- 1ECOMARE - Laboratory for Innovation and Sustainability of Marine Biological Resources, CESAM - Centre for Environmental and Marine Studies, Department of Biology, University of Aveiro, Aveiro, Portugal
- 2CCMAR - Centro de Ciências do Mar, University of Algarve, Faro, Portugal
- 3GEOBIOTEC - Department of Geosciences, University of Aveiro, Aveiro, Portugal
- 4INMARE - Department of Marine Ecology and Resources, Instituto de Investigaciones Marinas (IIM-CSIC), Vigo, Spain
Seahorses (Hippocampus spp.; Family Syngnathidae) are mainly targeted by Traditional Chinese Medicine, curio and ornamental trade, as dried or live specimens. Traceability tools may help fill the gaps on supply chains, securing information on geographic origin and identification of traded specimens. Fin-clipping, a non-lethal and well-established method in seahorse research, offers a potential approach to trace the geographic origin and certify the aquaculture of these flagship species. As such, this study aimed to investigate the existence of differences in isotopic profiles of four seahorse species cultured at research centers located in southern Portugal and northern Spain, as well as between cultured Hippocampus guttulatus sourced from two research centers, and between wild and cultured specimens of this species. This research also evaluated the potential of combining isotopic and elemental fingerprints for seahorse species discrimination, through inductively continuous-flow isotope ratio mass spectrometry (IR-MS) and plasma mass spectrometry (ICP-MS). Species cultured at the same research centers exhibited similar stable isotope composition (δ13C and δ15N), except in the case of temperate H. guttulatus from northern Spain, which differed significantly from tropical species H. kuda and H. reidi. These differences could be due to phylogenetic dissimilarities and differences in seawater temperature. The δ15N composition allowed to discriminate between cultured H. guttulatus from the two research centers and between cultured and wild specimens. While dorsal fin isotopes alone did not prove to be a reliable tool for the discrimination of different cultured species, combining them with elemental profiles from seahorses’ whole-body allowed to successfully discriminate between H. kuda and H. reidi. This preliminary research demonstrates the potential of stable isotope and elemental analyses for tracing seahorses’ geographic origin and species identification. However, further research should be performed to validate these findings for wild specimens, particularly those from illegal, unreported and unregulated (IUU) fisheries and trade.
1 Introduction
Seahorses (Hippocampus spp.) are marine teleosts that belong to family Syngnathidae, alongside pipefishes, pipehorses and seadragons (Foster and Vincent, 2004). These flagship species, whose appealing looks may contribute to their conservation, along with that of habitats they occupy (Cohen et al., 2017), are particularly vulnerable to anthropogenic pressures (Correia et al., 2015), such as habitat degradation (Vivas et al., 2023; Watchorn et al., 2022), overfishing (Cohen et al., 2017) and bycatch (Vaidyanathan and Vincent, 2021). Features such as reduced mobility, patchy distribution, habitat fidelity, monogamy and low fecundity exacerbate their vulnerability (Foster and Vincent, 2004). Hippocampus spp. were included, in 1996, in the Red List of Threatened Species from International Union for Conservation of Nature (IUCN) (Vincent et al., 2011).Two seahorse species, from the forty-two already described, are listed as endangered (EN), twelve listed as vulnerable (VU) and seventeen species, for which available information is scarce, are classified as Data Deficient (DD) (IUCN, 2023). Seahorses were also formally added in 2004, to Appendix II of the Convention on International Trade in Endangered Species of Wild Fauna and Flora (CITES), due to high pressure exerted on wild populations. Species included in Appendix II of CITES may be traded, with national government’s approval, as long as these practices do not add pressure to wild populations (Kuo et al., 2018). Seahorses are extensively traded, supplying demand in the form of dried specimens, targeted by Traditional Chinese Medicine (TCM) and curio trade (Boehm et al., 2023), as well as live specimens destined to the marine aquarium trade (Kuo and Vincent, 2018).
Traceability tools may contribute to fill gaps, along supply chains, and potentially provide origin certification, allowing consumers to make informed and sustainable choices (Cohen et al., 2013; Duarte et al., 2022; Leal et al., 2015). Initial studies explored the use of bacterial communities from live seahorse skin mucus to trace their geographic origin (Cohen et al., 2018). Additionally, elemental analysis of bony structures (vertebrae and bony plates) showed promise for tracing the geographic origin of dried cultured H. guttulatus specimens (Cabral et al., 2021).
Species identification in syngnathids can be challenging (Curtis et al., 2017), as some species lack distinctive characteristics, and intraspecific variability, such as in color or number of cirri, may hamper species discrimination (Curtis, 2006; Woodall et al., 2018). Genetic studies have already been performed for seahorse species differentiation, which is of major importance for understanding seahorse life-history and ecology, and the application of effective conservation measures (Luo et al., 2015; Woodall et al., 2018). Metal and element contents have also revealed species-specific differences in two closely related fish species, Sardina pilchardus and Engraulis encrasicolus, sampled at the same geographic locations, indicating that elemental fingerprints could be strongly influenced by species factor (Sofoulaki et al., 2018).
Geochemical tools offer a relatively fast and cost-effective approach compared to other molecular and biochemical tools (Leal et al., 2015), being potentially valuable for the traceability of geographic origin and discrimination of seahorse species apprehended from illegal, unreported, and unregulated (IUU) fisheries and trade. Stable isotope analysis (SIA) is a helpful tool for studying food-webs (Fredriksen, 2003; Fry, 1988; Post, 2002), including seahorse trophic niches and ecological features (Piñeiro-Corbeira et al., 2021; Valladares and Planas, 2020; Valladares et al., 2016). Carbon and nitrogen stable isotope ratios (13C/12C and 15N/14N, respectively) are frequently employed in ecological studies, with carbon indicating food sources and nitrogen reflecting trophic position (Valladares and Planas, 2012). However, consumers typically exhibit higher δ13C and δ15N values than their prey due to the fractionation of lighter isotopes (DeNiro and Epstein (1978, 1981). Given the conservation status of many Hippocampus species (IUCN, 2023), non-lethal techniques such as partial fin-clipping (Piñeiro-Corbeira et al., 2021; Planas, 2021; Planas et al., 2020a; Valladares et al., 2016; Woodall et al., 2012), have been employed for SIA in seahorses, providing a suitable matrix with similar isotopic composition to muscle tissues (Valladares and Planas, 2012).
Stable isotope analysis of dorsal fins could be considered an interesting non-lethal technique to trace and certify the origin of seahorse specimens. As such, to assess the reliability of this tool, this study aimed to: i) describe the dorsal fins’ isotopic fingerprints (δ13C and δ15N) in wild and cultured Hippocampus spp. (H. guttulatus, H. hippocampus, H. reidi and H. kuda); ii) assess the existence of isotopic differences among seahorse species cultured at the same research centers (Instituto de Investigaciones Marinas - IIM, Vigo, Spain or Centro de Ciências do Mar - CCMAR, Faro, Portugal), in cultured H. guttulatus originating from two different research centers (IIM or CCMAR), and between wild H. guttulatus (Ria Formosa coastal lagoon, Portugal) and cultured H. guttulatus; and iii) evaluate the potential of combining dorsal fin isotopes and whole-body elemental fingerprints for species discrimination when isotopic fingerprints alone may not be sufficient.
2 Materials and methods
2.1 Bioethics
Animal capture, handling and sampling followed all bioethical standards of the Spanish Government (Real Decreto 1201/2005, 10th October 2005) and the Regional Government Xunta de Galicia (REGA ES360570202001/15/FUN/BIOL.AN/MPO01). Additionally, the captive breeding program for H. hippocampus and H. guttulatus (Project HIPPONUTRE, reference 16-02-01-FMP-54) was approved by the ethics committee from the Portuguese Veterinary Medicines Directorate for the Ministry of Agriculture, Rural Development and Fisheries. Under this approval, the program was performed in compliance with the Guidelines of the European Union Council (86/609/EU) and Portuguese legislation for the use of laboratory animals. Frozen samples of wild H. guttulatus, were donated by CCMAR. The protocol for fish sacrifice allowed to immediately and rapidly euthanize H. guttulatus specimens with an excess of anesthetic (2-phenoxyethanol solution, 0.40 mg/L). Individuals were placed in the anesthetic solution for at least 20 minutes and removed not less than 10 minutes after ventilation stopped.
2.2 Seahorse samples
Seven wild H. guttulatus were sampled from Ria Formosa coastal lagoon (36°59’ N; 7°51’ W; southern Atlantic coast; Portugal), in 2001. Seven H. hippocampus and six H. guttulatus were cultured at CCMAR (University of Algarve, Faro, Portugal) and died, from natural causes, in the year 2020. These seahorses were maintained in seawater from Ria Formosa coastal lagoon and physicochemical parameters, temperature and salinity, followed seasonal patterns with annual mean values of 20°C and 35.7, respectively. In the present study, the Practical Salinity Scale (PSS) was adopted to report salinity (IAPSO, 1985). Therefore, salinity is expressed as a conductivity ratio and has no units. Hippocampus guttulatus and H. hippocampus were fed on wild mysids Mesopodopsis slabberi and/or Diamysis lagunaris., depending on natural availability. Eight H. guttulatus and nine H. reidi and H. kuda (five replicates of H. reidi and four replicates of H. kuda), were cultured at IIM (CSIC, Vigo, Spain), presenting death dates between 2013 and 2019. These seahorses were cultured in seawater from Ría de Vigo (42°21 N; 8°36’ and 8°54’ W; southwestern Atlantic coast; Galicia, Spain), being maintained at seasonal temperatures of 15°C (winter) and 19°C (summer), in the case of H. guttulatus and at a constant temperature of 26 ± 0.5°C, in the case of H. reidi and H. kuda. The salinity mean value (37 ± 1) was the same for the three species. Specimens cultured at IIM were fed on mixtures of adult enriched Artemia (Iberfrost, Spain), commercially frozen mysids Neomysis sp. (Ocean Nutrition, Spain) and mysids collected from the wild (Siriella armata and Leptomysis sp.).
In the laboratory, seahorse samples frozen at -20°C, were rapidly rinsed with distilled water and freeze-dried (CoolSafe 55-9L Pro, Labogene, Lillerød, Denmark). The dorsal fins were separated from the rest of the body (Figure 1) with the help of stainless-steel tweezers and cut into smaller portions with ceramic blades, before performing isotopic analysis. Tweezers and ceramic blades were cleaned with 99% (w/v) ethanol, between replicates of each species, to avoid cross contamination.
