- 1Oceans Centre, National Institute of Water and Atmospheric Research (NIWA), Wellington, New Zealand
- 2School of Biological Sciences, Victoria University of Wellington, Wellington, New Zealand
- 3College of Earth, Ocean, and Atmospheric Sciences, Oregon State University, Corvallis, OR, United States
- 4Department of Ecology, Evolution, and Marine Biology and the Marine Science Institute, University of California, Santa Barbara, Santa Barbara, CA, United States
- 5Department of Marine Sciences, University of Otago, Dunedin, New Zealand
- 6Scripps Institution of Oceanography, La Jolla, CA, United States
Cold seeps are areas characterised by specialized biological communities that rely on chemosynthesis for their nutrition. To date, research conducted on New Zealand’s Hikurangi Margin seep communities has focused on communities at 650-1200 m water depth. Here, we characterize the macrofaunal nematode communities of New Zealand cold seeps for the first time, and at deeper (> 1200 m) seep locations (Maungaroa, Glendhu and Urutī South). There were no significant difference in nematode abundance, species richness, diversity and evenness among the seep areas, which may reflect the lack of difference in most sediment variables. However, a consistent spatial pattern in nematode abundance was observed within all the seep areas on the Hikurangi Margin: abundance was highest at or near the seep centre, decreased steeply away from the centre and was low in the periphery. These spatially consistent patterns reflect the influence of methane seepage, which appears limited to the inner 150-200 m radius of each area, on nematode abundance via input of chemosynthetic food sources. We found significant differences in nematode community structure among all three areas, with most of the heterogeneity in community structure between the shallow Urutī South area and deeper Maungaroa and Glendhu areas, and differences among nematode communities of high, medium and low abundance associated with site-specific gradients in methane seepage. Within area variability in nematode community structure was mainly correlated with food availability and sediment grain size. Consistent with previous investigations of seep nematodes, we did not find evidence of seep endemics. Although deposit feeders were generally the most abundant feeding group, there were differences in the relative abundances of different feeding groups such as microvores and epigrowth feeders among the seep areas, and as a function of distance from the centre of the seep areas. Impact on seep communities from gas hydrate extraction processes may occur via reduction or potentially cessation of free-gas methane supply to the seafloor, ‘sand’ production at the seafloor due to the physical degradation of the substrate structure, or alteration of the structural integrity of the seafloor substrate. Any spatial management options considered for managing these impacts should reflect the differences in benthic community structure between depths and locations on the Hikurangi Margin.
Introduction
Cold seeps are areas of where methane-rich fluids emerge from the seafloor, leading to the establishment of specialized biological communities that rely on chemosynthesis for their nutrition (Paull et al., 1984; Paull et al., 1985). To date, most of the research on cold seep faunal communities has focused on mega-epifaunal taxa (Levin, 2005; Cordes et al., 2009). This megafauna is typically dominated by vestimentiferan tube worms, bathymodiolin mussels and vesicomyd clams hosting sulphide-oxidising and/or methanotrophic symbionts (Childress et al., 1986; Fiala-Medioni et al., 1993), with biomass far exceeding that of surrounding non-seep sediments. Macro-infaunal abundance in seep sediments also tends to be greater than in non-seep sediments, but in some cases the pattern is absent or even the reverse (Levin, 2005). Macrofauna are more likely to exhibit greater abundance in seep than non-seep sediments at greater water depths, presumably because food becomes more limiting in deeper environments (Levin and Michener, 2002). Seep macrofauna is typically dominated by polychaetes (e.g., dorvilleids, hesionids and ampharetids) and bivalves (e.g., vesicomyid, solemyid, nuculanid clams). Some of these deep-sea seep-associated macro-infaunal taxa are endemic to seeps, in particular the chemoautotrophic taxa, but most also occur in non-seep sediments (Sahling et al., 2002; Sahling et al., 2003; Bernardino et al., 2012). Geochemical characteristics of the sediments such as seepage rate and sulphide concentration are key determinant of macro-infaunal community structure at seeps (Sahling et al., 2003; Levin et al., 2003; Demopoulos et al., 2018), as well as the presence of megafauna (Menot et al., 2010; Portail et al., 2015). Macro-infaunal communities associated with mats of sulphur bacteria are usually characterised by low diversity and are often dominated by a few highly abundant species (Dando et al., 1994; Levin, 2005; Menot et al., 2010).
