- 1School of Life Sciences, Guangzhou University, Guangzhou, China
- 2Agro-Tech Extension Center of Guangdong Province, Guangzhou, China
Hypoxia is an unfavorable environmental condition that produces diverse negative effects in fish. High-density cultures of Epinephelus coioides are more likely to experience hypoxic conditions than those in natural environments. To assess the effects of hypoxia on E. coioides, we examined the related enzyme activities and gene expression after 48 h of hypoxia and 24 h of dissolved oxygen (DO) recovery. Under hypoxic stress (DO: 1.2 ± 0.1 mg/L), the energy supply mode of fish changed from aerobic metabolism to anaerobic metabolism, and the serum glucose content and lactate dehydrogenase activity were significantly upregulated. Total protein, hepatic glycogen, and two key regulatory enzymes (i.e., hexokinase and pyruvate kinase) were differentially expressed in the liver, and mRNA expression of three genes (i.e., LDHA, GLUT1, and MCT2) also showed a high expression trend. In serum, three immune-related enzymes (i.e., alanine aminotransferase, aspartate aminotransferase, and alkaline phosphatase) were found to be involved in regulation by hypoxia and showed different levels of changing patterns. Expression of inflammatory genes (i.e., IL-8, IFNγ, MyD88, and NF-kB) were significantly regulated in liver. With prolongation of hypoxic stress, high expression of apoptotic genes (i.e., p53, Bax, Bcl-2, and Caspase-9) was closely related to the degree of apoptosis in the liver. Our investigation of the changes in energy metabolism, immune response, and apoptosis of E. coioides under hypoxia and reoxygenation (DO, 6.0 ± 0.1 mg/L) provides a theoretical bases for healthy aquaculture and selection of varieties with tolerance to hypoxia.
1 Introduction
Dissolved oxygen (DO) concentrations in water bodies fluctuate significantly due to global warming, seasonal variations, aquatic plant photosynthesis, water body eutrophication, and aquaculture densities (Breitburg and Levin, 2018; Klaus et al., 2021; Qiu et al., 2024). After reaching a certain water depth, the DO concentration decreases with an increase in vertical height and sedimentation of decomposed organic matter (Zhai et al., 2012; Xie et al., 2022). The DO concentration is an important water quality indicator and an important factor for maintaining life activities of fish. The higher the DO concentration in a water body, the more beneficial it is to the activities of aquatic animals (Jeong et al., 2024). Oxygen deficiencies in nearshore coastal areas are increasing globally, causing irreversible negative effects on the fish farming industry (Diaz and Rosenberg, 2008; Domenici et al., 2017).
DO is not only directly related to fish habitat distribution and environmental homeostasis but also affects fish population structure (Wannamaker and Rice, 2000), behavior (Zhang et al., 2010), growth (He et al., 2022), migration (Boussinet et al., 2024), reproduction (Saha et al., 2022), and disease resistance (Evans et al., 2003). Prolonged hypoxia can lead to fish mortality (Abdel-Tawwab et al., 2019). Normal cells obtain energy through aerobic respiration; however, when the oxygen supply is insufficient, the respiratory mode shifts to anaerobic respiration, which consumes glucose and produces large amounts of lactic acid metabolites (Cuninghame et al., 2017). To adapt to low oxygen stress, fish enhance their anaerobic metabolic patterns to replenish the energy required for life activities (Sun et al., 2021).
Fish often activate various pathways to regulate homeostasis, and in addition to regulating energy metabolism patterns, apoptosis is also activated, which helps fish maintain relative homeostasis under hypoxic stress conditions (Qiang et al., 2020). Reactive oxygen species (ROS), which are small molecules with high activity, have irreversible effects on intracellular proteins, lipids, and DNA. Under hypoxic conditions, accumulated ROS may not be eliminated rapidly, leading to severe cellular damage and enhancing the autophagy signal of organic cells (Scherz-Shouval and Elazar, 2011; Yang et al., 2018). Genomic analyses have shown that continuous hypoxia induces signaling pathways associated with cytoprotection, immune regulation, and energy metabolism in fish (Wang et al., 2023). This indicates that hypoxia can significantly affect the physiological and immune responses of fish, making them more susceptible to diseases (Abdel-Tawwab et al., 2019). Previous studies have found that the hypoxia-inducible factor (HIF) signaling pathway is a key pathway for maintaining oxygen homeostasis in tissues and cells under hypoxia, with the hypoxia-inducible factor (HIF-1α) being a central regulatory gene in this pathway (Bayele et al., 2007; Palazon et al., 2014). A study on Megalobrama amblycephala showed that hypoxia may induce apoptosis by enhancing the HIF pathway and hence activating downstream pathways (Yu et al., 2023). Studies on the function of the HIF-1α gene revealed its involvement in immune regulation of Siniperca chuatsi against diseases (He et al., 2019). Hypoxic stress directly causes apoptosis and alterations in immune function by activating the HIF pathway (Guo and Tan, 2019).
Epinephelus coioides is an economically important farmed fish in Southeast Asia and a major container-farmed fish in China, with advantages of appealing taste and rich nutritional value, making it popular among consumers (Yu et al., 2018). However, with popularization of high-density container aquaculture models, the E. coioides aquaculture industry is facing the problem of low DO concentrations in water bodies (Wen et al., 2016). To address the hypoxia in water bodies caused by high stocking density and high nutrient input, and to elucidate the hypoxia regulation mechanisms of E. coioides, we conducted the study with E. coioides as the research subject. In the study, we examined enzyme activities and gene expressions in serum and the liver to evaluate the changes in energy metabolism, immune response, and apoptosis under low-oxygen conditions [DO: 1.2 ± 0.1 mg/L (hypoxia)] and normal conditions [6.0 ± 0.1 mg/L (normoxia)], providing a theoretical basis for the selection and breeding of new hypoxia-tolerant E. coioides varieties.
2 Materials and methods
2.1 Healthy experimental fish farming
Experiments were conducted at the Shenzhen Experimental Base of the South China Sea Fisheries Research Institute of the Chinese Academy of Fisheries Sciences. E. coioides (body weight, 15 ± 3 g; total length, 10 ± 2 cm) were domesticated for 2 weeks in recirculating water aquaculture tanks (110-cm diameter and 120-cm height). Fish were kept at 28°C ± 1°C in seawater and acclimatized with no bacterial or viral infection. The culture water salinity was 32 ± 1 ppt, and the DO concentration was 6.0 ± 0.1 mg/L, as measured using a water quality analyzer (LB-YSI, USA). During the domestication process, fish were fed commercial feed twice daily at a rate of 3% of the total weight of fish in each tank. All animal handling procedures and experimental protocols of the Experimental Animal Ethics Committee of the Guangzhou University of China were followed.
2.2 Hypoxia experiment and sample collection
The experimental procedure is illustrated in Figure 1. The experiment was set up with a normoxic control group, a hypoxic experimental group, and three parallel tanks in each group. The domesticated E. coioides were placed in six tanks, with 36 fish in each tank. Hypoxic treatment was according to our previous studies (Lai et al., 2022). Briefly, we stopped the circulating water, lowered the water level to approximately 30 cm, sealed the plastic film, and filled the water with nitrogen instead of oxygen. Then, when the DO concentration reached approximately 1.2 mg/L, we supplemented the water to match the level of the control group. This was a dynamic process; in order to maintain the DO of the water at 1.2 ± 0.1 mg/L, the circulating water and nitrogen were filled at the same time. The DO concentration in the three parallel experimental tanks in the hypoxia group decreased to the semi-lethal concentration (1.2 ± 0.1 mg/L) within 30 min. The dynamic DO concentration was constantly measured using a DO meter. The DO level of the hypoxia experiment was maintained at 1.2 ± 0.1 mg/L for 48 h. At the individual level, fish in hypoxic tanks reduced their swimming frequency to further decrease oxygen consumption, and at the same time, they surfaced to obtain more oxygen. They adapted to the hypoxic environment in this manner. After the 48-h hypoxia experiment, circulating water was immediately turned on and the oxygen pump was placed in the tank to increase the DO concentration to the normal level (6.0 ± 0.1 mg/L) for 24 h of recovery.
During the experiment, serum and liver samples were collected after 0 h (H0), 3 h (H3), 6 h (H6), 12 h (H12), 24 h (H24), and 48 h (H48) of hypoxia and 24 h of reoxygenation (R24). Three healthy fish were collected from each tank at each time point to obtain parallel samples. At this point, 0 h was the time when the DO concentration decreased to the desired low concentration. Livers for quantitative polymerase chain reaction (PCR) analysis were immediately placed into cryopreservation tubes containing RNA Keeper Tissue Stabilizer (Novozymes, Nanjing, China). Liver samples for enzyme activity measurements were rinsed with 0.9% physiological saline and dipped in filter paper before being placed into cryopreservation tubes. Tail vein blood was used to collect plasma, which was centrifuged after standing on ice for 4 h. The supernatant was then aspirated to obtain serum samples. The above samples were stored at −80°C for subsequent analysis.
2.3 Measurement of indices and enzyme activities
Serum samples used for enzyme activity determination were first three-mixed with 1, i.e., three fish sera from the same culture tank were mixed. Three replicate experiments were performed. Glucose (GLU: A154-1-1), lactate dehydrogenase (LDH: A020-2-2), and immunoenzymatic activities in serum samples were determined using enzyme activity kits. Alkaline phosphatase (AKP: A059-2-2), aspartate aminotransferase (AST: C010-2-1), and alanine aminotransferase (ALT: C009-2-1) were measured using a multifunctional enzyme labeling instrument (Infinite® 200 PRO, Switzerland).
Determination of liver enzyme activity was conducted using enzyme activity kits, including protein quantification [total protein (TP): A045-2-2), glycogen (glycogen: A043-1-1), pyruvate kinase (PK: A076-1-1), and hexokinase (HK: A077-3-1)]. Tissue samples were pre-treated according to the instruction manual, and the samples were three-mixed with 1 and set up in triplicate. The four indices were measured using a UV-visible spectrophotometer (UV1810; Shanghai, China). All kits were obtained from Nanjing Jiancheng Bioengineering Institute (Nanjing, China).
