- 1Aquaculture Program, Institut de Recerca i Tecnologia Agroalimentaries (IRTA), La Ràpita, Spain
- 2Mediterranean Institute for Advanced Studies (IMEDEA, CSIC-UIB), Esporles, Spain
In many meta-analyses and literature reviews on fish microbiota, the provenance of the animals (farmed vs. wild) is often overlooked. Given the well-established role of diet as a key factor in shaping gut microbiota, this study investigates the impact of dietary nature by comparing the microbiota of gilthead seabream (Sparus aurata) fed a commercial diet versus a wild-type diet, all reared within a recirculating aquaculture system. Over a 60-day period, we tracked changes in gut bacterial diversity, structure, and composition following a shift from a commercial feed to a diet exclusively based on pink shrimp (Parapenaeus longirostris). Gut bacterial communities were assessed using 16S rRNA gene sequencing (Illumina MiSeq platform) with primers targeting the V3-V4 hypervariable regions. Twenty days after the dietary change, microbial diversity (Shannon index) increased in fish fed the shrimp diet compared to those fed the commercial diet, while Dominance index values decreased. Additionally, inter-individual (beta-) diversity based on Bray-Curtis distances also differed between dietary treatments. These results support further that microbiota comparisons between farmed/captive and wild fish are challenging due to the unpredictable feeding regimes and dietary variations in wild fish. However, the diet impact on microbiota diminished over time, with the differences in intra- and inter-individual diversity being reduced after 40 days, which suggests an adaptation of microbial communities to dietary changes. At this point, gut microbial communities also showed a similar taxonomical composition. Moreover, a core microbiota consisting of species belonging to the genera Ralstonia, Paraburkholderia, Fulvimonas, Pseudomonas, and Cutibacterium was maintained in all sampling times under both dietary treatments. Overall, this study serves as a conceptual approach that shows a long-term adaptation of the gut microbiota after a radical dietary change, probably driven by host-inherent factors. Furthermore, these results may be a valuable insight for feed manufacturers aiming to develop sustainable and cost-effective ingredients since they suggest that some alternative feeds and ingredients do not have adverse long-term effects on fish gut microbiota.
1 Introduction
The fish gut microbiome plays an important role in numerous aspects of fish life-history and physiology, including digestion, nutrient absorption, immunity, and even behavior or stress response (Diwan et al., 2022). In recent years, understanding the microbial diversity and their metabolic function has garnered increasing attention due to its profound implications for fish condition and health, aquaculture practices, and ecosystem sustainability. The composition and diversity of the fish gut microbiota are influenced by various factors, such as habitat, host genetics, environmental conditions, and particularly, the diet-associated factors (Silva et al., 2011; Ghanbar et al., 2015; Degregori et al., 2024). Different dietary components, such as carbohydrates, proteins, lipids, and fiber, serve as specific substrates for microbial metabolism, that together with the feed-accompanying microbes may influence microbial species diversity (Li et al., 2014; Pan et al., 2023; Viver et al., 2023).
The growing interest in optimizing the utilization of fish meal and fish oil in aquafeeds has prompted to the search for sustainable and economically viable alternative ingredients. This search aims to identify substitutes that not only align with environmental sustainability but also contribute positively to fish health, with a particular focus on understanding the potential influence of dietary components on the gut microbiome (Rimoldi et al., 2018a; Niu et al., 2019; Xie et al., 2022). In addition, the importance of studying the diet-microbiome modulation also lies in the fact that dietary alternatives cannot only have health-promoting effects, but may also cause dysbiosis, impairing the composition and/or functions of the gut microbiota, which subsequently can have a physiological effect, such as the generation of an intestinal injury, inflammation or a reduced immune response (Infante-Villamil et al., 2021). Hence, examining microbial fluctuations over time after a diet change can offer valuable insights into the diet impact on the fish microbiota and intestinal health.
During the last years, numerous studies have highlighted that diet is a primary factor influencing gut microbial communities (Ringø et al., 2016; Egerton et al., 2018; Ruiz et al., 2024). However, the scientific literature often compares gut bacterial taxa in fish without accounting for whether they are reared under wild or cultured conditions. In this regard, several comparison studies have shown significant differences in gut microbial diversity, structure, and composition between farmed and wild species. This was the case for gilthead seabream (Sparus aurata) (Kormas et al., 2014), fine flounder (Paralichthys adspersus) (Ramírez and Romero, 2017), Nile tilapia (Oreochromis niloticus) (Bereded et al., 2021), common carp (Cyprinus carpio) (Ruzauskas et al., 2021), Korean rockfish (Sebastes schlegelii) (Yu et al., 2021), zig-zag eel (Mastacembelus armatus) (Liu et al., 2022), red cusk-eel (Genypterus chilensis) (Romero et al., 2022), brook trout (Salvelinus fontinalis) (Mugetti et al., 2023) and pike perch (Sander lucioperca) (Wang et al., 2024), among many other fish species. In addition, in our previous study we observed that two distinct wild fish species, the pearly razorfish (Xyrichtys novacula) and gilthead seabream, exhibited a more similar microbial profile than the wild and farmed gilthead seabream, suggesting that environmental and dietary factors significantly shape microbial composition (Viver et al., 2023). In this sense, numerous environmental factors influence microbial modulation, including the water temperature, salinity, and the microbial composition of the environment (Roeselers et al., 2011; Rudi et al., 2018). Furthermore, the ingredient formulation and composition of the commercial feeds differs significantly from the fish diets in natural environments. However, the aforementioned studies reflect that is challenging to discern between the impacts caused by the environmental conditions and by the diet on the gut microbial communities.
To elucidate the impact of the diet type on fish gut microbiota over time, this study aims to evaluate how the microbial communities of gilthead seabream are modulated over a 60-day period following a dietary shift from commercial feed to a diet exclusively composed of deep-water pink shrimp (Parapenaeus longirostris), which more closely mimics the fish diet under natural conditions. Gilthead seabream was selected as a biological model for this study due to its status as the second most farmed fish in the European Union (APROMAR, 2023) and its importance in aquafeed research within the framework of the search for novel sustainable ingredients (Matos et al., 2017; Porcino and Genovese, 2022). The current approach seeks to determine whether there is an adaptation period during which the microbiota adjusts to the diet change, and how the effect of the wild-type vs. commercial diet varies over time. The results of this study may provide valuable insights into the impact of the diet type on the frequently observed differences in microbiota composition when comparing wild and farmed fish, as well as supporting the exploration of novel aquafeeds formulated with more sustainable, cost-effective, and readily available ingredients.
2 Materials and methods
2.1 Experimental setup
The experiment was conducted at Insitute of Agrefood Research and Technology (IRTA) facilities, in La Ràpita (Tarragona, Spain). Gilthead seabream juveniles (1.6 g in body weight, BW) were purchased from a commercial fish hatchery in the East coast of Spain and transported by road to IRTA. A subset of these fish was used in our previous assay, so details about their acclimation may be found there (Viver et al., 2023). Fish with an average initial body weight (BWi) of 25.29 ± 4.90 g (standard deviation, SD) were randomly distributed in two tanks, each with a volume of 400 L (n = 30 per tank), connected to an IRTAmar™ water recirculation system, where they were maintained for 4 months. During three months the fish were fed with the commercial feed D-2 Optibream AE 1P (2.2 mm pellet size, Skretting; Nutreco N.V.), and in the fourth month, the commercial feed was progressively switched to D-4 Optibream AE 3P (4 mm pellet size, Skretting) in order to adjust pellet size to fish growth. The macronutrient proximate composition of D-2 Optibream AE 1P was 48.5% crude protein, 18% lipids and 18.5 MJ kg-1 digestible energy, while D-4 Optibream AE 3P contained 44% crude protein, 20% lipids and 18 MJ kg-1 digestible energy (Serie D2-D5 Fish meal composition is: fish oil, vegetable oils, cereal products and by-products, legume products and by-products, oilseed products and by-products, vitamins and minerals). After 4 months of being exclusively fed with commercial diets, the fish from the second tank were switched to a diet exclusively based on deep-water pink shrimp (T0), while the fish from the first tank continued being fed with D-4 Optibream AE 3P (Skretting), in order to compare the microbial dynamics induced by the diet change with respect to a control group over time. For the diet shift assay, 25 kg of entire individuals of deep-water pink shrimp (total length: 4-6 cm) which had been frozen only once just after capture with no further manipulation, were purchased from a local fishing company. However, commercial crustaceans normally contain sodium metabisulfite (E223), which is a synthetic antioxidant preservative added to avoid melanosis after removal from the water with a slight antimicrobial activity (Miraglia et al., 2021). To avoid the potential penetration of sodium metabisulfite into the shrimp tissues, before thawing, shrimps were cleaned with abundant sterile seawater. Then, shrimps were cut into pieces of between 2-3 cm length to facilitate their ingestion. Fish from both tanks were manually fed ad libitum with their respective diets three times per day during all the experiment, which extended for a period of 60 days after the diet change of the second tank (T0). Specimens from both tanks were collected at 20, 40, and 60 days after T0, (T20, T40, and T60, respectively). In each sampling time, eight specimens were collected from each condition (Supplementary Figure S1, Supplementary Table S1). To monitor the tanks’ water quality at the microbiological level during the experiment, each week an aliquot of 100 µL was spread onto TSA (Trypticase Soy Agar) + 0.6% NaCl, and TCBS (Thiosulphate Citrate Bile Salts Sucrose) Agar medium and incubated at 22°C for 48 h to obtain the Total Viable Counts (CFU) of heterotrophic microorganism and Vibrionaceae, respectively.
The water temperature (18–22°C) and dissolved oxygen (6.4 ± 0.6 mg L−1; OXI330, Crison Instruments) were daily measured and controlled, and pH (7.4 ± 0.2; pHmeter 507, Crison Instruments), salinity (36‰; MASTER-20 T, ATAGO Co. Ltd), ammonia (0.14 ± 0.1 mg NH4+ L−1) and nitrite (0.2 ± 0.1 mg NO2− L−1) levels (HACH DR9000 Colorimeter, Hach, Spain) were weekly monitored. Just after T20, the fish were transferred to two other 400 L tanks for the cleaning and maintenance of the tanks in this module, but the water quality and environmental conditions among modules were maintained.
2.2 Sample processing
For each sampling point, fish were manipulated as detailed previously (Viver et al., 2023). Briefly, all fish in each tank were deprived of feed for 86 h before the sampling in order to collect only the autochthonous microbiota (Hao and Lee, 2004; Naya-Català et al., 2021). Among them, fish were euthanized with an overdose of the buffered anesthetic (tricaine methanesulfonate, MS-222, Sigma-Aldrich; 300 mg L-1). The entire intestinal tract was aseptically extracted and the perivisceral fat surrounding the intestine was gently removed with a scalpel; then, a 3 cm section of the anterior intestine and another of the posterior intestine with the same length, were dissected using sterile scissors, and opened lengthwise. The mucosal content was gently scraped with a round edge spatula and immediately frozen at -80 °C for further microbial analysis. The rest of the individuals were fed again after the fasting period until the next sampling point when the above-mentioned process was repeated for the next 8 fish. The fish was considered as the experimental unit of this study since it is the smallest entity that is randomly assigned to a different treatment group (Wilson et al., 2023). In this sense, all fish used for the experiment had similar characteristics (fish age, size, origin), and they were exposed to the same controlled environmental conditions (temperature, dissolved oxygen, pH, salinity, nitrite, and ammonia levels) within the IRTAmar™ water recirculation system. The use of the fish as the experimental unit is a common practice in microbiota studies (Rimoldi et al., 2018b; Kokou et al., 2019; Naya-Català et al., 2021; Viver et al., 2023) which takes into account the inter-individual variation in the microbial communities (Legrand et al., 2020; Panteli et al., 2020) and aligns with the 3Rs principle (Zemanova, 2020).