Nematodes are among the most abundant metazoan taxon in deep-sea sediments (Zeppilli et al., 2018). They are particularly abundant in meiofaunal samples processed using a mesh size of 20-63 μm, often comprising >90% of metazoan abundance (Heip et al., 1985). Nematodes are also common, and sometimes dominant, in deep-sea macrofaunal samples (usually processed using a 250-500 μm mesh) (Hessler and Jumars, 1974; Hecker and Paul, 1979; Pavithran et al., 2009; Sharma et al., 2011), but they are seldom included in studies of macro-infauna. Studies have shown macrofaunal nematodes communities to be distinct from meiofaunal nematode communities, as well as exhibiting different functional response to environmental factors compared to their meiofaunal counterparts (Sharma et al., 2011; Baldrighi and Manini, 2015; Charrier et al., 2023). Macrofaunal nematode communities, on the other hand, appear to exhibit broadly similar trends to macrofaunal polychaete communities (Gunton et al., 2016).
In their review of nematodes associated with deep-sea chemosynthetic environments, Vanreusel et al. (2010a) found that nematodes are sometimes substantially more abundant in cold seep sediments than at nearby background sites, and that nematode communities at cold seeps are typically characterized by low diversity and are often dominated by a single species. Whilst studies of meiofaunal communities associated with shallow seeps have found high abundance of nematode species with endo- or ectosymbiotic bacteria (Dando et al., 1991; Jensen et al., 1992), symbiont-bearing nematode taxa have not been documented from deep-sea seeps. Unlike what has been observed for seep macro and megafaunal communities, there does not appear to be any nematode taxon endemic to seeps in the deep sea, and the available data show high variability in community structure among geographical locations (Vanreusel et al., 2010a). Investigations conducted since the review by Vanreusel et al. (2010a) largely confirm these observations (e.g., Hauquier et al., 2011; Pape et al., 2011; Portnova et al., 2011; Guilini et al., 2012).
Sites of active seepage have been identified on the Hikurangi Margin of New Zealand at depths of 600 to 2000 m (Greinert et al., 2010; Watson et al., 2020). Similar to seeps in other parts of the world, the mega-epifaunal communities at these Hikurangi Margin sites are dominated by vesicomyid clams, siboglinid worms and bathymodiolin mussels (Baco et al., 2010; Jones et al., 2010; Klaucke et al., 2010). The infaunal communities, however, are characterised by unusually high abundance and biomass of tube-building ampharetid polychaetes; the ampharetids are heterotrophic and derive most of their carbon from aerobic methanotrophy, probably by direct consumption of methane-oxidising bacteria (Thurber et al., 2010; Thurber et al., 2013). It is likely that ampharetids play a part in the early stages of seep community development on Hikurangi Margin by increasing the availability of sulphide and methane at the seabed (Bowden et al., 2013). Macro-infaunal seep communities on Hikurangi Margin were found to be distinct from slope, canyon and seamount communities in the region, largely due to a greater abundance of virtually all taxa, in particular spionid, ampharetid, glycerid and dorvilleid polychaetes as well as amphipods and cumaceans (Leduc et al., 2016). Rosli et al. (2016) showed that meiofauna abundance at the Opouawe Bank seep on Hikurangi Margin was similar to that of meiofauna from slope and seamount habitats at similar depths (ca. 1000 m), and lower than meiofauna from canyon habitats. Meiofaunal community structure differ between seep and all other deep-sea habitats, mainly due to a greater abundance of nauplii and amphipods in the seep samples relative to slope, canyon and seamount samples (Rosli et al., 2016). No data have yet been obtained, however, on nematode communities at seep sites on the Hikurangi Margin (Rosli et al., 2018).
Although seep sites have been identified at depths from 600 to 2000 m on the Hikurangi Margin, the bulk of the research conducted on the seep communities in the region to date has focused on seeps shallower than 1200 m water depth (Thurber et al., 2010; Thurber et al., 2013; Bowden et al., 2013; Leduc et al., 2016; Rosli et al., 2016; Rosli et al., 2018). These communities are exposed to varying degrees of trawling disturbance from fisheries, with some sites exposed to 100 or more trawls in a fifteen-year period, with signs of disturbance including areas of broken fragments of corals, abandoned gear and scour marks (Bowden et al., 2013). While no clear correlation between trawl intensity and benthic community structure has been quantified, tubeworm populations were found to be sparse, highly localised or absent at sites where trawling was most intense (Bowden et al., 2013). The Hikurangi Margin is an area where gas hydrates occur, and these deposits are a potentially extractable energy resource for New Zealand (Pecher and Henrys, 2003; Pecher et al., 2022). Shallow and deep seep communities may be vulnerable to the disturbance from large-scale extraction of seabed gas hydrates through direct physical impacts, smothering by resuspended sediments, modification of fluid flows, and large-scale destabilisation of slope sediments (Oluwunmi et al., 2023).