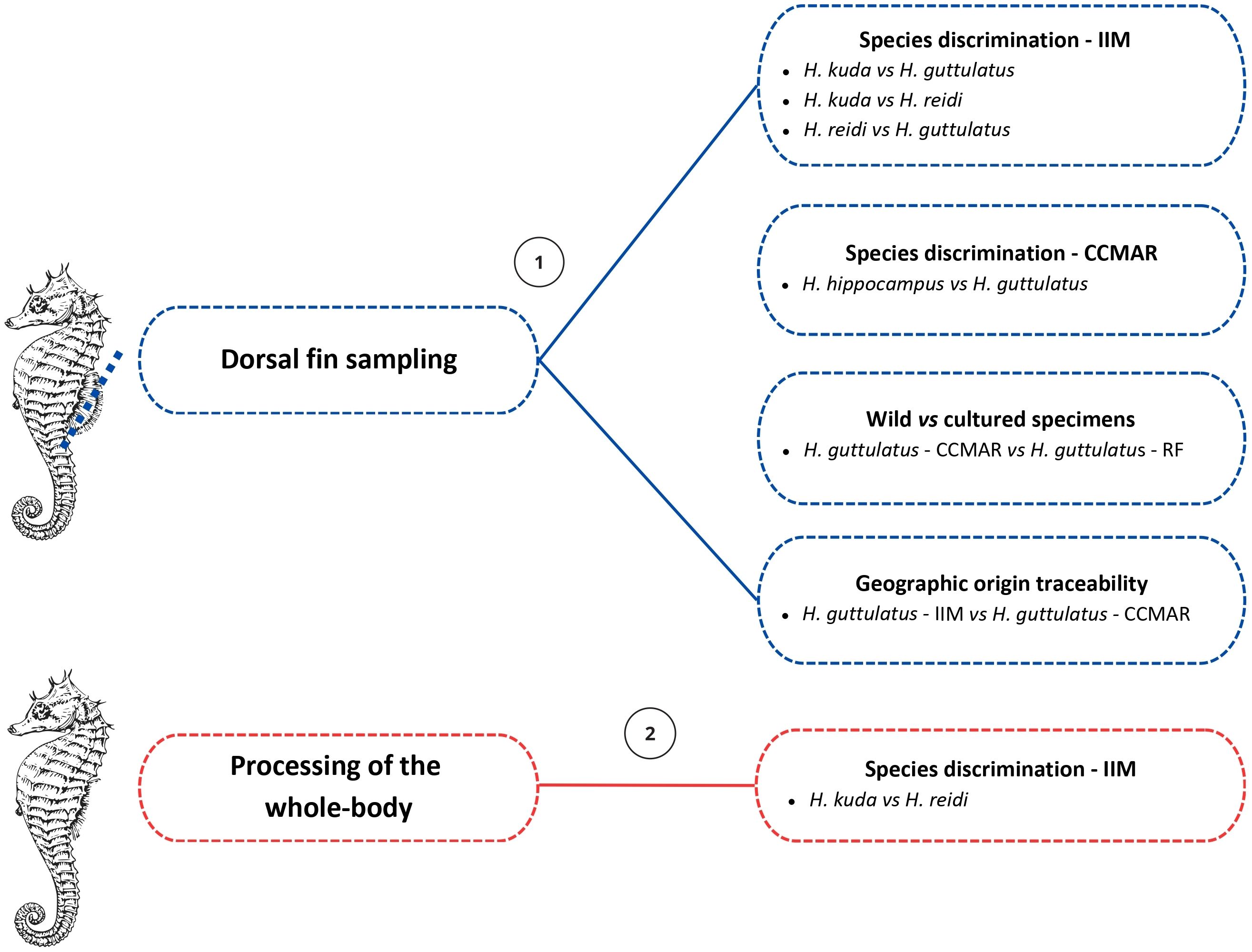
Figure 1. General scheme of the experimental design and matrices (dorsal fin and whole-body) applications. Number 1 denotes for stable isotopic analysis and number 2 for elemental analysis. IIM - Instituto de Investigaciones Marinas (CSIC, Vigo, Spain), CCMAR - Centro de Ciências do Mar (Faro, Portugal) and RF - Ria Formosa coastal lagoon (Portugal). Seahorse figure adapted from the graphical design platform Canva (https://www.canva.com/).
The whole-body replicates from species H. kuda and H. reidi (n = 9) (Figure 1), were cut into smaller portions and manually macerated with liquid nitrogen, using a ceramic mortar and pestle. Samples were then placed in a mortar grinder (RM 200, Retsch, Hann, Germany) and homogenized. The mortar grinder and the ceramic mortar and pestle were cleaned with quartz powder and alcohol (70%), between samples, to avoid cross contamination (Ricardo et al., 2020). For acidic digestion, a subsample from the whole-body, of approximately 0.5 g was employed.
2.3 Stable isotope analysis
Fin samples, used for determining δ13C and δ15N, were transferred to tin capsules and weighed (2.22 ± 0.66 mg). Sample analysis was performed at Servizos de Apoio á Investigación (SAI) from University of A Coruña, by a continuous-flow isotope ratio mass spectrometry equipment (FlashEA1112 elemental analyzer, Thermo Finnigan, Italy) coupled to a Delta Plus mass spectrometer (FinniganMat, Germany) through a Conflo II interface. Carbon and nitrogen isotopic values were expressed as permil (‰), relative to Vienna Pee Dee Belemnite (VPDB) and atmospheric air, as described in the following equation:
X corresponds to 13C or 15N and R to ratios 13C/12C or 15N/14N. International reference materials for 13C (NBS 22, IAEA-CH-6 and USGS24) and 15N (IAEA-N-1, IAEA-N-2 and USGS25) were employed in analytical sample batch runs. To evaluate the precision in the analysis of δ13C and δ15N, acetanilide was used, resulting in a standard deviation of ± 0.15‰ (1-sigma, n = 10). Standards were run in every 10 samples. The isotopic analysis procedure fulfils the requirements of the ISO 9001 standard. The laboratory is submitted to annual intercalibration exercises (e.g., Forensic isotope ratio mass spectrometry scheme - FIRMS, LGC Standards, UK).
2.4 Elemental extraction and ICP-MS analysis
The acidic digestion of the samples consisted in the addition of 3 mL of concentrated nitric acid (HNO3) and 1 mL of hydrochloric acid. After overnight digestion (14 - 16 h), the solutions were placed in a digestion block (DigiPrep, SCP Science; Montreal, QC, Canada) and three heating cycles were performed. These consisted in the increase, for 10 minutes, from room temperature to 50°C, which was kept stable during a period of 15 minutes. Temperature was then increased, in 15 minutes, from 50°C to 85°C and stabilized for another period of 15 minutes. The three heating cycles were interspersed with the addition of 2 mL of hydrogen peroxide. The drying process was performed in the digestion block at a temperature of 50°C. After evaporation, samples were diluted with Millipore water (Milli–Q) to a final concentration of 1-2% HNO3, to reduce acid concentration and avoid damaging the inductively coupled plasma mass spectrometry (ICP-MS) equipment.
Total concentrations of 21 elements, aluminum (Al), arsenic (As), boron (B), barium (Ba), calcium (Ca), cobalt (Co), chromium (Cr), copper (Cu), iron (Fe), potassium (K), lithium (Li), magnesium (Mg), manganese (Mn), sodium (Na), nickel (Ni), phosphorus (P), rubidium (Rb), selenium (Se), strontium (Sr), vanadium (V) and zinc (Zn), were determined in an Agilent 7700 ICP-MS (Agilent Technologies, Santa Clara, CA, USA) equipped with an octopole collision cell and autosampler. Calibrations with standard solutions of each analyte were performed for element quantification. A quality program, with reagent blanks, replicate samples and two certified reference materials, Febs-1 (NRC Canada) and ERM-BB422 (JRC - European Commission), was employed in order to evaluate the accuracy of the ICP-MS method. Precision was estimated by the relative standard deviation (RSD) of replicate samples, being ≤ 10%. The elements’ concentrations were expressed as ratios of Ca (mmol/mol Ca) for posterior statistical analyses (Ricardo et al., 2017).
2.5 Data analysis
Statistical analyses were performed using the software R v.4.3.2 (R Core Team, 2019) and PRIMER v6 (Primer-e, Auckland, New Zealand) with the add-on PERMANOVA+. Niche regions and niche overlap of seahorses cultured in the same research centers (IIM or CCMAR), cultured H. guttulatus from different origins and wild and cultured H. guttulatus, were analyzed through a multidimensional δ13C and δ15N bi-plot. The region of the niche was considered the probability of the density function, of both isotopes, at a confidence interval of 95%. The bi-plot accounts for species-specific distribution and bivariate projections of the niche regions (Swanson et al., 2015). The package Siber v.2.1.4, from R software, was used to obtain total convex hull areas (TA), which consist in a polygon that is drawn around the outermost points in the data, as well as trophic niche areas, corrected for small sample sizes (SEAc) (Jackson, 2023).The probability of trophic overlap, at a confidence interval of 95%, was estimated through NicheRover v.1.1.0 package, which uses Bayesian corrections (Swanson et al., 2015).
To assess the existence of significant differences (p-value ≤ 0.05) in the isotopic profiles (δ13C and δ15N) of fin samples from the four cultured seahorse species (H. reidi, H. kuda, H. guttulatus, and H. hippocampus), the groups originating from the same center (IIM or CCMAR), were compared through non-parametric analysis of similarities (ANOSIM). This analysis was performed on a resemblance matrix, which used normalized Euclidean distances. Additionally, isotopic fingerprints of wild and cultured H. guttulatus, cultured H. guttulatus specimens from CCMAR and IIM, as well as combined isotopic and elemental profiles of two cultured seahorse species (H. kuda and H. reidi), were analyzed using ANOSIM. The R statistic of this test can range from 0 to 1, which allows to measure differences between groups (Anderson et al., 2008).The ANOSIM tests were run with 9999 permutations. Due to the low number of replicates and after performing a Shapiro-Wilk test, for normality assessment, a non-parametric version of the t-test for unpaired samples (Mann -Whitney U) was performed in the raw data. This statistical analysis allowed to compare pairs of sample groups, in terms of stable isotopes of cultured seahorse species and cultured and wild H. guttulatus, as well as in terms of element composition of two cultured species. The Benjamini - Hochberg correction was applied whenever multiple comparisons were performed.
