- 1Unité d’Océanographie Chimique, Université de Liège, Liège, Belgium
- 2Department of Geosciences, Environment and Society, Université Libre de Bruxelles, Bruxelles, Belgium
- 3Organic Isotope Geochemistry group, Max Planck Institute for Chemistry, Mainz, Germany
- 4Institute of Earth Sciences, University of Lausanne, Lausanne, Switzerland
- 5Flanders Marine Institute (VLIZ), Oostende, Belgium
- 6Alfred Wegener Institute, Helmholtz Centre for Polar and Marine Research, Bremerhaven, Germany
Previous studies have reported an accumulation of nitrous oxide (N2O) on shallow continental shelves of the western Arctic Ocean. In this study, we sampled seawater profiles for N2O measurements in the eastern Arctic shelves, in the North Kara Sea, in the context of the Arctic Century Expedition. Despite some variability in the vertical distribution, we typically observe an accumulation of N2O in shelf bottom waters, which correlates with a fixed nitrogen (N) deficit. Longer residence times on the shelf promote greater N2O enrichment and a larger fixed N deficit. These observations point towards N2O production at depth, linked to benthic denitrification processes that are intensified on productive shelve areas. However, in surface waters, physical processes – i.e. temperature-dependent solubility and air-sea exchange – emerge as the main factor controlling N2O concentrations. We observe low saturations of 80% at the surface of open ocean stations influenced by water that has previously flowed beneath sea ice. Arctic surface water becomes undersaturated due to cooling and remains undersaturated due to limited air-sea exchange. River supply does not exert a discernable influence on N2O concentrations of the studied area. This study reveals the potential of the Arctic Siberian shelves as a sink of atmospheric N2O during the summer.
1 Introduction
Nitrous oxide (N2O) is a potent greenhouse gas (IPCC, 2023), and is the dominant ozone-depleting substance in the stratosphere (Ravishankara et al., 2009). N2O atmospheric concentrations have increased by > 23% since 1750, with a significant increase in the last 4 decades (IPCC, 2023). Anthropogenic N2O sources account for one third of the total emissions, but are the primary drivers of the observed increase in atmospheric N2O levels (Tian et al., 2024). Oceans naturally emit 4.7 Tg N y-1, which represents 26% of the total N2O emissions (or 40% of the natural emissions) (Tian et al., 2024). Oceanic N2O emissions predominantly occur in the tropics and in coastal upwelling systems (Yang et al., 2020). More accurate estimates of N2O emissions from ocean sources and their response to climate change, are hindered by a general lack of observational data from large areas of the ocean, in particular polar environments (Yang et al., 2020; Tian et al., 2024).
N2O is primarily produced via two microbial processes, namely nitrification and denitrification. These two processes are inherently linked to the production and remineralization of organic matter, and nitrogen cycling. Nitrification is a type of chemoautotrophy that takes place when ammonium (NH4+) is released during the decomposition of organic matter (Ward, 2008; Tang et al., 2023). It is the aerobic, two-step oxidation of ammonium (NH4+) to nitrite (NO2-) and then nitrate (NO3-). N2O is considered as a by-product of the oxidation of NH4+ to NO2-. The positive correlation between apparent oxygen utilization (AOU) and N2O concentration anomalies (i.e., ΔN2O) suggests that most of the N2O in the ocean is produced by nitrification processes (Cohen and Gordon, 1979; Bange, 2008). Denitrification, on the other hand, takes place under anaerobic conditions. Denitrification relates to the reduction of NO3- to dinitrogen (N2), with N2O being both, a product and an intermediate reactant of the reaction chain (Bothe et al., 2000). Denitrification is the major sink of fixed nitrogen (N) in the ocean by converting fixed N to N2 (Brandes and Devol, 2002; Bianchi et al., 2012).
Continental shelve regions represent an important source of N2O to the atmosphere. They represent 9% of the total ocean area (Harris et al., 2014) but contribute to 1.2 Tg N y-1 – i.e., 26% of the oceanic N2O emissions – to the atmosphere (Tian et al., 2024). According to global models, a large fraction of the denitrification occurs in continental shelf sediments (Seitzinger et al., 2006), where sedimentary organic carbon flux is highest (Bianchi et al., 2012) and pelagic and benthic processes are tightly coupled (Rowe et al., 1975; Sun et al., 2021). Therefore, continental shelves represent a major sink of fixed N, and a potentially important source of N2O to the atmosphere (Tian et al., 2024).
Half of the Arctic Ocean is characterized by vast, shallow shelf areas (Harris et al., 2014). It is estimated that the Arctic continental shelves account for 4% to 13% of the fixed N loss from the ocean (Devol et al., 1997; Chang and Devol, 2009; McTigue et al., 2016). Most studies, reporting N2O concentrations, are localized in the western Arctic Ocean (Hirota et al., 2009; Kitidis et al., 2010; Zhan et al., 2015, 2021; Zhang et al., 2015; Fenwick et al., 2017; Wu et al., 2017; Heo et al., 2021; Toyoda et al., 2021; Liu et al., 2022, 2024; Manning et al., 2022; Schuler and Tortell, 2023), and data from the eastern Arctic Ocean remain scarce. Observations suggest that benthic denitrification is the main process leading to N2O production over the shelves (Hirota et al., 2009; Zhan et al., 2015; Fenwick et al., 2017; Toyoda et al., 2021; Manning et al., 2022), while the observed concentrations in the intermediate and deep waters of the Arctic Ocean typically relate to a combination of nitrification and poor-ventilation rates of the Arctic waters that were exposed to past atmospheric concentrations (Zhang et al., 2015; Zhan et al., 2016; Fenwick et al., 2017; Heo et al., 2021; Toyoda et al., 2021). To the best of our knowledge, Wild et al. (2023) is the sole study reporting N2O concentrations in the eastern Arctic. They reported aerobic or anoxic N2O consumption in the water column or in particle microsites, respectively, rather than a sedimentary source.
Rivers contribute to a large amount of organic material and land-derived nutrient input (Opsahl et al., 1999; Holmes et al., 2012; McClelland et al., 2016), sustaining potentially one third of the annual primary production in the Arctic Ocean (Terhaar et al., 2021). The Kara Sea receives substantial river discharge accounting for more than one third of the total riverine freshwater input to the Arctic Ocean (Aagaard and Carmack, 1989). The West Siberian lowlands contain the most extensive peatlands and substantial fraction of the global terrestrial carbon stocks (Sheng et al., 2004; Smith et al., 2004). Arctic warming accelerates permafrost thaw and is expected to promote higher inputs of particulate and dissolved nitrogen from rivers into the ocean (Frey et al., 2007). Land-derived nitrogen input can serve as a substrate for the marine nitrogen cycle, potentially enhancing biogeochemical processes and contributing to increased N2O production.
In the present study, we provide full-depth water column profiles of N2O in the northern Kara Sea. The samples were collected in summer during the sea-ice melting season, in the context of the Arctic Century Expedition. N2O shows heterogenous concentrations, with contrasting results between open ocean and shelves areas, or between ice-free and ice-covered stations, underscoring the importance of water circulation and provenance on N2O inventories. We show that shelf bottom waters are enriched in N2O, likely due to benthic denitrification, while surface waters are near equilibrium with the atmosphere with N2O concentrations primarily being regulated by sea water temperature. This contrasts with ~80% saturation observed in surface waters of open ocean area, influenced by water that had previously flowed beneath sea ice. This undersaturation is likely driven by combination of cooling, limited air-sea exchange, and vertical mixing with deeper undersaturated water masses.
2 Materials and methods
2.1 Study site
The Kara Sea, like most eastern Arctic marginal seas, is distinguished by its extensive and shallow continental shelf, and is seasonally covered by sea ice (Figures 1, 2). Perpendicular to the shelf break, the continental shelf exhibits two distinct troughs: the St. Anna Trough to the west, reaching a maximum depth of 620 m, and the Voronin Trough to the east, with a maximum depth of 450 m (Figure 1). The overall circulation in the Kara Sea is characterized by a semi-cyclonic pattern. Waters of Atlantic origin enter the Kara Sea via the Fram Strait Branch Water (FSBW) from the northwestern side of the St. Anna Trough, flowing southwards. Simultaneously, the Barents Sea Branch Water (BSBW) enters the western side of the St. Anna Trough, flowing northwards (Figure 1). The area also receives substantial river discharge from the Ob and Yenisei, accounting for approximately 40% of the total river runoff in the Arctic Ocean (Aagaard and Carmack, 1989; Makkaveev et al., 2015). The resulting river plume typically flows eastward along the Siberian coast (Figure 1) (Janout et al., 2020).
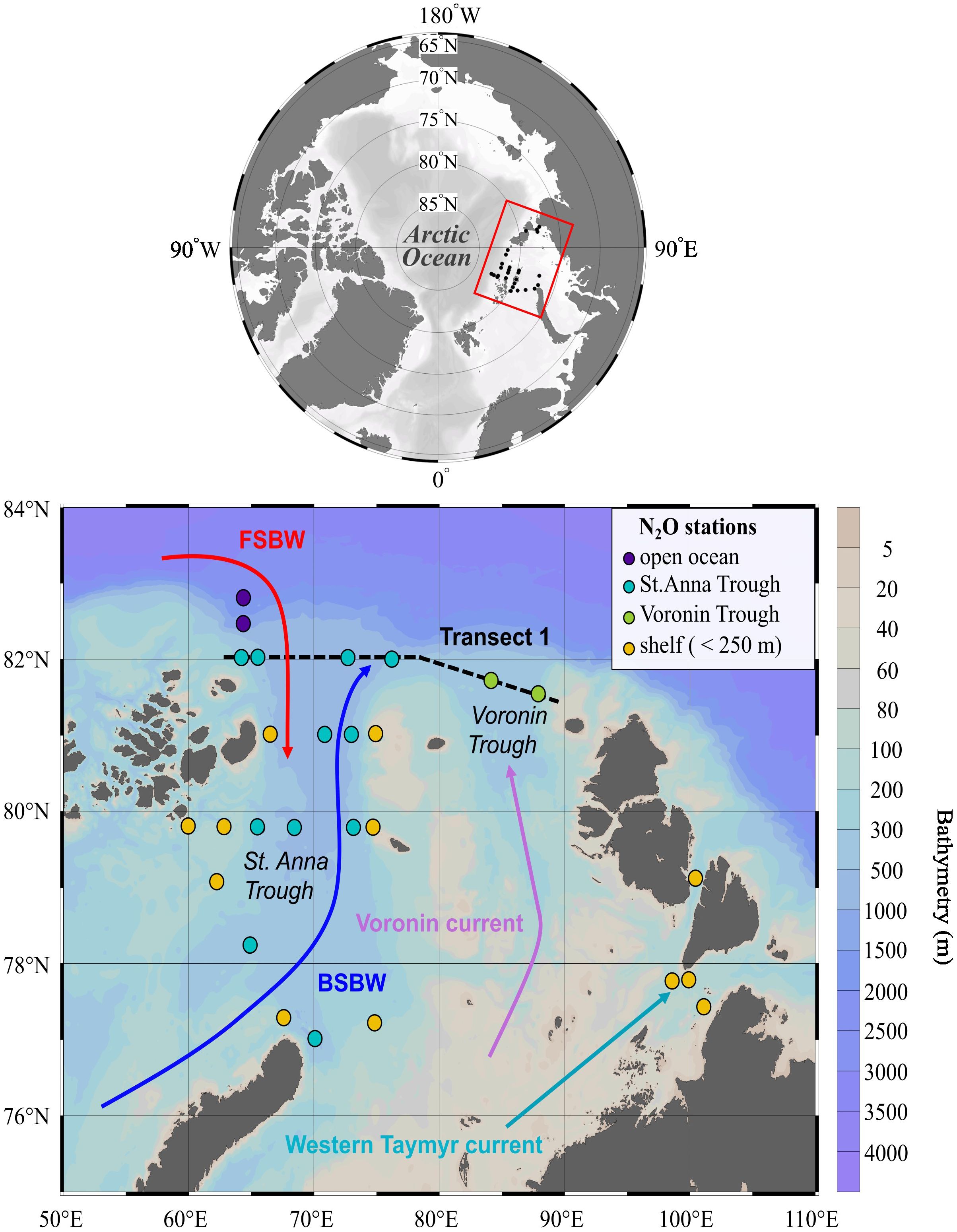
Figure 1. N2O sampling stations in circles, categorized by location and depth. Open ocean stations (in dark blue) are beyond the continental shelf (>2000 m depth), shallow shelf stations (in yellow) have a depth <250 m, and St. Anna (in light blue) and Voronin Trough stations (in green) are on the continental shelf (>250 m depth). Water circulation is indicated by colored arrays representing the Fram Strait Branch Water (FSBW), the Barents Sea Branch Water (BSBW), the Western Taymyr and the Voronin current. This circulation is further supported by the geostrophic current field shown in Figure 2. Squares on transect 1 represent additional profiles of temperature (white squares) and nutrients (black filled squares).