2.3 Microbial composition of commercial feed and shrimp
Commercial feed pellets (D-4 OptiBream AE 3P) and samples of shrimps were stored at -80 °C at each sampling time. For processing purposes, three feed pellets and three shrimps were crushed with an autoclaved mortar and cut into very small pieces with sterile scissors, respectively. A total amount of ca. 150 mg in wet weight from feed or shrimps were collected and used for DNA extraction by means of the FastDNA™ Spin Kit for Feces (MP Biomedicals) following the manufacturer’s protocol. Three extractions for feed and shrimps were performed from each sampling time.
2.4 DNA isolation, amplification, and sequencing
The FastDNA™ Spin Kit for Feces (MP Biomedicals) was used to perform DNA extraction from the scraped mucosa content (up to 150 mg), following the manufacturer’s instructions. Illumina amplicon sequencing was performed using the set of primers of the V3-V4 region of the 16S rRNA gene for Bacteria forward (5’ - CCTACGGGNGGCWGCAG - 3’) and reverse (5’ - GACTACHVGGGTATCTAATCC - 3’) (Herlemann et al., 2011) containing the forward (5’ - TCGTCGGCAGCGTCAGATGTGTATAAGAGACAG - 3’) and reverse (5’ - GTCTCGTGGGCTCGGAGATGTGTATAAGAGACAG - 3’) Illumina sequencing adapters. A first PCR was performed with the following program: an initial step of 30 s at 98°C for polymerase activation and DNA denaturation, followed by 35 cycles of 10 s at 98°C, 30 s at 55°C, 30 s at 72°C, and a final extension step of 2 min at 72°C. Another 8-cycle amplification with similar cycle conditions was then carried out to add specific dual-index barcodes to each template. After each PCR, the templates were purified using Ampure XP beads (Beckman Coulter, USA) and 1 μL of a 1:50 dilution was analyzed on a Bioanalyzer DNA 1000 Chip (Agilent Technologies, USA) to check the size of the final library product (Illumina, 2013). Then, DNA samples were pooled at equimolar concentrations and sequencing was performed using an Illumina MiSeq instrument (2x300 bp) (FISABIO, Valencia, Spain).
2.5 Amplicon sequence variant and operational phylogenetic unit approaches
Raw sequencing data were provided in a demultiplexed FASTQ format containing paired-end reads. The data were analyzed using the Qiime2 bioinformatic platform (Bolyen et al., 2019) with the parameters –p-trunc-len-f 280, –p-trunc-len-r 220, –p-trim-left-f 19 and –p-trim-left-r 22. Amplicon sequence variant analyses (ASV) were obtained with DADA2 software implemented in Qiime2. The representative sequence of each ASV was aligned using the non-redundant SILVA REF 138.1 database (Quast et al., 2013) and the ARB package (Ludwig et al., 2004). Sequence alignment was performed using the SINA tool implemented in the ARB program (Pruesse et al., 2012). The aligned sequences were inserted in the SILVA REF 138.1 database containing the pre-stablished operational phylogenetic units (OPUs) detected in Viver et al. (2023) for taxonomic classification, using the parsimony tool available in the ARB package. The non-affiliated sequences to the pre-stablished OPUs were inserted again in the SILVA REF 138.1 database to select the close relative sequences for phylogenetic analysis and de novo OPUs identification. In this context, the phylogenetic tree was manually inspected, and isolated sub-branches containing the query sequences along with at least one representative sequence were grouped into OPUs based on visual assessment of the final tree to ensure a high degree of accuracy (Mora-Ruiz et al., 2016; Viver et al., 2015). Predictive functional profiling was carried out with the R package Tax4Fun (Ref99NR) (Aßhauer et al., 2015) using the trans_func class function of microeco (Liu et al., 2021). Metagenomics functions were inferred from the Kyoto Encyclopedia of Genes and Genomes (KEGG) and collapsed into pathways (level 1 – level 3).
2.6 Statistical analyses
Since the values of BW did not follow a normal distribution (Shapiro-Wilk test, P < 0.05), Wilcoxon-test was used to evaluate significant differences in BW among groups (P ≤ 0.05). To analyze the alpha and beta diversity indices, the dataset was rarefied to the minimum sample depth (21,494 sequencing reads per sample) with the vegan R package (Oksanen et al., 2022). To assess the phylogenetic complexity of the samples, rarefaction curves, Shannon and Dominance indices were calculated using the PAST statistic tool (Hammer et al., 2001) based on the diversity and abundance of 16S-based OPUs. The microeco R package (Liu et al., 2021) was used to calculate the Bray-Curtis dissimilarity values between the different community profiles of samples and periods of the assay based on OPUs. Non-metric multidimensional scaling (NMDS) plot based on Bray-Curtis dissimilarity was constructed using the vegan package (Oksanen et al., 2022) and plotted using the R package ggplot2 (Wickham, 2016) in R tool version 3.6.3 (R Core Team, 2020). The values of Shannon diversity index and Bray-Curtis dissimilarity were also compared between the two fish tanks at T0 (before the diet change) verifying that the tanks in which fish were initially located did not influence the microbial communities (Supplementary Figure S2). A divergence test between samples based on OPU abundance and composition was performed using non-parametric two-sample Kolmogorov-Smirnov tests (Jarek, 2015), since the data did not meet the assumptions of normality and homoscedasticity. Differences in alfa diversity indices and in the predictive functional pathways between groups were determined by Wilcoxon test (P ≤ 0.05), with P-values adjusted by False Discovery Rate (FDR). Differences in beta diversity were evaluated by performing permutational multivariate analyses of variance (PERMANOVA), with P-values adjusted by FDR. According to the definition given in previous studies (Astudillo-García et al., 2017; Neu et al., 2021; Custer et al., 2023), in this work the core microbiota was considered as the microbial taxa shared by at least two individual samples among all the experimental conditions which was present at an average abundance of at least 2%. The cutoff for defining an OPU as highly abundant was set at 1% relative abundance.
2.7 Terminology on bacterial phyla
The authors are aware that certain phyla names have been officially proposed by Oren and Garrity (2021) as Bacillota (= Firmicutes), Bacteroidota (= Bacteroidetes), Pseudomonadota (= Proteobacteria), Actinomycetota (= Actinobacteria), Planctomycetota (= Planctomycetes), Chloroflexota (= Chloroflexi), Fusobacteriota (= Fusobacteria) and Chlamydiota (= Chlamydia), but for pragmatic reasons and comparative purposes with available published literature, the former nomenclature was used in this manuscript.
3 Results
3.1 Fish growth
Along the 60-day experimental trial, we observed a consistent BW pattern in both dietary groups. At the initial sampling day in which a group was submitted to the diet change (T0), the fish weighed an average of 158 ± 32.9 grams. At T20, the fish fed with shrimp showed a significantly higher BW (211.2 ± 23.8) than the fish fed with commercial feed (190.0 ± 25.1; P = 0.038). However, during T40 and T60, fish fed with both diets exhibited a similar BW (Wilcoxon-test; P > 0.05): an average BW of 202.1 ± 34.1 and 259 ± 48 g for those fed with commercial feed, respectively, and 220.3 ± 31.9 and 252 ± 31.8 g for those fed with deep-water pink shrimp, respectively (P = 0.290 in T40, P = 0634 in T60; Supplementary Table S1).
3.2 Phylogenetic diversity based on OPUs
After trimming and chimera removal, all samples included in this study rendered a total of 10,957,204 sequenced reads, and the number of sequences per sample ranged between 21,494 and 160,269. All sequences were clustered in 8,072 ASVs and after phylogenetic inference a total of 1,256 OPUs were detected (ranging between 22 and 428 OPUs per sample) (Supplementary Table S2). Taking into consideration that an OPU is the smallest cluster of query sequences that affiliates with at least one reference sequence and considering that the size of each cluster typically falls within a sequence distance of less than <97% (considering the relatively short length of ~400 pb for partial sequences), we can assert with confidence that an OPU represents a single species (Mora-Ruiz et al., 2016). Each OPUs is recognized as a distinct species within genera whenever possible. To ensure statistical accuracy, the dataset was rarefied at the lowest number of sequences detected in a single sample (21,494; Supplementary Table S3), and the rarefaction curves demonstrated that the number of OPUs had reached their plateau at this number of reads for all samples (Supplementary Figure S3).
The phylogenetic analysis showed that the 1,256 OPUs were classified in 620 genera and 314 families, affiliated with 38 different phyla (Supplementary Tables S4, S5). In agreement with our previous assay (Viver et al., 2023), the phylum Proteobacteria was the most prevalent phylum, accounting for 42.9% of the total OPUs. Within this phylum, the majority of OPUs were classified as Gamma- and Alphaproteobacteria, representing 20.7% and 18.9% of the OPUs, respectively. Firmicutes (22.9% of the OPUs), Actinobacteriota (12.7%), Bacteroidota (7.0%), and Planctomycetota (2.2%) were also predominant in the examined samples. Furthermore, the study identified a total of 620 genera, from which 514 were represented by only one OPU. However, there was a considerable OPU diversity within some genera, such as Vibrio (32 distinct OPUs), Bacillus (29 OPUs), Lactobacillus (27 OPUs), Pseudomonas (22 OPUs), and Paenibacillus (15 OPUs).