In July 2019, a research voyage to New Zealand’s Hikurangi Margin took place as part of the research programme “Gas hydrates: Economic opportunities and environmental implications” (HYDEE). The goal of this voyage was to obtain biological and biogeochemical data to investigate the potential impact of disturbances from any future gas-hydrate extractions to the marine ecosystems. This voyage provided an opportunity to characterize the nematode species communities of New Zealand cold seeps, which had not been investigated previously, and to study deeper (> 1200 m) seep locations unimpacted by current anthropogenic disturbance (i.e., trawling). Here, we describe the macrofaunal nematode communities at three seeps on Hikurangi Margin (Maungaroa, Glendhu and Urutī South) and investigate relationships with environmental variables. The results of this study are discussed in relation to the potential environments effects of gas hydrate extraction for the nematode communities. Results of analyses conducted on the broader macro-infaunal communities, as well as meiofaunal and mega-epifaunal communities, as part of the HYDEE research programme will be presented elsewhere.
Methods
Sampling
Samples were obtained from the southern end of the Hikurangi subduction margin, off the east coast of New Zealand’s North Island, in a region where the Pacific Plate subducts obliquely beneath the Australian Plate, and where potentially economically viable gas hydrate deposits are known to occur. Based on information from the geological/geophysical-focused research voyage HYDEE I (TAN1808) (Crutchley et al., 2018), three cold seeps were targeted during the biological/biogeochemical-focused research voyage HYDEE II (TAN1904) in July 2019: Maungaroa and Glendhu at ca. 2000 m depth, and Urutī South at ca. 1250 m depth (Law et al., 2024; Figure 1). Each seep area was approximately 1 km in diameter, at the centre of which was an active cold seep of approximately 