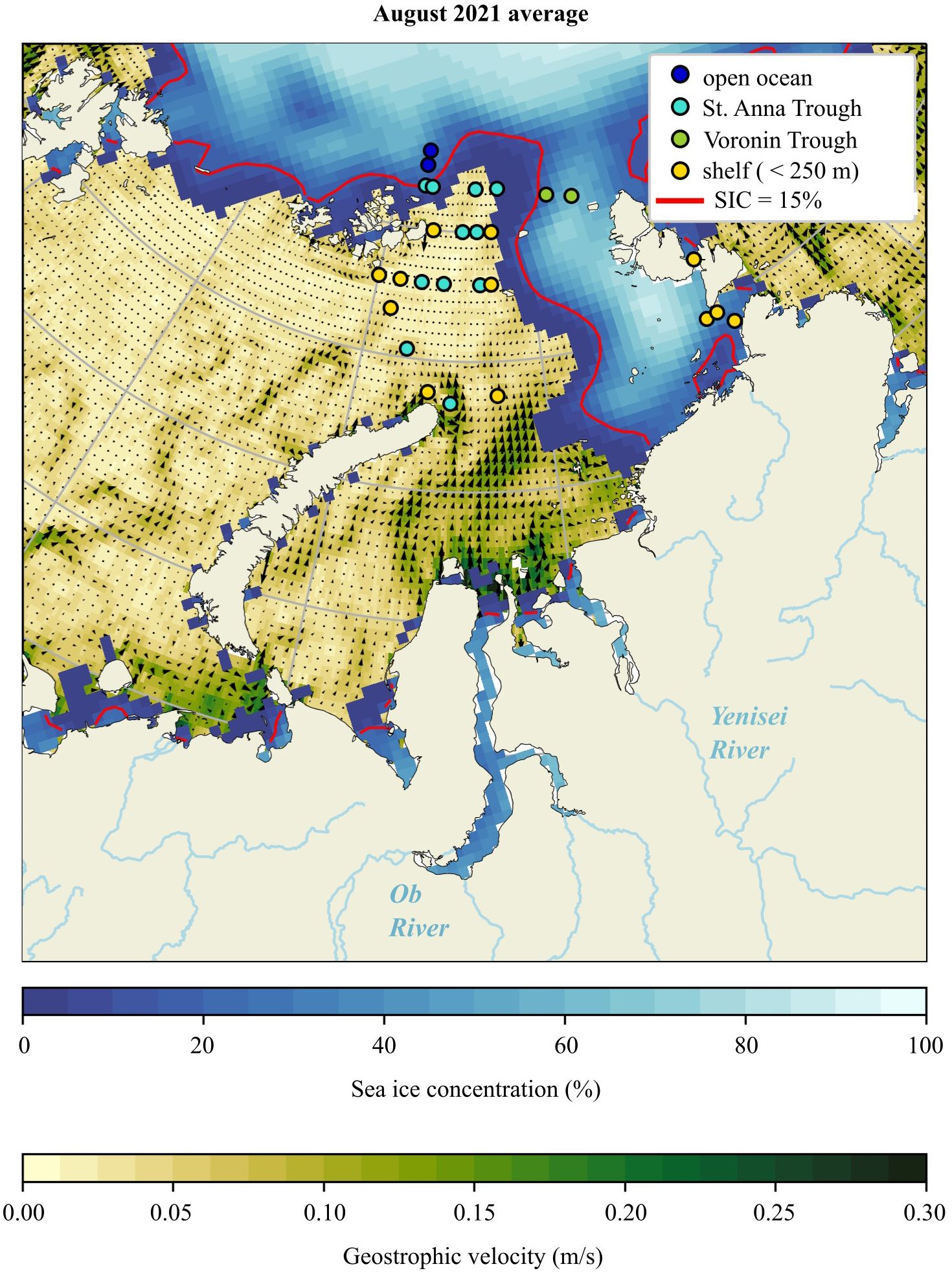
Figure 2. Sea surface current. Monthly average of the geostrophic velocity and the sea ice concentration for August 2021. Data is available on the Copernicus Marine Service for geostrophic velocity (doi:10.48670/moi-00145) and sea ice concentration (doi: 10.48670/moi-00136).
2.2 Sampling
Samples were collected as part of the Arctic Century Expedition, which took place from August 5th to September 6th, 2021, aboard RV Akademik Tryoshnikov. A total of 27 stations were sampled for full-depth profile measurements of N2O concentrations (Figure 1).
During the expedition, physical and biogeochemical properties were also investigated, including salinity, temperature, nutrient, and dissolved oxygen (O2) concentrations. Salinity and temperature data were collected using a CTD equipped with sensors for conductivity, temperature, and pressure (SBE911+) as described in Hölemann et al. (2022) and (Gangnus et al., 2022) for water bottle samples. To determine the mixed layer depth, we employed the threshold method with a finite-density difference criterion (0.03 kg m-3) relative to a near-surface reference value (de Boyer Montégut et al., 2004). Dissolved O2 was measured onboard via a standard Winkler titration using a Metrohm 916 TiTouch automatic titrator and handheld titrator (BRAND1) (Gangnus et al., 2022). Samples for nutrient analysis were stored frozen (-20°C) in MQ-cleaned HDPE 60ml bottles until their analysis at MPIC. Nutrient concentration (nitrate, NO3-, nitrite, NO2-, ammonium, NH4+, and phosphate, PO43-) were measured using standard colorimetric methods (Hydes et al., 2010) on board for NH4+ (Gangnus et al., 2022) and in home laboratory on frozen sample for NO3-, NO2-, PO43- (Muller et al., 2024). We used N* concentration to estimate the deficit in fixed N (i.e., biologically available nitrogen) in comparison to phosphate, according to N* = DIN – 16 * PO43- + 2.9 (Gruber and Sarmiento, 1997), with DIN (i.e., dissolved inorganic nitrogen) = NO3- + NO2- + NH4+.
2.3 N2O concentration
N2O samples were collected from the Niskin bottles using a Tygon® tube and transferred into borosilicate 60 ml serum bottles. Samples were poisoned by introducing 60 μL saturated mercury chloride (HgCl2) solution. The bottles were sealed with isobutyl stoppers and securely capped with aluminum crimps. The bottles were then kept in the dark at room temperature until analysis at home laboratory. Measurements of N2O concentration (Muller et al., 2024) were conducted using the headspace technique described by Weiss (1981) and Upstill-Goddard et al. (1996). Briefly, approximately 25 mL of the sea water sample was replaced by ultra-pure N gas (AirLiquide Belgium®, Alphagaz 2, N2 ≥ 99.9999%), followed by manual agitation and overnight equilibration in a water bath at room temperature. A 12 mL extraction from the headspace was injected into an SRI Instrument Model 8610C Gas Chromatograph with Electron Capture Detector, for analysis. The calibration was performed using precision gas mixtures containing N2O, CH4, CO2, and N2, provided by AirLiquide Belgium®. These calibration standards bracketed the sample concentrations, with a mixing ratio of 0.19 ± 0.02 ppm and 0.94 ± 0.09 ppm. Measurements derived from a 12-replicate-sampling of Arctic waters showed a precision of ± 0.5 nM (1σ) and ± 4% (1 relative standard deviation). The accuracy of the ECD was tested using an internal standard with a N2O concentration of 0.55 ± 0.09 ppm (Air Liquide) and we report a difference of 3% with the certified value which is within the bracketed error of the internal standard (that is at 10%).
The N2O saturation is estimated using the following equation:
where Cobs represents the observed concentration of N2O, while Ceq represents the theoretical equilibrium concentration of N2O in sea water. The determination of the latter is conducted through the application of the Henry’s Law, as formulated by Weiss and Price (1980), to compute the equilibrium concentration of N2O, based on the specific temperature and salinity of sea water (i.e., the solubility of N2O in seawater). The mean atmospheric N2O mixing ratios were obtained from the discrete dataset at Ny-Alesund (ZUP), Svalbard – from July 1st, 2021 to September 30th, 2021 (NOAA 2024). Error propagation, considering the precision in N2O concentration measurements, results in a standard deviation of 3% for N2O saturation (1σ).
The N2O concentration anomaly (ΔN2O) was calculated using the following equation:
2.4 Air-sea fluxes
Sea-air flux calculation was performed using the gas transfer velocity parametrization of Ho et al. (2006), with Schmidt numbers for N2O following Wanninkhof (2014). Positive values represent a flux from the sea to the air (source) and negative values a flux from the air to the sea (sink). In situ wind speed was measured aboard R/V Akademik Tryoshnikov with two 2-D sonic anemometers (models W S425 and WMT702), which were operated as part of an automated weather station (AWS; model AWS420, Vaisala) (Thurnherr et al., 2024). Wind speeds were converted from 30 m to 10 m height using the equation from Hsu et al. (1994):
A correction factor was added to areas partially covered by sea ice. Sea-air flux estimates were scaled to the fraction of open water within the sea ice area (Butterworth and Miller, 2016):
where fice is the sea ice fraction derived from OSI SAF (doi: 10.48670/moi-00136), Kcoeff is the gas transfer velocity, and ΔpN2O is the partial pressure difference between the seawater and the atmosphere.
2.5 Satellite-based datasets
This study uses daily satellite-based absolute geostrophic velocity fields, gridded onto a regular 0.25° × 0.25° grid. The data product ‘Global Ocean Gridded L 4 Sea Surface Heights And Derived Variables Reprocessed Copernicus Climate Service’, is produced and provided by the Copernicus Climate Change Service (C3S). The geostrophic velocities are derived from altimetry sea level records obtained from a stable two-satellite constellation, ensuring the stability and homogeneity of the dataset (doi:10.48670/moi-00145). For the period of the cruise, monthly means were calculated from the daily data (Figure 2).
In addition, satellite-based daily sea ice concentration fields provided by the European Organization for the Exploitation of Meteorological Satellites (EUMETSAT) are incorporated in this study (Figure 2). Specifically, the OSI-430-a product produced by the Ocean and Sea Ice Satellite Application Facility (OSI SAF), covering the period from 2021 to the present. This product calculates sea ice concentration using atmospherically corrected Passive Microwave (PMW) brightness temperatures derived from the Scanning Multichannel Microwave Radiometer (SMMR), Special Sensor Microwave/Imager (SSM/I), and Special Sensor Microwave Imager/Sounder (SSMIS). The data, classified as Level 4 and gridded at a spatial resolution of 25 × 25 km, are accessible via the Copernicus Marine Service (CMEMS) under the full title ‘Global Ocean Sea Ice Concentration Time Series REPROCESSED (OSI-SAF)’ doi: 10.48670/moi-00136).
Furthermore, this study utilizes daily sea surface salinity (SSS) and sea surface temperature (SST) data from the TOPAZ5 Arctic Ocean reanalysis system. The TOPAZ5 product, identified as ‘Arctic Ocean Physics Analysis and Forecast’ (doi:10.48670/moi-00016), is provided by the CMEMS. The operational TOPAZ5 system employs the HYCOM model and a 100-member Ensemble Kalman Filter (EnKF) assimilation scheme. The TOPAZ5 product for SSS and SST has a spatial resolution of 6 x 6 km.
3 Results
3.1 N2O concentration
Sea water N2O concentrations in the Kara Sea typically ranged from 11.1 nM to 18.5 nM, with a mean concentration of 15.1 ± 1.3 nM (Figure 3A). Despite the heterogeneity of the data, a clear pattern emerged when the stations were categorized based on their location and depth (Figures 1, 3A). We designated stations beyond the continental shelf break (> 2000 m depth) as open ocean stations (n = 2; blue circles in Figure 1). Over the shelf area, a further distinction was made between shallow shelf stations (< 250 m; n = 12; yellow circles) and those located in the St. Anna Trough (n = 11; cyan circles) and Voronin Trough (n = 2; green circles), at > 250 m.