2.4 Measurement of mRNA levels using real-time qPCR
Total RNA was extracted from liver samples using RNA isolate Total RNA Extraction Reagent (Vazyme, Nanjing, China), and absorbance of the products was measured using an ultra-microplate spectrophotometer (BioTek EPOCH, USA). Samples with OD260/280 values in the range of 1.8–2.0 were selected as qualified samples, and the integrity of RNA was verified by 1.0% agarose gel electrophoresis. The obtained products were then used to synthesize template cDNA using HiScript® II Q RT SuperMix for qPCR (+gDNA wiper) (Vazyme, Nanjing, China) and were diluted fivefold for real-time fluorescence quantitative PCR (RT-qPCR) detection.
RT-qPCR primers were designed according to the mRNA coding sequence of E. coioides, and β-actin was used as a reference. The primers used for qPCR are listed in Table 1. A reaction system was added to a 96-well plate (Monad, Suzhou, China) containing 2 µL of cDNA at a concentration of 80 ± 5 ng/µL, 10 µL of chamQ Universal SYBR GPCR Master Mix (Vazyme, Nanjing, China), 0.4 µL each of forward and reverse primers at a concentration of 10 µM, and 7.2 µL of RNase-free H2O. The program was set to 30 s at 95°C, followed by 40 cycles of 10 s at 95°C and 30 s at 60°C. Finally, a melting curve was obtained after 15 s at 95°C, 60 s at 60°C, and 15 s at 95°C. The final data were calculated using the 2−ΔΔCT method. This process was conducted using a LightCycler® 480 Instrument II (Roche, Switzerland).
2.5 Statistical analysis
Before the data analysis, we assessed the normality of the data using a P-P plot. The relative expression levels of the target gene were subjected to one-way ANOVA, with Tukey’s multiple comparison test used as a post-hoc test. The determination of differences between groups was performed using IBM SPSS Statistics 26; p-values < 0.05 determined significant difference.
Bar graphs were plotted using Origin 2018 64 Bit.
3 Results
3.1 Effects on immunoenzymatic activity in serum
To investigate the effects of hypoxia on immune-related enzymes, we examined the activity of AKP, ALT, and AST (Figure 2). The peak ALT activity only appeared at H3, and its expression in the experimental group was 2.19-fold higher than that in the control group (Figure 2A). AST was highly expressed throughout the entire experiment, and the peak appeared at H3; the difference in the expression of the two groups was 2.04-fold (Figure 2B). AKP was highly expressed only at H48 and R24 (1.41- and 1.48-fold, respectively) (Figure 2C).
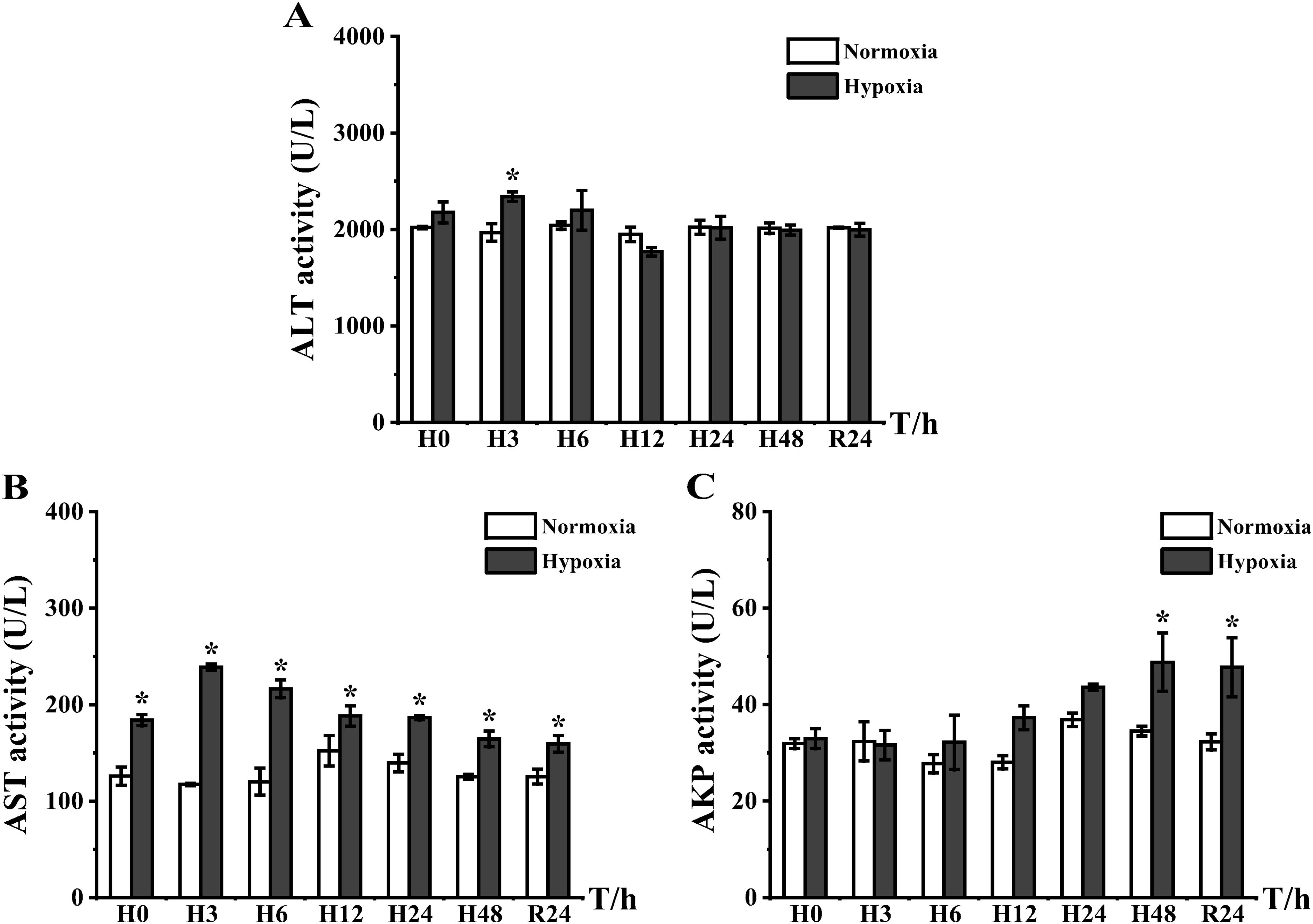
Figure 2. Expression of serum non-specific immunoenzymatic activity in E coioides: (A) ALT, (B) AST, and (C) AKP. Meanings of each abbreviation’s capital letter: H, hypoxia; R, reoxygenation. The results were analyzed by SPSS analysis. Data are presented as the mean ± standard error of mean (SEM) of three individual fish (n = 3). Asterisks (*) indicate significant differences (p < 0.05).
3.2 Effects of hypoxic stress on inflammatory genes
As shown in Figure 3, hypoxic stress resulted in increased mRNA expression levels of interleukin-8 (IL-8), interferon γ (IFNγ), myeloid differentiation primary response gene 88 (MyD88), and nuclear factor kappa-B (NF-κB). In the hypoxia group, IL-8, IFNγ, and NF-κB peaked at H6: IFNγ was highly expressed at H0 and then recovered to a level not significantly different from that of the control group after reaching the peak at H6 (Figure 3A), and IL-8 and NF-κB were significantly elevated at H3 and reached peaks at H6, which were 6.09- and 6.33-fold higher than that of the control group, respectively (Figures 3B, D). The high expression of IL-8 and NF-κB persisted until the late stage of hypoxia before decreasing to the same level as the control group with body acclimatization and DO restoration. IFNγ, although preferentially responding to stress, only showed high expression compared to the control group in the pre-experimental period, which was dissimilar to the high expression of MyD88 at H3 (2.67-fold) and H6 (2.47-fold). Notably, MyD88 continued to show high expression after DO restoration (Figure 3C), which differed from the expression of the other three genes.