To evaluate the potential of using combined elemental and isotopic profiles for species discrimination, Principal Component Analysis (PCA) was performed on normalized data. Both stable isotopes (δ13C and δ15N), as well as elements that differed significantly (p-value ≤ 0.05, Mann-Whitney U) between two cultured seahorse species (H. kuda and H. reidi) were selected for PCA. The packages factoMineR (Husson et al., 2020), factoextra v1.0.7 (Kassambara, 2020) and corrplot v0.8.4 (Wei et al., 2017), from R software, were used for PCA.
All graphical representations were constructed with ggplot2 v3.3.0 (Wickham, 2016) package for R.
3 Results
3.1 Isotopic profile and trophic niche
The δ13C mean values of dorsal fin samples from four seahorse species ranged between -20.38 ± 0.71‰ (H. kuda from IIM) and -14.37 ± 0.34‰ (H. hippocampus from CCMAR) (Table 1). In the case of δ15N, the mean values ranged from 8.81 ± 0.31‰ (H. hippocampus from CCMAR) to 16.34 ± 0.82‰ (H. kuda from IIM) (Table 1).
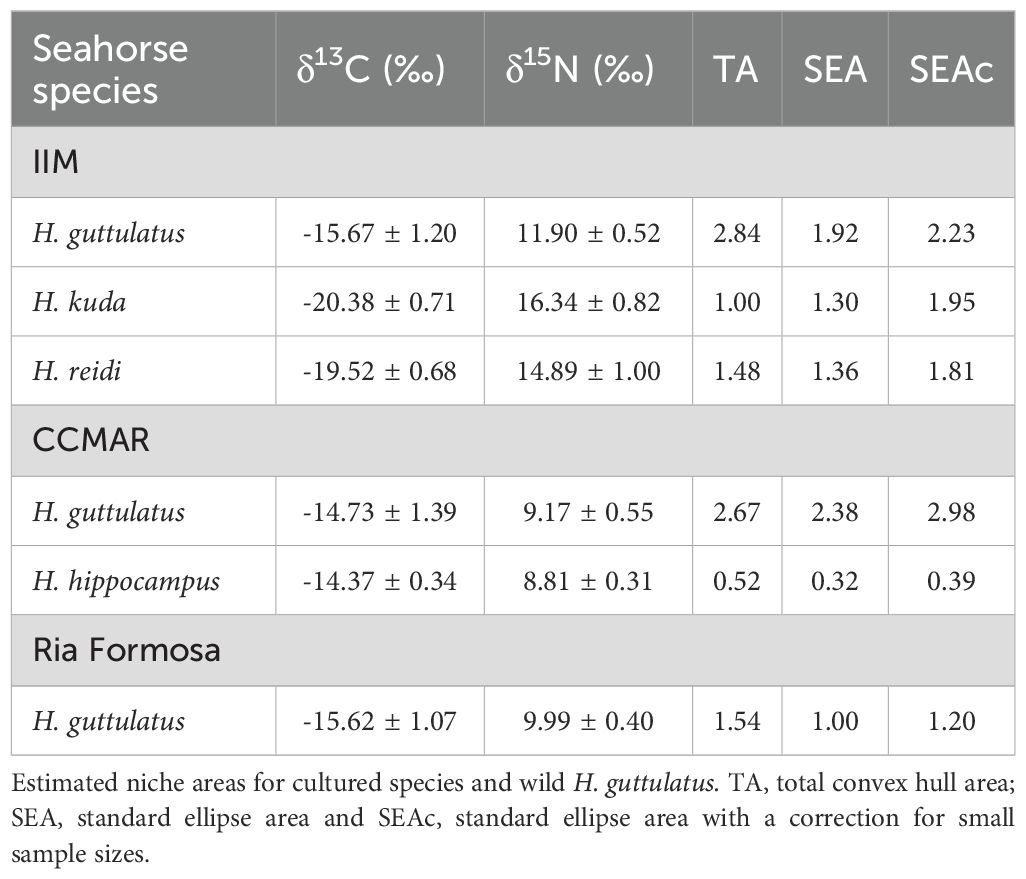
Table 1. Isotopic values (δ13C and δ15N, mean ± standard deviation) from dorsal fin samples of four seahorse species (Hippocampus guttulatus, H. kuda, H. reidi and H. hippocampus) cultured at Instituto de Investigaciones Marinas (IIM - CSIC, Vigo, Spain) and Centro de Ciências do Mar (CCMAR, Faro, Portugal), as well as wild H. guttulatus from Ria Formosa coastal lagoon (Portugal).
Hippocampus guttulatus from CCMAR presented the largest standard ellipse, corrected for small sample sizes, SEAc (2.98), while H. hippocampus from CCMAR occupied the smallest SEAc (0.39) (Table 1; Figure 2). The centroids of the ellipses corresponding to species cultured at the same research center were positioned closer to each other, except in the case of H. guttulatus from IIM (Figure 2).
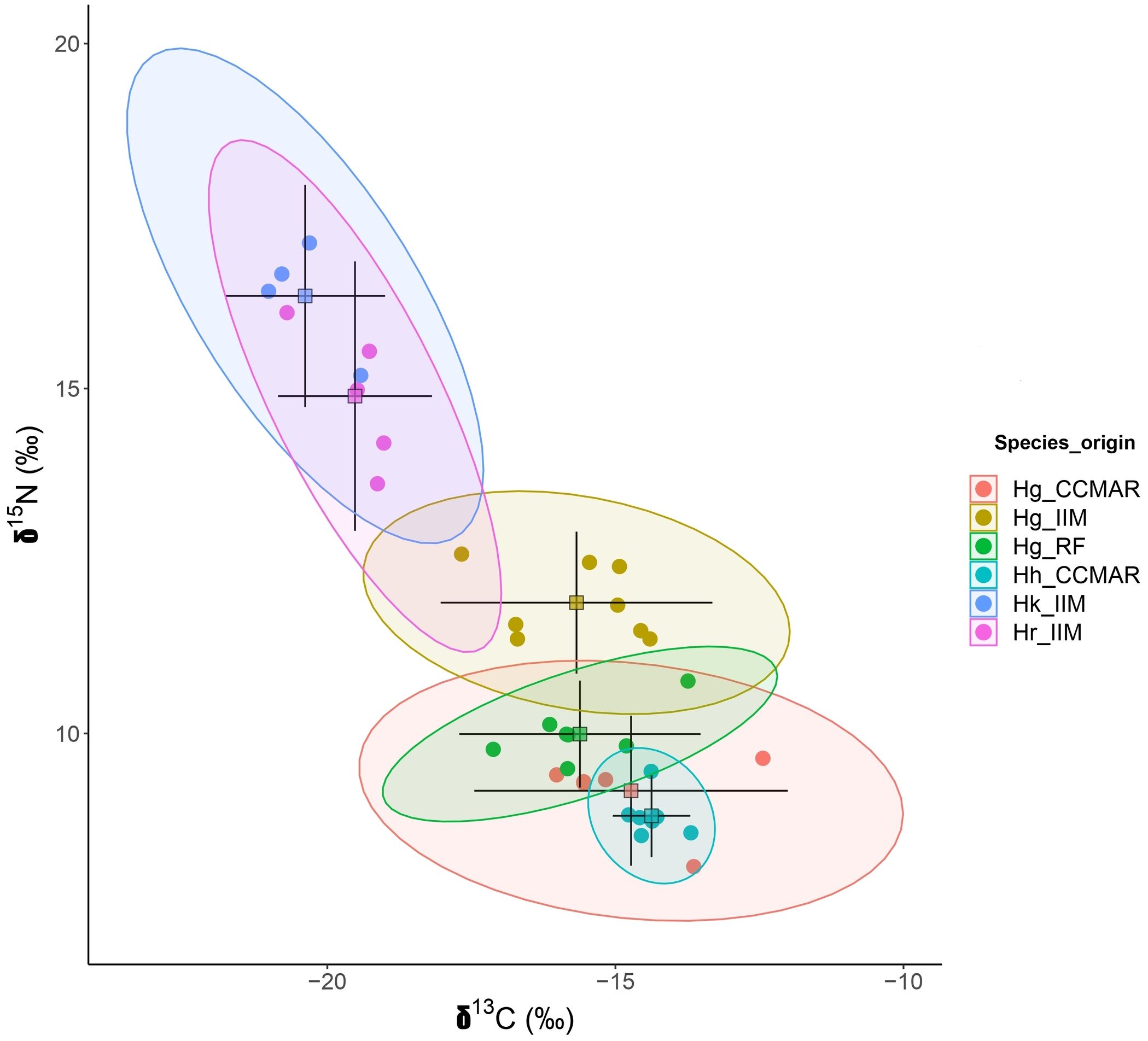
Figure 2. Isotopic bi-plot relative to average δ13C and δ15N signatures of dorsal fin samples from four Hippocampus species (H. guttulatus, H. kuda, H. reidi and H. hippocampus), cultured at Instituto de Investigaciones Marinas (IIM - CSIC, Vigo, Spain) and Centro de Ciências do Mar (CCMAR, Faro, Portugal), as well as wild H. guttulatus from Ria Formosa coastal lagoon (RF, Portugal). Standard ellipses with 95% credible intervals for the means. Hg, H. guttulatus; Hk, H. kuda; Hr, H. reidi and Hh, H. hippocampus.
Niche overlap estimates of seahorse species from IIM showed the highest value (55.65%) for the probability of H. kuda being found within the niche of H. reidi, while the lowest probability (0.12%) was registered for H. kuda being found within the niche of H. guttulatus (Table 2). In the case of seahorse species from CCMAR, H. hippocampus’ niche almost fully overlapped (95.49%) with the one of H. gutttulatus. When comparing H. guttulatus, cultured at different research centers (IIM and CCMAR), the highest probability value, even if low (2.05%), was registered for niche overlap of specimens from IIM and specimens from CCMAR. Probability of wild H. guttulatus niche overlaying the one from H. guttulatus, cultured at CCMAR, was higher than the other way around, with a value of 59.18% (Table 2).