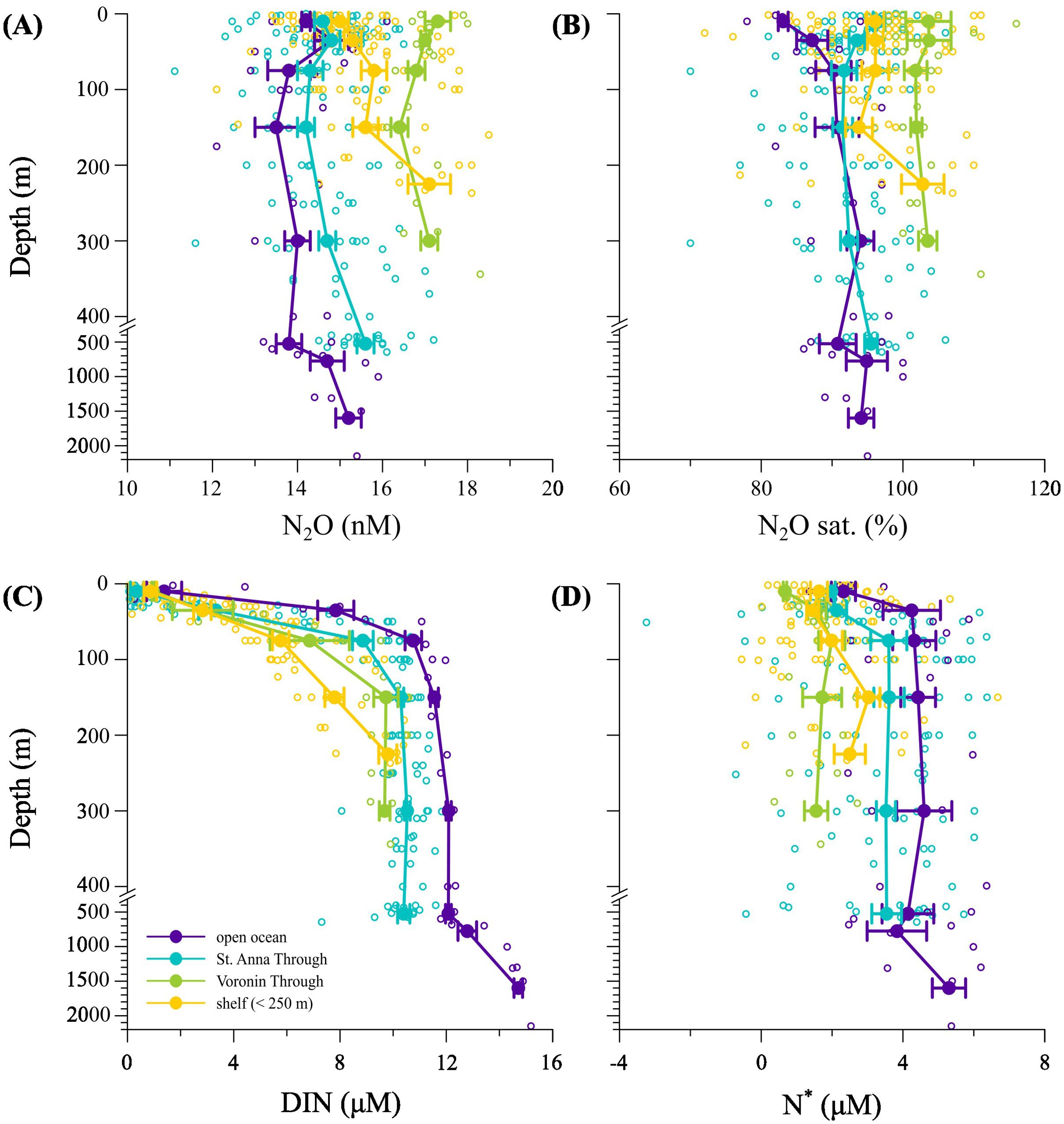
Figure 3. N2O (A), N2O saturation (B), DIN concentration (C) and N* concentration (D) vs depth. Empty circles represent the individual samples and the lines with filled circles represent the mean concentrations of each group of stations as defined in Figure 1, at specific depth ranges (0 m – 20 m; 20 m – 50 m; 50 m – 100 m; 100 m – 200 m; 200 m – 400 m; 400 m – 650 m; 650 m -1000 m; 1000 m – 2200 m). Each averaged point is calculated from at least three samples, except for the DIN surface point of the Voronin Trough (0 m – 20 m), that was calculated from two points due to lack of data. The error bars represent the standard error.
Overall, averaged N2O concentrations profiles increased from the open ocean to the shelf stations (Figure 3A). The highest concentrations were observed in the Voronin Trough, followed by the shallow shelf, with the St. Anna Trough stations exhibiting values slightly higher than those of the open ocean stations. Vertical profiles revealed a consistent rise in N2O concentration towards the bottom, with the exception of the Voronin Trough, which showed relatively constant yet higher concentrations throughout the entire water column. The increase in N2O concentrations at depth was more pronounced for the shelf stations (<250 m). However, a large scattering appeared between stations (Supplementary Figure S1) with 2 stations showing a decrease in N2O concentration with depth (stations 32 and 41; S1), 8 stations showing similar N2O concentration with depth (stations 17, 26, 38, 40, 59, 104, 109, 111) and 17 stations showing an increase in N2O concentration with depth (all the other stations). Both, the St. Anna Trough and the shallow shelf exhibited surface concentrations of 14.0 nM to 15.0 nM, as the open ocean, whereas the surface in the Voronin Trough showed N2O concentrations of 17.3 nM, i.e., in the same concentration range than shelf bottom water. Such distribution was further illustrated with one transect, located at the northern continental shelf break (Figure 4 and dashed black line in Figure 1), showing a contrast between the two throughs: N2O concentrations were higher in the Voronin Trough. In parallel to these variations in N2O concentrations, lower DIN concentrations and lower N* (i.e., higher deficit in N relative to P) concentrations appeared over the shelves and the Voronin Trough compared to the St. Anna Trough and the open ocean (Figures 3C, D).
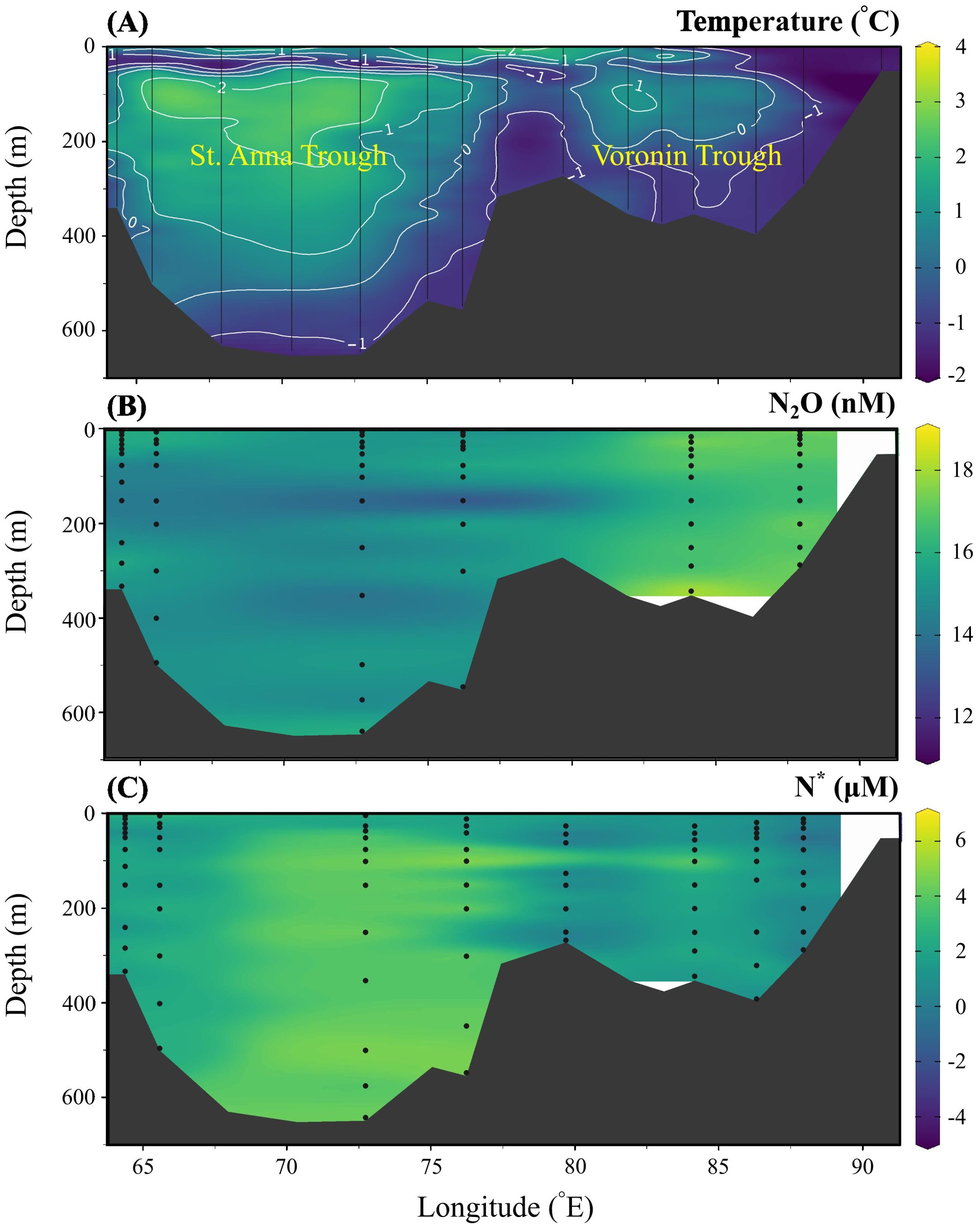
Figure 4. Depth sections of temperature (A), N2O concentration (B) and N* (C) in the northern Kara Sea (transect shown with the black dashed line in Figure 1). Temperature data comes from CTD dataset (Hölemann et al., 2022).
3.2 N2O saturation
N2O saturations within the mixed layer ranged from 78% to 111%, with a mean value of 95 ± 6% (Figure 3B). When considering the entire water column, the saturation levels ranged from 70% to 116%, with an average of 95 ± 7% (Figure 3B). The Voronin Trough exhibited a less variable profile that is oversaturated throughout the entire water column. In contrast, the shelves were undersaturated at the surface (96%), similar to the St. Anna Trough, and transition to oversaturation levels like the Voronin Trough at bottom depth (103%). The saturations levels for the St. Anna Trough first decreased, reaching levels similar to the open ocean (~90%) at a depth of 75m, and then slightly increased with depth, following the trend observed in the open ocean. The open ocean and St. Anna Trough were undersaturated along the entire water column. By contrast to all mean profiles, the open ocean revealed a saturation decrease near surface waters, and the strongest undersaturation, with a mean value of 83%.
3.3 N2O sea-air fluxes
We calculated the sea-air fluxes across the northern Kara Sea (Table 1). We further distinguished the ice-free area and the off-shelf area that shows the strongest ΔN2O gradient. Fluxes for the entire Kara Sea ranged from -4.0 μmol N2O m-2 d-1 to 1.8 μmol N2O m-2 d-1 with a mean and standard deviation of -0.6 ± 1.0 μmol N2O m-2 d-1, suggesting a minor sink for the area during summertime. Off-shelf area displayed a stronger sink with a mean value of -2.9 ± 1.5 μmol N2O m-2 d-1, despite the partial presence of sea ice, largely due to the strong saturation gradient between seawater and the atmosphere.