200-300 m in diameter as determined by the extent of the seafloor backscatter signal for the carbonate rock that forms at the main seepage location. At each area, several transects were conducted using a deep-towed imaging system (DTIS) equipped with still and video camera (Bowden et al., 2013) and a methane sensor (Franatech, Oslo, Norway; Law et al., 2024). These transects were used to characterise the mega-epifauna and the substrate across gradients of seepage, obtain in situ measurements of methane concentrations, and help determine suitable coring location (Law et al., 2024, Bowden et al. in prep.). Methane readings closest to each core site were used for the analyses.
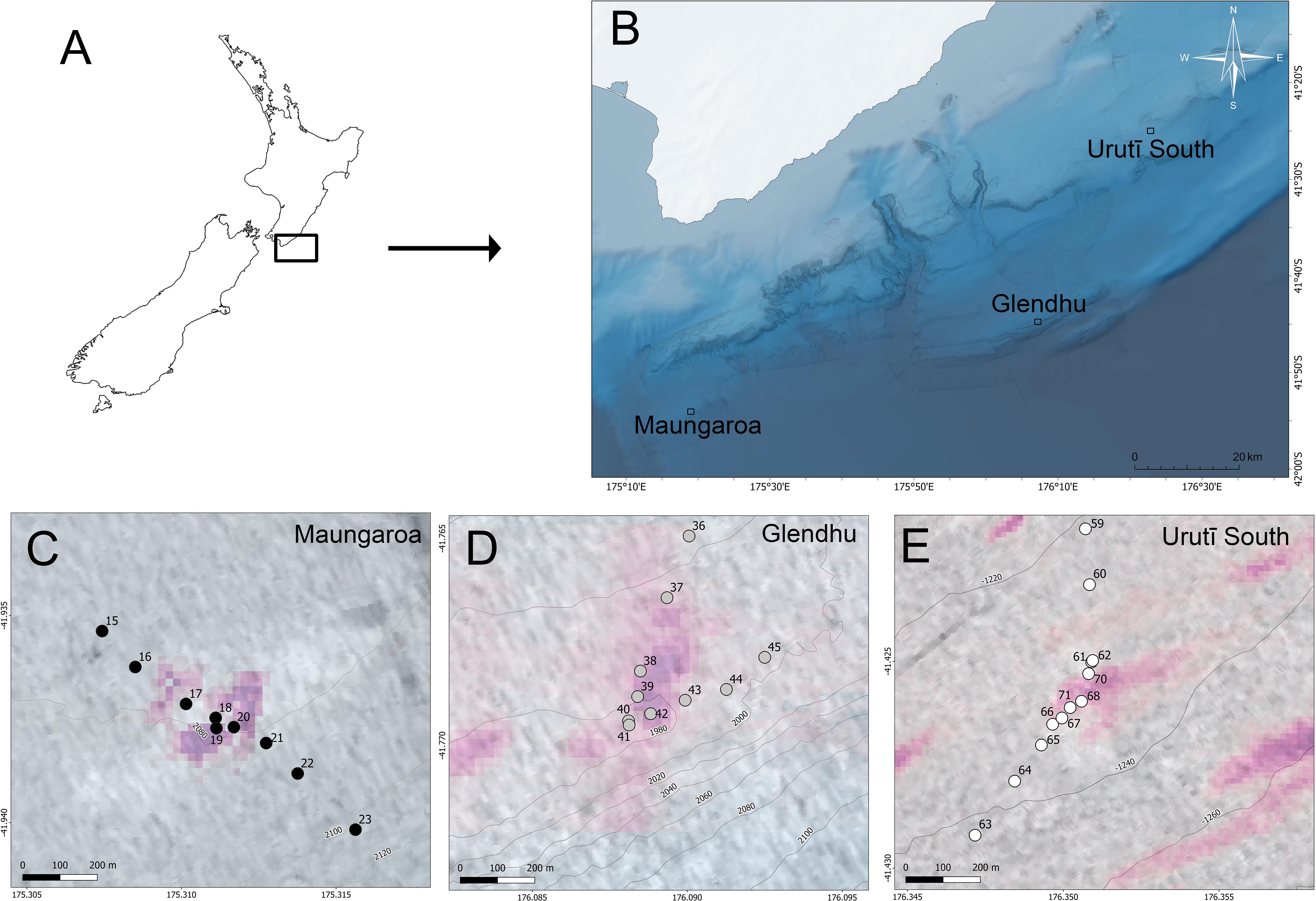
Figure 1. Map of (A) New Zealand and (B) Hikurangi Margin showing location of the three seep areas and details of the core sampling stations at the (C) Maungaroa, (D) Glendhu and (E) Urutī South seep areas. Pink shading in (C-E) is a representation of flare extent and intensity measured prior to core sampling using multibeam echosounder data (see Law et al., 2024 for details).
At each seep area, a video-guided multicorer was deployed once at each of 9-12 locations along a transect spanning the outer periphery of the area, the edge of the seep, and the centre of the seep (Table 1). The Ocean Instruments MC-800A multicorer was equipped with cores of 9.5 cm internal diameter. At most locations, two cores from the same deployment (station) were used for analyses of macro-infauna, with the exception of stations 42 and 71 where only one core was obtained. One core for analyses of sediment physicochemical characteristics was obtained from nine locations at each seep area (Table 1). The top 5 cm of sediment was sliced for analyses of macrofauna. The sediment was sieved using a 300 μm mesh, then fixed in 10% formalin and stained with Rose Bengal. Cores for analyses of sediment characteristics were sliced at one centimetre intervals.