3.3 Microbial composition of the feed pellets and shrimp
The microbial diversity patterns showed that the commercial feed and shrimps presented the highest diversity values, as measured by the Shannon index (average of 2.09 ± 0.22 in the commercial feed and 3.35 ± 0.58 in pink shrimps), and the lowest dominance values (average of 0.22 ± 0.04 in the commercial feed and 0.13 ± 0.1 in pink shrimps), indicating a more even distribution of species compared to the fish gut microbiomes (Figure 1; Supplementary Table S6). Shannon diversity values were positively correlated with the detection of a higher number of specific OPUs from commercial feed datasets. Out of the 424 OPUs detected in commercial feed and 810 OPUs detected in pink shrimp, a substantial proportion (30% and 35.7%, respectively) were exclusive to each set of samples. In contrast, the exclusive detected OPUs in the fish microbiomes ranged from 6.1% to 2.0% (Supplementary Table S4). The microbial diversity of the commercial feed remained relatively consistent throughout the experiment, with the lowest range of Bray-Curtis dissimilarity values (average value of 0.12 ± 0.06) (Supplementary Table S7) suggesting that storage did not significantly alter the microbial assemblage of feed pellets for at least 60 days. The OPU analysis revealed that 12 microbial species were consistently abundant (with an abundance close to 1% in all samples), and their combined abundance ranged from 89.8% to 95.02%. Among them, six of these species affiliated with phylum Firmicutes, specifically the class Bacilli, where the OPU0015, OPU0011, OPU0036 and OPU0037 affiliated with Lactobacillus (average abundance of 36.02 ± 5.8%, 9.02 ± 1.4%, 1.75 ± 0.4% and 1.67 ± 0.3%, respectively), Bacillus OPU0145 (2.61 ± 0.4%) and Staphylococcus OPU0076 (1.49 ± 0.3%). The other highly abundant OPUs affiliated with Ralstonia OPU0522 (4.4 ± 3.6%) and Photobacterium OPU0624 (3.47 ± 0.9%). Moreover, a high abundance of chloroplasts (OPU1059) and mitochondria (OPU1038) sequences were detected in all samples (Figure 2; Supplementary Table S8). Ralstonia OPU0522 was found to be shared among the commercial feed (4.39 ± 3.65%), deep-water pink shrimp (18.84 ± 19.51%) and all fish gut microbiome samples (37.81 ± 29.37%) (Figure 2).
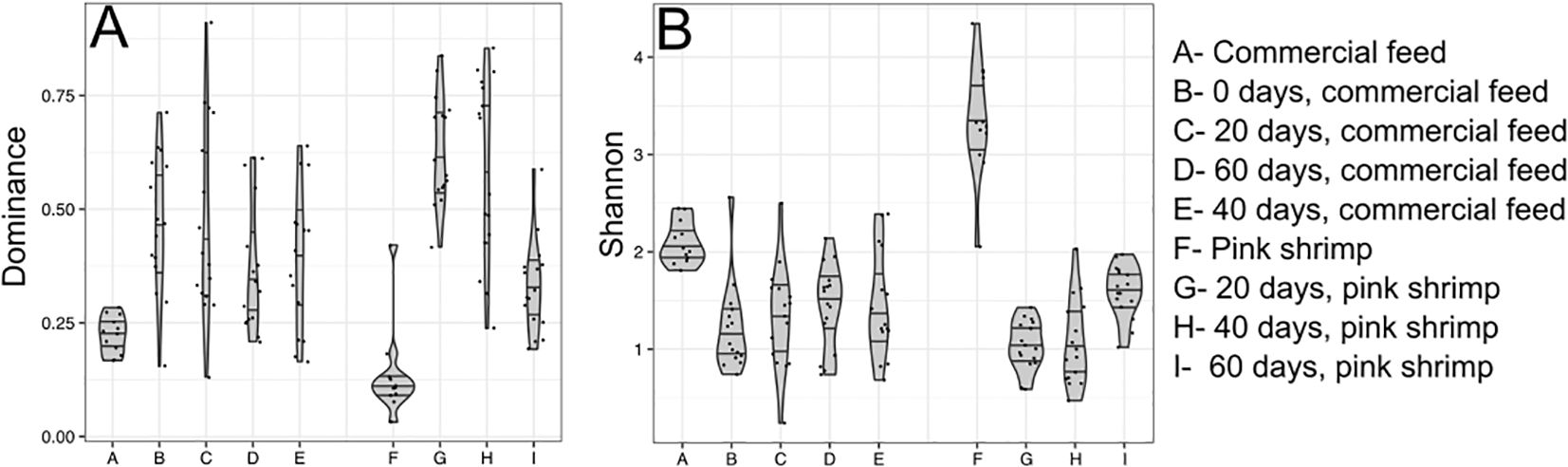
Figure 1. Alpha diversity variation among the experimental groups in relation to the Dominance (A) and Shannon (B) indices. Column A and F represent the direct microbial analysis of the commercial feed and deep-water pink shrimp (Parapenaeus longirostris), respectively. Columns B to E represent the gut microbiomes of gilthead seabream (Sparus aurata) collected at the beginning of the experiment and through 60 days of continuous feeding with commercial feed in chronological order. Columns G, H, and I represent the gut microbiomes of gilthead seabream fed with deep-water pink shrimp after 20, 40, and 60 days from the beginning of the experiment.
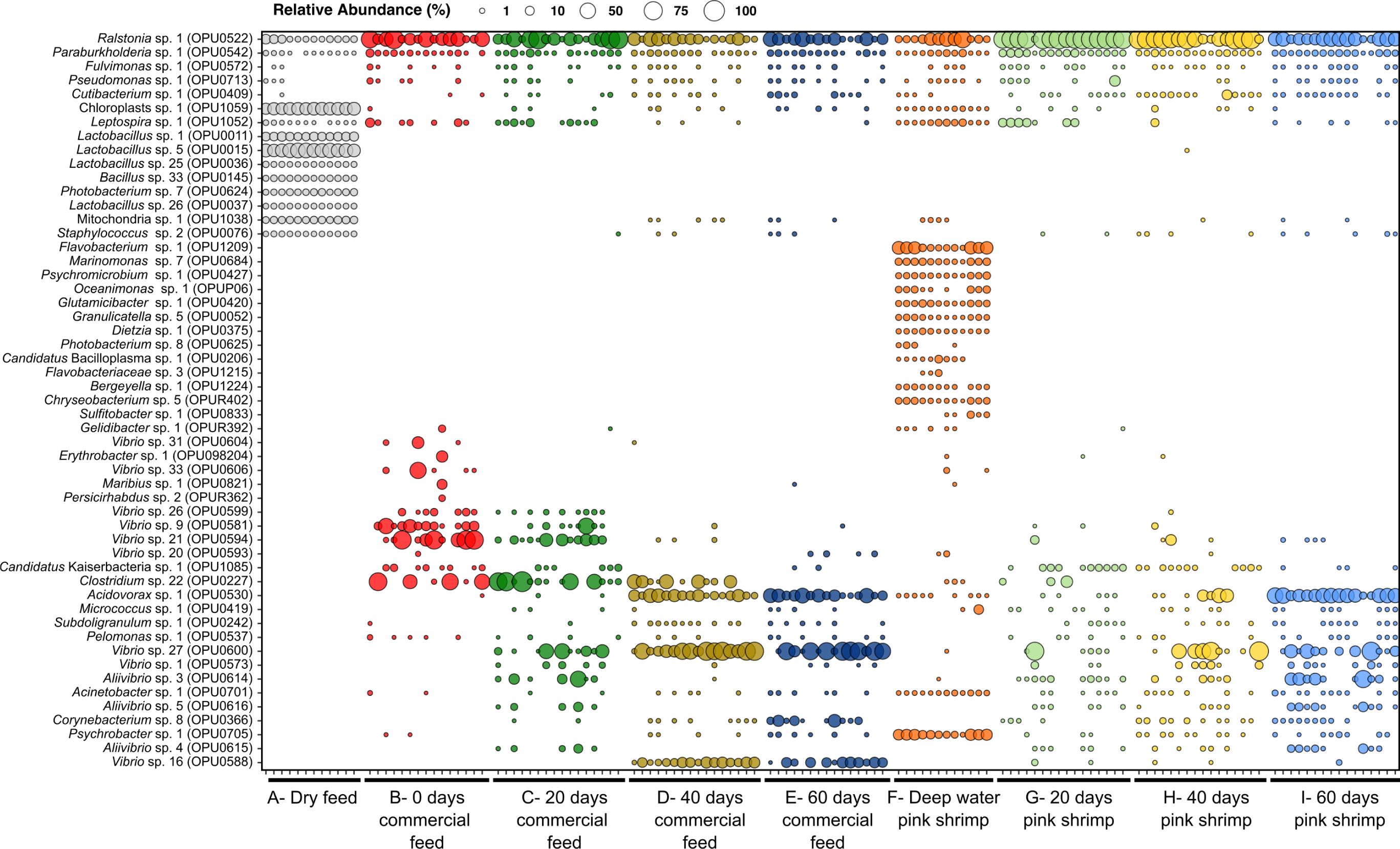
Figure 2. Relative abundance of the OPUs having a total average abundance >2%. Samples A and F represent the direct microbial analysis of the commercial feed and deep-water pink shrimp (Parapenaeus longirostris), respectively. Samples B to E represent the gut microbiomes of gilthead seabream (Sparus aurata) collected at the beginning of the experiment and through 60 days of continuous feeding with commercial feed in chronological order. Samples G, H, and I represent the gut microbiomes of gilthead seabream fed with deep-water pink shrimp after 20, 40, and 60 days from the beginning of the experiment.
The microbial composition of shrimp exhibited a slightly higher level of heterogeneity (average Bray-Curtis dissimilarity value of 0.54 ± 0.2) than the compound feed, but all samples were closely clustered in the NMDS plot (Figure 3A). This variability could be associated to the batch selected for DNA extraction and sequencing. The high abundant OPUs detected in shrimp and absent or poorly detected in fish microbiomes affiliated with the Flavobacterium OPU1209 (relative abundance of 14.4 ± 11.9%) and Chryseobacterium OPU_R402 (2.1 ± 1.5%) of the Bacteroidia class; Glutamicibacter OPU0420 (2.4 ± 1.4%) and Psychromicrobium OPU0427 (2.07 ± 1.4%) of the Actinobacteria class; the Psychrobacter OPU0705 (11.9 ± 7.3%), the Oceanimonas OPU_P06 (1.9 ± 1.9%) and Marinomonas OPU0684 (3.5 ± 1.78%) of the Gammaproteobacteria class.