4 Discussion
4.1 N2O production over the bottom waters of the eastern Arctic continental shelves
Accumulations of N2O up to ~25 nM have been reported across the productive and shallow (< 100 m) continental shelves of the western Arctic Ocean, including the Bering Sea, the Chukchi Sea and the Canadian Arctic Archipelago (Hirota et al., 2009; Fenwick et al., 2017; Manning et al., 2022). These shelves are partly influenced by nutrient-rich Pacific waters entering the Arctic Ocean through the Bering Strait (Grebmeier et al., 2006). The accumulation of N2O was mostly observed in shelf bottom waters, and showed a negative correlation with N*, suggesting that benthic denitrification or benthic coupled nitrification-denitrification processes were likely the sources of N2O (Hirota et al., 2009; Fenwick et al., 2017; Toyoda et al., 2021). However, there are also findings that indicated a positive correlation between ΔN2O and AOU (Toyoda et al., 2021), suggesting that remineralization followed by nitrification, either in the reactive sedimentary layer or in the water column, may also contribute to the observed N2O accumulation (Nevison et al., 2003). Despite ongoing discussion about the precise mechanism accounting for the accumulation of N2O, it is likely that this feature resulted from the enhanced productivity on continental shelves. Enhanced productivity is usually attributed to high nutrient availability at the surface, due to enhanced upwelling and mixing, and proximity to terrestrial nutrient sources (Pabi et al., 2008; Tremblay et al., 2015; Terhaar et al., 2021). Elevated productivity leads, in turn, to elevated carbon export, abundant benthic carbon supply, and, therefore, enhanced benthic remineralization (Grebmeier et al., 2006). As a result, the water column and benthic biogeochemical cycling - including benthic denitrification and nitrification - are particularly linked and intensified on the continental shelves (Grebmeier et al., 2006; Chang and Devol, 2009; Granger et al., 2011). The N2O-enriched shelf bottom waters of the western Arctic shelves, are then transported into the central basin, resulting in a persistent peak in N2O concentrations in the subsurface waters (Zhang et al., 2015; Fenwick et al., 2017; Heo et al., 2021; Toyoda et al., 2021; Manning et al., 2022).
Despite being influenced by nutrient-poor Atlantic waters, marginal seas in the eastern Arctic Ocean are characterized by primary productivity similar to the marginal seas in the western Arctic Ocean (Arrigo and van Dijken, 2011). High primary productivity may be attributed to a higher terrigenous nutrient supply via rivers and coastal erosion (Opsahl et al., 1999; Frey et al., 2007; Terhaar et al., 2021). Strong benthic-pelagic biogeochemical couplings have indeed been documented in the region, with evidence of benthic denitrification and benthic coupled nitrification-denitrification (Anderson et al., 2011; Fripiat et al., 2018; Sun et al., 2021). Accordingly, an increase in N2O concentrations in shelf bottom waters can be expected. This is supported by our observations, where an accumulation of N2O up to approximately 18 nM was reported in the Kara Sea. This accumulation was more important for shallower areas (Figure 3A) and exhibited a negative correlation with N* when considering the average depth profiles (p-value < 0.01 and R2 = 33.4%; Figure 5A). No significant relationship (p-value ≥ 0.1) was found between N2O and DIN or between ΔN2O and AOU (Figures 5B, C), suggesting that nitrification was not the main N2O production pathway. Benthic denitrification and/or benthic coupled nitrification-denitrification appear therefore to be the primary sources of N2O in shelf bottom waters throughout the Arctic Ocean.
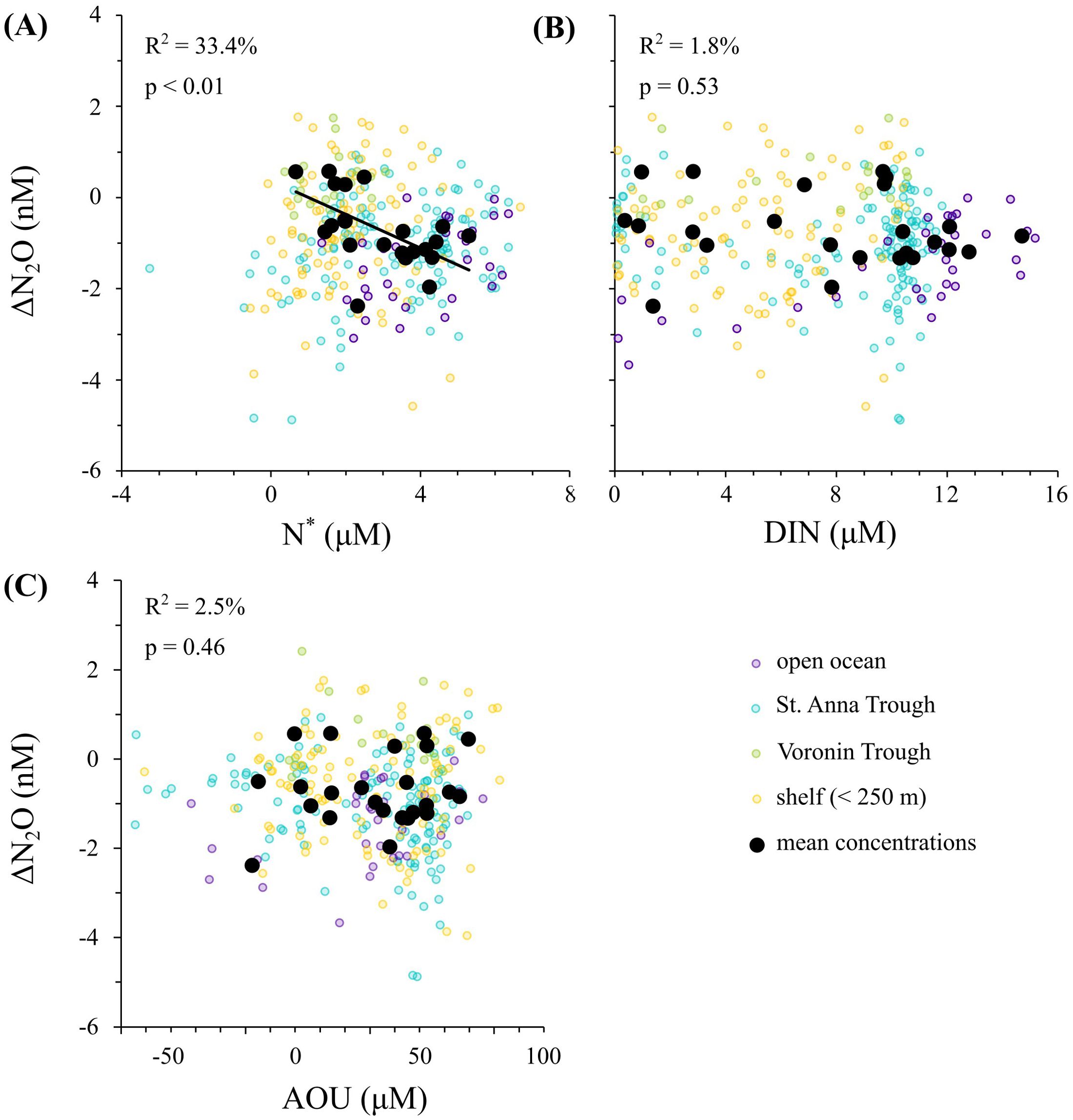
Figure 5. N2O versus N* (A) and DIN (B), and ΔN2O versus AOU (C). Small circles represent individual samples, and the big filled black circles represent the mean concentrations of the samples, as described in Figure 2. Regression lines are shown where there is significant statistical correlation between the mean concentrations.
The expression of the benthic-pelagic couplings on biogeochemical properties, including N2O concentration, of shelf bottom waters was likely correlated with the time that these waters spent over the continental shelves. Water residence times in the eastern Arctic continental shelves were estimated to range approximately between 1.5 years up to 5 years (Schlosser et al., 1994; Liu et al., 2019). The quasi-cyclonic circulation in the Kara Sea implies that shelf bottom waters in the Voronin Trough were exposed to the active sedimentary layer for the longest time (Figures 1 and 2), leading to higher concentrations of N2O, lower concentrations of DIN and a more pronounced deficit in fixed N (denoted as lower N*; Figures 3A, C, D, 4B, C).
We estimated the required time to generate the observed increase in N2O concentration from the open ocean to the Voronin Trough (i.e., 2.0 ± 1.0 nM). By considering the average sediment-water N2O flux of 110 ± 116 nmol m-2 d-1, derived from sediment-core incubations in the Kara, Laptev, and East Siberian Seas (Wild et al., 2023), we estimated this time to be 10 ± 16 years for a water column of 200 m. Large uncertainties in the sedimentary flux preclude a more thorough analysis, although they fall within the range given by the residence time of the waters on eastern Arctic continental shelves. It is interesting to note that shorter residence times of shelf bottom waters were observed over the western Arctic shelves (0.1 - 2.3 years in Liu et al., 2019). Therefore, to replicate the N2O buildup observed in the shelf bottom waters of the western Arctic shelves, a higher N2O sedimentary flux would be required.
The N2O distribution in the water column over the shelves diverged from the results reported by Wild et al. (2023), which, to the best of our knowledge, is the sole study documenting N2O concentrations across the continental shelves of the eastern Arctic Ocean. This study encompasses the South Kara Sea near river mouths, the Laptev Sea, and the East Siberian Sea. Unlike our observations, the majority of their stations do not show an accumulation of N2O in shelf bottom waters. This divergence from our data and from previous findings in the western Arctic Ocean (Hirota et al., 2009; Fenwick et al., 2017; Manning et al., 2022) led Wild et al. (2023) to attribute this disparity to the intricate dynamics of N2O. These dynamics likely involved production and consumption processes occurring within the water column, sediments, and particle microenvironments (Farías et al., 2013; Rees et al., 2016; Bianchi et al., 2018). The complexity of N2O dynamics warrants further investigation. Overall, our dataset suggests that N2O accumulation in the Arctic predominantly occurs in bottom shelf waters close to the reactive sedimentary layer, where benthic denitrification and coupled nitrification-denitrification takes place. However, the heterogeneity in our data (including some profiles showing decreasing trends with depth over the shelves) implies that N2O dynamics are more intricate than this conceptual view suggests, in agreement with Wild et al. (2023). Additional research, particularly process-based studies, is needed to fully comprehend these processes.
4.2 N2O undersaturation in intermediate and deep waters
The intermediate and deep waters of the open ocean and St. Anna Trough are undersaturated (Figure 3B). The undersaturation in intermediate and deep waters of the central Arctic Ocean has previously been reported in the literature (Zhang et al., 2015; Zhan et al., 2016; Fenwick et al., 2017; Manning et al., 2022). This undersaturation is attributed to the poor ventilation rates of the deep Arctic Ocean (Heo et al., 2021; Toyoda et al., 2021). This implies that these water masses were exposed to the atmosphere when atmospheric N2O concentrations were significantly lower than today (Schilt et al., 2014). The open ocean supplies mainly the St. Anna Trough, through FSBW (Figure 1), which is characterized by warmer intermediate water masses (i.e., temperatures > 0°C) as depicted in Figure 4A, leading to a similar undersaturation observed between the former and the latter (Figure 3B).
4.3 N2O saturation in the surface waters
In summer, surface waters over the Kara Sea shelves were on average near equilibrium or slightly undersaturated with respect to the atmosphere and might function as a minor sink (Figures 6, 7). As the equilibrium is controlled by solubility that is as function of the temperature, we observed a significant (p-value < 0.001 and R2 = 33.5%) negative correlation between N2O concentrations and temperature in the mixed surface layer (Figure 8). On a broader scale, Zhan et al. (2017) observed a similar pattern. They reported a latitudinal gradient in N2O concentrations in surface waters, aligned with the latitudinal solubility gradient resulting from the change in sea surface temperature. Specifically, N2O concentrations were lowest in the warmer tropical and subtropical waters, while the highest concentrations were found in colder polar waters (Zhan et al., 2017).