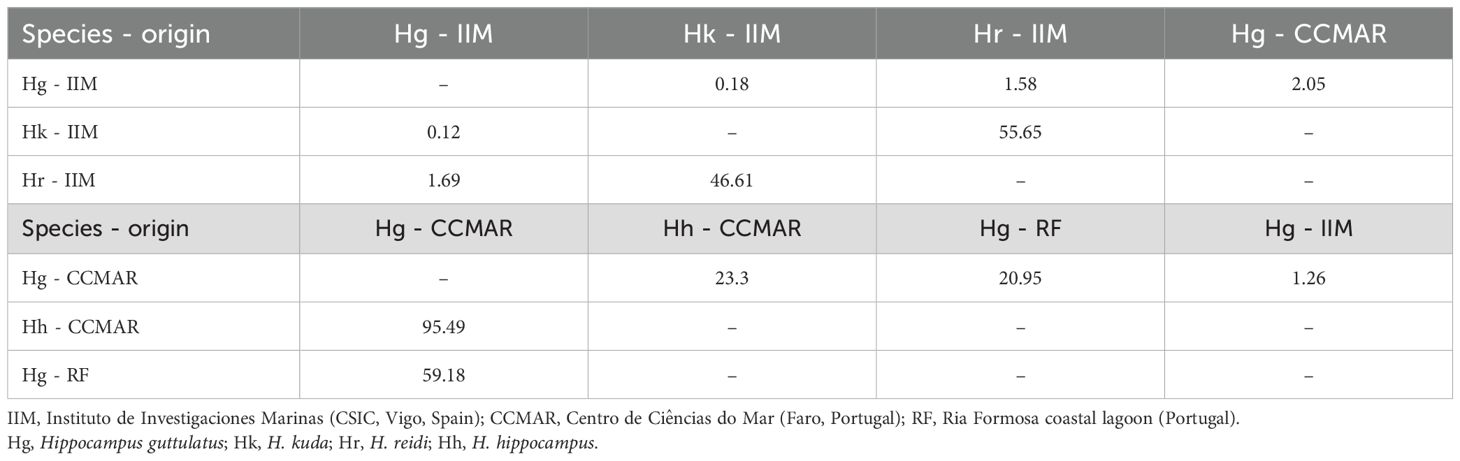
Table 2. Niche overlap estimates, expressing the probability (%) at a α = 0.95 of species in the rows being found within the niche of species in the columns.
3.2 Potential of isotopic profiles for species and geographic origin discrimination
The stable isotopic profiles (δ13C and δ15N) of seahorse’s (H. kuda, H. reidi and H. guttulatus) dorsal fins from IIM were significantly different (ANOSIM, p-value< 0.001). However, H. kuda and H. reidi did not differ significantly in terms of isotopic composition (ANOSIM, p-value = 0.095), while H. guttulatus and H. reidi, as well as H. guttulatus and H. kuda were significantly different (ANOSIM, p-value = 0.002 and 0.003, respectively) (Table 3). Moreover, H. guttulatus and H. reidi differed significantly in δ13C and δ15N compositions (Mann- Whitney U, p-value = 0.003 for both isotopes), as was the case of H. guttulatus and H. kuda (Mann- Whitney U, p-value = 0.007 for both isotopes) (Table 3). The two species cultured at CCMAR (H. hippocampus and H. guttulatus) were significantly different in terms of the isotopic profile (ANOSIM, p-value = 0.011), even though there were no significant differences for δ13C and δ15N (Mann- Whitney U, p-value = 0.317 and 0.306, respectively) (Table 3). Cultured H. guttulatus sourced from CCMAR and IIM differed significantly in the isotopic profile (ANOSIM, p-value< 0.001), with δ15N as the variable contributing for significant differences (Mann- Whitney U, p-value = 0.004). Wild H. guttulatus from Ria Formosa and cultured H. guttulatus from CCMAR, revealed significant differences in the isotopic profiles (ANOSIM, p-value = 0.006), with δ15N as the variable significantly different (Mann- Whitney U, p-value = 0.008) between the two groups (Table 3).
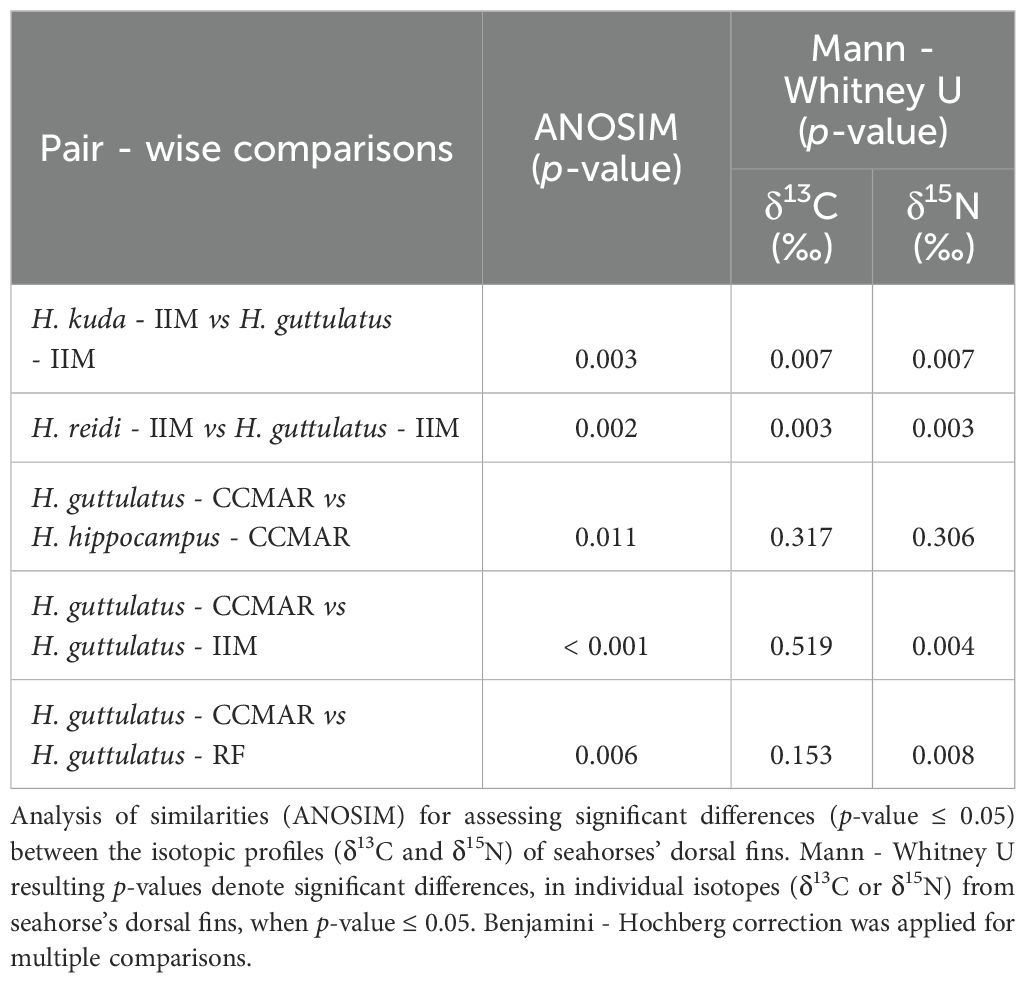
Table 3. Pair-wise comparisons between seahorse species cultured at different research centers, Instituto de Investigaciones Marinas (IIM - CSIC, Vigo, Spain) or Centro de Ciências do Mar (CCMAR, Faro, Portugal), between cultured Hippocampus guttulatus from different locations (IIM or CCMAR) and between wild H. guttulatus from Ria Formosa coastal lagoon (RF, Portugal) and cultured H. guttulatus from CCMAR.
3.3 Combined elemental and isotopic fingerprints for species discrimination
Hippocampus reidi and H. kuda were not significantly different concerning their stable isotope profile of dorsal fin samples (ANOSIM, p-value = 0.095). Thus, the potential of combining elemental fingerprints from the whole-body and isotopes from dorsal fins, was assessed. Phosphorous (P) was the element that revealed higher mean ratio values (mmol/mol Ca) in the whole-body of both species (771.782 ± 54.188 and 657.125 ± 17.534 mmol/mol Ca for H. kuda and H. reidi, respectively) (Table 4). Arsenic and V were the elements with lower mean Ca ratios in both H. kuda (0.002 ± 0.004 e-1 and 0.001 ± 0.009 e-2 mmol/mol Ca, respectively) and H. reidi (0.001 ± 0.004 e-1 and 0.001 ± 0.968 e-4 mmol/mol Ca, respectively) (Table 4). The combined isotopic and elemental profiles of H. kuda and H. reidi were significantly different (ANOSIM, p-value = 0.008). The elements that most contributed to the significant differences recorded between the two species were P, K, Na, Mg, Rb, Co, Sr and Ba (p-value = 0.035, Mann-Whitney U for all elements) (Table 4).