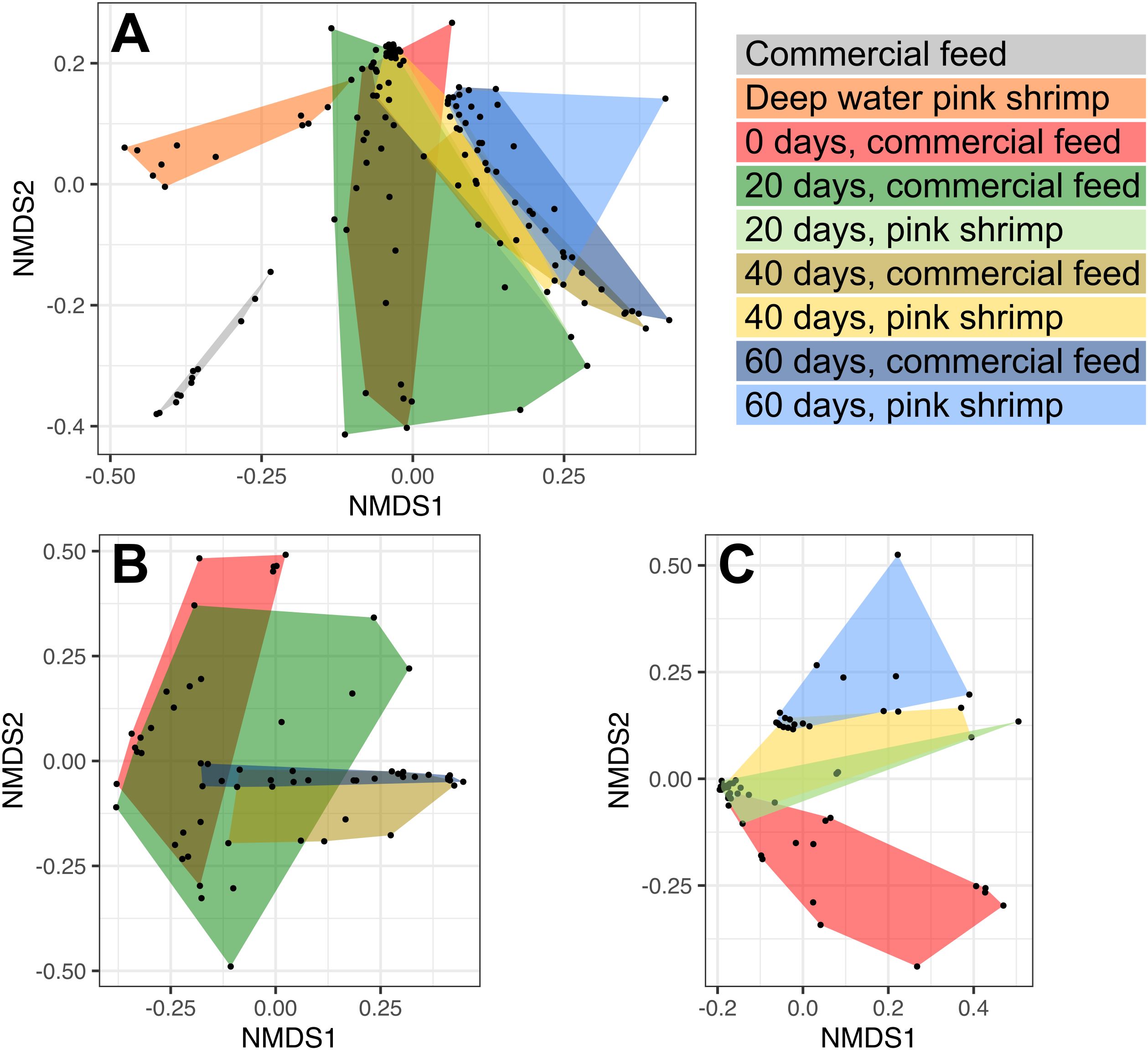
Figure 3. NMDS analyses based on Bray-Curtis distances of the microbiota of: (A) all samples included in this study; (B) gilthead seabream (Sparus aurata) fed with commercial feed; (C) gilthead seabream fed with deep-water pink shrimp (Parapenaeus longirostris). “Commercial feed” (grey) and “Pink shrimp” (orange) represents the direct microbial analysis of the commercial feed and deep-water pink shrimp, respectively. The dataset includes samples labeled by colors according to the day of the experiment followed by the type of feed provided to the fish.
3.4 Gut microbial communities in fish fed with commercial diet
All gilthead seabream juveniles were fed with commercial pellets upon arrival to the experimental facilities and during the 4 months preceding the start of the experiment on the shift of food items. At time zero (T0), the control group continued being fed with the commercial diet over a period of 60 days and we did not observe any differences in species diversity and abundance between distal and proximal intestine for 80% of the specimens studied (n = 16 intestinal samples per diet) as indicated by the Kolmogorov-Smirnov test (Supplementary Table S9) and the NMDS plots (Supplementary Figure S4), which shows the distinction between distal and proximal intestine samples over time and diet. At T0, all samples exhibited a similar dominance and Shannon indices with T20 (with an average dominance value ranging from 0.47 ± 0.15 to 0.47 ± 0.22 and Shannon index ranging from 1.22 ± 0.46 to 1.33 ± 0.53 in T0 and T20, respectively). We detected a slight increase in diversity with a decrease in the dominance after 40 and 60 days (average dominance value ranging from 0.37 ± 0.14 to 0.39 ± 0.16 and a Shannon index ranging from 1.44 ± 0.43 to 1.46 ± 0.55 in T40 and T60, respectively) (Figure 1; Supplementary Table S6). No significant differences were observed in dominance and Shannon indices over time (Wilcoxon-test; P > 0.05). The NMDS plot, based on the Bray-Curtis index, indicated that the dissimilarity between the control samples from the T0 and T20 datasets was higher (averaging 0.67 ± 0.24 and 0.7 ± 0.24, respectively) compared to that within the T40 and T60 datasets (averaging 0.46 ± 0.23 and 0.53 ± 0.29, respectively), suggesting a higher species homogeneity among individuals in the final stages of the experiment (Figure 3B; Supplementary Table S7). Comparisons between T0 and the rest of sampling times indicated that T60 showed the most significant microbial diversity changes (average Bray-Curtis dissimilarity value of 0.85 ± 0.12 between T0 and T60 samples) (Supplementary Table S7).
Commercial feed and control fish in all time points shared 206 OPUs, including the species Ralstonia OPU0522, Paraburkholderia OPU0542, Leptospira OPU1052, Fulvimonas OPU0572, Pseudomonas OPU0713 and Cutibacterium OPU0409 (Supplementary Table S8). There were microbial species exclusive in each dataset or sampling day. Out of the 319 total species detected, 5.6% were exclusive of the initial time (T0). At 20 days (T20), the percentage of exclusive species decreased to 4.6%, and at T40 and T60 the values were of 2.2% and 3.6%, respectively (Supplementary Table S4). The genus Vibrio exhibited the highest richness with 32 OPUs identified. In the fish, ten Vibrio species were identified as highly abundant (Figure 2). However, the prevalence of these species varied significantly over time. Specifically, Vibrio OPU0604, OPU0606, OPU0599, OPU0581 and OPU0594 were highly abundant in T0 and T20 days, and Vibrio OPU0600 and OPU0588 showed higher abundance in samples T20, T40 and T60. Aliivibrio OPU0614, OPU0615 and OPU0616 were abundant in T20. Candidatus Kaiserbacteria OPU1085 and Leptospira OPU1052 were highly abundant at T0 and T20 days, while Psychrobacter OPU0705, Corynebacterium OPU0366, Acinetobacter OPU0701 and Subdoligranulum OPU0242 were highly abundant in T40 and/or T60 days.
3.5 Gut microbial communities in fish fed with shrimp
The feed of the second group was replaced by shrimp at T0 and was maintained during 60 days. In this case, the alpha diversity values showed that the switch to a shrimp diet resulted in a reduction in microbial diversity along the first 20 days, as indicated by the non-significant, but numeral decrease in Shannon values (average from 1.22 ± 0.46 at T0 to 1.04 ± 0.25 at T20 (Wilcoxon-test; P = 0.42)), accompanied by a significant increase in dominance (average from 0.47 ± 0.15 at T0 to 0.63 ± 0.12 at T20 (Wilcoxon-test; P = 0.008)). However, after a gradual increase in diversity and a decrease in dominance occurred, reaching similar levels to the control at T60 [average Shannon value of 1.6 ± 0.27 (Wilcoxon-test; P = 0.26) and dominance of 0.33 ± 0.1 at T60 (Wilcoxon-test; P = 0.28)] (Figure 1; Supplementary Table S6).
Regarding beta diversity, the NMDS plot shows that the samples became more homogeneous after 20 days reflected by decrease in the average Bray-Curtis dissimilarity value from 0.67 ± 0.24 at T0 to 0.3 ± 0.25 at T20. This shift coincided with a significant increase in the dominance of the Ralstonia OPU0522, which exhibited an average abundance value of 73.59 ± 20.49% at T20. All the groups compared in this experiment were significant different among them (showing P-values < 0.01; PERMANOVA), except for fish fed commercial feed at T0 vs. fish fed commercial feed at T20 (P = 0.137), fish fed commercial feed at T40 vs. fish fed commercial feed at T60 (P = 0.204), and fish fed pink shrimp at T20 vs. fish fed pink shrimp at T40 (P = 0.098). A temporal shift in beta diversity was observed during the experiment, with the most significant changes occurring in the samples collected at T60, as shown by the differences in beta diversity observed during the rest of timepoints (P < 0.01) and represented in the NMDS (Figure 3C). We observed a similar pattern for the control dataset in the percentage of exclusive OPUs, with 6.1%, 6%, and 2% of OPUs being unique to T20, T40, and T60, respectively (Supplementary Table S4). A total of 324 OPUs shared between the shrimps and the gut microbiota of the fish fed with them were also observed. Among the most abundant OPUs from shrimp, very few were shared between the fish gut microbiome and the shrimp, such as Paraburkholderia OPU0542 and Pseudomonas OPU0713 (Figure 2). The Candidatus Kaiserbacteria OPU1085 was manly present in samples T20 and Vibrio OPU0600 and OPU0573 were mainly detected in specimens collected at T40 and T60. On the other hand, Acidovorax OPU0530 and Aliivibrio OPU0614, OPU0615 and OPU0616 were most abundant in T60 (Figure 2).
Despite significant differences between the two dietary treatments in all the timepoints (P < 0.01), the NMDS based on Bray-Curtis dissimilarity showed a higher overlapping in the microbial structure of fish fed both diets at the final stage of the experiment (T60) with respect to T20 groups (Figure 3A). Moreover, when evaluating dominance and Shannon index values at T60, no statistically significant differences were observed between fish fed with commercial feed and shrimp (Wilcoxon-test; P = 0.05).
4 Discussion
In this study, we examined the impact of a dietary shift (from a compound diet to a wild type shrimp-based diet) on the resident (autochthonous) gut microbiota of a commercially significant fish species. In all cases, bacteria accompanying the feed had a limited influence on the diversity in the gut, which was evidenced by the independent clustering of the diets with respect to the fish as well as by the different Shannon diversity and dominance indices. For instance, highly abundant species in the commercial feed or in shrimps did not establish in fish gut. Other studies also described different bacterial compositions between feed and intestinal autochthonous microbiota of many teleosts as gilthead seabream (Rimoldi et al., 2018b), European sea bass (Dicentrarchus labrax) (Rimoldi et al., 2020) or rainbow trout (Oncorhynchus mykiss) (Rimoldi et al., 2021). Our findings are also supported by similar studies using experimental diets for gilthead seabream with increasing levels of Firmicutes that not only did not establish, but rather decreased in abundance over time (Moroni et al., 2021), and by a previous study in European sea bass (Rimoldi et al., 2021), where the feed pellets were dominated by Lactobacillus that did not either establish in their guts (Rimoldi et al., 2021). In this sense, while some studies have shown the presence of feed-associated bacteria in the fish digesta (Wilkes Walburn et al., 2019; Gaudioso et al., 2021; Ruiz et al., 2024), these bacteria may not establish in the gut as part of the autochthonous commensal microbiota, as suggested by previous works (Karlsen et al., 2022; Viver et al., 2023). In addition, commercial feeds are submitted to an extrusion process reaching high temperatures (> 100°C) that would washout the vast majority of viable bacteria (Encarnação, 2016; Konovalenko et al., 2022), but some species, as the observed Lactobacillus sp. may develop as a consequence of fermentative conditions during feed processing, manipulation or storage. These organisms or their residual DNA can be identified through amplicon sequencing, even in cases where they are non-viable, signifying their transient presence in the fish gut.