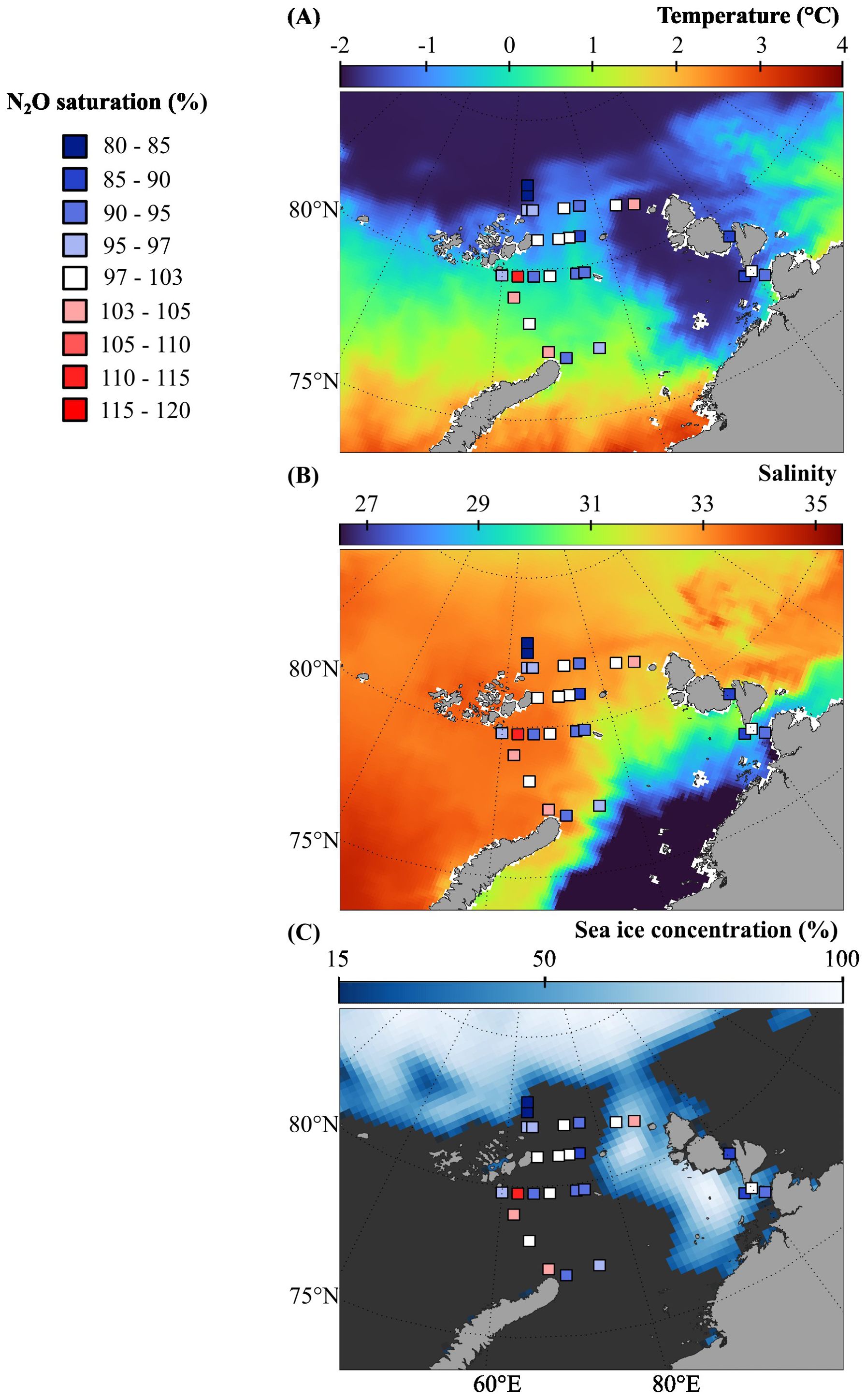
Figure 6. Distribution of N2O saturation in the sea surface waters (coloured squares). Background contours show sea surface temperature (A), sea surface salinity (B), and sea ice concentration (C) on August 21st, 2021. Ice-free ocean is represented in dark grey in panel (C). Data is available on the Copernicus Marine Service for sea surface temperature and salinity (doi:10.48670/moi-00016), and sea ice concentration (doi: 10.48670/moi-00136).
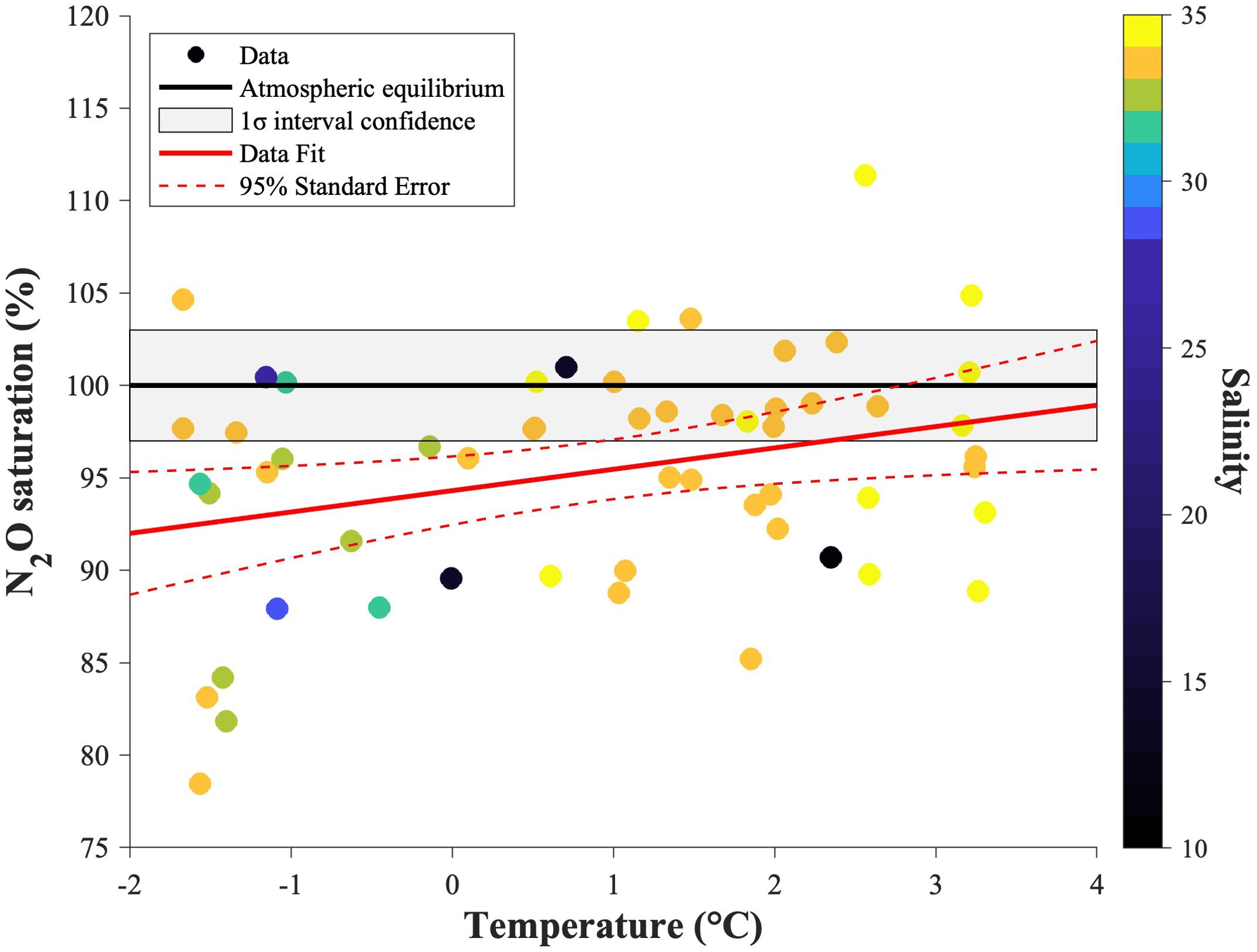
Figure 7. N2O saturations (%) in the mixed surface layer. The N2O saturation (y axis) is compared to the temperature (°C; x axis) and salinity (colorbar). The black line and grey shade represent the equilibrium state (at 100%) and saturation error (2σ), respectively. The solid and dashed red lines represent the fit of the data with the standard error, respectively. It is observed that most of the MSL is at equilibrium with the atmosphere with regard N2O. The saturation deficit is the strongest for temperatures near the freezing point. The samples with a river signal are at equilibrium or undersaturated.
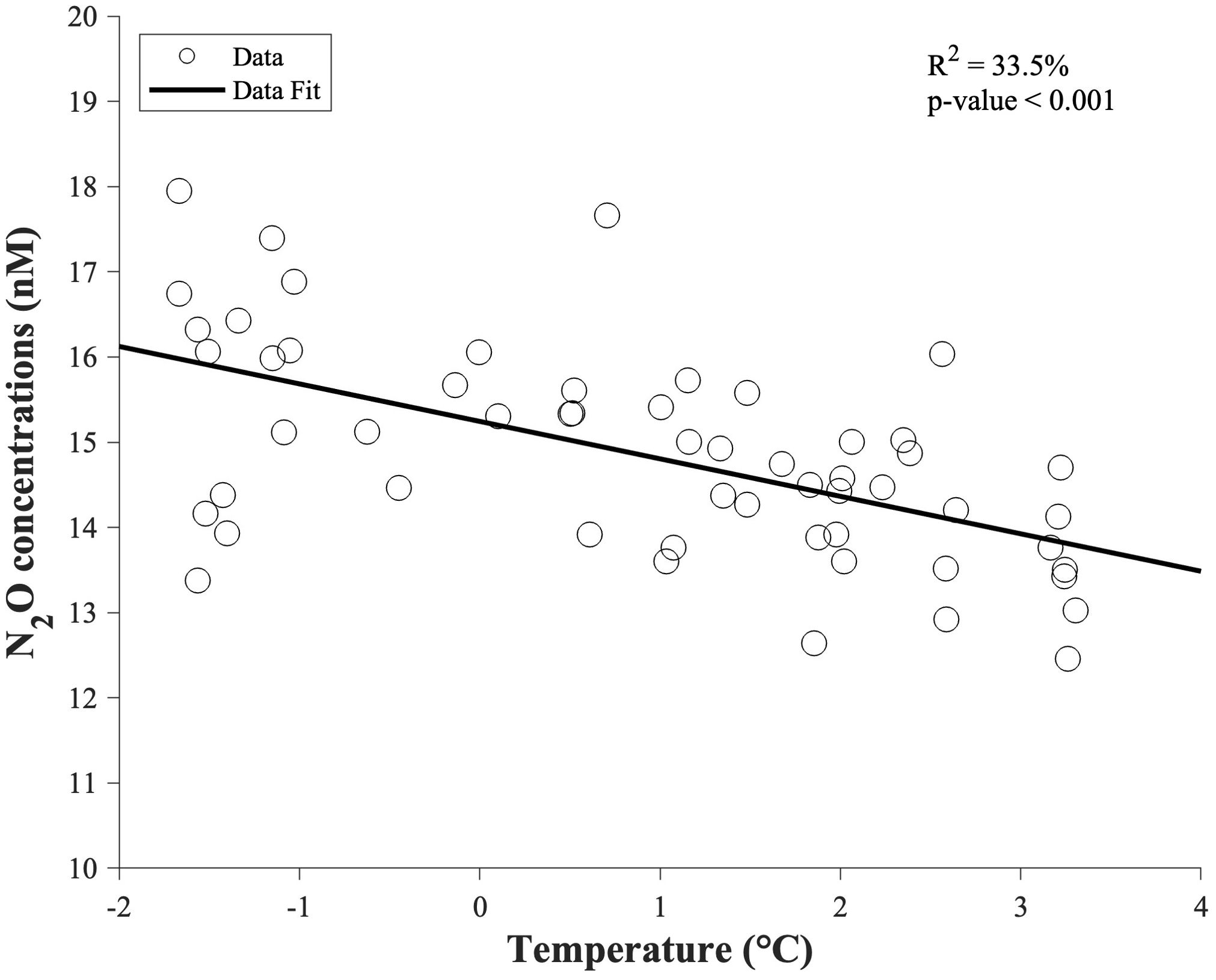
Figure 8. N2O concentrations in the mixed surface layer. The N2O concentration is compared to temperature. The regression line is shown in black.