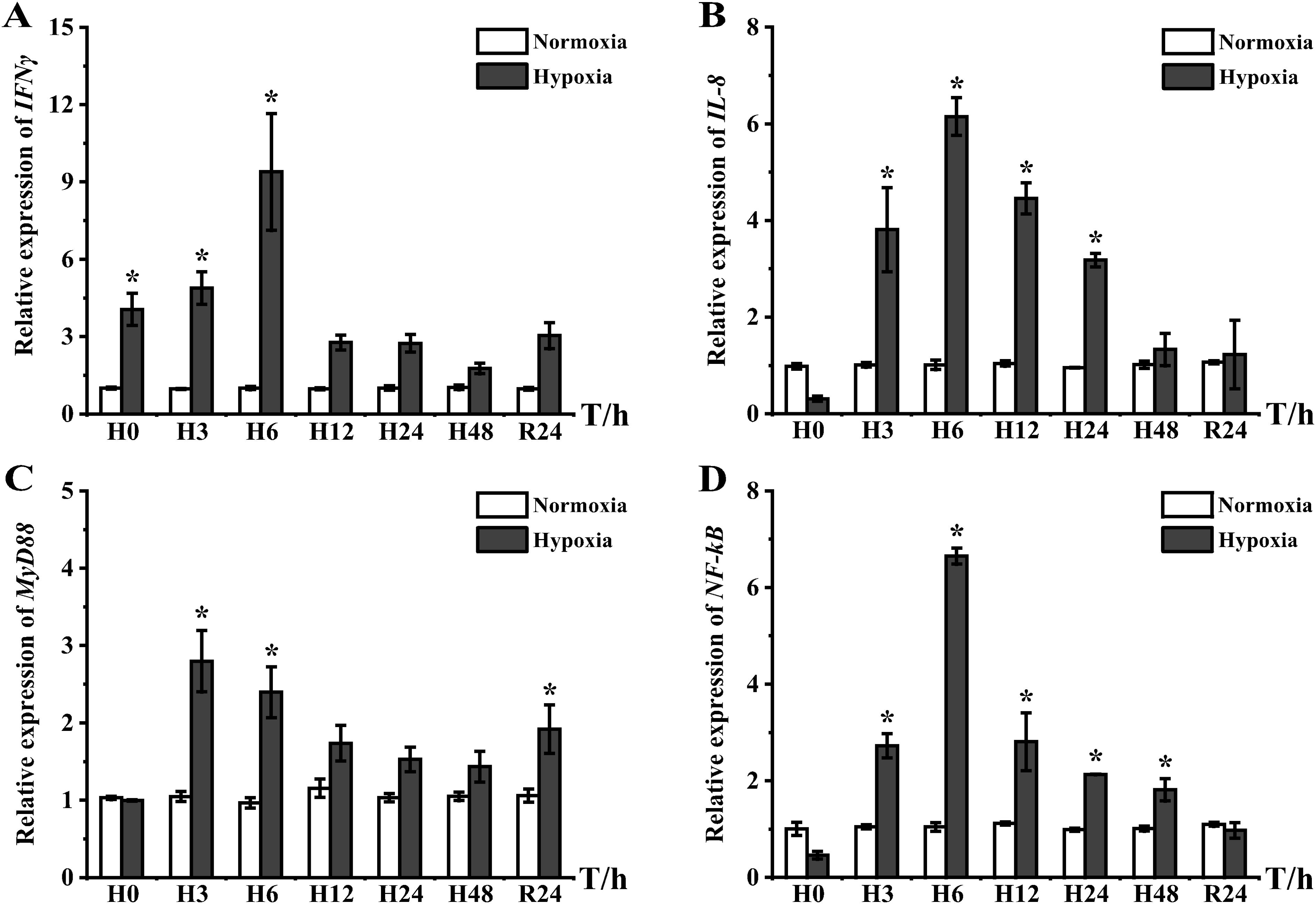
Figure 3. Inflammatory response gene expression in liver tissues of E coioides: (A) IFNγ, (B) IL-8, (C) MyD88, and (D) NF-κB. The results were analyzed by SPSS analysis. Data were presented as the mean ± SEM of three individual fish (n = 3). Asterisks (*) indicate significant differences (p < 0.05).
3.3 Effects on energy metabolism indices in serum
Serum GLU and LDH are involved in the glycolysis process of organisms (Wang et al., 2022), and the two indexes were significantly higher than those of the control group at the beginning of the experiment. The GLU content started to increase from H0 and slowed after reaching a peak (1.87-fold) at H3. Finally, the cells adapted to 48 h of hypoxia and recovered to the same level as that of the control group (Figure 4A). LDH (i.e., an enzyme that catalyzes lactic acid decomposition) was highly expressed at all time points; the peak appeared at H6 with a 1.84-fold difference in expression (Figure 4B).
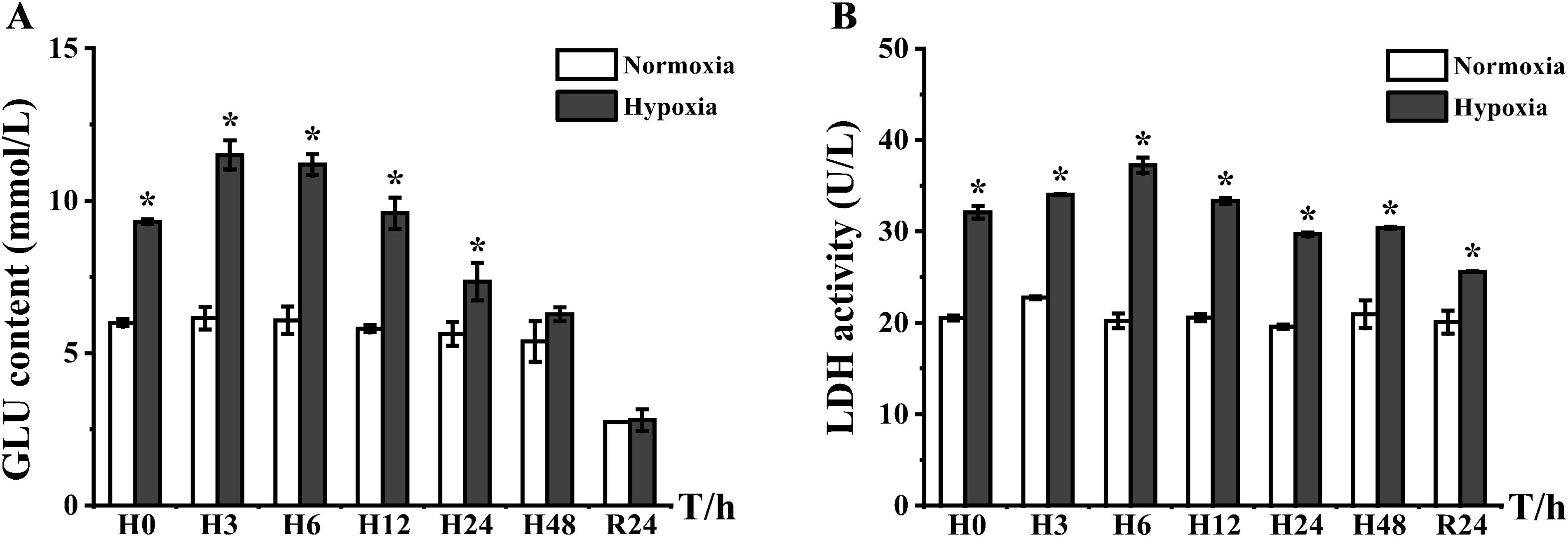
Figure 4. Plot of lactate dehydrogenase activity and blood glucose levels in serum of E coioides: (A) GLU and (B) LDH. The results were analyzed by SPSS analysis. Data were presented as the mean ± SEM of three individual fish (n = 3). Asterisks (*) indicate significant differences (p < 0.05).
3.4 Effects on energy metabolism indices in the liver
TP measurements revealed significant changes in tissue proteins in the hypoxic experimental group at H6 and R24 (Figure 5A). The glycogen content in the liver appeared to decrease at H12, when the fish broke down hepatic glycogen to provide energy. Immediately following the period from H24 to R24, energy storage and the glycogen content in liver increased; at R24, the liver glycogen content of the experimental group was 1.39-fold higher than that of the control group (Figure 5B). PK and HK, which are directly related to the glycolytic pathway, were both highly expressed at H3; however, after H24, PK activity was restored to the same level as that of the control group (Figure 5C). Conversely, HK activity was downregulated, and its expression did not return to the same level as the control group even after DO recovery (Figure 5D).
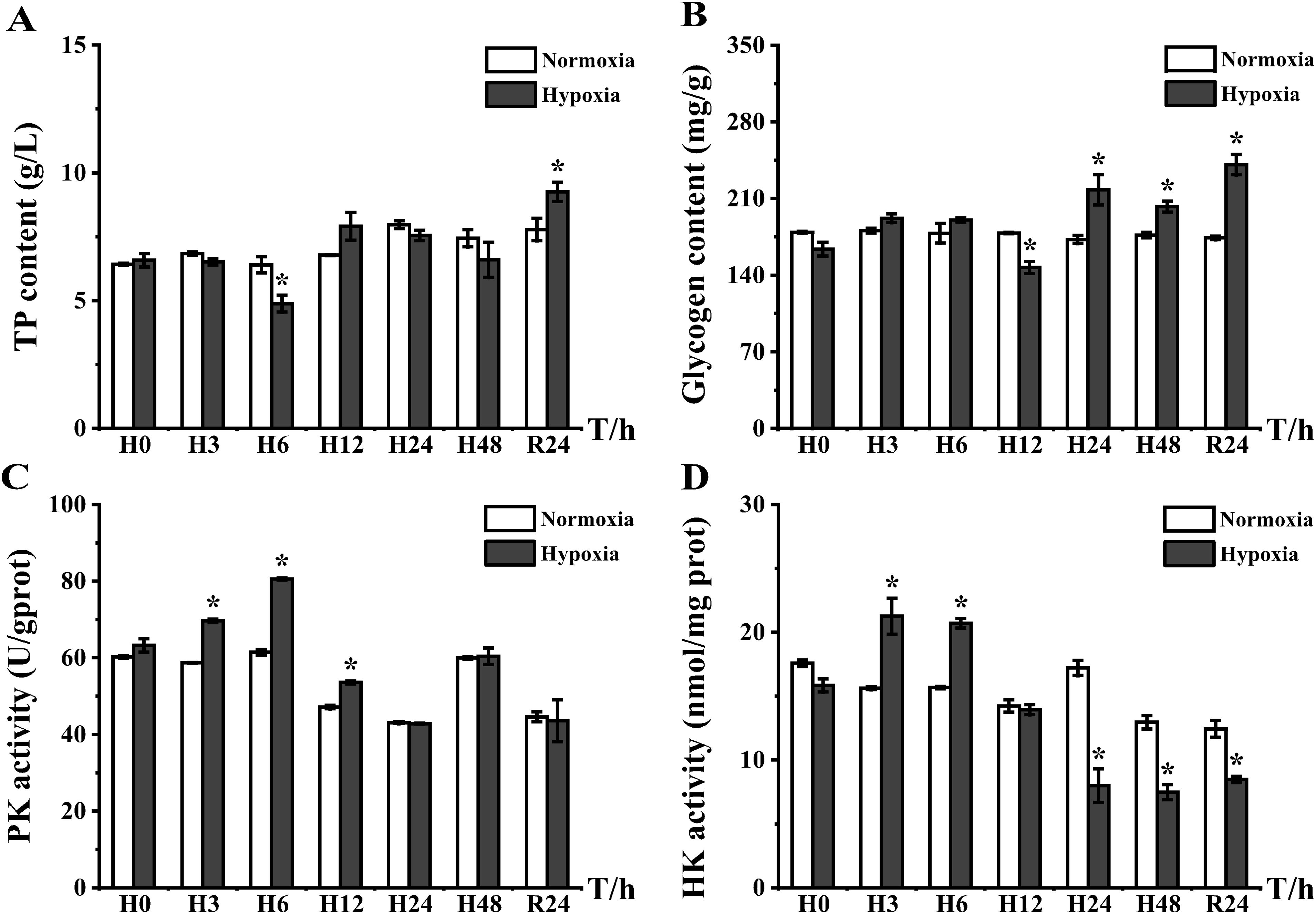
Figure 5. Plots of total protein content, liver glycogen content, and pyruvate kinase and hexokinase activities in the liver of the E coioides: (A) TP, (B) glycogen, (C) PK, and (D) HK. The results were analyzed by SPSS analysis. Data are presented as the mean ± SEM of three individual fish (n = 3). Asterisks (*) indicate significant differences (p < 0.05).