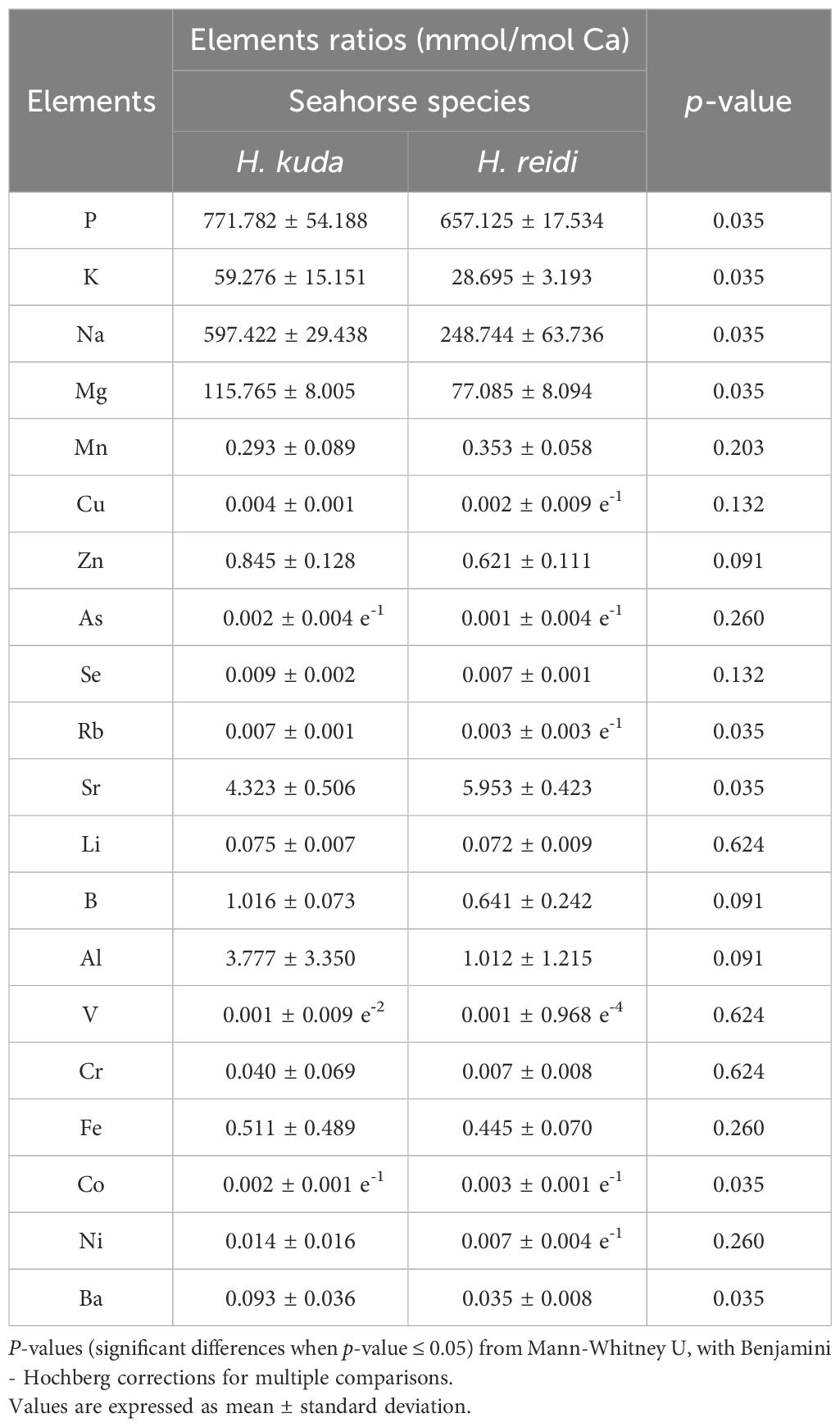
Table 4. Elemental ratios (mmol/mol Ca) from the whole-body of Hippocampus kuda (n = 4) and H. reidi (n = 5), cultured at Instituto de Investigaciones Marinas (IIM - CSIC, Vigo, Spain).
The elements that revealed significant differences in the whole-body of H. kuda and H. reidi, as well as the stable isotopes from dorsal fins, were included in the PCA. The first two components explained 90.2% of group variability (Figure 3). The centroids for species were distanced from each other and groups were well discriminated. The variables Mg and Na were strongly correlated since the corresponding lines were overlapped (Figure 3). These two variables also revealed a strong relationship with K, Rb, P and Ba appearing, however, less correlated to the remaining variables. The two elements Sr and Co were also positively correlated, while the stable isotopes revealed a negative correlation (Figure 3). Hippocampus kuda replicates presented higher values for K, Rb, Mg, P, Na and Ba than those of H. reidi, with the opposite being true for Co and Sr, which revealed higher values for H. reidi replicates (Figure 3).
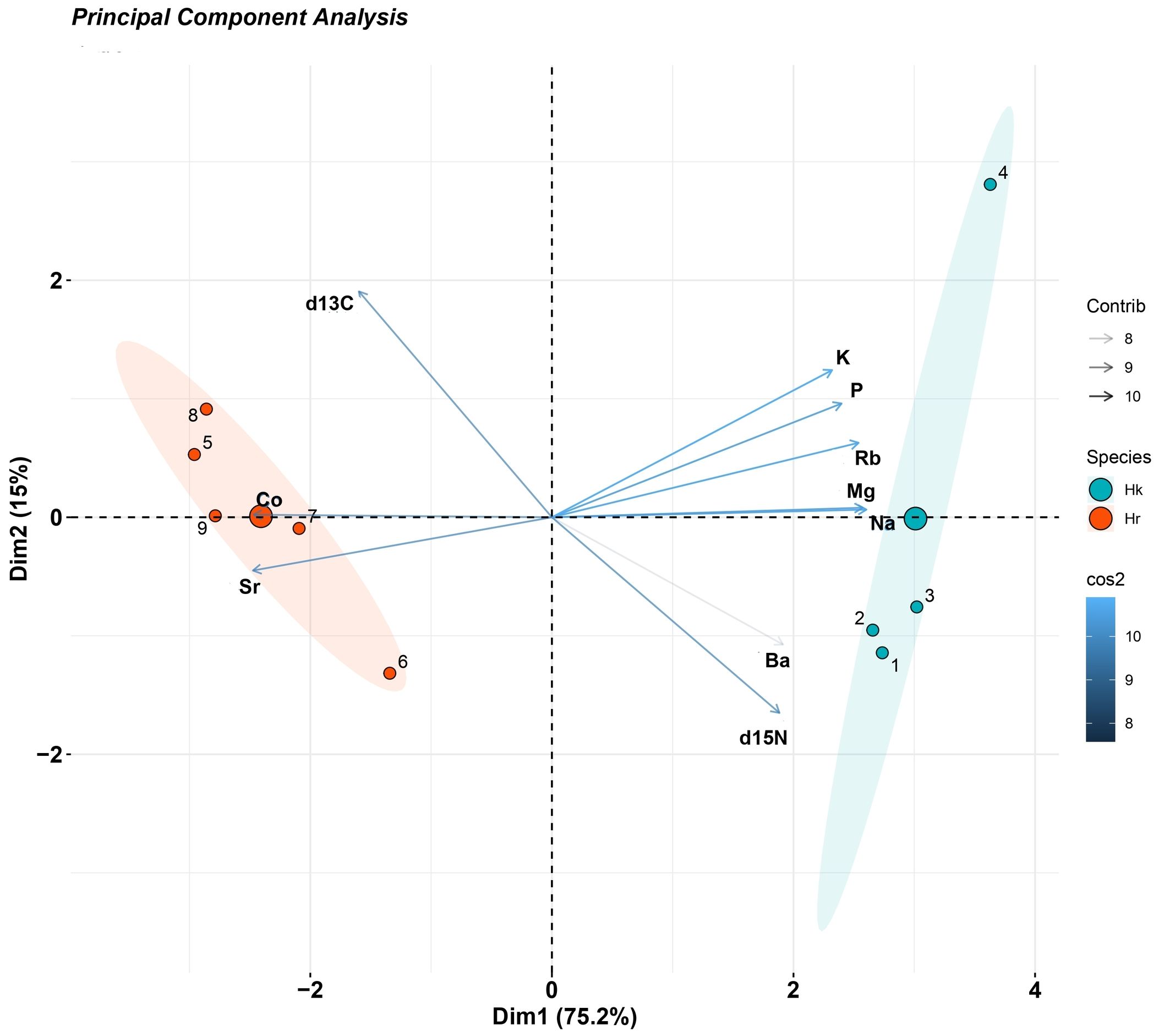
Figure 3. Principal Component Analysis (PCA) for Hippocampus kuda (Hk) (n = 4) and H. reidi (Hr) (n = 5) cultured at Instituto de Investigaciones Marinas (IIM - CSIC, Vigo, Spain). Variables: potassium (K), rubidium (Rb), magnesium (Mg), phosphorus (P), sodium (Na), strontium (Sr), cobalt (Co), barium (Ba), δ13C (d13C) and δ15N (d15N). Elemental profile was obtained from seahorses’ whole bodies, while stable isotopes were obtained from dorsal fins. Ellipses correspond to 95% confidence.
4 Discussion
4.1 Potential of isotopic profiles for species and geographic origin discrimination
Isotopic profiles in animals’ whole bodies reflect dietary intake and composition (DeNiro and Epstein, 1978, 1981; Hobson and Clark, 1992; Fry, 2006; Martínez del Rio et al., 2009). However, isotopic profiles (δ13C and δ15N) can vary depending on tissue type and diet (DeNiro and Epstein, 1978, 1981). Valladares and Planas (2012) reported similar δ13C and δ15N values in dorsal fins and muscle tissues of H. guttulatus, validating both matrices for SIA. This analysis has already been applied to fish migration studies, using matrices such as otoliths (Huijbers et al., 2013) and muscle tissues (Davidsen et al., 2017). However, to the authors best knowledge, there are no studies addressing the use of dorsal fins’ isotopic fingerprints for tracing Hippocampus spp. geographic origin or for species discrimination.
The dorsal fins of H. kuda and H. reidi from IIM exhibited similar mean δ13C and δ15N values, as was the case for H. guttulatus and H. hippocampus from CCMAR (Tables 1, 3), expressing the dietary sources of both sites. Nevertheless, H. guttulatus from IIM displayed significantly different δ13C and δ15N values to the other two cultured species, H. kuda and H. reidi (Tables 1, 3). The results of a study performed with freshwater fish species, revealed that phylogeny, as well as morphology, could be associated with δ13C and δ15N, even though the correlation between isotopic data and phylogeny was not considered very strong (Keppeler and Winemiller, 2020). Hippocamus kuda and H. reidi are phylogenetically closer than H. guttulatus (Teske et al., 2004), which may partially explain the similarities in isotopic fingerprints recorded in H. kuda and H. reidi. Additionally, it has been described that temperature may affect δ13C values in H. guttulatus juveniles (Valladares and Planas, 2020), which could also be related with the differences reported, as H. kuda and H. reidi are both tropical species and, thus, were cultured at higher water temperatures than temperate seahorse species H. guttulatus. The ellipses referring to δ13C and δ15N showed a trend for species from the same research center being placed closer to each other, except for H. guttulatus from IIM (Figure 2). Trophic overlap evidenced dietary similarities between H. reidi and H. kuda from IIM and between H. hippocampus and H. guttulatus from CCMAR (Table 2). Nevertheless, H. guttulatus from CCMAR displayed a low probability of niche overlap with H. hippocampus, potentially due to intra-specific variability, increasing niche area (Table 1). Factors, such as specimens’ size, may contribute to variability in δ13C and δ15N values (Davidsen et al., 2017). In seahorses, size can influence δ13C and δ15N values due to changes in prey preferences with growth (Valladares et al., 2016).