One of the most relevant species detected was Ralstonia sp. 1, which was found in remarkable abundance in the gut of gilthead seabream in our previous study (Viver et al., 2023). Members of this genus are normally found as part of the autochthonous gut microbiota in several fish species, such as rainbow trout (Kim et al., 2007), yellow catfish (Pelteobagrus fulvidraco) (Wu et al., 2010), European sea bass (Carda-Diéguez et al., 2014), yellowtail kingfish (Seriola lalandi) (Dam et al., 2020), large yellow croaker (Larimichthys crocea) (Zhang et al., 2022), pearly razorfish, and gilthead seabream (Viver et al., 2023). In the present study, Ralstonia was present in all samples, but its abundance was clearly enhanced in fish fed with shrimps. Contrarily to the commercial feed, beyond freezing, shrimps were not submitted to a pre-processing to destroy or minimize the accompanying bacteria. Thus, the increased amounts of Ralstonia in the fish fed with shrimps may partially respond to the colonization of this genus having its origin in the feed. Despite the commercial feed also contained Ralstonia, probably originated from fish meal, these bacteria may be not viable and this fact would explain the lack of their growth in fish guts. Although further research is needed to unravel the role of the members of this aerobic genus in the fish intestine, they may play an important role in marine animals, and their presence in the guts of fish may respond to a symbiotic or mutualistic relation (Cerezo-Ortega et al., 2021). Indeed, some Ralstonia species have been suggested to have antimicrobial activity biosynthesize bioactive compounds, and produce beneficial secondary metabolites for the host fish (Cerezo-Ortega et al., 2021).
Altogether, here the type of feed does not seem to be a key factor as a source of bacteria, as no remarkable changes were found between the two groups for the rest of represented species (> 2%). However, the diet ingredients could modulate the gut autochthonous microbiomes. Utilizing diverse protein, lipid, and carbohydrate sources, or incorporating varying levels of dietary supplements, can induce a spectrum of pronounced effects on the microbial profile of the fish. These effects may be directly and/or indirectly initiated by alterations in host physiology (Ringø et al., 2016). For example, here, the effect of the diet was reflected in the differential relative abundances of some Aliivibrio species in the group fed shrimps, especially at T60, that were not detected when using the commercial diet. These species related to A. finisterrensis, A. fischeri, and A. logei, seem to be established in the gut of marine teleosts (Dunn, 2012; Hatje et al., 2014; Bazhenov et al., 2019). Some Aliivibrio species in the gut of the Atlantic cod (Gadus morhua) has been reported to be stimulated by the supplementation with chitin (Ringø et al., 2012), one of the main components of exoskeletal shells from crustaceans (Kurita, 2006). In aquafeed, chitin is considered as a prebiotic, able to increase bacterial intestinal richness by favoring the growth of beneficial and chitin-degrading bacteria (Mohan et al., 2023; Rimoldi et al., 2023). In this sense, many Aliivibrio species have chitinase activity (Raimundo et al., 2021), which may aid in the digestion of this ingredient since many marine fish, such as gilthead seabream, have shown a low chitin digestibility (Piccolo et al., 2017; Belghit et al., 2018; Basto et al., 2020). Then, it seems plausible that the higher growth of Aliivibrio in fish fed with shrimp compared to those fed with commercial feed was due to the probiotic effect of chitin. However, no differences on the KEGG pathway “Other glycan degradation” were observed at T60 between both groups (fish fed commercial feed: 0.04%; fish fed pink shrimp: 0.03%; P = 0.31). Nonetheless, this metabolic pathway does not only reflect degradation of chitin, but also of other glycans, such as starch, cellulose, and xylans, which are likely present in the commercial feed and may be degraded by the fish gut microbiota (Pedrotti et al., 2015; Maas et al., 2020). Further research on transcriptomic and/or activity characterization is needed to confirm whether the absence of changes in this metabolic pathway responded to the ability of different taxa to degrade the distinct types of glycans. In addition, we cannot discard that the transient feed-associated microbes that do not establish in the gut, if viable, may also influence the gut autochthonous microbiota (e.g., the probiotic effects in modulating microbial functionality and exerting a beneficial effect on the fish health; Rimoldi et al., 2021; Marco, 2019; Schoultz and Keita, 2019).
Beyond dietary factors, the gut microbiome is also influenced by the environmental conditions such as the temperature which is a paramount driver determining fish microbiomes (Rudi et al., 2018; Soriano et al., 2018; Kim et al., 2021). In this context, we observed and increase in abundance of Staphylococcus, Acidovorax, Vibrio, Corynebacterium, and Psychrobacter in both groups regardless the diet provided just after T20 when we relocated the fish in the new tanks for the cleaning and maintenance. Although we maintained the environmental conditions between tanks after the change, the differential microbiota in the water and on the tanks’ wall biofilms might have been the source of these taxonomic changes (Minich et al., 2020; Zeng et al., 2020). Unfortunately, we did not collect samples from water or biofilms from the tanks to compare their taxonomic profiles, and therefore, we cannot confirm whether these bacteria were present in the new tanks. Additionally, some of the changes induced by the relocation were more marked in gilthead seabream fed with the commercial diet, such as the differential abundances of some Vibrio species and Clostridium sp. 22 before and after T20. The higher impact of the relocation in fish fed with the commercial diet in comparison to those fed with pink shrimp may be attributed to their longer adaptation period to the diet before the relocation. In this context, at the moment of the transference fish fed the commercial feed had been already adapting to this diet type for 7 weeks plus other 3 previous months when they were fed with a similar commercial diet. In this sense, a microbial adaptation over time can be expected in response to external changing factors, such as shifts in the diet (Michl et al., 2017, 2019), the feeding regime (Sherif et al., 2020), an antibiotic administration (Payne et al., 2022), or an induced stress (Webster et al., 2021). Considering that the period of adaptation to a diet can last for some weeks (up to 12 weeks in some cases), with variations depending on the fish species, size and diet formulation among other experimental factors (Heikkinen et al., 2006; Ingerslev et al., 2014; Keating et al., 2021), the changes in the gut microbial communities of fish fed with pink shrimp observed from T20 on may reflect the combined or synergetic effect of the adaptation to the diet change and to the relocation.
Under current experimental conditions, the most striking observation was to find that there were some bacteria which were present in all groups of individuals regardless the sampling time and diet provided, such as Ralstonia sp. 1 (15.6-73.6%), Paraburkholderia sp. 1 (1.6-16.3%), Fulvimonas sp. 1 (0.2-1.4%), Pseudomonas sp. 1 (0.1-0.7%) and Cutibacterium sp. 1 (0.1-5.2%). Thus, this bacterial members could be considered as part of the core microbiota of this farmed species (Astudillo-García et al., 2017; Roeselers et al., 2011; Rudi et al., 2018; Kokou et al., 2019). In addition, the same OPUs identified as Ralstonia sp. 1, Paraburkholderia sp. 1, Pseudomonas sp. 1 and Cutibacterium sp. 1 were found at high abundances in our previous study in the intestine of farmed and wild fasted gilthead seabream (Viver et al., 2023), which reinforces the hypothesis that these genera may be considered as part of the core microbiota of this species regardless the external conditions, such as the type of diet and/or the animal lifestyle. The similarities in terms of species composition and abundance among individuals were reflected in a similar diversity (Shannon diversity and Dominance indices) and structure (representation of Bray-Curtis distances in NMDS, despite significant differences) of both dietary groups at the later stages of the experiment (T40 and T60). It seems that the core microbiome prevalence is not affected by the environmental changes after the adaptation processes in the tanks. In contraposition, studies in juveniles of rainbow trout (Michl et al., 2017) and brown trout (Salmo trutta) (Michl et al., 2019) have shown that the microbiome composition is directly dependent in the actual diet provided after a dietary shift, but barely influenced by the initial diet provided before the change. However, these juveniles were younger and with much lesser body weight (around two orders of magnitude smaller) than ours at the end of the assay, which pinpoints that the differential plasticity of the fish gut microbiota to adapt to diet changes depends on the animal age as it was previously postulated in gilthead seabream (Piazzon et al., 2019) as well as in mammals' gut (Quercia et al., 2014; Barreto et al., 2020) and rumen (Yin et al., 2021).
Previous results have already showed that there are host-inherent factors which act as paramount modulators of the fish gut microbiota, such as the species, sex, age, and genetic background (Navarrete et al., 2012; Piazzon et al., 2019). Overall, the present results suggest that a diet change have much less weight in the modulation of the gut microbiota than these host-inherent factors, as demonstrated by the presence of a core microbiome in all fish regardless the diet provided, leading to gut bacterial communities with a similar diversity, structure, and composition in the long-term. These findings are consistent with those of Keating et al. (2021), who observed that the effect of time on the hindgut microbiota of Atlantic cod was significantly higher than the impact of different dietary treatments which resulted in similar microbial communities over time. In addition, these authors also identified a core microbiome that persisted throughout the 12-week duration of the experiment. Furthermore, previous studies in rainbow trout have shown that its gut microbiota is highly sensitive to new diets, but stabilizes over time (Ingerslev et al., 2014). Similarly, Niu et al. (2020) observed that when comparing the gut microbial profiles of olive flounder (Paralichthys olivaceus) fed two diets with different replacement levels of fish meal by plant-based protein sources over an 8-month period, the effect of the growth stage on the gut microbiota was much higher than the diet effect. Moreover, Kokou et al. (2019) reported a common core microbiome over time in European seabass fed with different diets over a 6-week period. Our hypothesis is also in consistency with the existing evidence of a host-specific shaping of the fish gut microbiota regardless the environmental conditions (Nikouli et al., 2021). However, in the present trial the diversity, structure, and composition of the microbial communities of gilthead seabream fed each type of diet was very different in the first 20 days after the dietary shift. Given that there are many variables that may affect the fish feeding regimes in natural conditions (i.e., dietary changes based on prey availability), our results indicate that even when considering the specific environmental conditions of each study, it is not advisable to establish comparisons of the microbiota of wild and farmed fish. Furthermore, the relevance of these findings lies in the adaptation of the microbial communities to the diets over time, which offers the possibility to explore novel feeds formulated with more sustainable, cost-effective, and readily available ingredients and which may not have a long-term effect on the fish microbiota.
Data availability statement
The datasets presented in this study can be found in online repositories. The names of the repository/repositories and accession number(s) can be found below: https://www.ebi.ac.uk/ena, PRJEB64773.
Ethics statement
The animal study was approved by the Ethical Committee of Animal Experimentation of the Institute of Agrifood Research and Technology (Spain) and the Generalitat of Catalunya (Ref. CEEA 219/2020). The study was carried out by trained competent personnel in accordance with European Directive 2010/63/UE and Spanish Royal Decree RD53/2013 to ensure good practices for animal care, health, and welfare. The study was conducted in accordance with the local legislation and institutional requirements.
