. J. Mar. Sci. Eng. 9, 1025. doi: 10.3390/jmse9091025
Lee K. W., Raisuddin S., Rhee J. S., Hwang D. S., Yu I. T., Lee Y. M., et al. (2008). Expression of glutathione S-transferase (GST) genes in the marine copepod Tigriopus japonicus exposed to trace metals. Aquat. Toxicol. 89, 158–166. doi: 10.1016/j.aquatox.2008.06.011
Li A. J., Leung P. T. Y., Bao V. W. W., Yi A. X. L., Leung K. M. Y. (2014). Temperature-dependent toxicities of four common chemical pollutants to the marine medaka fish, copepod and rotifer. Ecotoxicology. 23, 1564–1573. doi: 10.1007/s10646-014-1297-4
Lim A. G., Jiskra M., Sonke J. E., Loiko S. V., Kosykh N., Pokrovsky O. S. (2020). A revised pan-Arctic permafrost soil Hg pool based on Western Siberian peat Hg and carbon observations. Biogeosciences. 17, 3083–3097. doi: 10.5194/bg-17-3083-2020
Lode T., Heuschele J., Andersen T., Titelman J., Hylland K., Borgå K. (2018). Predation risk potentiates toxicity of a common metal contaminant in a coastal copepod. Environ. Sci. Technol. 52, 13535–13542. doi: 10.1021/acs.est.8b03685
Lu Z., Cai M., Wang J., Yin Z., Yang H. (2013). Levels and distribution of trace metals in surface sediments from Kongsfjorden, Svalbard, Norwegian Arctic. Environ. Geochem. Health 35, 257–269. doi: 10.1007/s10653-012-9481-z
Lu Z. B., Kang M. (2018). Risk assessment of toxic metals in marine sediments from the Arctic Ocean using a modified BCR sequential extraction procedure. J. Environ. Sci. Heal. A. 53, 278–293. doi: 10.1080/10934529.2017.1397443
Nicu I. C., Lombardo L., Rubensdotter L. (2021). Preliminary assessment of thaw slump hazard to Arctic cultural heritage in Nordenskiöld Land, Svalbard. Landslides. 18, 2935–2947. doi: 10.1007/s10346-021-01684-8
Orr J. A., Macauley S. J., Mordente A., Burgess B., Albini D., Hunn J. G., et al. (2024). Studying interactions among anthropogenic stressors in freshwater ecosystems: a systemic review of 2396 multiple-stressor experiments. Ecol. Lett. 27, e14463. doi: 10.1111/ele.14463
Øverjordet I. B., Altin D., Berg T., Jenssen B. M., Gabrielsen G. W., Hansen B. H. (2014). Acute and sub-lethal response to mercury in Arctic and boreal calanoid copepods. Aquat. Toxicol. 155, 160–165. doi: 10.1016/j.aquatox.2014.06.019
Park Y., Park J. G., Kang H. M., Jung J. H., Kim M., Lee K. W. (2023). Toxic effects of the wastewater produced by underwater hull cleaning equipment on the copepod Tigriopus japonicus. Mar. pollut. Bull. 191, 114991. doi: 10.1016/j.marpolbul.2023.114991
Perryman C. R., Wirsing J., Bennett K. A., Brennick O., Perry A. L., Williamson N., et al. (2020). Heavy metals in the Arctic: Distribution and enrichment of five metals in Alaskan soils. PloS One 15, e0233297. doi: 10.1371/journal.pone.0233297
Raisuddin S., Kwok K. W. H., Leung K. M. Y., Schlenk D., Lee J. S. (2007). The copepod Tigriopus: a promising marine model organism for ecotoxicology and environmental genomics. Aquat. Toxicol. 83, 161–173. doi: 10.1016/j.aquatox.2007.04.005
Reinardy H. C., Syrett J. R., Jeffree R. A., Henry T. B., Jha A. N. (2013). Cobalt-induced genotoxicity in male zebrafish (Danio rerio), with implications for reproduction and expression of DNA repair genes. Aquat Toxicol. 126, 224–230. doi: 10.1016/j.aquatox.2012.11.007
Reinardy H. C., Bodnar A. G. (2015). Profiling DNA damage and repair capacity in sea urchin coelomocytes and larvae exposure to genotoxicants. Mutagenesis. 30, 829–839. doi: 10.1093/mutage/gev052