Hippocampus guttulatus cultured at IIM exhibited significantly higher δ15N values than those of conspecifics cultured at CCMAR (Tables 1, 3). These differences likely originated from dissimilarities in the diet provided at each site. A study on the pipefish Syngnathus acus revealed that juveniles, with a higher habitat dispersion than adults, exhibited isotopic heterogeneity, mainly at δ15N level, due to high dietary variability (Planas, 2022). Moreover, in the study developed by Planas et al. (2020b), dorsal fins from H. guttulatus fed on different diets (Artemia and/or a mixture of frozen and captured mysidaceans) displayed differences in the stable isotopic profiles. Nonetheless, it is noteworthy that the similarities in δ13C of H. guttulatus from IIM and CCMAR may be due to a similar assimilation of dietary items in both groups, while differences in δ15N likely reflect variations in trophic levels of consumed prey (Planas et al., 2020b).
Wild H. guttulatus from Ria Formosa coastal lagoon exhibited significantly higher mean δ15N values than those of cultured specimens from CCMAR (Tables 1, 3). This pattern aligns with findings for wild Sparus aurata, which also displayed higher δ15N values than cultured conspecifics (Guerra-García et al., 2023). These differences could be due to a higher trophic level occupied by fishes that feed in the marine environment (Arechavala-Lopez et al., 2013). Additionally, the relationship between δ15N values in estuarine fish and urbanization levels has been documented (Morris et al., 2015). In fact, between 2001 and 2002, when studied H. guttulatus were sourced from the wild, Ria Formosa coastal lagoon experienced changes in seagrass meadows due to abnormal wastewater discharges (Cabaço et al., 2008).
4.2 Combined elemental and isotopic fingerprints for species discrimination
Previous studies have resorted to the combination of different analyses to improve geographic origin discrimination (Ricardo et al., 2024). Dorsal fin isotopic profiles, more specifically δ15N, effectively differentiated cultured H. guttulatus from different origins, and distinguished between wild and cultured specimens. However, this approach proved insufficient for discriminating between phylogenetically close species (Table 3). As such, the potential of combining both isotopic profiles from dorsal fins, as well as elemental fingerprints from the whole body, was assessed. The elements that significantly differed between H. kuda and H. reidi from IIM were P, K, Na, Mg, Rb, Co, Sr and Ba (Table 4). Seahorses are known to be rich in Na, K, P (Lin et al., 2008) and Mg (Lall and Lewis-McCrea, 2007), a finding in line with the results recorded for cultured H. kuda and H. reidi (Table 4).
There are several factors that influence elemental composition of fish calcified structures, such as temperature, diet, water chemistry, physiology and genetics (Clarke et al., 2011; Kerr and Campana, 2014; Sturrock et al., 2015). In some cases, environmental factors are assumed to outweigh physiological aspects, when it comes to elemental composition, as is the case of Sr (Sturrock et al., 2014). This element has been pointed out as a marker of calcified structures, influenced by environmental conditions, such as water chemistry (Bath et al., 2000; Campana, 2005), even though physiological aspects can also exert a strong influence on its regulation (Sturrock et al., 2014).
Elements under rigorous physiological control, such as P, Na and K, could be interesting for species discrimination, since they are less easily shaped by environmental factors (Campana, 2005; Kerr and Campana, 2014). Magnesium present in calcified structures was previously used as a fish stock marker (Avigliano et al., 2019), even though the effects of environmental factors on Mg/Ca from calcified structures remains unclear (Nishimoto et al., 2010). It has also been described that Ba/Ca in estuarine fish otoliths may be negatively related with salinity, being the opposite true for temperature (Nelson et al., 2018). According to Barnes and Gillanders (2013), stock genetics also affected Ba/Ca incorporation in marine species Argyrosomus japonicus fingerlings. The differences recorded in Mg, Sr and Ba composition in H. reidi and H. kuda might be attributed to physiological regulation, as seawater parameters, such as salinity and temperature, were kept identical and stable for both species. In the case of Co, a study on the marine flatfish Scophthalmus maximus has shown that abiotic factors such as pH and temperature, do not have a significant effect on its assimilation (Pouil et al., 2018). Additionally, a study by Sturrock et al. (2014) found that the concentration of Rb in blood plasma of the marine flatfish Pleuronectes platessa was not largely influenced by physiological aspects. It has been suggested that this element’s uptake by fish is influenced not only by its environmental abundance, but also by concentrations of other metallic and alkali elements in the marine environment (Campbell et al., 2005).
It is important to highlight that the analysis of different biological matrices may result in different elemental profiles, due to aspects related with metabolic pathways (Sadeghi et al., 2020). Indeed, a study led by Uncumusaoğlu et al. (2012), revealed that cadmium (Cd), Cu, lead (Pb) and Zn compositions in the muscle and liver tissues of wild H. hippocampus differed significantly. The cultured adults of H. kuda and H. reidi surveyed in the present study were maintained under the same physicochemical conditions, with whole-body elemental analyses suggesting that inter-specific variability was the primary driver of elemental fingerprint differences. Thus, combining significantly different elements from the whole body and stable isotopes from dorsal fins could enhance seahorse species identification in apprehensions from illegal, unreported and unregulated (IUU) fisheries and trade. As seafood samples may undergo different processing methods, which sometimes may damage DNA quality (Marín et al., 2018), geochemical tools could offer an alternative for discriminating dry specimens from different seahorse species.
5 Conclusions
The results of this preliminary study suggest that stable isotopes, mainly δ15N, determined from cultured seahorse dorsal fins, can be successfully used to trace their geographic origin, as well as discriminate between wild and cultured conspecifics. Fin-clipping is already a well stablished, non-lethal practice, employed on live seahorses (Valladares and Planas, 2012). Thus, resulting stable isotopic profiles may be useful for reconstructing the life history of specimens destined to the marine aquarium trade and originating from the wild or from culture facilities. The analysis of stable isotopes from dorsal fins could therefore be paramount to certify cultured specimens and aquaculture facilities. It is, however, worth further investigating the potential effects of phylogeny on isotopic fingerprints, as the temperate species seahorse H. guttulatus significantly differed from tropical species cultured at IIM.
Stable isotopic analysis alone might not be sufficient to discriminate between cultured seahorse species, as H. kuda and H. reidi from IIM, as well as H. guttulatus and H. hippocampus from CCMAR, did not differ significantly in δ13C and δ15N compositions. However, combining isotopic and elemental fingerprints, from seahorse dorsal fins and whole-body, may prove useful for species discrimination, namely for apprehended dry specimens originating from IUU fisheries and trade. Further research on these topics is still required to evaluate the accuracy of the findings here reported, with an emphasis on specimens sourced from the wild.
Data availability statement
The original contributions presented in the study are included in the article/supplementary material. Further inquiries can be directed to the corresponding authors.
Ethics statement
The animal study was approved by the ethics committee from the Portuguese Veterinary Medicines Directorate for the Ministry of Agriculture, Rural Development and Fisheries. Under this approval, the captive breeding program for Hippocampus hippocampus and H. guttulatus (Project HIPPONUTRE, reference 16-02-01-FMP-54) was performed in compliance with the Guidelines of the European Union Council (86/609/EU) and Portuguese legislation for the use of laboratory animals. Animal capture, handling and sampling also followed all bioethical standards of the Spanish Government (Real Decreto 1201/2005, 10th October 2005) and the Regional Government Xunta de Galicia (REGA ES360570202001/15/FUN/BIOL.AN/MPO01). The study was conducted in accordance with the local legislation and institutional requirements.
Author contributions
AC: Conceptualization, Data curation, Formal analysis, Investigation, Software, Visualization, Writing – original draft, Writing – review & editing. RC: Conceptualization, Funding acquisition, Investigation, Project administration, Resources, Supervision, Writing – review & editing. JP: Resources, Writing – review & editing. FR: Resources, Writing – review & editing. EF: Resources, Writing – review & editing. CP: Conceptualization, Investigation, Methodology, Resources, Supervision, Validation, Writing – review & editing. MP: Conceptualization, Data curation, Formal analysis, Funding acquisition, Investigation, Methodology, Project administration, Resources, Software, Supervision, Validation, Writing – review & editing.
Funding
The author(s) declare financial support was received for the research, authorship, and/or publication of this article. This study was financially supported by project CITAQUA, “Desenvolvimento do Projeto de Reforço do Polo de Aveiro (H4)”, framed within Measure 10 of Investment TC-C10-i01 -Hub Azul -Rede de Infraestruturas para a Economia Azul, financed by the Recovery and Resilience Plan (RRP) and supported by Fundo Azul of the Portuguese Government. This work was also supported by national funds through Fundação para a Ciência e Tecnologia (FCT) under a PhD grant to AC (UI/BD/153063/2022). This study received Portuguese national funds from FCT through projects UIDB/04326/2020, UIDP/04326/2020 and LA/P/0101/2020 and was financed by the scientific project HIPPONUTRE -”Cultivo do cavalo marinho de focinho comprido, Hippocampus guttulatus: Optimização zootécnica e avaliação de requisitos nutricionais” (Ref. proj. 16-02-01-FMP-54), financed by the Programa Operacional MAR2020. Seahorses from IIM-CSIC were cultured under project Hippoeco through Spanish national funds (Ref CGL2015-68110-R, Ministerio de Ciencia, Innovación y Universidades) and co-funding by FEDER. Funding for isotopic analysis was provided by project Hippo-DEC: Diagnóstico del estado de conservación de caballitos de mar en el litoral español, áreas críticas y medidas de conservación”, financed by Ministerio para la Transición Ecológica y el Reto Demográfico. We acknowledge FCT/MEC for the financial support to CESAM (UIDP/50017/2020+UIDB/50017/2020) and to GEOBIOTEC (UIDP/04035/2020) through national funds and co-funding by FEDER, within the PT2020 Partnership Agreement and Compete 2020. We also acknowledge the project “Impacto e Consolidação em I&DT da Unidade de Investigação Química Orgânica, Produtos Naturais e Agroalimentares em áreas Agroalimentares e afins ICT_2009_02_005_2034” for financing the ICP-MS.
Acknowledgments
We acknowledge Miguel Cabral for statistical advisement and Pedro Pato Martins for technical support.
Conflict of interest
The authors declare that the research was conducted in the absence of any commercial or financial relationships that could be construed as a potential conflict of interest.
The author(s) declared that they were an editorial board member of Frontiers, at the time of submission. This had no impact on the peer review process and the final decision.
Publisher’s note
All claims expressed in this article are solely those of the authors and do not necessarily represent those of their affiliated organizations, or those of the publisher, the editors and the reviewers. Any product that may be evaluated in this article, or claim that may be made by its manufacturer, is not guaranteed or endorsed by the publisher.