Author contributions
AR: Conceptualization, Data curation, Formal Analysis, Investigation, Methodology, Validation, Visualization, Writing – original draft, Writing – review & editing. JA: Conceptualization, Investigation, Writing – original draft, Writing – review & editing, Funding acquisition, Supervision. EG: Conceptualization, Funding acquisition, Supervision, Writing – original draft, Writing – review & editing, Resources, Validation. DF: Conceptualization, Funding acquisition, Resources, Supervision, Validation, Writing – original draft, Writing – review & editing. TV: Conceptualization, Validation, Writing – original draft, Writing – review & editing, Data curation, Formal Analysis, Investigation, Methodology, Software, Visualization.
Funding
The author(s) declare financial support was received for the research, authorship, and/or publication of this article. IRTA internal funding contributed to the design and development of the study. The research was carried out within the framework of the activities of the Spanish Government through the “Maria de Maeztu Centre of Excellence” accreditation to IMEDEA (CSIC-UIB) (CEX2021-001198). AR was supported by a pre-doctoral grant (PRE2019-091259) linked to the ADIPOQUIZ project (RTI2018-095653-R-I00), funded by the Spanish Ministry of Science and Innovation. JA received funding by the METARAOR Project (Grant num. PID2022-139349OB-I00) funded by MCIN/AEI/10.13039/501100011033/FEDER, UE. TV was supported by the “Margarita Salas” postdoctoral grant, funded by the Spanish Ministry of Universities, within the framework of the Recovery, Transformation and Resilience Plan funded by the European Union (NextGenerationEU), with the participation of the University of the Balearic Islands (UIB).
Acknowledgments
We acknowledge Ramon Rossello-Mora for critical reading and recommendations, and also for participating in funding the amplicon sequencing of the samples included in this study by the projects CTM2017-91490-EXP and PID2021-126114NB-C42 of the Spanish Ministry of Science, Innovation and Universities which were also supported by the European Regional Development Fund (FEDER). Authors also would like to acknowledge Edgar Bertomeu, Maria Curto and Mercedes Urdiain for their contribution to the sampling and sample processing in this study.
Conflict of interest
The authors declare that the research was conducted in the absence of any commercial or financial relationships that could be constructed as a potential conflict of interest.
Publisher’s note
All claims expressed in this article are solely those of the authors and do not necessarily represent those of their affiliated organizations, or those of the publisher, the editors and the reviewers. Any product that may be evaluated in this article, or claim that may be made by its manufacturer, is not guaranteed or endorsed by the publisher.
Supplementary material
The Supplementary Material for this larticle can be found online at: https://www.frontiersin.org/articles/10.3389/fmars.2024.1498892/full#supplementary-material
References
Aßhauer K. P., Wemheuer B., Daniel R., Meinicke P. (2015). Tax4Fun: predicting functional profiles from metagenomic 16S rRNA data. Bioinformatics 31, 2882–2884. doi: 10.1093/bioinformatics/btv287
APROMAR (2023). Aquaculture in Spain 2023 (Spain: Asociación de Empresas de Acuicultura de España (APROMAR).
Astudillo-García C., Bell J. J., Webster N. S., Glasl B., Jompa J., Montoya J. M., et al. (2017). Evaluating the core microbiota in complex communities: a systematic investigation. Environ. Microbiol. 19, 1450–1462. doi: 10.1111/1462-2920.13647
Barreto H. C., Sousa A., Gordo I. (2020). The landscape of adaptive evolution of a gut commensal bacterium in aging mice. Curr. Biol. 30, 1102–1109. doi: 10.1016/j.cub.2020.01.037
Basto A., Matos E., Valente L. M. (2020). Nutritional value of different insect larvae meals as protein sources for European sea bass (Dicentrarchus labrax) juveniles. Aquaculture 521, 735085. doi: 10.1016/j.aquaculture.2020.735085
Bazhenov S. V., Khrulnova S. A., Konopleva M. N., Manukhov I. V. (2019). Seasonal changes in luminescent intestinal microflora of the fish inhabiting the Bering and Okhotsk seas. FEMS Microbiol. Ecol. 366, fnz040. doi: 10.1093/femsle/fnz040
Belghit I., Liland N. S., Waagbø R., Biancarosa I., Pelusio N., Li Y., et al. (2018). Potential of insect-based diets for Atlantic salmon (Salmo salar). Aquaculture 491, 72–81. doi: 10.1016/j.aquaculture.2018.03.016
Bereded N. K., Abebe G. B., Fanta S. W., Curto M., Waidbacher H., Meimberg H., et al. (2021). The impact of sampling season and catching site (wild and aquaculture) on gut microbiota composition and diversity of Nile tilapia (Oreochromis niloticus). Biology 10, 180. doi: 10.3390/biology10030180
Bolyen E., Rideout J. R., Dillon M. R., Bokulich N. A., Abnet C. C., Al-Ghalith G. A., et al. (2019). Reproducible, interactive, scalable and extensible microbiome data science using QIIME 2. Nat. Biotechnol. 37, 852–857. doi: 10.1038/s41587-019-0252-6
Carda-Diéguez M., Mira A., Fouz B. (2014). Pyrosequencing survey of intestinal microbiota diversity in cultured sea bass (Dicentrarchus labrax) fed functional diets. FEMS Microbiol. Ecol. 87, 451–459. doi: 10.1111/1574-6941.12236
Cerezo-Ortega I. M., Di Zeo-Sánchez D. E., García-Márquez J., Ruiz-Jarabo I., Sáez-Casado M. I., Balebona M. C., et al. (2021). Microbiota composition and intestinal integrity remain unaltered after the inclusion of hydrolysed Nannochloropsis gaditana in Sparus aurata diet. Sci. Rep. 11, 18779. doi: 10.1038/s41598-021-98087-5
Custer G. F., Gans M., van Diepen L. T., Dini-Andreote F., Buerkle C. A. (2023). Comparative analysis of core microbiome assignments: implications for ecological synthesis. Msystems 8, e01066–e01022. doi: 10.1128/msystems.01066-22
Dam C. T., Booth M., Pirozzi I., Salini M., Smullen R., Ventura T., et al. (2020). Alternative feed raw materials modulate intestinal microbiota and its relationship with digestibility in Yellowtail Kingfish Seriola lalandi. Fishes 5, 14. doi: 10.3390/fishes5020014
Degregori S., Schiettekatte N. M., Casey J. M., Brandl S. J., Mercière A., Amato K. R., et al. (2024). Host diet drives gut microbiome convergence between coral reef fishes and mammals. Mol. Ecol. 33, e17520. doi: 10.1111/mec.17520
Diwan A. D., Harke S. N., Panche A. N. (2022). Aquaculture industry prospective from gut microbiome of fish and shellfish: An overview. J. Anim. Physiol. Anim. Nutr. 106, 441–469. doi: 10.1111/jpn.13619
Dunn A. K. (2012). Vibrio fischeri metabolism: symbiosis and beyond. Adv. Microb. Physiol. 61, 37–68. doi: 10.1016/B978-0-12-394423-8.00002-0
Egerton S., Culloty S., Whooley J., Stanton C., Ross R. P. (2018). The gut microbiota of marine fish. Front. Microbiol. 9. doi: 10.3389/fmicb.2018.00873
Encarnação P. (2016). “Functional feed additives in aquaculture feeds,” in Aquafeed formulation, 1st edn. Ed. Nates S. F. (Academic Press, Oxford), 217–237. doi: 10.1016/B978-0-12-800873-7.00005-1
Gaudioso G., Marzorati G., Faccenda F., Weil T., Lunelli F., Cardinaletti G., et al. (2021). Processed animal proteins from insect and poultry by-products in a fish meal-free diet for rainbow trout: Impact on intestinal microbiota and inflammatory markers. Int. J. Mol. Sci. 22, 5454. doi: 10.3390/ijms22115454
Ghanbar M., Kneifel W., Domig K. J. (2015). A new view of the fish gut microbiome: advances from next-generation sequencing. Aquaculture 448, 464–475. doi: 10.1016/j.aquaculture.2015.06.033
Hammer Ø., Harper D., Ryan P. (2001). PAST: Paleontological Statistics software package for education and data analysis. Palaeontol. Electron. 4, 9.
Hao W. L., Lee Y. K. (2004). Microflora of the gastrointestinal tract: a review. Methods Mol. Biol 268, 491–502. doi: 10.1385/1-59259-766-1:491
Hatje E., Neuman C., Stevenson H., Bowman J. P., Katouli M. (2014). Population dynamics of Vibrio and Pseudomonas species isolated from farmed Tasmanian Atlantic salmon (Salmo salar L.): a seasonal study. Microbial Ecol. 68, 679–687. doi: 10.1007/s00248-014-0462-x
Heikkinen J., Vielma J., Kemiläinen O., Tiirola M., Eskelinen P., Kiuru T., et al. (2006). Effects of soybean meal based diet on growth performance, gut histopathology and intestinal microbiota of juvenile rainbow trout (Oncorhynchus mykiss). Aquaculture 261, 259–268. doi: 10.1016/j.aquaculture.2006.07.012
Herlemann D. P., Labrenz M., Jürgens K., Bertilsson S., Waniek J. J., Andersson A. F. (2011). Transitions in bacterial communities along the 2000 km salinity gradient of the Baltic Sea. ISME J. 5, 1571–1579. doi: 10.1038/ismej.2011.41
Illumina (2013). 16S Metagenomic Sequencing Library Preparation: Preparing 16S Ribosomal RNA Gene Amplicons For The Illumina Miseq System. Available online at: https://www.Illumina.Com/Content/Dam/Illumina-Support/Documents/Documentation/Chemistry_Documentation/16s/16s-Metagenomic-Library-Prep-Guide-15044223-B.Pdf (Accessed November 28, 2024).
Infante-Villamil S., Huerlimann R., Jerry D. R. (2021). Microbiome diversity and dysbiosis in aquaculture. Rev. Aquac. 13, 1077–1096. doi: 10.1111/raq.12513
Ingerslev H. C., von Gersdorff Jørgensen L., Strube M. L., Larsen N., Dalsgaard I., Boye M., et al. (2014). The development of the gut microbiota in rainbow trout (Oncorhynchus mykiss) is affected by first feeding and diet type. Aquaculture 424, 24–34. doi: 10.1016/j.aquaculture.2013.12.032
Jarek S. (2015). Package ‘mvnormtest’: Normality test for multivariate variables. RPackage Version. 0, 1–9.