Rhee J. S., Raisuddin S., Lee K. W., Seo J. S., Ki J. S., Kim I. C., et al. (2009). Heat shock protein (Hsp) gene responses of the intertidal copepod Tigriopus japonicus to environmental toxicants. Comp. Biochem. Phys. C. 149, 104–112. doi: 10.1016/j.cbpc.2008.07.009
Rhee J. S., Yu I. T., Kim B. M., Jeong C. B., Lee K. W., Kim M. J., et al. (2013). Copper induces apoptotic cell death through reactive oxygen species-triggered oxidative stress in the intertidal copepod Tigriopus japonicus. Aquat. Toxicol. 132, 182–189. doi: 10.1016/j.aquatox.2013.02.013
Rocha-Olivares A., Fleeger J. W., Foltz D. W. (2004). Differential tolerance among cryptic species: A potential cause of pollutant-related reductions in genetic diversity. Environ. Toxicol. Chem. 23, 2132–2137. doi: 10.1897/03-512
Sahlmann A., Lode T., Heuschele J., Borgå K., Titelman J., Hylland K. (2019). Genotoxic response and mortality in 3 marine copepods exposed to waterborne copper. Environ. Toxicol. Chem. 38, 2224–2232. doi: 10.1002/etc.4541
Sasaki M., Dam H. G. (2021). Global patterns in copepod thermal tolerance. J. Plankton Res. 43, 598–609. doi: 10.1093/plankt/fbab044
Schuijt L. M., Peng F. J., van den Berg S. J. P., Dingemans M. M. L., Van den Brink P. J. (2021). (Eco)toxicological tests or assessing impacts of chemical stress to aquatic ecosystems: Facts, challenges, and future. Sci. Total Environ. 795, 148776. doi: 10.1016/j.scitotenv.2021.148776
Schuster P. F., Schaefer K. M., Aiken G. R., Antweiler R. C., Dewild J. F., Gryziec J. D., et al. (2018). Permafrost stores a globally significant amount of mercury. Geophys. Res. Lett. 45, 1463–1471. doi: 10.1002/2017GL075571
Sigg L., Xue H. (1994). “Metal speciation: concepts, analysis and effects,” in Chemistry of aquatic systems: local and global perspectives. Eds. Bidoglio G., Stumm W. (Springer, Dordrecht), 153–181. doi: 10.1007/978-94-017-1024-4_7
Soetan K. O., Olaiya C. O., Oyewole O. E. (2010). The importance of mineral elements for humans, domestic animals and plants: A review. Afr. J. Food Sci. 4, 200–222. doi: 10.5897/AJFS.9000287
Sokolova I. M., Lannig G. (2008). Interactive effects of metal pollution and temperature on metabolism in aquatic ectotherms: implications of global climate change. Clim. Res. 37, 181–201. doi: 10.3354/cr00764
Søndergaard J., Tamstorf M., Elberling B., Larsen M. M., Mylius M. R., Lund M., et al. (2015). Mercury exports from a High-Arctic river basin in Northeast Greenland (74 N) largely controlled by glacial lake outburst floods. Sci. Total Environ. 514, 83–91. doi: 10.1016/j.scitotenv.2015.01.097
Sweeney M. D., Naidu A. S. (1989). Heavy metals in sediments of the inner shelf of the Beaufort Sea, northern arctic Alaska. Mar. pollut. Bull. 20, 140–143. doi: 10.1016/0025-326X(88)90820-X
Thirunavukkarasu S., Hwang J.-S. (2024). Genotoxic effects of marine pollutants on coastal meso-zooplankton populations – a mini review. Mar. Pollut. Bull. 205, 116548. doi: 10.1016/j.marpolbul.2024.116548
Tollefsen K. E., Song Y., Høgåsen T., Øverjordet I. B., Altin D., Hansen B. H. (2017). Mortality and transcriptional effects of inorganic mercury in the marine copepod Calanus finmarchicus. J. Toxicol. Environ. Health A. 80, 845–861. doi: 10.1080/15287394.2017.1352198