3.5 Effects of hypoxic stress on energy metabolism genes
mRNA expression levels of three proteins related to energy metabolism, i.e., monocarboxylate transporter 2 (MCT2), glucose transporter 1 (GLUT1), and recombinant lactate dehydrogenase A (LDHA), were analyzed in the liver and all exhibited elevated expression levels. MCT2 was significantly upregulated from H0 to R24 and reached a peak at H6 with 6.5-fold (Figure 6A). GLUT1 was highly expressed from H0 to H48, and peaked at H12, with 16.17-fold higher expression than that of the control group (Figure 6B). LDHA showed high expression from H3 to H24, and its peak expression at H6 was 25.69-fold higher than that in the control group (Figure 6C). GLUT1 and LDHA were gradually restored to normal levels with prolonged stress and DO recovery.
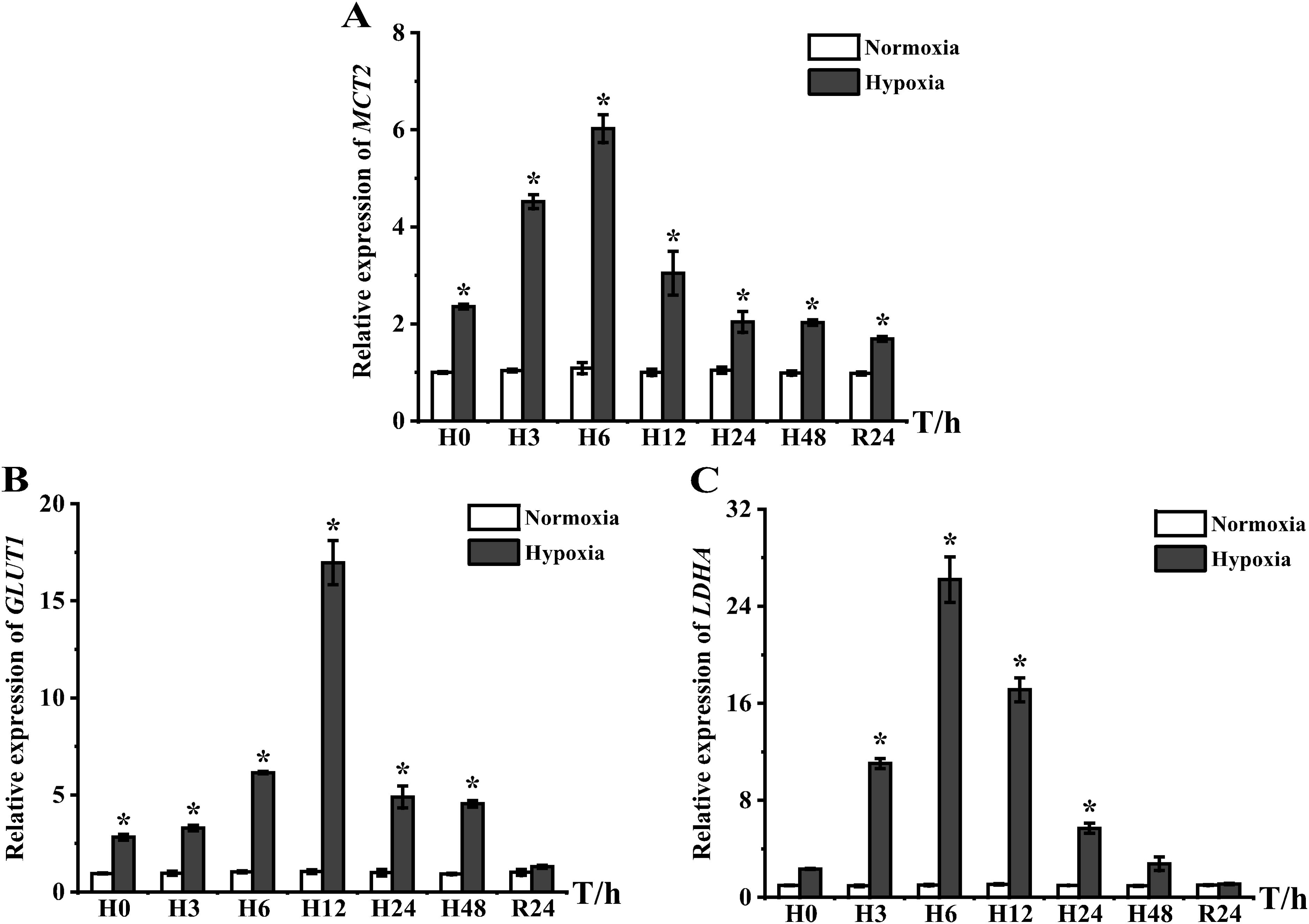
Figure 6. Expression of energy metabolism genes in liver tissues of E coioides: (A) MCT2, (B) GLUT1, and (C) LDHA. The results were analyzed by SPSS analysis. Data are presented as the mean ± SEM of three individual fish (n = 3). Asterisks (*) indicate significant differences (p < 0.05).
3.6 Effects of hypoxic stress on apoptotic genes
Acute hypoxic stress results in elevated mRNA expression levels of bcl2-associated X protein (Bax), B-cell lymphoma-2 (Bcl-2), apoptosis-related cysteine protease 9 (Caspase-9), and the tumor suppressor gene p53 (p53). All four apoptosis-related regulatory genes showed high expression in the liver, which was significantly different from that in the control group at H3, and all reached a peak at H6 (Figure 7). Notably, p53 showed low expression at the beginning of the hypoxia experiment and then continued to increase, reaching a peak at H6 with 2.52-fold higher expression than that of the control group, and recovered to the same level as the control group at H24 (Figure 7A). Bax and Bcl-2 showed higher expression than the control group from H3−H48 and recovered to the same level as the control group after DO recovery. Expression peaks of Bax and Bcl-2 appeared at H6, which were 4.05- and 8.85-fold higher than that of the control group, respectively (Figures 7B, C). Caspase-9 also showed significantly higher expression from H3, reaching a peak of 4.53-fold higher expression than that of the control group at H6, and recovered at H48 (Figure 7D).
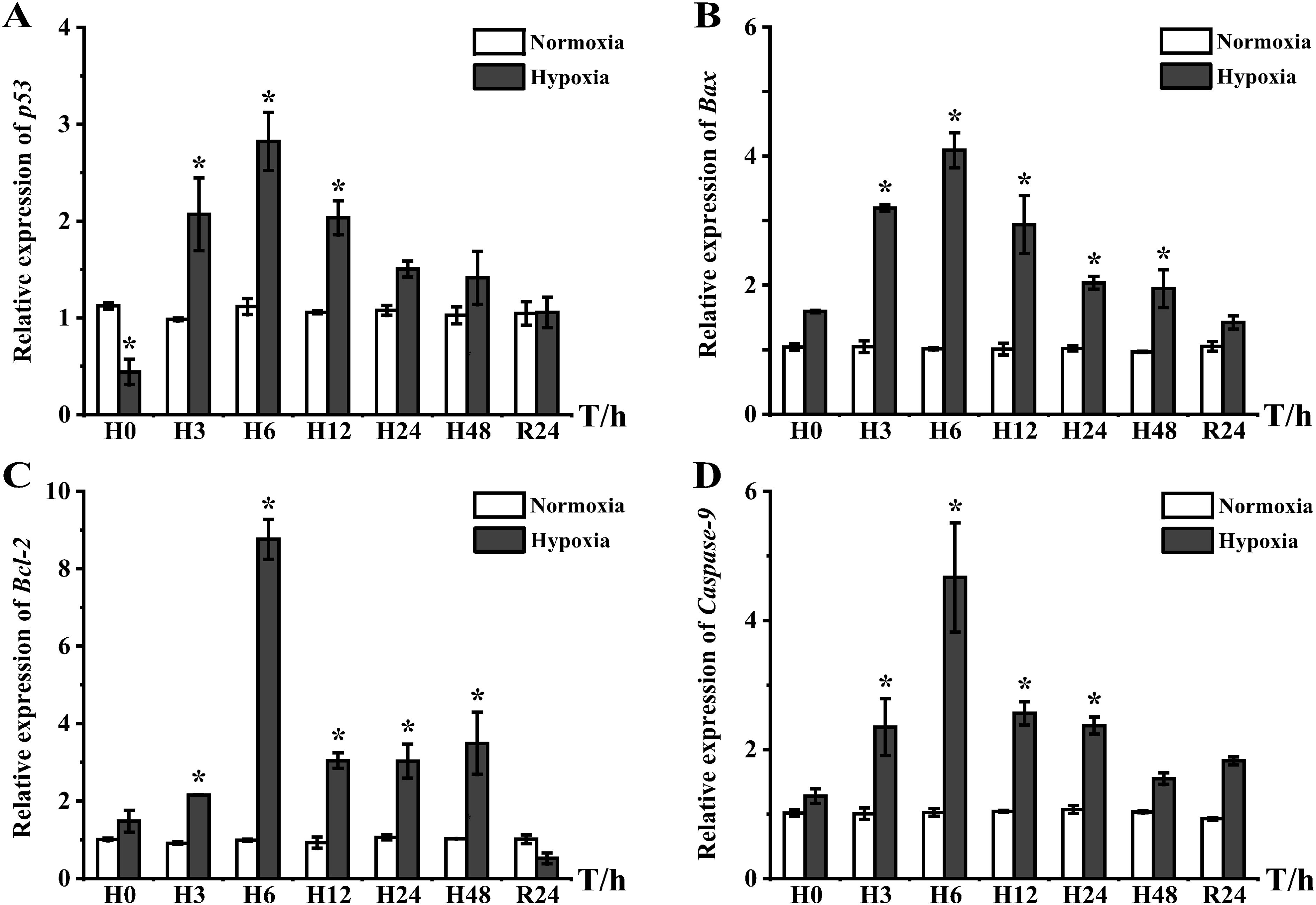
Figure 7. Expression of apoptosis-regulated genes in liver tissues of E coioides: (A) p53, (B) Bax, (C) Bcl-2, and (D) Caspase-9. The results were analyzed by SPSS analysis. Data are presented as the mean ± SEM of three individual fish (n = 3). Asterisks (*) indicate significant differences (p < 0.05).