Globally, N2O saturation (Equation 1) in the ocean is either close to equilibrium or slightly oversaturated for surface waters (Nevison et al., 2003; Zhan et al., 2017; Yang et al., 2020), resulting in a net source to the atmosphere (Tian et al., 2020, 2024; Yang et al., 2020). However, the Arctic Ocean exhibits a significant scatter in saturation levels compared to other areas (Zhan et al., 2017). The scattered distribution likely stems from the extensive and shallow continental shelves that span half of the Arctic Ocean (Harris et al., 2014; Zhan et al., 2017). These shelves host diverse biogeochemical processes, including primary production, organic matter decomposition, nitrogen cycling (i.e., nitrification and denitrification), and carbon sequestration, which are influenced by varying levels of vertical mixing and river discharge (Aagaard and Carmack, 1989; Opsahl et al., 1999; Terhaar et al., 2021). Furthermore, factors such as the presence of sea ice and generally low wind speeds prevent air-sea gas exchange (Butterworth and Miller, 2016; Rutgers van der Loeff et al., 2014; Zhan et al., 2017), yielding local minima and maxima in N2O saturation. In the Arctic Ocean, oversaturation values (reaching up to 157%) have been reported over the shallow continental shelves, while the central Arctic Ocean experiences saturation values either close to the equilibrium or undersaturated (Hirota et al., 2009; Zhang et al., 2015; Fenwick et al., 2017; Zhan et al., 2017; Manning et al., 2022; Wild et al., 2023). The occurrence of oversaturation over continental shelves is attributed to the substantial N2O production within the active sedimentary layer, which then diffuses into the overlying water column and hence, the atmosphere. These oversaturation are often reported close to marginal ice zones or sea ice covered areas, since sea ice inhibits air-sea gas exchange (Kitidis et al., 2010; Fenwick et al., 2017; Manning et al., 2022). Conversely, the undersaturation in the central Arctic Ocean or the shelves is usually attributed to several factors, including: the dilution by sea-ice meltwater (i), rapid cooling of surface waters (ii), upwelling of undersaturated deep waters (iii), or microbiological N2O consumption (iv) (Kitidis et al., 2010; Randall et al., 2012; Verdugo et al., 2016; Zhan et al., 2016; Liu et al., 2022; Wild et al., 2023). Our study confirms this spatial heterogeneity in surface N2O saturation (Figure 6). Specifically, we observed 15 stations with undersaturated values (below 97%), 8 stations close to the equilibrium (i.e., between 97-103%) and 4 stations with oversaturated values (i.e., above 103%).
We observed significant undersaturation in N2O, down to 80%, off the shelf at the two open ocean stations located North of 82°N (Figure 6). A lower degree of undersaturation was also observed South of the open ocean stations, at the northwestern stations of the Kara Sea, influenced by the inflow of surface waters from the FSBW (Figures 1, 6). Sea surface temperature at these stations was close to the freezing point (-1.6°C to – 1.4°C for the two open ocean stations; Figure 6A). This suggests that these waters were recently below sea ice, consistent with their proximity to the sea ice edge and ocean circulation (Figures 2, 6C). Our assumption attributes this undersaturation to water cooling coupled to limited air-sea exchange. The surface waters cool down as they enter the Arctic Ocean’s sea ice zone through Fram Strait or during winter in the central Arctic Ocean, enhancing solubility resulting in undersaturation. The deficit cannot be offset due to restrained air-sea gas exchange inherent to sea ice cover (Rutger van der Loeff et al., 2014), along their transit to the Kara Sea. Furthermore, the possibility of vertical mixing with undersaturated deep waters may contribute to this surface water undersaturation, as observed by Zhan et al. (2016) in the Greenland Basin. However, contrary to Zhan et al. (2016), we observe a decrease in saturation towards the surface, suggesting that additional processes are required to explain the observed undersaturation.
To test our hypothesis, we quantified the change in solubility caused by changes in temperature and salinity. The temperature effect was examined by cooling the water mass, while the salinity effect was induced by the melting of sea ice. Our analysis focused on a water mass near the equilibrium point (N2O saturation of 100%), and we prescribed an initial sea surface temperature, salinity and N2O concentration at 2°C, 34 and 14.5 nM, respectively. These conditions are representative of surface water in the Kara Sea in equilibrium with the atmosphere (Figure 7). We then calculated the amount of ice meltwater required to reach a salinity of 32, which resulted in a corresponding concentration of 13.7 nM when assuming a concentration of 2.1 nM in sea ice. We assume that sea ice concentration follows a conservative behavior, i.e., a linear relationship between N2O concentration and salinity. When considering only ice meltwater, the N2O saturation decreased from 100% to 93%, indicating a relatively weak impact from the dilution effect of ice meltwater and solubility effect of salinity. However, when solely considering the temperature effect (i.e., a cooling from 2°C to -1.8°C at a salinity of 34), the saturation dropped to 85%. By combining both effects (i.e., ice meltwater and water cooling), we were able to replicate the observed saturation of 80%. These suggest that the significant undersaturation observed at these stations are influenced by physical processes, with three quarters attributed to a cooling effect and one quarter to sea-ice meltwater. Considering the mixed layer depth of 12 m at these stations, an estimated 0.9 m of melted sea ice thickness per m2 would be required to reach that salinity, which, while significant, remains possible (Karvonen et al., 2022).
The eastern coastal stations located at 100°E, exhibited elevated concentrations of N2O, as the Voronin Trough (S. 2), but with lower saturation levels ranging from 90% to 101% (Figure 6). This area is under the influence of river discharge, characterized by its overall lower sea surface salinity (Figure 6B). Enhanced concentration in N2O can be due to N2O supply from the rivers but also due to enhanced pelagic – benthic coupling in shallow bathymetries and over the shelves. In addition, higher concentrations can be due to atmospheric N2O uptake, given that these waters are undersaturated in N2O relative to the atmosphere. Wild et al. (2023), who also found little relationship between N2O and salinity in the plume of the Ob and Yenisey rivers, suggested that the processes at play are more intricate that a simple N2O supply from the rivers. We attribute the saturation deficit to a salinity effect on solubility that outweighs enhanced N2O concentration. For instance, if we consider a water mass in equilibrium with the atmosphere representative of the northern Kara Sea as above (i.e., 14,5 nM with a temperature of 2°C and salinity of 34 for 100% saturation), a decrease in the salinity to 14.6, a change in temperature to 0°C, and a constant N2O concentration, as observed in the river discharge area, would lead to a significant change in saturation down to 82%. Consequently, the Ob and Yenisey rivers are not contributing to an enriched N2O signal that would serve as a source to the atmosphere but likely promote undersaturation and N2O uptake in summer.
Oversaturation was observed in four stations (stations 1, 10, 16 and 59 in Figure 6 and Supplementary Figure S1A), three are located on the western side of the Kara Sea, at the entrance of the Barents Sea, and one in the Voronin Trough. The common point between these stations is that they are all categorized as shelf stations, meaning that their water column is, on average, enriched in N2O (Figure 3). Oversaturation at the entrance of the Barents Sea may result from the mixing between N2O-poor, warm and saline surface waters from the Barents Sea with N2O-rich, cold and fresh surface waters in the Kara Sea. (S. 2A). Knowing that a change of 1°C in temperature induces a change of approximately 5% in N2O saturation while preserving the N2O concentration, these conditions would yield oversaturation, implying that this change in solubility is occurring faster than air-sea exchange. There is a semi-cyclonic circulation in the St. Anna Trough, where the warmer and oversaturated waters flow northward, and the colder and undersaturated waters flow southward (Figures 2 and 6A). This water circulation caused the stations in the central St. Anna Trough to reach equilibrium with the atmosphere while forming a latitudinal saturation gradient.
The oversaturation of the surface waters in the Voronin Trough likely results from the N2O production integrated over the continental shelf of the Kara Sea and exiting the Kara Sea via the Voronin Trough (Figures 1, 2). The presence of sea ice in this area (Figure 6S) prevents surface waters from reaching atmospheric equilibrium, inhibiting air-sea gas exchange (Rutgers van der Loeff et al., 2014). Fenwick et al. (2017) and Kitidis et al. (2010) observed a similar tendency of N2O build-up and oversaturation under sea ice covered areas in the Canadian Archipelago.
4.4 N2O sea-air fluxes
Our observations in the northern Kara Sea suggest that the area acts as a minor sink during summertime (Table 1). Mean N2O sea-air fluxes in this study (-0.6 μmol N2O m-2 d-1) are reasonably close to previous studies in the western shelves of the Arctic Ocean (Fenwick et al., 2017; Manning et al., 2022), ranging between -1 μmol N2O m-2 d-1 to 0 μmol N2O m-2 d-1. By contrast, the mean flux in this study is much lower than the mean value measured in the Kara Sea by Wild et al. (2023), who measured positive fluxes up to 58.1 μmol N2O m-2 d-1. An explanation for our lower N2O fluxes observed can be attributed to the comparatively narrower range of N2O saturation observed in our study, as opposed to Wild et al. (2023), who observed a larger spatial variability in surface waters of the eastern Arctic shelves. We denoted a stronger sink for the off-shelf stations (Table 1), which is related to the enhanced N2O undersaturation compared to on-shelf stations. As explained in section 4.3, this strong undersaturation at these stations results from a combination of increased solubility that is driven by cooling and sea ice melting and the presence of sea ice that inhibited air-sea exchange prior to being transported into the area.
Based on the average sea-air fluxes and considering solely the ice-free zone, we computed the monthly average emission of N2O during summertime for the northern Kara Sea to be -0.1 Gg N month-1 (Table 1). As the area is undergoing substantial changes in sea ice melting and retreat during summer (S3) and the river discharge can drastically change with the season (Holmes et al., 2012), we did not consider our dataset to be representative of an annual average, but it underlines that the Kara Sea can act as N2O sink for the atmosphere. This calls for further fall to spring measurements in the continental shelf area.
5 Conclusion
Our study provides an analysis of N2O dynamics across the eastern Arctic shelves, in the Kara Sea during summertime. Despite the observed heterogeneity between N2O vertical profiles, an overall pattern indicates accumulation of N2O in the bottom waters of the Kara Sea shelves. Similar N2O enrichment was observed for the Voronin Trough, that receives water from the shallow shelves on their transit to the central Arctic Ocean. In contrast, low N2O accumulation was observed in the intermediate and deep waters of the open ocean and St. Anna Trough areas. N2O-enrichment correlated with N* deficit but showed no significant relationship with DIN nor AOU. Accumulation of N2O in shelf bottom waters is likely driven by benthic denitrification and/or coupled benthic nitrification-denitrification processes, and appears to be modulated by residence times of shelf bottom waters in contact with the reactive sedimentary layer.
In surface waters, N2O saturation was in average close to equilibrium with the atmosphere. Changes in solubility that are driven by temperature appeared as the main controlling factor for surface N2O concentration and saturation. Some spatial heterogeneity in surface saturation values resulted from the effects of freshwater discharge from the rivers and the presence of sea ice. River discharge would promote undersaturation, while sea ice would inhibit air-sea exchange, promoting either undersaturation or N2O build-up underneath or nearby. Air-sea exchange estimates determined that the Kara Sea acts as a minor sink during summertime.
These findings, and especially the spatial variability, highlight the complex interplay of physical and biogeochemical processes governing N2O dynamics in the Arctic Ocean and underscore the necessity for further process-based studies to enhance our understanding of these mechanisms. The climate-driven shift the Arctic Ocean is undergoing may potentially alter the nitrogen cycle and N2O dynamics in response to changing Arctic environments.
Data availability statement
The N2O dataset for this study can be found on the Arctic Century repository on PANGEA doi: 10.1594/PANGAEA.973143. The nutrient dataset for this study can be found on the Arctic Century repository on PANGEA doi: 10.1594/PANGAEA.973439. The wind speed dataset for this study can be found on the Arctic Century repository on PANGEA doi: https://climate.copernicus.eu/. Absolute geostrophic velocity data is available through the Copernicus Climate Service https://climate.copernicus.eu/, product name “Global Ocean Gridded L 4 Sea Surface Heights And Derived Variables Reprocessed Copernicus Climate Service” (product ID: SEALEVEL_GLO_PHY_CLIMATE_L4_MY_008_057; doi:10.48670/moi-00145).
Author contributions
SM: Data curation, Formal analysis, Software, Writing – original draft, Writing – review & editing. FF: Writing – original draft, Writing – review & editing, Supervision. SJ: Writing – review & editing. LP: Software, Writing – review & editing. JH: Data curation, Writing – review & editing. AM-G: Writing – review & editing. BD: Supervision, Writing – original draft, Writing – review & editing.
Funding
The author(s) declare that financial support was received for the research, authorship, and/or publication of this article. This work was supported by the F.R.S-FNRS (grants J.0051.20 and J.0060.22). BD has received funding from GreenFeedBack (Greenhouse gas fluxes and earth system feedback) funded by the European Union’s HORIZON research and innovation program under grant agreement No 101056921. Views and opinions expressed are however those of the authors only and do not necessarily reflect those of the European Union or CINEA. Neither the European Union nor the granting authority can be held responsible for them. SM & BD are FRIA PhD student and Research Associate, respectively, at the F.R.S.-FNRS.