Trefry J. H., Dunton K. H., Trocine R. P., Schonberg S. V., McTigue N. D., Hersh E. S., et al. (2013). Chemical and biological assessment of two offshore drilling sites in the Alaskan Arctic. Mar. Environ. Res. 86, 35–45. doi: 10.1016/J.MARENVRES.2013.02.008
Van Leeuwen C. J. (1995). “Ecotoxicological effects,” in Risk assessments of chemicals. Eds. van Leeuwen C. J., Hermens J. L. M. (Springer, Dordrecht), 175–237. doi: 10.1007/978-94-015-8520-0_6
Verriopoulos G., Moraitou-Apostolopoulou M. (1982). Differentiation of the sensitivity to copper and cadmium in different life stages of a copepod. Mar. Pollut. Bull. 13, 123–125. doi: 10.1016/0025-326X(82)90368-X
Wang M. H., Wang G. Z. (2009). Biochemical response of the copepod Tigriopus japonicus mori experimentally exposed to cadmium. Arch. Environ. Con. Tox. 57, 707–717. doi: 10.1007/s00244-009-9319-6
Weaver R. J., Hill G. E., Kuan P. L., Tseng Y. C. (2016). Copper exposure reduces production of red carotenoids in a marine copepod. Ecol. Indic. 70, 393–400. doi: 10.1016/j.ecolind.2016.06.040
Zaborska A. (2017). Sources of 137Cs to an arctic fjord (Hornsund, svalbard). J. Environ. Radioactiv. 180, 19–26. doi: 10.1016/j.jenvrad.2017.09.021
Zaborska A., Beszczyńska-Möller A., Włodarska-Kowalczuk M. (2017). History of heavy metal accumulation in the Svalbard area: Distribution, origin and transport pathways. Environ. pollut. 231, 437–450. doi: 10.1016/j.envpol.2017.08.042
Zaborska A., Strzelewicz A., Rudnicka P., Moskalik M. (2020). Processes driving heavy metal distribution in the seawater of an Arctic fjord (Hornsund, southern Spitsbergen). Mar. Pollut. Bull. 161, 111719. doi: 10.1016/j.marpolbul.2020.111719
Zamora L., King C. K., Payne S. J., Virtue P. (2015). Sensitivity and response time of three common Antarctic marine copepods to metal exposure. Chemosphere. 120, 267–272. doi: 10.1016/j.chemosphere.2014.07.051
Zdanowicz C., Krümmel E. M., Lean D., Poulain A. J., Yumvihoze E., Chen J., et al. (2013). Accumulation, storage and release of atmospheric mercury in a glaciated Arctic catchment, Baffin Island, Canada. Geochim. Cosmochim. Ac. 107, 316–335. doi: 10.1016/j.gca.2012.11.028
Zhou L., Wang S. (2023). The bright side of ecological stressors. Trends Ecol. Evol. 38, 568–578. doi: 10.1016/j.tree.2023.01.010
Keywords: Calanus, Acartia longiremis, genotoxicity, temperature increase, LC50, multi-stress, metals
Citation: Halsband C, Thomsen N and Reinardy HC (2024) Climate Change increases the risk of metal toxicity in Arctic zooplankton. Front. Mar. Sci. 11:1510718. doi: 10.3389/fmars.2024.1510718
Received: 13 October 2024; Accepted: 18 November 2024;
Published: 05 December 2024.
Edited by:
Jean-Pierre Desforges, University of Winnipeg, CanadaReviewed by:
Levent Bat, Sinop University, TürkiyeCopyright © 2024 Halsband, Thomsen and Reinardy. This is an open-access article distributed under the terms of the Creative Commons Attribution License (CC BY). The use, distribution or reproduction in other forums is permitted, provided the original author(s) and the copyright owner(s) are credited and that the original publication in this journal is cited, in accordance with accepted academic practice. No use, distribution or reproduction is permitted which does not comply with these terms.
*Correspondence: Claudia Halsband, Y2xoQGFrdmFwbGFuLm5pdmEubm8=