Karlsen C., Tzimorotas D., Robertsen E. M., Kirste K. H., Bogevik A. S., Rud I. (2022). Feed microbiome: confounding factor affecting fish gut microbiome studies. ISME Commun. 2, 14. doi: 10.1038/s43705-022-00096-6
Keating C., Bolton-Warberg M., Hinchcliffe J., Davies R., Whelan S., Wan A. H. L., et al. (2021). Temporal changes in the gut microbiota in farmed Atlantic cod (Gadus morhua) outweigh the response to diet supplementation with macroalgae. Anim. Microbiome 3, 7. doi: 10.1186/s42523-020-00065-1
Kim D. H., Brunt J., Austin B. (2007). Microbial diversity of intestinal contents and mucus in rainbow trout (Oncorhynchus mykiss). J. Appl. Microbiol. 102, 1654–1664. doi: 10.1111/j.1365-2672.2006.03185.x
Kim P. S., Shin N. R., Lee J. B., Kim M. S., Whon T. W., Hyun D. W., et al. (2021). Host habitat is the major determinant of the gut microbiome of fish. Microbiome 9, 166. doi: 10.1186/s40168-021-01113-x
Kokou F., Sasson G., Friedman J., Eyal S., Ovadia O., Harpaz S., et al. (2019). Core gut microbial communities are maintained by beneficial interactions and strain variability in fish. Nat. Microbiol. 4, 2456–2465. doi: 10.1038/s41564-019-0560-0
Konovalenko L. Y., Nemenushchaya L. A., Shchegolikhina T. A. (2022). Techniques for up-to-date aquaculture compound feed production facilities. IOP Conf. Ser.: Earth Environ. Sci. 954, 012038. doi: 10.1088/1755-1315/954/1/012038
Kormas K. A., Meziti A., Mente E., Frentzos A. (2014). Dietary differences are reflected on the gut prokaryotic community structure of wild and commercially reared sea bream (Sparus aurata). Microbiologyopen 3, 718–728. doi: 10.1002/mbo3.202
Kurita K. (2006). Chitin and chitosan: functional biopolymers from marine crustaceans. Mar. Biotechnol. 8, 203–226. doi: 10.1007/s10126-005-0097-5
Legrand T. P., Wynne J. W., Weyrich L. S., Oxley A. P. (2020). A microbial sea of possibilities: current knowledge and prospects for an improved understanding of the fish microbiome. Rev. Aquaculture. 12, 1101–1134. doi: 10.1111/raq.12375
Li J., Ni J., Li J., Wang C., Li X., Wu S., et al. (2014). Comparative study on gastrointestinal microbiota of eight fish species with different feeding habits. J. Appl. Microbiol. 117, 1750–1760. doi: 10.1111/jam.12663
Liu C., Cui Y., Li X., Yao M. (2021). Microeco: an R package for data mining in microbial community ecology. FEMS Microbiol. Ecol. 97, fiaa255. doi: 10.1093/femsec/fiaa255
Liu X., Fan Y., Mo T., Chen Q., Chen W. (2022). Comparative study of the gut microbiota community between the farmed and wild Mastacembelus armatus (zig-zag eel). Metabolites 12, 1193. doi: 10.3390/metabo12121193
Ludwig W., Strunk O., Westram R., Richter L., Meier H., Yadhukumar A., et al. (2004). ARB: a software environment for sequence data. Nucleic Acid. Res. 32, 1363–1371. doi: 10.1093/nar/gkh293
Maas R. M., Verdegem M. C., Wiegertjes G. F., Schrama J. W. (2020). Carbohydrate utilisation by tilapia: a meta-analytical approach. Rev. Aquac. 12, 1851–1866. doi: 10.1111/raq.12413
Marco M. L. (2019). Is Probiotic Colonization Essential? (USA: International Scientific Association for Probiotics and Prebiotics (ISAPP).
Matos E., Dias J., Dinis M. T., Silva T. S. (2017). Sustainability vs. Quality in gilthead seabream (Sparus aurata L.) farming: are trade-offs inevitable? Rev. Aquac. 9, 388–409. doi: 10.1111/raq.12144
Michl S. C., Beyer M., Ratten J. M., Hasler M., LaRoche J., Schulz C. (2019). A diet-change modulates the previously established bacterial gut community in juvenile brown trout (Salmo trutta). Sci. Rep. 9, 2339. doi: 10.1038/s41598-019-38800-7
Michl S. C., Ratten J. M., Beyer M., Hasler M., LaRoche J., Schulz C. (2017). The malleable gut microbiome of juvenile rainbow trout (Oncorhynchus mykiss): Diet-dependent shifts of bacterial community structures. PloS One 12, e0177735. doi: 10.1371/journal.pone.0177735
Minich J. J., Poore G. D., Jantawongsri K., Johnston C., Bowie K., Bowman J., et al. (2020). Microbial ecology of Atlantic salmon (Salmo salar) hatcheries: impacts of the built environment on fish mucosal microbiota. Appl. Environ. Microbiol. 86, e00411–e00420. doi: 10.1128/AEM.00411-20
Miraglia D., Castrica M., Esposto S., Roila R., Selvaggini R., Urbani S., et al. (2021). Quality evaluation of shrimp (Parapenaeus longirostris) treated with phenolic extract from olive vegetation water during shelf-life, before and after cooking. Foods 10, 2116. doi: 10.3390/foods10092116
Mohan K., Rajan D. K., Ganesan A. R., Divya D., Johansen J., Zhang S. (2023). Chitin, chitosan and chitooligosaccharides as potential growth promoters and immunostimulants in aquaculture: A comprehensive review. Int. J. Biol. Macromol. 251, 126285. doi: 10.1016/j.ijbiomac.2023.126285
Mora-Ruiz M. D. R., Font-Verdera F., Orfila A., Rita J., Rossello-Mora R. (2016). Endophytic microbial diversity of the halophyte Arthrocnemum macrostachyum across plant compartments. FEMS Microbiol. Ecol. 92, 1–10. doi: 10.1093/femsec/fiw145
Moroni F., Naya-Català F., Piazzon M. C., Rimoldi S., Calduch-Giner J., Giardini A. (2021). The effects of nisin-producing Lactococcus lactis strain used as probiotic on gilthead sea bream (Sparus aurata) growth, gut microbiota, and transcriptional response. Front. Mar. Sci. 8. doi: 10.3389/fmars.2021.659519
Mugetti D., Pastorino P., Beltramo C., Audino T., Arillo A., Esposito G., et al. (2023). The gut microbiota of farmed and wild brook trout (Salvelinus fontinalis): evaluation of feed-related differences using 16s rRNA gene metabarcoding. Microorganisms 11, 1636. doi: 10.3390/microorganisms11071636
Navarrete P., Magne F., Araneda C., Fuentes P., Barros L., Opazo R., et al. (2012). PCR-TTGE analysis of 16S rRNA from rainbow trout (Oncorhynchus mykiss) gut microbiota reveals host-specific communities of active bacteria. PloS One 7, e31335. doi: 10.1371/journal.pone.0031335
Naya-Català F., do Vale Pereira G., Piazzon M. C., Fernandes A. M., Calduch-Giner J. A., Sitjà-Bobadilla A., et al. (2021). Cross-talk between intestinal microbiota and host gene expression in gilthead sea bream (Sparus aurata) juveniles: insights in fish feeds for increased circularity and resource utilization. Front. Physiol. 12. doi: 10.3389/fphys.2021.748265
Neu A. T., Allen E. E., Roy K. (2021). Defining and quantifying the core microbiome: challenges and prospects. Proc. Natl. Acad. Sci. U.S.A. 118, e2104429118. doi: 10.1073/pnas.2104429118
Nikouli E., Meziti A., Smeti E., Antonopoulou E., Mente E., Kormas K. A. (2021). Gut microbiota of five sympatrically farmed marine fish species in the Aegean Sea. Microb. Ecol. 81, 460–470. doi: 10.1007/s00248-020-01580-z
Niu K. M., Khosravi S., Kothari D., Lee W. D., Lim J. M., Lee B. J. (2019). Effects of dietary multi-strain probiotics supplementation in a low fishmeal diet on growth performance, nutrient utilization, proximate composition, immune parameters, and gut microbiota of juvenile olive flounder (Paralichthys olivaceus). Fish Shellfish Immunol. 93, 258–268. doi: 10.1016/j.fsi.2019.07.056
Niu K. M., Lee B. J., Kothari D., Lee W. D., Hur S. W., Lim S. G., et al. (2020). Dietary effect of low fish meal aquafeed on gut microbiota in olive flounder (Paralichthys olivaceus) at different growth stages. Microbiologyopen 9, e992. doi: 10.1002/mbo3.992
Oksanen J., Blanchet F. G., Kindt R., Legendre P., Minchin P. R., O’hara R. B. (2022). Vegan: community ecology package. R Package version 2, 6–4.
Oren A., Garrity G. M. (2021). Valid publication of the names of forty-two phyla of prokaryotes. Int. J. Syst. Evol. Microbiol. 71, 5056. doi: 10.1099/ijsem.0.005056
Pan B., Han X., Yu K., Sun H., Mu R., Lian C. A. (2023). Geographical distance, host evolutionary history and diet drive gut microbiome diversity of fish across the Yellow River. Mol. Ecol. 32, 1183–1196. doi: 10.1111/mec.16812
Panteli N., Mastoraki M., Nikouli E., Lazarina M., Antonopoulou E., Kormas K. A. (2020). Imprinting statistically sound conclusions for gut microbiota in comparative animal studies: A case study with diet and teleost fishes. Comp. Biochem. Physiol. Part D Genomics Proteomics. 36, 100738. doi: 10.1016/j.cbd.2020.100738
Payne C. J., Turnbull J. F., MacKenzie S., Crumlish M. (2022). The effect of oxytetracycline treatment on the gut microbiome community dynamics in rainbow trout (Oncorhynchus mykiss) over time. Aquaculture 560, 738559. doi: 10.1016/j.aquaculture.2022.738559
Pedrotti F. S., Davies S., Merrifield D. L., Marques M. R. F., Fraga A. P. M., Mouriño J. L. P., et al. (2015). The autochthonous microbiota of the freshwater omnivores jundiá (Rhamdia quelen) and tilapia (Oreochromis niloticus) and the effect of dietary carbohydrates. Aquac. Res. 46, 472–481. doi: 10.1111/are.12195
Piazzon M. C., Naya-Català F., Simó-Mirabet P., Picard-Sánchez A., Roig F. J., Calduch-Giner J. A., et al. (2019). Sex, age, and bacteria: how the intestinal microbiota is modulated in a protandrous hermaphrodite fish. Front. Microbiol. 10. doi: 10.3389/fmicb.2019.02512
Piccolo G., Iaconisi V., Marono S., Gasco L., Loponte R., Nizza S., et al. (2017). Effect of Tenebrio molitor larvae meal on growth performance, in vivo nutrients digestibility, somatic and marketable indexes of gilthead sea bream (Sparus aurata). Anim. Feed Sci. Technol. 226, 12–20. doi: 10.1016/j.anifeedsci.2017.02.007
Porcino N., Genovese L. (2022). Review on alternative meals for gilthead seabream, Sparus aurata. Aquac. Res. 53, 2109–2145. doi: 10.1111/are.15770
Pruesse E., Peplies J., Glöckner F. O. (2012). SINA: Accurate high-throughput multiple sequence alignment of ribosomal RNA genes. Bioinformatics 28, 1823–1829. doi: 10.1093/bioinformatics/bts252
Quast C., Pruesse E., Yilmaz P., Gerken J., Schweer T., Yarza P., et al. (2013). The SILVA ribosomal RNA gene database project: improved data processing and web-based tools. Nucleic Acids Res. 41, D590–D596. doi: 10.1093/nar/gks1219
Quercia S., Candela M., Giuliani C., Turroni S., Luiselli D., Rampelli S., et al. (2014). From lifetime to evolution: timescales of human gut microbiota adaptation. Front. Microbiol. 5. doi: 10.3389/fmicb.2014.00587
Raimundo I., Silva R., Meunier L., Valente S. M., Lago-Lestón A., Keller-Costa T., et al. (2021). Functional metagenomics reveals differential chitin degradation and utilization features across free-living and host-associated marine microbiomes. Microbiome 9, 1–18. doi: 10.1186/s40168-020-00970-2
Ramírez C., Romero J. (2017). Fine flounder (Paralichthys adspersus) microbiome showed important differences between wild and reared specimens. Front. Microbiol. 8. doi: 10.3389/fmicb.2017.00271
R Core Team (2020). R: A language and environment for statistical computing (Vienna, Austria: R. Foundation for Statistical Computing).