Acknowledgments
We thank the chief scientist Heidi Kassens, and the Master and the crew of the RV Akademik Tryoshnikov for their support in sample collection. Arctic Century expedition funded by the Swiss Polar Foundation, a joint initiative led by the Swiss Polar Institute, the Antarctic and Arctic Research Institute and GEOMAR Helmholtz Centre for Ocean Research Kiel (GEOMAR) under the auspices of the research project The Changing Arctic Transpolar System (CATS). We also thank Iris Thurnherr for sharing the wind speed data with us.
Conflict of interest
The authors declare that the research was conducted in the absence of any commercial or financial relationships that could be construed as a potential conflict of interest.
Publisher’s note
All claims expressed in this article are solely those of the authors and do not necessarily represent those of their affiliated organizations, or those of the publisher, the editors and the reviewers. Any product that may be evaluated in this article, or claim that may be made by its manufacturer, is not guaranteed or endorsed by the publisher.
Supplementary material
The Supplementary Material for this article can be found online at: https://www.frontiersin.org/articles/10.3389/fmars.2024.1497360/full#supplementary-material
References
Aagaard K., Carmack E. C. (1989). The role of sea ice and other fresh water in the Arctic circulation. J. Geophysical Research: Oceans 94, 14485–14498. doi: 10.1029/JC094iC10p14485
Anderson L. G., Björk G., Jutterström S., Pipko I., Shakhova N., Semiletov I., et al. (2011). East Siberian Sea, an Arctic region of very high biogeochemical activity. Biogeosciences 8, 1745–1754. doi: 10.5194/bg-8-1745-2011
Arctic Ocean Physics Analysis and Forecast. Available online at: https://data.marine.copernicus.eu/product/ARCTIC_ANALYSISFORECAST_PHY_002_001/description (Accessed July 5, 2024).
Arrigo K. R., van Dijken G. L. (2011). Secular trends in Arctic Ocean net primary production. J. Geophysical Research: Oceans 116, C09011. doi: 10.1029/2011JC007151
Global ocean gridded L 4 sea surface heights and derived variables reprocessed copernicus climate service. In: Copernicus marine service. Available online at: https://data.marine.copernicus.eu/product/SEALEVEL_GLO_PHY_CLIMATE_L4_MY_008_057/description (Accessed May 31, 2024).
Global ocean sea ice concentration time series REPROCESSED (OSI-SAF). Available online at: https://data.marine.copernicus.eu/product/SEAICE_GLO_SEAICE_L4_REP_OBSERVATIONS_011_009/description (Accessed May 31, 2024).
Bange H. W.. (2008). “Chapter 2 - Gaseous nitrogen compounds (NO, N2O, N2, NH3) in the ocean,” in Nitrogen in the marine environment (Second edition) Ed. Capone D. G., Bronk D. A., Mulholland M. R., Carpenter E. J. (Academic Press, San Diego), 51–94. doi: 10.1016/B978-0-12-372522-6.00002-5
Bianchi D., Dunne J. P., Sarmiento J. L., Galbraith E. D. (2012). Data-based estimates of suboxia, denitrification, and N2O production in the ocean and their sensitivities to dissolved O2. Global Biogeochemical Cycles 26, GB2009. doi: 10.1029/2011GB004209
Bianchi D., Weber T. S., Kiko R., Deutsch C. (2018). Global niche of marine anaerobic metabolisms expanded by particle microenvironments. Nat. Geosci. 11, 263–268. doi: 10.1038/s41561-018-0081-0
Bothe H., Jost G., Schloter M., Ward B. B., Witzel K. (2000). Molecular analysis of ammonia oxidation and denitrification in natural environments. FEMS Microbiol. Rev. 24, 673–690. doi: 10.1111/j.1574-6976.2000.tb00566.x
Brandes J. A., Devol A. H. (2002). A global marine-fixed nitrogen isotopic budget: Implications for Holocene nitrogen cycling. Global Biogeochemical Cycles 16, 67-1-67–14. doi: 10.1029/2001GB001856
Butterworth B. J., Miller S. D. (2016). Air-sea exchange of carbon dioxide in the Southern Ocean and Antarctic marginal ice zone. Geophysical Res. Lett. 43, 7223–7230. doi: 10.1002/2016GL069581
Chang B. X., Devol A. H. (2009). Seasonal and spatial patterns of sedimentary denitrification rates in the Chukchi sea. Deep Sea Res. Part II: Topical Stud. Oceanography 56, 1339–1350. doi: 10.1016/j.dsr2.2008.10.024
Cohen Y., Gordon L. I. (1979). Nitrous oxide production in the Ocean. J. Geophysical Research: Oceans 84, 347–353. doi: 10.1029/JC084iC01p00347
de Boyer Montégut C., Madec G., Fischer A. S., Lazar A., Ludicone D. (2004). Mixed layer depth over the global ocean: An examination of profile data and a profile-based climatology. J. Geophysical Research: Oceans 109, C12003. doi: 10.1029/2004JC002378
Devol A. H., Codispoti L. A., Christensen J. P. (1997). Summer and winter denitrification rates in western Arctic shelf sediments. Continental Shelf Res. 17, 1029–1050. doi: 10.1016/S0278-4343(97)00003-4
Farías L., Faúndez J., Fernández C., Cornejo M., Sanhueza S., Carrasco C. (2013). Biological N2O fixation in the Eastern South Pacific Ocean and marine cyanobacterial cultures. PloS One 8, e63956. doi: 10.1371/journal.pone.0063956
Fenwick L., Capelle D., Damm E., Zimmermann S., Williams W. J., Vagle S., et al (2017). Methane and nitrous oxide distributions across the North American Arctic Ocean during summe. J. Geophysical Research: Oceans 122, 390–412. doi: 10.1002/2016JC012493
Frey K. E., McClelland J. W., Holmes R. M., Smith L. C. (2007). Impacts of climate warming and permafrost thaw on the riverine transport of nitrogen and phosphorus to the Kara Sea. J. Geophysical Research: Biogeosciences 112, G04S58. doi: 10.1029/2006JG000369
Fripiat F., Declercq M., Sapart C. J., Anderson L. G., Bruechert V., Deman F., et al. (2018). Influence of the bordering shelves on nutrient distribution in the Arctic halocline inferred from water column nitrate isotopes. Limnology Oceanography 63, 2154–2170. doi: 10.1002/lno.10930
Gangnus I., Makhotin M., Cherniavskaia E., Hölemann J. A., Kusse-Tiuz N., Lavinen N., et al. (2022). Nutrients, oxygen, total alkalinity, pH, and physical oceanography measured on water bottle samples during Akademik Tryoshnikov cruise Arctic Century 2021 Expedition (AT21), Arctic Ocean. PANGAEA. doi: 10.1594/PANGAEA.947808
Granger J., Prokopenko M. G., Sigman D. M., Mordy C. W., Morse Z. M., Morales L. V., et al. (2011). Coupled nitrification-denitrification in sediment of the eastern Bering Sea shelf leads to 15N enrichment of fixed N in shelf waters. J. Geophysical Research: Oceans 116, C11006. doi: 10.1029/2010JC006751
Grebmeier J. M., Cooper L. W., Feder H. M., Sirenko B. I., et al. (2006). Ecosystem dynamics of the pacific-influenced northern bering and chukchi seas in the amerasian arctic. Prog. Oceanography 71, 331–361. doi: 10.1016/j.pocean.2006.10.001
Gruber N., Sarmiento J. L. (1997). Global patterns of marine nitrogen fixation and denitrification. Global Biogeochemical Cycles 11, 235–266. doi: 10.1029/97GB00077
Harris P. T., Macmillan-Lawler M., Rupp J., Baker E. K. (2014). Geomorphology of the oceans. Mar. Geology 352, 4–24. doi: 10.1016/j.margeo.2014.01.011
Heo J.-M., Kim S.-S., Kang S.-H., Yang E. J., Park K.-T., Jung J., et al. (2021). N2O dynamics in the western Arctic Ocean during the summer of 2017. Sci. Rep. 11, 12589. doi: 10.1038/s41598-021-92009-1
Hirota A., Ijiri A., Komatsu D. D., Ohkubo S. B., Nakagawa F., Tsunogai U. (2009). Enrichment of nitrous oxide in the water columns in the area of the Bering and Chukchi Seas. Mar. Chem. 116, 47–53. doi: 10.1016/j.marchem.2009.09.001
Ho D. T., Law C. S., Smith M. J., Schlosser P., Harvey M., Hill P., et al. (2006). Measurements of air-sea gas exchange at high wind speeds in the Southern Ocean: Implications for global parameterizations. Geophysical Res. Lett. 33, L16611. doi: 10.1029/2006GL026817
Hölemann J. A., Kusse-Tiuz N., Ruiz-Castillo E., Malinovskiy S., Merkulov V., Burkhardt M., et al. (2022). Physical oceanography measured with Underway-CTD (UCTD) during Akademik Tryoshnikov cruise Arctic Century 2021 Expedition (AT21), Arctic Ocean. PANGAEA. doi: 10.1594/PANGAEA.947810
Holmes R. M., McClelland J. W., Peterson B. J., Tank S. E., Bulygina E., Eglinton T. I., et al. (2012). Seasonal and annual fluxes of nutrients and organic matter from large rivers to the arctic ocean and surrounding seas. Estuaries Coasts 35, 369–382. doi: 10.1007/s12237-011-9386-6
Hsu S. A., Meindl E. A., Gilhousen D. B. (1994). Determining the Power-Law Wind-Profile Exponent under Near-Neutral Stability Conditions at Sea. J. Appl. Meteorology Climatology 33, 757–765. doi: 10.1175/1520-0450(1994)033<0757:DTPLWP>2.0.CO;2
Hydes D. J., Aoyama M., Aminot A., Bakker K., Becker S., Coverly S., et al. (2010). Determination of dissolved nutrients (N, P, SI) in seawater with high precision and inter-comparability using gas-segmented continuous flow analysers. doi: 10.25607/OBP-15
IPCC (2023). “Climate change 2023: synthesis report. Contribution of working groups I, II and III to the sixth assessment report of the intergovernmental panel on climate change [Core writing team, H. Lee and J. Romero (eds.)].,” (IPCC, Geneva, Switzerland), p. 184. doi: 10.59327/IPCC/AR6-9789291691647
Janout M. A., Hölemann J., Laukert G., Smirnov A., Krumpen T., Bauch D., et al. (2020). On the variability of stratification in the freshwater-influenced laptev sea region. Front. Mar. Sci. 7. doi: 10.3389/fmars.2020.543489
Karvonen J., Rinne E., Sallila H., Uotila P., Mäkynen M. (2022). Kara and Barents sea ice thickness estimation based on CryoSat-2 radar altimeter and Sentinel-1 dual-polarized synthetic aperture radar. Cryosphere 16, 1821–1844. doi: 10.5194/tc-16-1821-2022
Kitidis V., Upstill-Goddard R. C., Anderson L. G. (2010). Methane and nitrous oxide in surface water along the North-West Passage, Arctic Ocean. Mar. Chem. 121, 80–86. doi: 10.1016/j.marchem.2010.03.006
Liu X., Dunne J. P., Stock C. A., Harrison M. J., Adcroft A., Resplandy L. (2019). Simulating water residence time in the coastal ocean: A global perspective. Geophysical Res. Lett. 46, 13910–13919. doi: 10.1029/2019GL085097
Liu J., Zhan L., Wang Q., Wu M., Ye W., Zhang J., et al. (2022). Distribution and driving mechanism of N2O in sea ice and its underlying seawater during arctic melt season. Water 14, 145. doi: 10.3390/w14020145
Liu J., Chen L., Ling M., Zhuang Y., Zhang J., Ye W., et al. (2024). Potential contributions of ammonia-oxidizing microorganisms to the distributions of nitrous oxide in the northern bering sea. J. Geophysical Research: Oceans 129, e2023JC020677. doi: 10.1029/2023JC020677
Makkaveev P. N., Melnikova Z. G., Polukhin A. A., Stepanova S. V., Khlebopashev P. V., Chultsova A. L. (2015). Hydrochemical characteristics of the waters in the western part of the Kara Sea. Oceanology 55, 485–496. doi: 10.1134/S0001437015040116