4 Discussion
Oxygen-sensing signaling pathways enhance tolerance to hypoxia by activating transcription or repressing translation. Fish tended to respond to the stimulation of low DO by enhancing immune defense mechanisms, a finding consistent with studies on Pseudobagrus ussuriensis (Liu et al., 2024) and Larimichthys crocea (Wang et al., 2017). Recently, enormous economic losses have occurred due to the frequent occurrence of hypoxia in marine environments (Sobocinski et al., 2018). Therefore, in this study, we investigated the effects of hypoxic stress on energy metabolism, immune responses, and apoptosis in orange-spotted groupers.
Activity of AST, ALT, and AKP enzymes in extracellular fluid or serum is a sensitive indicator of liver function, and when elevated, it is suggestive of minor cellular damage or stressful tissue injury. They are also key indicators of the nutritional and health status of animals (Palanivelu et al., 2005; Akbary et al., 2018). ALT and AST are positively correlated with the lysis of hepatocytes and generally accepted as relevant stress indicators as biomarkers for the diagnosis of liver function damage (Dassarma et al., 2018). When animals’ liver was damaged and hepatic cells are broken, ALT and AST in hepatic cells would be elevated in the blood (Sheikhzadeh et al., 2011). Activities of AST, ALT, and AKP are susceptible to hypoxia (Li et al., 2022). Hypoxia induces increased AST and ALT activities in Sebastes schlegelii (Jia et al., 2023). As shown in Figure 2, hypoxic stress resulted in high AST levels in serum, which has also been reported in Hyphessobrycon callistus (Pan et al., 2010), suggesting that AST is more sensitive to hypoxic stress. AKP plays a protective role in physiological functions and immune defense, and elevated AKP activity in serum indicates impaired cell membrane permeability and integrity, leading to cellular damage and host hepatobiliary inflammation (Lin et al., 2015). Consequently, fish function could not be restored to the same level as that of the control group after DO restoration, which was the reason for the high AKP expression in serum at H48 and R24, suggesting that the liver had already suffered a more severe inflammatory injury at this time. The increased AKP activity, which is used as an indicator of immune status, also showed modulation of fish immunity by hypoxia (Ren et al., 2022). These results suggested that E. coioides activated an immune response to hypoxic stress.
Enzyme expression in serum suggested that hypoxia led to a significant immune response in the fish, which was confirmed by the expression of inflammatory factor mRNAs. Interferons (IFNs) and interleukins (ILs) are important factors in the immune system; IFNγ is a type of excreted protein that induces antiviral activity and is involved in apoptosis and cellular immunity regulation (Yang et al., 2017), and IL-8 acts as a chemokine that primarily mediates activity of neutrophils, T-lymphocytes, and basophils in vitro (Whyte, 2007). In zebrafish, hypoxia regulates the migration of neutrophils to the injured site to play an inflammatory response by upregulating the expression of IL8. The long-term effects of hypoxia were regulated by increasing the phosphorylation of ERK (He et al., 2022). In our experiments, IFNγ showed a peak from H0−H6 (Figure 3A), and IL-8 showed high expression from H3−H24 (Figure 3B), suggesting that the reduction in DO exacerbated the inflammatory response, which is similar to experimental results in Danio rerio (He et al., 2022) and Oreochromis niloticus (Dawood et al., 2021). Unlike IFNγ and IL-8 expression, the MyD88 gene not only showed high expression at H3 and H6 but also showed increased expression after reoxygenation (Figure 3C). NF-κB can be activated by MyD88 as a downstream molecule, and we found that NF-κB was significantly expressed throughout the hypoxia phase (Figure 3D). NF-κB is a protein complex that controls transcription of many genes and is involved in cellular responses to stimuli such as external environmental stresses, ROS, and cytokines (Moniruzzaman et al., 2018). Notably, NF-κB can induce transcriptional expression of HIF-1α, which is involved in the function of innate immunity and inflammatory response in hypoxic environments (Rius et al., 2008). Our previous studies also found that hypoxia-induced HIF-1α expression (Wu et al., 2023). This result indicated that hypoxia induces the expression of NF-κB, promotes the expression of HIF-1α, and thereby regulates hypoxia stress. Together, these results indicated that hypoxia activated the immune response by upregulating cytokines.
Results from previous transcriptomic analyses of fish livers have shown that hypoxic stress alters the energy metabolic pattern of oxidative phosphorylation in fish, which tends to enhance glycolysis/gluconeogenesis and pyruvate metabolism pathways (Qi et al., 2018; Ding et al., 2020; Lai et al., 2022). During anaerobic glycolysis, sugars are first broken down into glucose, which is then catalyzed by the enzyme LDH to catalyze the primary product (i.e., pyruvate) into lactic acid and produce ATP (Mizock, 1995). The gene HIF-1α, which is closely related to hypoxia, serves as an important factor in glucose metabolism that also activates a variety of enzymes required for glycolysis (e.g., LDA) under hypoxic conditions (Yang et al., 2019). Higher blood glucose levels during hypoxic exposure suggested that glucose metabolism played an important role in energy supply under hypoxic stress (Figure 4A). The high LDH expression in serum also showed that anaerobic glycolysis pattern was continued until DO recovery (Figure 4B). These results implied that the energy supply under hypoxic conditions may have involved the anaerobic glycolysis pathway.
Under hypoxia, major energy-demanding processes (e.g., protein synthesis) shut down rapidly before expression of proteins required for ATP production is enhanced, indicating that hypoxia triggers metabolic inhibition (Catz and Johnson, 2001). We found that TP in the liver decreased significantly after H6 and increased significantly at R24 (Figure 5A). It has been suggested that the total amount of tissue functional proteins in the liver may be affected by hypoxic stress at these two time points (Gracey and Somero, 2001). Because a 6-h period is a critical time for metabolic changes and cellular imbalance, protein inactivation and damage may have occurred during this time, or energy expenditure may have been reduced by shutting down protein synthesis pathways, which was also shown in Trachinotus ovatus (Wang and Wu, 2024). Hepatic glycogen is an important component of energy storage systems. However, the results of the present study showed that hepatic glycogen levels were significantly reduced at H12 (Figure 5B). We hypothesize that hepatic glycogen was degraded into glucose to maintain life activities. From H24 until R24, the hepatic glycogen content increased as the fish continued to enhance anaerobic glycolysis for energy supply. This increase was a regulation of energy storage that occurred in fish in response to prolonged hypoxia, and promotion of fish recovery, which was also observed in Carassius auratus (Mandic et al., 2008). Hepatic glycogen degradation is also inhibited in hypoxia-tolerant Trachinotus blochii under hypoxic conditions (Resende and Mauro Carneiro Pereira, 2022). Eirocheir sinensis showed increased liver glycogen levels after 24 h of hypoxic stress (Chen et al., 2023). HK and PK (i.e., the key enzymes of glycolysis) showed high expression during short periods of hypoxic stress (Figures 5C, D), which was also observed in Pelteobagrus fulvidraco (Wang et al., 2022). The low HK expression in liver at H24, in conjunction with the glycolytic pathway, suggested that HK acted as the “inlet” of glucose into glycolysis and PK acted as the “outlet” (Ng et al., 2022). The decrease in HK expression implied a tightening of the inlet, and PK expression did not increase. Considering that the aerobic glycolytic pathway was weakened at this point, anaerobic glycolysis becomes the dominant mode of metabolism. These results further suggested that carbohydrates were stored after hypoxic stress to provide energy substrates for different tissues.
Monocarboxylate transporters (MCTs) can help move lactate across cell membranes and thus redistribute carbohydrates (Ngan and Wang, 2009). In the present study, MCT2 expression appeared high during both hypoxic stress and recovery phases, which was related to its involvement in regulation of both aerobic and anaerobic metabolism (Figure 6A). GLUT1 (i.e., a anaerobic metabolism marker gene) is a downstream target gene of HIF-1α, and GLUT1 also shows a high expression pattern in Micropterus salmoides (Yang et al., 2017) and Rachycentron canadum (Wang et al., 2019) under hypoxia (Figure 6B). The upregulation of GLUT-1 exposed to acute hypoxia effectively increased glucose uptake and maintained energy supplementation in hypoxic conditions (Yang et al., 2017). From H3−H24 in the present study, LDHA expression in liver increased (Figure 6C), suggesting that anaerobic glycolysis functioned in liver at this stage, which is similar to the results in M. salmoides (Yang et al., 2019). The enzyme activities and genes discussed above suggested that the hypoxia-induced metabolic pattern shifted from aerobic to anaerobic.