References
Anderson M. J., Gorley R. N., Clarke K. R. (2008). PERMANOVA+ for PRIMER: Guide to Software and Statistical Methods. Available online at: http://www.primer-e.com. (Accessed July 18, 2024).
Arechavala-Lopez P., Fernandez-Jover D., Black K. D., Ladoukakis E., Bayle-Sempere J. T., Sanchez-Jerez P., et al. (2013). Differentiating the wild or farmed origin of Mediterranean fish: A review of tools for sea bream and sea bass. Rev. Aquac 5, 137–157. doi: 10.1111/raq.12006
Avigliano E., Carvalho B. M., Miller N., Gironde S. C., Tombari A., Limburg K., et al. (2019). Fin spine chemistry as a non-lethal alternative to otoliths for stock discrimination in an endangered catfish. Mar. Ecol. Prog. Ser. 614, 147–157. doi: 10.3354/meps12895
Barnes T. C., Gillanders B. M. (2013). Combined effects of extrinsic and intrinsic factors on otolith chemistry: implications for environmental reconstructions. Can. J. Fish. Aquat. Sci. 70, 1159–1166. doi: 10.1139/cjfas-2012-0442
Bath G. E., Thorrold S. R., Jones C. M., Campana S. E., Mclaren J. W., Lam J. W. H. (2000). Strontium and barium uptake in aragonitic otoliths of marine fish. Geochim. Cosmochim. Acta 64, 1705–1714. doi: 10.1016/S0016-7037(99)00419-6
Boehm J. T., Bovee. E., Harris S. E., Eddins K., Akahoho I., Foster M., et al. (2023). The United States dried seahorse trade: A comparison of traditional Chinese medicine and ecommerce-curio markets using molecular identification. PloS One 18, e0291874. doi: 10.1371/journal.pone.0291874
Cabaço S., Machás R., Vieira V., Santos R. (2008). Impacts of urban wastewater discharge on seagrass meadows (Zostera noltii). Estuar. Coast. Shelf Sci. 78, 1–13. doi: 10.1016/j.ecss.2007.11.005
Cabral A. E., Ricardo F., Patinha C., da Silva E. F., Correia M., Palma J., et al. (2021). Successful use of geochemical tools to trace the geographic origin of long-snouted seahorse Hippocampus guttulatus raised in captivity. Animals 11. doi: 10.3390/ani11061534
Campana S. E. (2005). “Otolith Elemental Composition as a Natural Marker of Fish Stocks,” in Stock Identification Methods: Applications in Fishery Science. Eds. Cadrin S. X., Friedland K. D., Waldman J. R. (Amsterdam: Academic Press), 227–245. doi: 10.1016/B978-0-12-154351-8.X5000-X
Campbell L. M., Fisk A. T., Wang X., Köck G., Muir D. C. (2005). Evidence for biomagnification of rubidium in freshwater and marine food webs. Can. J. Fish. Aquat. Sci. 62, 1161–1167. doi: 10.1139/f05-027
Clarke L. M., Conover D. O., Thorrold S. R. (2011). Population differences in otolith chemistry have a genetic basis in Menidia menidia. Can. J. Fish. Aquat. Sci. 68, 105–114. doi: 10.1139/F10-147
Cohen F. P. A., Pimentel T., Valenti W. C., Calado R. (2018). First insights on the bacterial fingerprints of live seahorse skin mucus and its relevance for traceability. Aquaculture 492, 259–264. doi: 10.1016/j.aquaculture.2018.04.020
Cohen F. P. A., Valenti W. C., Calado R. (2013). Traceability issues in the trade of marine ornamental species. Rev. Fish. Sci. 21, 98–111. doi: 10.1080/10641262.2012.760522
Cohen F. P. A., Valenti W. C., Planas M., Calado R. (2017). Seahorse aquaculture, biology and conservation: knowledge gaps and research opportunities. Rev. Fish. Sci. Aquac 25, 100–111. doi: 10.1080/23308249.2016.1237469
Correia M., Caldwell I. R., Koldewey H. J., Andrade J. P., Palma J. (2015). Seahorse (Hippocampinae) population fluctuations in the Ria Formosa Lagoon, south Portugal. J. Fish Biol. 87, 679–690. doi: 10.1111/jfb.12748
Curtis J. M. R. (2006). A case of mistaken identity: skin filaments are unreliable for identifying Hippocampus guttulatus and Hippocampus hippocampus. J. Fish Biol. 69, 1855–1859. doi: 10.1111/j.1095-8649.2006.01228.x
Curtis J. M. R., Santos S. V., Nadeau J. L., Gunn B., Bigney Wilner K., Balasubramanian H., et al. (2017). Life history and ecology of the elusive European short-snouted seahorse Hippocampus hippocampus. J. Fish Biol. 91, 1603–1622. doi: 10.1111/jfb.13473
Davidsen J. G., Knudsen R., Power M., Sjursen A. D., Rønning L., Hårsaker K., et al. (2017). Trophic niche similarity among sea trout Salmo trutta in central Norway investigated using different time-integrated trophic tracers. Aquat. Biol. 26, 217–227. doi: 10.3354/ab00689
DeNiro M. J., Epstein S. (1978). Influence of diet on the distribution of carbon isotopes in animals. Geochim. Cosmochim. Acta 42, 495–506. doi: 10.1016/0016-7037(78)90199-0
DeNiro M. J., Epstein S. (1981). Influence of diet on the distribution of nitrogen isotopes in animals. Geochim. Cosmochim. Acta 45, 341–351. doi: 10.1016/0016-7037(81)90244-1
Duarte B., Carreiras J., Mamede R., Duarte I. A., Caçador I., Reis-Santos P., et al. (2022). Written in ink: Elemental signatures in octopus ink successfully trace geographical origin. J. Food Compos. Anal. 109, 104479. doi: 10.1016/j.jfca.2022.104479
Foster S. J., Vincent A. C. J. (2004). Life history and ecology of seahorses: implications for conservation and management. J. Fish Biol. 65, 1–61. doi: 10.1111/j.1095-8649.2004.00429.x
Fredriksen S. (2003). Food web studies in a Norwegian kelp forest based on stable isotope (d13C and d15N) analysis. Mar. Ecol. Prog. Ser. 260, 71–81. doi: 10.3354/MEPS260071
Fry B. (1988). Food web structure on Georges Bank from stable C, N and S isotopic compositions. Limnol. Oceanogr. 33, 1182–1190. doi: 10.4319/LO.1988.33.5.1182
Fry B. (2006). “Scanning the future,” in Stable isotope ecology, vol. 277–284. (Springer, New York, NY).
Guerra-García J. M., Calero-Cano S., Donázar-Aramendía I., Giráldez I., Morales E., Arechavala-Lopez P., et al. (2023). Farming Sparus aurata (Teleostei: Sparidae) in marsh ponds: trophic characterization and trace metal accumulation. Mar. Environ. Res. 188, 106007. doi: 10.1016/j.marenvres.2023.106007
Hobson K. A., Clark R. G. (1992). Assessing avian diets using stable isotopes I: Turnover of 13C in tissues. Condor 94, 181–188. doi: 10.2307/1368807
Huijbers C. M., Nagelkerken I., Debrot A. O., Jongejans E. (2013). Geographic coupling of juvenile and adult habitat shapes spatial population dynamics of a coral reef fish. Ecology 94, 1859–1870. doi: 10.1890/11-1759.1
Husson F., Josse J., Le S., Mazet J. (2020). FactoMineR: multivariate exploratory data analysis and data mining. R Package version 1.
IAPSO Working Group on Symbols, Units and Nomenclature in Physical Oceanography (1985). “The International System of Units (SI) in Oceanography,” in Unesco Technical Papers in Marine Science 45. (Paris (France): United Nations Educational, Scientific, and Cultural Organization) doi: 10.25607/OBP-666IUCN
Jackson A. L. (2023). Comparing populations. Available online at: https://cran.r-project.org/web/packages/SIBER/vignettes/siber-comparing-populations.html (Accessed February 20, 2024).
Kassambara A. (2020). Factoextra: Extract and Visualize the Results of Multivariate Data Analyses. Available online at: https://CRAN.R-project.org/package=factoextra (Accessed February 20, 2024).