Rimoldi S., Antonini M., Gasco L., Moroni F., Terova G. (2021). Intestinal microbial communities of rainbow trout (Oncorhynchus mykiss) may be improved by feeding a Hermetia illucens meal/low-fishmeal diet. Fish Physiol. Biochem. 47, 365–380. doi: 10.1007/s10695-020-00918-1
Rimoldi S., Ceccotti C., Brambilla F., Faccenda F., Antonini M., Terova G. (2023). Potential of shrimp waste meal and insect exuviae as sustainable sources of chitin for fish feeds. Aquaculture 567, 739256. doi: 10.1016/j.aquaculture.2023.739256
Rimoldi S., Terova G., Ascione C., Giannico R., Brambilla F. (2018a). Next generation sequencing for gut microbiome characterization in rainbow trout (Oncorhynchus mykiss) fed animal by-product meals as an alternative to fishmeal protein sources. PloS One 13, e0193652. doi: 10.1371/journal.pone.0193652
Rimoldi S., Gliozheni E., Ascione C., Gini E., Terova G. (2018b). Effect of a specific composition of short-and medium-chain fatty acid 1-Monoglycerides on growth performances and gut microbiota of gilthead sea bream (Sparus aurata). PeerJ 6, e5355. doi: 10.7717/peerj.5355
Rimoldi S., Torrecillas S., Montero D., Gini E., Makol A., Valdenegro V. V., et al. (2020). Assessment of dietary supplementation with galactomannan oligosaccharides and phytogenics on gut microbiota of European sea bass (Dicentrarchus labrax) fed low fishmeal and fish oil based diet. PloS One 15, e0231494. doi: 10.1371/journal.pone.0231494
Ringø E., Zhou Z., Olsen R. E., Song S. K. (2012). Use of chitin and krill in aquaculture–the effect on gut microbiota and the immune system: a review. Aquac. Nutr. 18, 117–131. doi: 10.1111/j.1365-2095.2011.00919.x
Ringø E. Z., Zhou Z., Vecino J. G., Wadsworth S., Romero J., Krogdahl Å. (2016). Effect of dietary components on the gut microbiota of aquatic animals. A never-ending story? Aquac. Nutr. 22, 219–282. doi: 10.1111/anu.12346
Roeselers G., Mittge E. K., Stephens W. Z., Parichy D. M., Cavanaugh C. M., Guillemin K., et al. (2011). Evidence for a core gut microbiota in the zebrafish. ISME J. 5, 1595–1608. doi: 10.1038/ismej.2011.38
Romero J., Díaz O., Miranda C. D., Rojas R. (2022). Red cusk-eel (Genypterus Chilensis) gut microbiota description of wild and aquaculture specimens. Microorganisms 10, 105. doi: 10.3390/microorganisms10010105
Rudi K., Angell I. L., Pope P. B., Vik J. O., Sandve S. R., Snipen L. G. (2018). Stable core gut microbiota across the freshwater-to-saltwater transition for farmed Atlantic salmon. Appl. Environ. Microbiol. 84, e01974–e01917. doi: 10.1128/AEM.01974-17
Ruiz A., Gisbert E., Andree K. B. (2024). Impact of the diet in the gut microbiota after an inter-species microbial transplantation in fish. Scient. Rep. 14, 4007. doi: 10.1038/s41598-024-54519-6
Ruzauskas M., Armalytė J., Lastauskienė E., Šiugždinienė R., Klimienė I., Mockeliūnas R., et al. (2021). Microbial and antimicrobial resistance profiles of microbiota in common carps (Cyprinus carpio) from aquacultured and wild fish populations. Animals 11, 929. doi: 10.3390/ani11040929
Schoultz I., Keita Å.V. (2019). Cellular and molecular therapeutic targets in inflammatory bowel disease—focusing on intestinal barrier function. Cells 8, 193. doi: 10.3390/cells8020193
Sherif A. H., Gouda M. Y., Naena N. A., Ali A. H. (2020). Alternate weekly exchanges of feeding regime affect the diversity of intestinal microbiota and immune status of Nile tilapia Oreochromis niloticus. Aquac. Res. 51, 4327–4339. doi: 10.1111/are.14778
Silva F. C. D. P., Nicoli J. R., Zambonino-Infante J. L., Kaushik S., Gatesoupe F. J. (2011). Influence of the diet on the microbial diversity of faecal and gastrointestinal contents in gilthead sea bream (Sparus aurata) and intestinal contents in goldfish (Carassius auratus). FEMS Microbiol. Ecol. 78, 285–296. doi: 10.1111/j.1574-6941.2011.01155.x
Soriano E. L., Ramírez D. T., Araujo D. R., Gómez-Gil B., Castro L. I., Sánchez C. G. (2018). Effect of temperature and dietary lipid proportion on gut microbiota in yellowtail kingfish Seriola lalandi juveniles. Aquaculture 497, 269–277. doi: 10.1016/j.aquaculture.2018.07.065
Viver T., Cifuentes A., Díaz S., Rodríguez-Valdecantos G., González B., Antón J., et al. (2015). Diversity of extremely halophilic cultivable prokaryotes in Mediterranean, Atlantic and Pacific solar salterns: evidence that unexplored sites constitute sources of cultivable novelty. Syst. Appl. Microbiol. 38, 266–275. doi: 10.1016/j.syapm.2015.02.002
Viver T., Ruiz A., Bertomeu E., Martorell-Barceló M., Urdiain M., Grau A., et al. (2023). Food determines ephemerous and non-stable gut microbiome communities in juvenile wild and farmed Mediterranean fish. Sci. Total. Environ. 889, 164080. doi: 10.1016/j.scitotenv.2023.164080
Wang J., Li S., Sun Z., Lu C., Zhao R., Liu T., et al. (2024). Comparative study of immune responses and intestinal microbiota in the gut-liver axis between wild and farmed pike perch (Sander lucioperca). Front. Immunol. 15. doi: 10.3389/fimmu.2024.1473686
Webster T. M. U., Consuegra S., de Leaniz C. G. (2021). Early life stress causes persistent impacts on the microbiome of Atlantic salmon. Comp. Biochem. Physiol. Part D Genomics Proteomics. 40, 100888. doi: 10.1016/j.cbd.2021.100888
Wickham H. (2016). ggplot2: Elegant Graphics for Data Analysis (Springer). doi: 10.1007/978-0-387-98141-3
Wilkes Walburn J., Wemheuer B., Thomas T., Copeland E., O'Connor W., Booth M., et al. (2019). Diet and diet-associated bacteria shape early microbiome development in Yellowtail Kingfish (Seriola lalandi). Microb. Biotechnol. 12, 275–288. doi: 10.1111/1751-7915.13323
Wilson E., Ramage F. J., Wever K. E., Sena E. S., Macleod M. R., Currie G. L. (2023). Designing, conducting, and reporting reproducible animal experiments. J. Endocrinol. 258, e220330. doi: 10.1530/JOE-22-0330
Wu S., Gao T., Zheng Y., Wang W., Cheng Y., Wang G. (2010). Microbial diversity of intestinal contents and mucus in yellow catfish (Pelteobagrus fulvidraco). Aquaculture 303, 1–7. doi: 10.1016/j.aquaculture.2009.12.025
Xie X., Wang J., Guan Y., Xing S., Liang X., Xue M., et al. (2022). Cottonseed protein concentrate as fishmeal alternative for largemouth bass (Micropterus salmoides) supplemented a yeast-based paraprobiotic: Effects on growth performance, gut health and microbiome. Aquaculture 551, 737898. doi: 10.1016/j.aquaculture.2022.737898
Yin X., Ji S., Duan C., Tian P., Ju S., Yan H., et al. (2021). Age-related changes in the ruminal microbiota and their relationship with rumen fermentation in lambs. Front. Microbiol. 12. doi: 10.3389/fmicb.2021.679135
Yu J., Kang M. J., Kim Y. J., Park M. J., Lim J. K., Noh C. H., et al. (2021). Comparison of intestine microbiota between wild and farmed Korean rockfish, Sebastes schlegelii. Ocean Sci. J. 56, 297–306. doi: 10.1007/s12601-021-00022-2
Zemanova M. A. (2020). Towards more compassionate wildlife research through the 3Rs principles: moving from invasive to non-invasive methods. Wildl. Biol. 2020, wlb.00623. doi: 10.2981/wlb.00607
Zeng A., Tan K., Gong P., Lei P., Guo Z., Wang S. (2020). Correlation of microbiota in the gut of fish species and water. 3 Biotech. 10, 1–10. doi: 10.1007/s13205-020-02461-5
Zhang J., Dong Y., Song K., Wang L., Li X., Lu K., et al. (2022). Substituting fish meal with a bacteria protein (Clostridium autoethanogenum protein) derived from industrial-scale gas fermentation: effects on growth and gut health of juvenile large yellow croakers (Larimichthys crocea). Fishes 7, 228. doi: 10.3390/fishes7050228
Keywords: diet change, wild-type diet, commercial diet, gut microbiota, time course, core microbiota, aquafeeds
Citation: Ruiz A, Alós J, Gisbert E, Furones D and Viver T (2024) Long-term adaptation to dietary shifts of gut microbiota in gilthead seabream (Sparus aurata). Front. Mar. Sci. 11:1498892. doi: 10.3389/fmars.2024.1498892
Received: 19 September 2024; Accepted: 30 November 2024;
Published: 17 December 2024.
Edited by:
Antonio Luna-González, National Polytechnic Institute (IPN), MexicoReviewed by:
Eleni Nikouli, University of Thessaly, GreeceCarlos Alfonso Alvarez-González, Universidad Juárez Autónoma de Tabasco, Mexico
Copyright © 2024 Ruiz, Alós, Gisbert, Furones and Viver. This is an open-access article distributed under the terms of the Creative Commons Attribution License (CC BY). The use, distribution or reproduction in other forums is permitted, provided the original author(s) and the copyright owner(s) are credited and that the original publication in this journal is cited, in accordance with accepted academic practice. No use, distribution or reproduction is permitted which does not comply with these terms.
*Correspondence: Tomeu Viver, dHZpdmVyQGltZWRlYS51aWItY3NpYy5lcw==; Alberto Ruiz, YWxiZXJ0by5ydWl6QGlydGEuY2F0