With prolonged hypoxia treatment, the apoptotic index increased, and the expression of apoptotic genes (p53, Bax, Bcl-2, and Caspase-9) was closely related to the degree of tissue necrosis. In black rockfish, hypoxia stress impaired hepatic antioxidant capacity, induced oxidative damage and apoptosis via the p53-bax-bcl2 and the caspase-dependent pathways, but enhanced host immunity by regulating AST and ALT activity and related gene expression to maintain homeostasis (Jia et al., 2023). Hypoxia significantly increased apoptosis in the liver of Pelteobagrus vachelli (Zheng et al., 2021). p53 is associated with the cell cycle and can activate downstream genes to bind to the cell cycle protein–CDK complex and inhibit protein kinase activity, thereby blocking normal cell cycle progression (Imamura et al., 2001; Jones et al., 2005). Hypoxia-inducible factors and p53 regulate each other, and the transcription of these two genes demonstrates the competition law. Initially, with the increasing degree of hypoxia, p53 gradually tended to be enriched from an unstable form and occupied a key position in determining cell fate; however, it was degraded again when sustained high expression of HIF-1α occurred, which is consistent with the experimental process when p53 appeared to have low expression at the first experimental node and was subsequently sustained and elevated (Figure 7A). Furthermore, p53 that is fully activated by HIF-1α simultaneously activates the downstream gene Bax, which jointly promotes cell apoptosis (Wang et al., 2019). Bax and Bcl-2 belong to the Bcl-2 family of proteins that are involved in apoptosis regulation. Bcl-2 has antiapoptotic effects and inhibits most cell death types (Hockenbery et al., 1993); however, Bax is pro-apoptotic and a major inhibitor of Bcl-2 (Jin et al., 2011). The Bcl-2/Bax ratio increased under hypoxic stress and reached twofold at H6 until decreasing at R24 (Figures 7B, C). When the Bcl-2/Bax ratio decreased, fish exhibited a dominant role of Bax, clearing damaged cells in vivo, as also reported for T. blochii (Gu et al., 2023). When exposed to short-term hypoxia, cells upregulate Bcl-2 expression to inhibit apoptosis; whereas, prolonged hypoxia leads to downregulation of Bcl-2 expression to accelerate the apoptotic process (Shroff et al., 2007). A change in the Bcl-2/Bax ratio reflects the antagonistic effect of the two proteins, which contributes to enhanced apoptosis under environmental stress and, in turn, protects cells from excessive death. In addition, Caspase-9 acts as a downstream gene, causing endoplasmic reticulum stress and mitochondrial damage to activate the Cas signaling pathway and induce apoptosis (Liu et al., 2023). Our results showed that Caspase-9 expression significantly increased after hypoxia treatment, suggesting that hypoxia activated the Cas signaling pathway (Figure 7D). Three apoptosis-related genes (i.e., Bax, Bcl-2, and Caspase-9) were upregulated under hypoxia, suggesting that the body activates the Bax-Bcl2 and Cas pathways to promote cells to enter the apoptotic program to adapt to hypoxic stress. Furthermore, the transcriptional expression of Bcl-2 is regulated by NF-κB, which can activate the Bcl-2 promoter to promote gene expression (Catz and Johnson, 2001), which is consistent with the expression pattern of these genes in the present study. This suggested that apoptosis and innate immune responses acted simultaneously under hypoxia to maintain homeostasis.
5 Conclusions
The results of the present study indicated that environmental hypoxia, followed by reoxygenation, had serious effects on the liver of E. coioides. Hypoxia affected the activities of glycolytic enzymes and immunoenzymes in serum and the liver and induced fluctuating expression of genes involved in energy metabolism, inflammatory response, and cellular regulation in the liver. To adapt to a low-oxygen environment, E. coioides used anaerobic glycolysis as an important metabolic energy supply pathway and activated the immune response and apoptosis to maintain homeostasis.
Data availability statement
The original contributions presented in the study are included in the article/supplementary material. Further inquiries can be directed to the corresponding authors.
Ethics statement
All animal handling procedures and experimental protocols were approved by the Experimental Animal Ethics Committee of Guangzhou University of China. The study was conducted in accordance with the local legislation and institutional requirements. The study was conducted in accordance with the local legislation and institutional requirements.
Author contributions
YW: Writing – original draft, Data curation. YL: Writing – original draft, Investigation. BL: Writing – review & editing. YH: Writing – review & editing, Supervision. ZY: Writing – review & editing. YM: Writing – review & editing. YF: Writing – review & editing, Data curation. QC: Writing – review & editing, Validation, Data curation. AG: Writing – review & editing, Validation, Supervision, Methodology. HS: Writing – review & editing, Supervision, Resources, Funding acquisition.
Funding
The author(s) declare financial support was received for the research, authorship, and/or publication of this article. This study was supported by grants from Science and Technology Program of Guangdong Province (2019B030316022), Research on breeding technology of candidate species for Guangdong modern marine ranching (2024-MRB-00-001), Guangdong Financial Budget (2023) No. 6, and China-ASEAN Fisheries Resources Conservation and Exploitation (CAMC-2018F). The Natural Science Foundation of China (No. 42177262), 2023 National Undergraduate Innovation and Entrepreneurship Training Program (202311078043) and the “2 + 5” platform funding support from Guangzhou University.
Acknowledgments
We thank Chong Han and Ruiqi Liu for valuable input on the manuscript.
Conflict of interest
The authors declare that the research was conducted in the absence of any commercial or financial relationships that could be construed as a potential conflict of interest.
Publisher’s note
All claims expressed in this article are solely those of the authors and do not necessarily represent those of their affiliated organizations, or those of the publisher, the editors and the reviewers. Any product that may be evaluated in this article, or claim that may be made by its manufacturer, is not guaranteed or endorsed by the publisher.
References
Abdel-Tawwab M., Monier M. N., Hoseinifar S. H., Faggio C. (2019). Fish response to hypoxia stress: growth, physiological, and immunological biomarkers. Fish Physiol. Biochem. 45, 997–1013. doi: 10.1007/s10695-019-00614-9
Akbary P., Sartipi Yarahmadi S., Jahanbakhshi A. (2018). Hematological, hepatic enzymes’ activity and oxidative stress responses of gray mullet (Mugil cephalus) after sub-acute exposure to copper oxide. Environ. Sci. pollut. Res. Int. 25, 1800–1808. doi: 10.1007/s11356-017-0582-1
Bayele H. K., Peyssonnaux C., Giatromanolaki A., Arrais-Silva W. W., Mohamed H. S., Collins H., et al. (2007). HIF-1 regulates heritable variation and allele expression phenotypes of the macrophage immune response gene SLC11A1 from a Z-DNA forming microsatellite. Blood 110, 3039–3048. doi: 10.1182/blood-2006-12-063289
Boussinet E., Nachón D. J., Sottolichio A., Lochet A., Stoll S., Bareille G., et al. (2024). Juvenile downstream migration patterns of an anadromous fish, allis shad (Alosa alosa), before and after the population collapse in the Gironde system, France. J. Fish Biol. 104, 1054–1066. doi: 10.1111/jfb.15647
Breitburg D., Levin L. A. (2018). Declining oxygen in the global ocean and coastal waters. Science 359. doi: 10.1126/science.aam7240
Catz S. D., Johnson J. L. (2001). Transcriptional regulation of bcl-2 by nuclear factor kappa B and its significance in prostate cancer. Oncogene 20, 7342–7351. doi: 10.1038/sj.onc.1204926
Chen Z., Chen J., Li Y., Wang B., Lu Y., Jian J., et al. (2024). Functional properties of ATPIF1 in the orange-spotted grouper (Epinephelus coioides) in response to viral infection. Fish Shellf. Immun. 145, 109329. doi: 10.1016/j.fsi.2023.109329
Chen X., Feng W., Yan F., Li W., Xu P., Tang Y. (2023). Alteration of antioxidant status, glucose metabolism, and hypoxia signal pathway in Eirocheir sinensis after acute hypoxic stress and reoxygenation. Comp. Biochem. Physiol. C Toxicol. Pharmacol. 268, 109604. doi: 10.1016/j.cbpc.2023.109604