Keppeler F. W., Winemiller K. O. (2020). Can ancestry and morphology be used as surrogates for species niche relationships? Ecol. Evol. 10, 6562–6578. doi: 10.1002/ece3.6390
Kerr L. A., Campana S. E. (2014). “Chemical Composition of Fish Hard Parts as a Natural Marker of Fish Stocks,” in In Stock Identification Methods: Applications in Fishery Science, 2nd Edition. Eds. Cadrin S. X., Kerr L. A., Mariani S. (Amsterdam: Academic Press), 205–234. doi: 10.1016/B978-0-12-397003-9.00011-4
Kuo T. C., Laksanawimol P., Aylesworth L., Foster S. J., Vincent A. C. J. (2018). Changes in the trade of bycatch species corresponding to CITES regulations: the case of dried seahorse trade in Thailand. Biodivers. Conserv. 27, 3447–3468. doi: 10.1007/s10531-018-1610-2
Kuo T. C., Vincent A. (2018). Assessing the changes in international trade of marine fishes under CITES regulations – A case study of seahorses. Mar. Policy 88, 48–57. doi: 10.1016/j.marpol.2017.10.031
Lall S. P., Lewis-McCrea L. M. (2007). Role of nutrients in skeletal metabolism and pathology in fish - An overview. Aquaculture 267, 3–19. doi: 10.1016/j.aquaculture.2007.02.053
Leal M. C., Pimentel T., Ricardo F., Rosa R., Calado R. (2015). Seafood traceability: current needs, available tools, and biotechnological challenges for origin certification. Trends Biotechnol. 33, 331–336. doi: 10.1016/j.tibtech.2015.03.003
Lin Q., Lin J., Lu J., Li B. (2008). Biochemical composition of six seahorse species, Hippocampus sp., from the Chinese coast. J. World Aquac Soc. 39, 225–234. doi: 10.1111/j.1749-7345.2008.00159.x
Luo W., Qu H., Li J., Wang X., Lin Q. (2015). A novel method for the identification of seahorses (genus Hippocampus) using cross-species amplifiable microsatellites. Fish. Res. 172, 318–324. doi: 10.1016/j.fishres.2015.07.017
Marín A., Serna J., Robles C., Ramírez B., Reyes-Flores L. E., Zelada-Mázmela E., et al. (2018). A glimpse into the genetic diversity of the Peruvian seafood sector: Unveiling species substitution, mislabeling and trade of threatened species. PloS One 13, e0206596. doi: 10.1371/journal.pone.0206596
Martínez del Rio C., Wolf N., Carleton S. A., Gannes L. Z. (2009). Isotopic ecology ten years after a call for more laboratory experiments. Biol. Rev. Camb. Philos. 84, 91–111. doi: 10.1111/j.1469-185X.2008.00064.x
Morris C., Lee S. Y., Van De Merwe J. (2015). [amp]]delta;15N of estuarine fishes as a quantitative indicator of urbanization. Ecol. Indic 56, 41–49. doi: 10.1016/j.ecolind.2015.03.028
Nelson T. R., DeVries D. R., Wright R. A. (2018). Salinity and temperature effects on element incorporation of Gulf Killifish Fundulus grandis otoliths. Estuaries Coast. 41, 1164–1177. doi: 10.1007/s12237-017-0341-z
Nishimoto M. M., Washburn L., Warner R. R., Love M. S., Paradis G. L. (2010). Otolith elemental signatures reflect residency in coastal water masses. Environ. Biol. Fishes 89, 341–356. doi: 10.1007/s10641-010-9698-6
Piñeiro-Corbeira C., Iglesias L., Nogueira R., Campos S., Jiménez A., Regueira M., et al. (2021). Structure and trophic niches in mobile epifauna assemblages associated with seaweeds and habitats of syngnathid fishes in cíes archipelago (Atlantic islands marine national park, North West Iberia). Front. Mar. Sci. 8. doi: 10.3389/fmars.2021.773367
Planas M. (2021). Carry-over effects of pre-breeding diets on seahorse (Hippocampus reidi) reproductive success. Aquaculture 533, 736148. doi: 10.1016/j.aquaculture.2020.736148
Planas M. (2022). Ecological traits and trophic plasticity in the greater pipefish syngnathus acus in the NW Iberian peninsula. Biology 11, 712. doi: 10.3390/biology11050712
Planas M., Chamorro A., Paltrinieri A., Campos S., Jiménez A., Nedelec K., et al. (2020b). Effect of diet on breeders and inheritance in syngnathids: application of isotopic experimentally derived data to field studies. Mar. Ecol. Prog. Ser. 650, 107–123. doi: 10.3354/meps13315
Planas M., Paltrinieri A., Carneiro M. D. D., Hernández-Urcera J. (2020a). Effects of tissue preservation on carbon and nitrogen stable isotope signatures in syngnathid fishes and prey. Animals 10, 1–16. doi: 10.3390/ani10122301
Post D. M. (2002). Using stable isotopes to estimate trophic position: models, methods, and assumptions. Ecology 83, 703–718. doi: 10.1890/0012-9658(2002)083[0703:USITET]2.0.CO;2
Pouil S., Oberhänsli F., Bustamante P., Metian M. (2018). Investigations of temperature and pH variations on metal trophic transfer in turbot (Scophthalmus maximus). Environ. Sci. pollut. Res. 25, 11219–11225. doi: 10.1007/s11356-017-8691-4
R Core Team (2019). A Language and Environment for Statistical Computing. Available online at: https://cran.r-project.org/faqs.html (Accessed February 20, 2024).
Ricardo F., Lopes M. L., Mamede R., Domingues M. R., Ferreira da Silva E., Patinha C., et al. (2024). Combined use of fatty acid profiles and elemental fingerprints to trace the geographic origin of live baits for sports fishing: the solitary tube worm (Diopatra neapolitana, annelida, onuphidae) as a case study. Animals 14, 1361. doi: 10.3390/ani14091361
Ricardo F., Mamede R., Bispo R., Santos A., Ferreira da Silva E., Patinha C., et al. (2020). Cost-efficiency improvement of bivalves shells preparation when tracing their geographic origin through ICP-MS analysis of elemental fingerprints. Food Control 118, 107383. doi: 10.1016/j.foodcont.2020.107383
Ricardo F., Pimentel T., Génio L., Calado R. (2017). Spatio-Temporal variability of trace elements fingerprints in cockle (Cerastoderma edule) shells and its relevance for tracing geographic origin. Sci. Rep. 7, 3475. doi: 10.1038/s41598-017-03381-w
Sadeghi P., Loghmani M., Frokhzad S. (2020). Human health risk assessment of heavy metals via consumption of commercial marine fish (Thunnus albacares, Euthynnus affinis, and Katsuwonus pelamis) in Oman Sea. Environ. Sci. pollut. Res. 27, 14944–14952. doi: 10.1007/s11356-020-07907-0
Sofoulaki K., Kalantzi I., Machias A., Mastoraki M., Chatzifotis S., Mylona K., et al. (2018). Metals and elements in sardine and anchovy: species specific differences and correlations with proximate composition and size. Sci. Total Environ. 645, 329–338. doi: 10.1016/j.scitotenv.2018.07.133
Sturrock A. M., Hunter E., Milton J. A., Johnson R. C., Waring C. P., Trueman C. N., et al. (2015). Quantifying physiological influences on otolith microchemistry. Methods Ecol. Evol. 6, 806–816. doi: 10.1111/2041-210X.12381
Sturrock A. M., Trueman C. N., Milton J. A., Waring C. P., Cooper M. J., Hunter E. (2014). Physiological influences can outweigh environmental signals in otolith microchemistry research. Mar. Ecol. Prog. Ser. 500, 245–264. doi: 10.3354/meps10699
Swanson H. K., Lysy M., Power M., Stasko A. D., Johnson J. D., Reist J. D. (2015). A new probabilistic method for quantifying n-dimensional ecological niches and niche overlap. Ecology 96, 318–324. doi: 10.1890/14-0235.1
Teske P. R., Cherry M. I., Matthee C. A. (2004). The evolutionary history of seahorses (Syngnathidae: Hippocampus): Molecular data suggest a West Pacific origin and two invasions of the Atlantic Ocean. Mol. Phylogenet. Evol. 30, 273–286. doi: 10.1016/S1055-7903(03)00214-8
The IUCN Red List of Threatened Species (2023). Available online at: https://www.iucnredlist.org (Accessed April 26, 2024). Version 2023-1.2023.
Uncumusaoğlu A. A., Gürkan Ş., Özkan E. Y., Büyükışık H. B. (2012). A preliminary research on heavy metals accumulated in liver and muscle tissue of Seahorse (Hippocampus hippocampus) catched from Tirebolu coasts (Giresun, Eastern Black Sea). Fresenius Environ. Bull. 21, 3418–3420.
Vaidyanathan T., Vincent A. C. J. (2021). State of seahorse fisheries in India, nearly two decades after they were banned. Biodivers Conserv. 30, 2223–2253. doi: 10.1007/s10531-021-02188-6
Valladares S., Planas M. (2012). Non-lethal dorsal fin sampling for stable isotope analysis in seahorses. Aquat. Ecol. 46, 363–370. doi: 10.1007/s10452-012-9407-y
Valladares S., Planas M. (2020). Application of effective day degrees in the assessment of stable isotope patterns in developing seahorses under different temperatures. Animals 10, 1–14. doi: 10.3390/ani10091571
Valladares S., Soto D. X., Planas M. (2016). Dietary composition of endangered seahorses determined by stable isotope analysis. Mar. Freshw. Res. 68, 831–839. doi: 10.1086/303250
Vincent A. C. J., Foster S. J., Koldewey H. J. (2011). Conservation and management of seahorses and other Syngnathidae. J. Fish Biol. 78, 1681–1724. doi: 10.1111/j.1095-8649.2011.03003.x
Vivas M., Peñalver J., Oliver J. A., López Giraldo J. D., Mena C. (2023). Population dynamics of the long-snouted seahorse (Hippocampus guttulatus Cuvier 1829) in the Mar Menor coastal lagoon. J. Fish Biol. 104, 163–170. doi: 10.1111/jfb.15564
Watchorn D. J., Cowan M. A., Driscoll D. A., Nimmo D. G., Ashman K. R., Garkaklis M. J., et al. (2022). Artificial habitat structures for animal conservation: design and implementation, risks and opportunities. Front. Ecol. Environ. 20, 301–309. doi: 10.1002/fee.2470
Wei T., Simko V., Levy M., Xie Y., Jin Y., Zemla J. (2017). corrplot: Visualization of a Correlation Matrix. Available online at: https://cran.r-project.org/package=corrplot (Accessed February 20, 2024).
Wickham H. (2016). Ggplot2: elegant graphics for data analysis. 2nd ed (New York, USA: Springer International Publishing). doi: 10.1007/978-3-319-24277-4
Woodall L. C., Jones R., Zimmerman B., Guillaume S., Stubbington T., Shaw P., et al. (2012). Partial fin-clipping as an effective tool for tissue sampling seahorses, Hippocampus spp. J. Mar. Biolog. Assoc. U.K. 92, 1427–1432. doi: 10.1017/S0025315411001810
Keywords: syngnathids, Hippocampus spp., trophic niche, stable isotopes, fin clipping, ICP-MS, geochemical tools
Citation: Cabral AE, Calado R, Palma J, Ricardo F, Ferreira da Silva E, Patinha C and Planas M (2024) Use of isotopic and elemental fingerprints for seahorse species discrimination and traceability of geographic origin. Front. Mar. Sci. 11:1494320. doi: 10.3389/fmars.2024.1494320
Received: 11 September 2024; Accepted: 04 November 2024;
Published: 20 November 2024.
Edited by:
Ana M. Faria, University of Porto, PortugalReviewed by:
Dilian Noemi Anguas Cabrera, The South Border College (ECOSUR), MexicoSule Gurkan, Ege University, Türkiye
Copyright © 2024 Cabral, Calado, Palma, Ricardo, Ferreira da Silva, Patinha and Planas. This is an open-access article distributed under the terms of the Creative Commons Attribution License (CC BY). The use, distribution or reproduction in other forums is permitted, provided the original author(s) and the copyright owner(s) are credited and that the original publication in this journal is cited, in accordance with accepted academic practice. No use, distribution or reproduction is permitted which does not comply with these terms.
*Correspondence: Miquel Planas, bXBsYW5hc0BpaW0uY3NpYy5lcw==; Ricardo Calado, cmpjYWxhZG9AdWEucHQ=