Manning C. C. M., Zheng Z., Fenwick L., McCulloch R. D., Damm E., Izett R. W., et al. (2022). Interannual variability in methane and nitrous oxide concentrations and sea-air fluxes across the north american arctic ocean (2015-2019). Global Biogeochemical Cycles 36, e2021GB007185. doi: 10.1029/2021GB007185
McClelland J. W., Holmes R. M., Peterson B. J., Raymond P. A., Striegl R. G., Zhulidov A. V., et al. (2016). Particulate organic carbon and nitrogen export from major Arctic rivers. Global Biogeochemical Cycles 30, 629–643. doi: 10.1002/2015GB005351
McTigue N. D., Gardner W. S., Dunton K. H., Hardison A. K. (2016). Biotic and abiotic controls on co-occurring nitrogen cycling processes in shallow Arctic shelf sediments. Nat. Commun. 7, 13145. doi: 10.1038/ncomms13145
Muller S., Jaccard S. L., Hölemann J. A., Fripiat F., Martínez-García A., Makhotin M., et al. (2024). N2O concentrations measured on water bottle samples during Akademik Tryoshnikov cruise Arctic Century 2021 Expedition (AT21), Arctic Ocean. PANGAEA. doi: 10.1594/PANGAEA.973143
Muller S., Fripiat F., Schmitt M., Jaccard S. L., Hölemann J. A., Delille B., et al. (2024). Nutrients concentrations measured on water bottle samples during Akademik Tryoshnikov cruise Arctic Century 2021 Expedition (AT21), Arctic Ocean [dataset]. PANGAEA. doi: 10.1594/PANGAEA.973439
Nevison C., Butler J. H., Elkins J. W. (2003). Global distribution of N2O and the ΔN2O-AOU yield in the subsurface ocean. Global Biogeochemical Cycles 17, 1119. doi: 10.1029/2003GB002068
Opsahl S., Benner R., Amon R. M. W. (1999). Major flux of terrigenous dissolved organic matter through the Arctic Ocean. Limnology Oceanography 44, 2017–2023. doi: 10.4319/lo.1999.44.8.2017
Pabi S., van Dijken G. L., Arrigo K. R. (2008). rimary production in the arctic ocean 1998-2006. J. Geophysical Research: Oceans 113, C08005. doi: 10.1029/2007JC004578
Randall K., Scarratt M., Levasseur M., Michaud S., Xie H., Gosselin M. (2012). First measurements of nitrous oxide in Arctic sea ice. J. Geophysical Research: Oceans 117, C00G15. doi: 10.1029/2011JC007340
Ravishankara A. R., Daniel J. S., Portmann R. W. (2009). Nitrous oxide (N2O): the dominant ozone-depleting substance emitted in the 21st century. Science 326, 123–125. doi: 10.1126/science.1176985
Rees A. P., Brown I. J., Jayakumar A., Ward B. B. (2016). The inhibition of N2O production by ocean acidification in cold temperate and polar waters. Deep Sea Res. Part II: Topical Stud. Oceanography 127, 93–101. doi: 10.1016/j.dsr2.2015.12.006
Rowe G. T., CliQord C. H., Smith K. L., Hamilton P. L. (1975). Benthic nutrient regeneration and its coupling to primary productivity in coastal waters. Nature 255, 215–217. doi: 10.1038/255215a0
Rutgers van der Loeff M. M., Cassar N., Nicolaus M., Rabe B., Stimac I. (2014). The influence of sea ice cover on air-sea gas exchange estimated with radon-222 profiles. J. Geophysical Research: Oceans 119, 2735–2751. doi: 10.1002/2013JC009321
Schilt A., Brook E. J., Bauska T. K., Baggenstos D., Fischer H., Joos F., et al. (2014). Isotopic constraints on marine and terrestrial N2O emissions during the last deglaciation. Nature 516, 234–237. doi: 10.1038/nature13971
Schlosser P., Bauch D., Fairbanks R., Bönisch G.. (1994). Arctic river-runoff: mean residence time on the shelves and in the halocline. Deep Sea Res. Part I: Oceanographic Res. Papers 41, 1053–1068. doi: 10.1016/0967-0637(94)90018-3
Schuler K. H., Tortell P. D. (2023). Impacts of vertical mixing and ice-melt on N2O and CH4 concentrations in the Canadian Arctic Ocean. Continental Shelf Res. 269, 105124. doi: 10.1016/j.csr.2023.105124
Seitzinger S., Harrison J. A., Böhlke J. K., Bouwman A. F., Lowrance R., Peterson B., et al. (2006). Denitrification across landscapes and waterscapes: a synthesis. Ecol. Applications: A Publ. Ecol. Soc. America 16, 2064–2090. doi: 10.1890/1051-0761(2006)016[2064:dalawa]2.0.co;2
Sheng Y., Smith L. C., MacDonald G. M., Kremenetski K. V., Frey K. E., Velichko A. A., et al. (2004). A high-resolution GIS-based inventory of the west Siberian peat carbon pool. Global Biogeochemical Cycles 18, GB3004. doi: 10.1029/2003GB002190
Smith L. C., MacDonald G. M., Velichko A. A., Beilman D. W., Borisova O. K., Frey K. E., et al. (2004). Siberian peatlands a net carbon sink and global methane source since the early Holocene. Sci. (New York N.Y.) 303, 353–356. doi: 10.1126/science.1090553
Sun X., Humborg C., Mörth C.-M., Brüchert V. (2021). The importance of benthic nutrient fluxes in supporting primary production in the laptev and east siberian shelf seas. Global Biogeochemical Cycles 35, e2020GB006849. doi: 10.1029/2020GB006849
Tang W., Ward B. B., Beman M., Bristow L., Clark D., Fawcett S., et al. (2023). Database of nitrification and nitrifiers in the global ocean. Earth System Sci. Data 15, 5039–5077. doi: 10.5194/essd-15-5039-2023
Terhaar J., Lauerwald R., Regnier P., Gruber N., Bopp L. (2021). Around one third of current Arctic Ocean primary production sustained by rivers and coastal erosion. Nat. Commun. 12, 169. doi: 10.1038/s41467-020-20470-z
Thurnherr I., Moallemi A., Makhotin M. (2024). Flow-distortion corrected meteorological measurements from the Arctic century expedition in August/September 2021. ETH Zurich. doi: 10.3929/ethz-b-000693692
Tian H., Xu R., Canadell J. G., Thompson R. L., Winiwarter W., Suntharalingam P., et al. (2020). A comprehensive quantification of global nitrous oxide sources and sinks. Nature 586, 248–256. doi: 10.1038/s41586-020-2780-0
Tian H., Pan N., Thompson R. L., Canadell J. G., Suntharalingam P., Regnier P., et al. (2024). Global nitrous oxide budget, (1980-2020). Earth System Sci. Data 16, 2543–2604. doi: 10.5194/essd-16-2543-2024
Toyoda S., Kakimoto T., Kudo K., Yoshida N., Sasano D., Kosugi N., et al. (2021). Distribution and production mechanisms of N2O in the western arctic ocean. Global Biogeochemical Cycles 35, e2020GB006881. doi: 10.1029/2020GB006881
Tremblay J.-É., Anderson L. G., Matrai P., Coupel P., Bélanger S., Michel C., et al. (2015). Global and regional drivers of nutrient supply, primary production and CO2 drawdown in the changing Arctic Ocean. Prog. Oceanography 139, 171–196. doi: 10.1016/j.pocean.2015.08.009
Upstill-Goddard R. C., Rees A. P., Owens N. J. P. (1996). Simultaneous high-precision measurements of methane and nitrous oxide in water and seawater by single phase equilibration gas chromatography. Deep Sea Res. Part I: Oceanographic Res. Papers 43, 1669–1682. doi: 10.1016/S0967-0637(96)00074-X
National Oceanic and Atmospheric Administration (NOAA), n.d. (2024). Global Monitoring Laboratory (GML). Available online at: https://gml.noaa.gov/ (Accessed June 11, 2024).
Verdugo J., Damm E., Snoeijs P., Díez B., Farías L. (2016). Climate relevant trace gases (N2O and CH4) in the Eurasian Basin (Arctic Ocean). Deep Sea Res. Part I: Oceanographic Res. Papers 117, 84–94. doi: 10.1016/j.dsr.2016.08.016
Wanninkhof R. (2014). Relationship between wind speed and gas exchange over the ocean revisited. Limnology Oceanography: Methods 12, 351–362. doi: 10.4319/lom.2014.12.351
Ward B. B. (2008). “Chapter 5 - nitrification in marine systems,” in Nitrogen in the marine environment, 2nd. Ed. Capone D. G., Bronk D. A., Mulholland M. R., Carpenter E. J. (Academic Press, San Diego), 199–261. doi: 10.1016/B978-0-12-372522-6.00005-0
Weiss R. F. (1981). Determinations of carbon dioxide and methane by dual catalyst flame ionization chromatography and nitrous oxide by electron capture chromatography. J. Chromatographic Sci. 19, 611–616. doi: 10.1093/chromsci/19.12.611
Weiss R. F., Price B. A. (1980). Nitrous oxide solubility in water and seawater. Mar. Chem. 8, 347–359. doi: 10.1016/0304-4203(80)90024-9
Wild B., Ray N. E., Lett C., Davies A.J., Kirillova E., Holmstrand H., et al. (2023). Nitrous oxide dynamics in the siberian arctic ocean and vulnerability to climate change. J. Geophysical Research: Biogeosciences 128, e2022JG007326. doi: 10.1029/2022JG007326
Wu M., Chen L., Zhan L., Zhang J., Li Y., Liu J. (2017). Spatial variability and factors influencing the air-sea N2O flux in the bering sea, chukchi sea and chukchi abyssal plain. Atmosphere 8, 65. doi: 10.3390/atmos8040065
Yang S., Chang B.X., Warner M.J., Weber T.S., Bourbonnais A.M., Santoro A.E., et al. (2020). Global reconstruction reduces the uncertainty of oceanic nitrous oxide emissions and reveals a vigorous seasonal cycle. Proc. Natl. Acad. Sci. 117, 11954–11960. doi: 10.1073/pnas.1921914117
Zhan L., Chen L., Zhang J., Li Y. (2015). A vertical gradient of nitrous oxide below the subsurface of the Canada Basin and its formation mechanisms. J. Geophysical Research: Oceans 120, 2401–2411. doi: 10.1002/2014JC010337
Zhan L., Chen L., Zhang J., Yan J., Li Y., Wu M. (2016). A permanent N2O sink in the Nordic Seas and its strength and possible variability over the past four decades. J. Geophysical Research: Oceans 121, 5608–5621. doi: 10.1002/2016JC011925
Zhan L., Wu M., Chen L., Zhang J., Li Y., Liu J. (2017). The air-sea nitrous oxide flux along cruise tracks to the arctic ocean and southern ocean. Atmosphere 8, 216. doi: 10.3390/atmos8110216
Zhan L., Zhang J., Ouyang Z., Lei R., Xu S., Qi D., et al. (2021). High-resolution distribution pattern of surface water nitrous oxide along a cruise track from the Okhotsk Sea to the western Arctic Ocean. Limnology Oceanography 66, S401–S410. doi: 10.1002/lno.11604
Keywords: nitrous oxide, N2O flux, air-sea exchange, Kara Sea, arctic ocean, nitrification, denitrification, nitrogen cycle
Citation: Muller S, Fripiat F, Jaccard SL, Ponsoni L, Hölemann JA, Martínez-García A and Delille B (2024) Nitrous oxide dynamics in the Kara Sea, Arctic Ocean. Front. Mar. Sci. 11:1497360. doi: 10.3389/fmars.2024.1497360
Received: 16 September 2024; Accepted: 28 October 2024;
Published: 22 November 2024.
Edited by:
Travis Blake Meador, Academy of Sciences of the Czech Republic (ASCR), CzechiaReviewed by:
Frank Dehairs, Vrije University Brussels, BelgiumWangwang Ye, State Oceanic Administration, China
Copyright © 2024 Muller, Fripiat, Jaccard, Ponsoni, Hölemann, Martínez-García and Delille. This is an open-access article distributed under the terms of the Creative Commons Attribution License (CC BY). The use, distribution or reproduction in other forums is permitted, provided the original author(s) and the copyright owner(s) are credited and that the original publication in this journal is cited, in accordance with accepted academic practice. No use, distribution or reproduction is permitted which does not comply with these terms.
*Correspondence: Sofia Muller, c29maWFtdWxsZXIuYmVAaG90bWFpbC5jb20=; c29maWEubXVsbGVyQHVsaWVnZS5iZQ==; Bruno Delille, YnJ1bm8uZGVsaWxsZUB1bGllZ2UuYmU=