Cuninghame S., Jackson R., Lees S. J., Zehbe I. (2017). Two common variants of human papillomavirus type 16 E6 differentially deregulate sugar metabolism and hypoxia signalling in permissive human keratinocytes. J. Gen. Virol. 98, 2310–2319. doi: 10.1099/jgv.0.000905
Dassarma B., Nandi D. K., Gangopadhyay S., Samanta S. (2018). Hepatoprotective effect of food preservatives (butylated hydroxyanisole, butylated hydroxytoluene) on carbon tetrachloride-induced hepatotoxicity in rat. Toxicol. Rep. 5, 31–37. doi: 10.1016/j.toxrep.2017.12.009
Dawood M. A. O., Noreldin A. E., Sewilam H. (2021). Long term salinity disrupts the hepatic function, intestinal health, and gills antioxidative status in Nile tilapia stressed with hypoxia. Ecotoxicol. Environ. Saf. 220, 112412. doi: 10.1016/j.ecoenv.2021.112412
Diaz R. J., Rosenberg R. (2008). Spreading dead zones and consequences for marine ecosystems. Science 321, 926–929. doi: 10.1126/science.1156401
Ding J., Liu C., Luo S., Zhang Y., Gao X., Wu X., et al. (2020). Transcriptome and physiology analysis identify key metabolic changes in the liver of the large yellow croaker (Larimichthys crocea) in response to acute hypoxia. Ecotoxicol. Environ. Saf. 189, 109957. doi: 10.1016/j.ecoenv.2019.109957
Domenici P., Steffensen J. F., Marras S. (2017). The effect of hypoxia on fish schooling. Philos. Trans. R. Soc Lond. B Biol. Sci. 372. doi: 10.1098/rstb.2016.0236
Evans J. J., Shoemaker C. A., Klesius P. H. (2003). Effects of sublethal dissolved oxygen stress on blood glucose and susceptibility to Streptococcus agalactiae in Nile Tilapia Oreochromis niloticus. J. Aquat. 15, 202–208. doi: 10.1577/H03-024
Gracey A. Y., Somero G. N. (2001). Hypoxia-induced gene expression profiling in the euryoxic fish Gillichthys mirabilis. Proc. Natl. Acad. Sci. U. S. A. 98, 1993–1998. doi: 10.1073/pnas.98.4.1993
Gu Y., Sun J. L., Yao F. C., Jiang T., Jin C. X., Shi L. P., et al. (2023). Long-term hypoxia and reoxygenation induced oxidative stress lead to immunosuppression and apoptosis in golden pompano (Trachinotus blochii). Front. Mar. Sci 10. doi: 10.3389/fmars.2023.1212571
Guo Y., Tan J. (2019). Effects of microvesicles on cell apoptosis under hypoxia. Oxid. Med. Cell Longev. 2019, 5972152. doi: 10.1155/2019/5972152
He M., Sun S., Chen A. Q., Lv S. J., Qiu C. Z., Wei M. L., et al. (2022). Hypoxia regulates cytokines expression and neutrophils migration by ERK signaling in zebrafish. Fish Shellf. Immun. 125, 212–219. doi: 10.1016/j.fsi.2022.05.006
He J., Yu Y., Qin X. W., Zeng R. Y., Wang Y. Y., Li Z. M., et al. (2019). Identification and functional analysis of the Mandarin fish (Siniperca chuatsi) hypoxia-inducible factor-1α involved in the immune response. Fish Shellf. Immun. 92, 141–150. doi: 10.1016/j.fsi.2019.04.298
He Y., Yu H., Zhang Z., Zhang J., Kang S., Zhang X. (2022). Effects of chronic hypoxia on growth performance, antioxidant capacity and protein turnover of largemouth bass (Micropterus salmoides). Aquaculture 561, 738673. doi: 10.1016/j.aquaculture.2022.738673
Hockenbery D. M., Oltvai Z. N., Yin X. M., Milliman C. L., Korsmeyer S. J. (1993). Bcl-2 functions in an antioxidant pathway to prevent apoptosis. Cell 75, 241–251. doi: 10.1016/0092-8674(93)80066-n
Imamura K., Ogura T., Kishimoto A., Kaminishi M., Esumi H. (2001). Cell cycle regulation via p53 phosphorylation by a 5’-AMP activated protein kinase activator, 5-aminoimidazole- 4-carboxamide-1-beta-D-ribofuranoside, in a human hepatocellular carcinoma cell line. Biochem. Biophys. Res. Commun. 287, 562–567. doi: 10.1006/bbrc.2001.5627
Jeong J., Awosile B., Thakur K. K., Stryhn H., Boyce B., Vanderstichel R. (2024). Longitudinal dissolved oxygen patterns in Atlantic salmon aquaculture sites in British Columbia, Canada. Front. Mar. Sci. 10. doi: 10.3389/fmars.2023.1289375
Jia Y., Wang F., Gao Y., Qin H., Guan C. (2023). Hypoxia stress induces hepatic antioxidant activity and apoptosis, but stimulates immune response and immune-related gene expression in black rockfish Sebastes schlegelii. Aquat. Toxicol 258, 106502. doi: 10.1016/j.aquatox.2023.106502
Jin Y., Zheng S., Pu Y., Shu L., Sun L., Liu W., et al. (2011). Cypermethrin has the potential to induce hepatic oxidative stress, DNA damage and apoptosis in adult zebrafish (Danio rerio). Chemosphere 82, 398–404. doi: 10.1016/j.chemosphere.2010.09.072
Jones R. G., Plas D. R., Kubek S., Buzzai M., Mu J., Xu Y., et al. (2005). AMP-activated protein kinase induces a p53-dependent metabolic checkpoint. Mol. Cell 18, 283–293. doi: 10.1016/j.molcel.2005.03.027
Klaus M., Karlsson J., Seekell D. (2021). Tree line advance reduces mixing and oxygen concentrations in arctic-alpine lakes through wind sheltering and organic carbon supply. Glob. Change Biol. 27, 4238–4253. doi: 10.1111/gcb.15660
Lai X. X., Zhang C. P., Wu Y. X., Yang Y., Zhang M. Q., Qin W. J., et al. (2022). Comparative transcriptome analysis reveals physiological responses in liver tissues of Epinephelus coioides under acute hypoxia stress. Comp. Biochem. Physiol. Part D Genomics Proteomics 43, 101005. doi: 10.1016/j.cbd.2022.101005
Li X., Liu B., Yang J., Li G., Wen H., Zhang M., et al. (2022). DNA methylation in promoter region of immune related genes STAT3 and VEGFA and biochemical parameters change in muscle of Japanese flounder under acute hypoxia. Dev. Comp. Immunol. 129, 104295. doi: 10.1016/j.dci.2021.104295
Lin H., Zhou X., Niu C., Xia J., Huang D., Wang Z., et al. (2015). Effect of dietary arginine levels on the growth performance, feed utilization, non-specific immune response and disease resistance of juvenile golden pompano Trachinotus ovatus. Aquaculture 437, 382-389. doi: 10.1016/j.aquaculture.2014.12.025
Liu H., Dong X., Chi S., Yang Q., Zhang S., Chen L., et al. (2016). Molecular cloning of glucose transporter 1 in grouper Epinephelus coioides and effects of an acute hyperglycemia stress on its expression and glucose tolerance. Fish Physiol. Biochem. 43, 103–114. doi: 10.1007/s10695-016-0271-x
Liu Q., Li Y., Cao Y., Gu L., Li T., Liu Y., et al. (2024). Transcriptome analysis of brain and skin reveals immune responses to acute hypoxia and reoxygenation in Pseudobagrus ussuriensis. Animals 14. doi: 10.3390/ani14020246
Liu Q., Wang H., Ge J., Li L., Luo J., He K., et al. (2023). Chronic hypoxia and Cu(2+) exposure induce gill remodeling of largemouth bass through endoplasmic reticulum stress, mitochondrial damage and apoptosis. Aquat. Toxicol. 255, 106373. doi: 10.1016/j.aquatox.2022.106373
Luo S.-W., Kang H., Kong J.-R., Xie R.-C., Liu Y., Wang W.-N., et al. (2017). Molecular cloning, characterization and expression analysis of (B-cell lymphoma-2) Bcl-2 in the orange-spotted grouper (Epinephelus coioides). Dev. Comp. Immunol. 76, 150–162. doi: 10.1016/j.dci.2017.06.003
Mandic M., Lau G. Y., Nijjar M. M., Richards J. G. (2008). Metabolic recovery in goldfish: A comparison of recovery from severe hypoxia exposure and exhaustive exercise. Comp. Biochem. Physiol. C Toxicol. Pharmacol. 148, 332–338. doi: 10.1016/j.cbpc.2008.04.012
Mizock B. A. (1995). Alterations in carbohydrate metabolism during stress: a review of the literature. Am. J. Med. 98, 75–84. doi: 10.1016/s0002-9343(99)80083-7
Moniruzzaman M., Ghosal I., Das D., Chakraborty S. B. (2018). Melatonin ameliorates H(2)O(2)-induced oxidative stress through modulation of Erk/Akt/NFkB pathway. Biol. Res. 51, 17. doi: 10.1186/s40659-018-0168-5
Ng Y. S., Lee D. Y., Liu C. H., Tung C. Y., He S. T., Wang H. C. (2022). White spot syndrome virus triggers a glycolytic pathway in Shrimp immune cells (hemocytes) to benefit its replication. Front. Immunol. 13. doi: 10.3389/fimmu.2022.901111
Ngan A. K., Wang Y. S. (2009). Tissue-specific transcriptional regulation of monocarboxylate transporters (MCTs) during short-term hypoxia in zebrafish (Danio rerio). Comp. Biochem. Physiol. B Biochem. Mol. Biol. 154, 396–405. doi: 10.1016/j.cbpb.2009.08.003
Nguyen P. T. D., Giovanni A., Maekawa S., Pham T. H., Wang P.-C., Chen S.-C. (2023). An Integrated in silico and in vivo study of nucleic acid vaccine against Nocardia seriolae infection in orange-spotted grouper Epinephelus coioides. Fish Shellf. Immun. 143, 109202. doi: 10.1016/j.fsi.2023.109202
Palanivelu V., Vijayavel K., Balasubramanian S. E., Balasubramanian M. P. (2005). Influence of insecticidal derivative (cartap hydrochloride) from the marine polycheate on certain enzyme systems of the fresh water fish Oreochromis mossambicus. J. Environ. Biol. 26, 191–195. Available online at: https://imsear.searo.who.int/handle/123456789/113773.
Palazon A., Goldrath A. W., Nizet V., Johnson R. S. (2014). HIF transcription factors, inflammation, and immunity. Immunity 41, 518–528. doi: 10.1016/j.immuni.2014.09.008
Pan C. H., Chien Y. H., Wang Y. J. (2010). The antioxidant capacity response to hypoxia stress during transportation of characins (Hyphessobrycon callistus Boulenger) fed diets supplemented with carotenoids. Aquac. Res. 41, 973–981. doi: 10.1111/j.1365-2109.2009.02380.x
Qi D., Chao Y., Wu R., Xia M., Chen Q., Zheng Z. (2018). Transcriptome analysis provides insights into theadaptive responses to hypoxia of a schizothoracine fish (Gymnocypris eckloni). Front. Physiol. 9. doi: 10.3389/fphys.2018.01326
Qi Z.-H., Liu Y.-F., Luo S.-W., Chen C.-X., Liu Y., Wang W.-N. (2013). Molecular cloning, characterization and expression analysis of tumor suppressor protein p53 from orange-spotted grouper, Epinephelus coioides in response to temperature stress. Fish Shellf. Immun. 35, 1466–1476. doi: 10.1016/j.fsi.2013.08.011
Qiang J., He J., Tao Y. F., Bao J. W., Zhu J. H., Xu P. (2020). Hypoxia-induced miR-92a regulates p53 signaling pathway and apoptosis by targeting calcium-sensing receptor in genetically improved farmed tilapia (Oreochromis niloticus). PloS One 15, e0238897. doi: 10.1371/journal.pone.0238897
Qiu J., Zhang C., Lv Z., Zhang Z., Chu Y., Shang D., et al. (2024). Analysis of changes in nutrient salts and other water quality indexes in the pond water for largemouth bass (micropterus salmoides) farming. Heliyon 10, e24996. doi: 10.1016/j.heliyon.2024.e24996
Ren Y., Men X., Yu Y., Li B., Zhou Y., Zhao C. (2022). Effects of transportation stress on antioxidation, immunity capacity and hypoxia tolerance of rainbow trout (Oncorhynchus mykiss). Aquacult. Rep. 22. doi: 10.1016/j.aqrep.2021.100940
Resende A. C., Mauro Carneiro Pereira D. (2022). Effects of heat shock on energy metabolism and antioxidant defence in a tropical fish species Psalidodon bifasciatus. J. Fish Bio. 100, 1245–1263. doi: 10.1111/jfb.15036
Rius J., Guma M., Schachtrup C., Akassoglou K., Zinkernagel A. S., Nizet V., et al. (2008). NF-kappaB links innate immunity to the hypoxic response through transcriptional regulation of HIF-1alpha. Nature 453, 807–811. doi: 10.1038/nature06905
Saha N., Koner D., Sharma R. (2022). Environmental hypoxia: A threat to the gonadal development and reproduction in bony fishes. Aquac. Fish. 7, 572–582. doi: 10.1016/j.aaf.2022.02.002
Scherz-Shouval R., Elazar Z. (2011). Regulation of autophagy by ROS: physiology and pathology. Trends Biochem. Sci. 36, 30–38. doi: 10.1016/j.tibs.2010.07.007
Sheikhzadeh N., Tayefi-Nasrabadi H., Khani Oushani A., Najafi Enferadi M. H. (2011). Effects of Haematococcus pluvialis supplementation on antioxidant system and metabolism in rainbow trout (Oncorhynchus mykiss). Fish Physiol. Biochem. 38, 413–419. doi: 10.1007/s10695-011-9519-7
Shroff E. H., Snyder C., Chandel N. S. (2007). Bcl-2 family members regulate anoxia-induced cell death. Antioxid. Redox Signal. 9, 1405–1409. doi: 10.1089/ars.2007.1731
Sobocinski K. L., Ciannelli L., Wakefield W. W., Yergey M. E., Johnson-Colegrove A. (2018). Distribution and abundance of juvenile demersal fishes in relation to summer hypoxia and other environmental variables in coastal Oregon, USA. Estuar. Coast. Shelf 205, S0272771417304511. doi: 10.1016/j.ecss.2018.03.002
Sun J. L., Liu Y. F., Jiang T., Li Y. Q., Song F. B., Wen X., et al. (2021). Golden pompano (Trachinotus blochii) adapts to acute hypoxic stress by altering the preferred mode of energy metabolism. Aquaculture 542, 736842. doi: 10.1016/j.aquaculture.2021.736842
Wang P., Guan D., Zhang X. P., Liu F., Wang W. (2019). Modeling the regulation of p53 activation by HIF-1 upon hypoxia. FEBS Lett. 593, 2596–2611. doi: 10.1002/1873-3468.13525
Wang Q. F., Shen W. L., Hou C. C., Liu C., Wu X. F., Zhu J. Q. (2017). Physiological responses and changes in gene expression in the large yellow croaker Larimichthys crocea following exposure to hypoxia. Chemosphere 169, 418–427. doi: 10.1016/j.chemosphere.2016.11.099
Wang S., Sun M., Ning Z., Chen Y., Zhou H., Mu W. (2023). The effects of sustained and diel-cycling hypoxia on high-latitude fish Phoxinus lagowskii. Comp. Biochem. Physiol. Part D Genomics Proteomics 45, 101059. doi: 10.1016/j.cbd.2023.101059
Wang J., Wang M., Li B., Guo H., Zhu X., Zhang L. (2022). The combined effect of acute hypoxic stress and feeding status on the metabolism of yellow catfish (Pelteobagrus fulvidraco). Aquaculture 560, 738605. doi: 10.1016/j.aquaculture.2022.738605
Wang Q. H., Wu R. X. (2024). Integrated transcriptomics and metabolomics reveal changes in cell homeostasis and energy metabolism in Trachinotus ovatus in response to acute hypoxic stress. Int. J. Mol. Sci. 25. doi: 10.3390/ijms25021054
Wang J., Zhang W., Dong X., Wang H., Zhang S. (2019). Molecular cloning, characterization and expression analysis of glucose transporters from Rachycentron canadum. Aquac. Res. 50. doi: 10.1111/are.14205
Wannamaker C. M., Rice J. A. (2000). Effects of hypoxia on movements and behavior of selected estuarine organisms from the southeastern United States. J. Exp. Mar. Biol. Ecol. 249, 145–163. doi: 10.1016/s0022-0981(00)00160-x
Wen H., Liu Q., Xie J., Wang W., Xiao J. (2016). Characterization of triploid hybrid groupers from interspecies hybridization (Epinephelus coioides ♀ × Epinephelus lanceolatus ♂). Aquac. Res. 47, 2195–2204. doi: 10.1111/are.12672
Whyte S. K. (2007). The innate immune response of finfish–a review of current knowledge. Fish Shellf. Immun. 23, 1127–1151. doi: 10.1016/j.fsi.2007.06.005
Wu Y., Lai X., Lin B., Lin Y., Yang Y., Zhang M., et al. (2023). Transcriptome and hypoxia-responsive gene expression analyses reveal the physiological reaction to acute hypoxia and reoxygenation in Epinephelus coioides. Aquacult. Rep 31, 101655. doi: 10.1016/j.aqrep.2023.101655
Xie L., Gao X., Liu Y., Yang B., Wang B., Zhao J., et al. (2022). Biogeochemical properties and fate of dissolved organic matter in wet deposition: Insights from a mariculture area in North Yellow Sea. Sci. Total Environ. 844, 157130. doi: 10.1016/j.scitotenv.2022.157130
Yang Y., Wang G., Wu W., Yao S., Han X., He D., et al. (2018). Camalexin induces apoptosis via the ROS-ER stress-mitochondrial apoptosis pathway in AML cells. Oxid. Med. Cell Longev. 2018, 7426950. doi: 10.1155/2018/7426950
Yang S., Wu H., He K., Yan T., Zhou J., Zhao L. L., et al. (2019). Response of AMP-activated protein kinase and lactate metabolism of largemouth bass (Micropterus salmoides) under acute hypoxic stress. Sci. Total Environ. 666, 1071–1079. doi: 10.1016/j.scitotenv.2019.02.236
Yang S., Yan T., Wu H., Xiao Q., Fu H. M., Luo J., et al. (2017). Acute hypoxic stress: Effect on blood parameters, antioxidant enzymes, and expression of HIF-1alpha and GLUT-1 genes in largemouth bass (Micropterus salmoides). Fish Shellf. Immun. 67, 449–458. doi: 10.1016/j.fsi.2017.06.035
Yu H., You X., Li J., Zhang X., Zhang S., Jiang S., et al. (2018). A genome-wide association study on growth traits in orange-spotted grouper (Epinephelus coioides) with RAD-seq genotyping. Sci. China Life Sci. 61, 934–946. doi: 10.1007/s11427-017-9161-4
Yu X. X., Zhang Y. R., Li S. S., Zheng G. D., Zou S. M. (2023). Effects of hypoxia on the gill morphological structure, apoptosis and hypoxia-related gene expression in blunt snout bream (Megalobrama amblycephala). Fish Physiol. Biochem. 49, 939–949. doi: 10.1007/s10695-023-01233-1
Zhai W., Zhao H., Zheng N., Xu Y. (2012). Coastal acidification in summer bottom oxygen-depleted waters in northwestern-northern Bohai Sea from June to August in 2011. Chin. Sci. Bull. 57, 1062–1068. doi: 10.1007/s11434-011-4949-2
Zhang W., Cao Z. D., Peng J. L., Chen B. J., Fu S. J. (2010). The effects of dissolved oxygen level on the metabolic interaction between digestion and locomotion in juvenile southern catfish (Silurus meridionalis Chen). Comp. Biochem. Physiol. A Mol. Integr. Physiol. 157, 212–219. doi: 10.1016/j.cbpa.2010.06.184
Zhang B.-Y., Nie Q.-J., Xu J.-M., Cai G.-H., Ye J.-D., Jin T., et al. (2024). Preventive and reparative potentials of heat-inactivated and viable commensal Bacillus pumilus SE5 in ameliorating the adverse impacts of high soybean meal in grouper (Epinephelus coioides). Fish Shellf. Immun. 153, 109846. doi: 10.1016/j.fsi.2024.109846
Keywords: Epinephelus coioides, hypoxia, reoxygenation, energy metabolism, immune response
Citation: Wu Y, Lin Y, Lin B, Huang Y, Yu Z, Ma Y, Feng Y, Chen Q, Gao A and Shu H (2024) Effects of hypoxia and reoxygenation on energy metabolism, immune response, and apoptosis in orange-spotted grouper (Epinephelus coioides). Front. Mar. Sci. 11:1495068. doi: 10.3389/fmars.2024.1495068
Received: 12 September 2024; Accepted: 07 November 2024;
Published: 13 December 2024.
Edited by:
Tao Wang, Nanjing Normal University, ChinaReviewed by:
Basanta Kumar Das, Central Inland Fisheries Research Institute (ICAR), IndiaAdnan H. Gora, Central Marine Fisheries Research Institute (ICAR), India
Copyright © 2024 Wu, Lin, Lin, Huang, Yu, Ma, Feng, Chen, Gao and Shu. This is an open-access article distributed under the terms of the Creative Commons Attribution License (CC BY). The use, distribution or reproduction in other forums is permitted, provided the original author(s) and the copyright owner(s) are credited and that the original publication in this journal is cited, in accordance with accepted academic practice. No use, distribution or reproduction is permitted which does not comply with these terms.
*Correspondence: Along Gao, YWxnYW9AZ3podS5lZHUuY24=; Hu Shu, c2h1aHUwMDFAMTI2LmNvbQ==