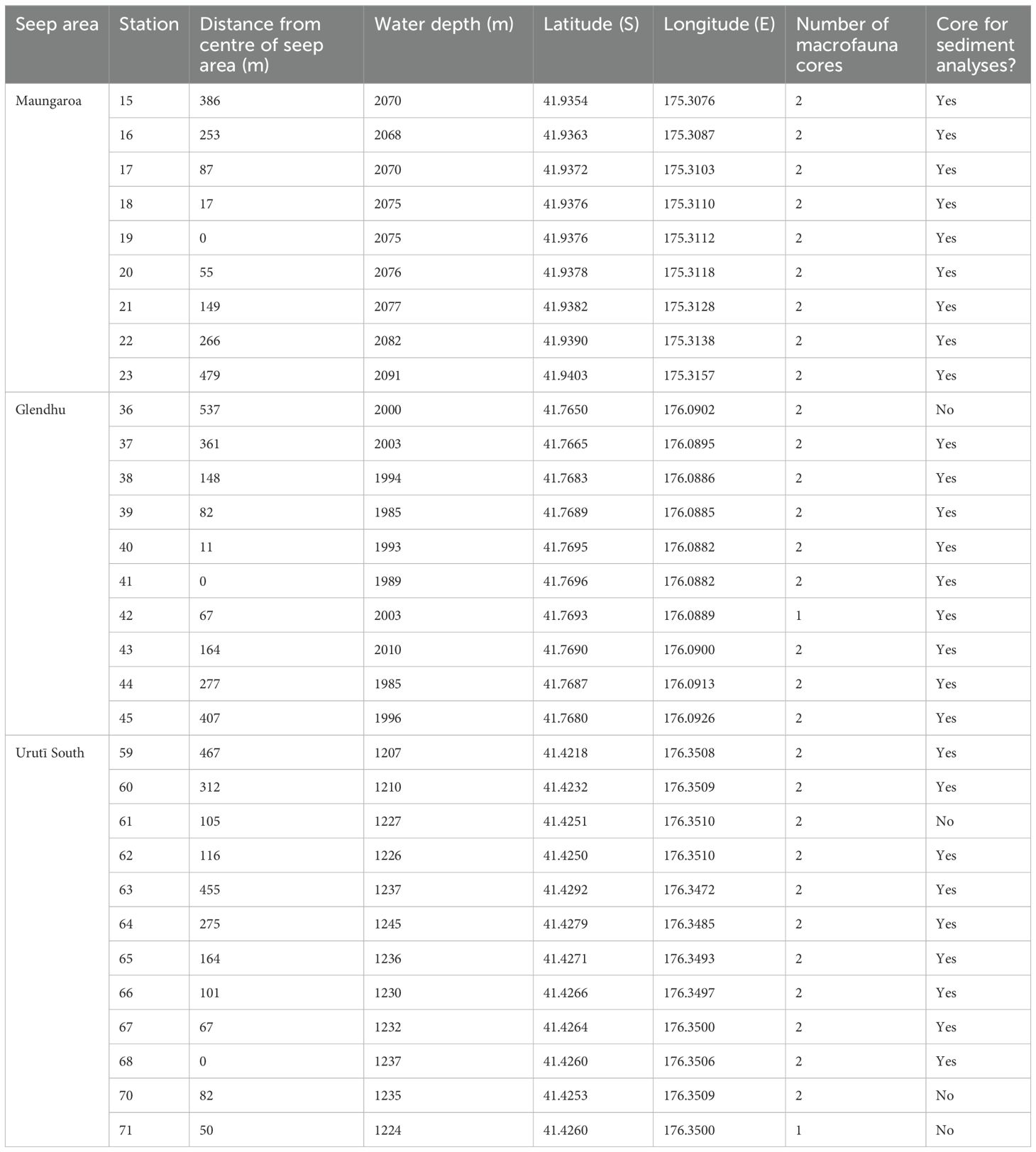
Table 1. Details of the Hikurangi Margin study areas sampled in July 2019 during NIWA voyage TAN1904.
Sediment and nematode analyses
The following sediment variables were determined: mean grain size (μm), proportion of silt/clay particles (%silt/clay), particle skewness, kurtosis and sorting. Biogeochemical parameters were also determined, including porewater methane and sulphide concentrations, total organic matter (%TOM) as a measure of food availability, chlorophyll a (Chl a (μg/g of dry weight sediment)) as a measure of ‘fresh’ phytodetrital organic matter, phaeopigments (Phaeo (μg/g of dry weight sediment)) as a measure of degraded phytodetrital organic matter, and their ratio (Chl a:Phaeo) as a measure of the ‘freshness’ of the phytodetrital organic matter, with higher values being ‘fresher’. Physical variables were determined by laser diffraction, while %TOM were determined by loss-on-ignition (4 h at 500°C) (Eleftheriou and Moore, 2005). Calcium carbonate percentages were determined using a gasometric technique. Chlorophyll a and phaeopigments were determined by standard spectrophotometric techniques subsequent to freeze-drying and extraction in 90% acetone (Sartory, 1982). Methane was measured from sediment via the headspace equilibration method (Magen et al., 2014). In brief, 5 mL of sediments were subsampled with a syringe into a glass vial with 2.5% NaOH and vials were capped and sealed. Headspace methane concentration was measured onshore using gas chromatography and a flame ionisation detector. Sulphide was measured from sediment porewater, sampled with Rhizons from sediment cores following retrieval, preserved with zinc acetate and measured via the methylene blue assay (Cline, 1969). Sediment grain size variables were measured based on the 0-1 cm, 1-2 or 2-3 cm, and 4-5 cm layers, and data shown here are shown as average of these three layers. Pigment and %TOM data were derived from the top five cm layers, and carbonate data were obtained from the 0-1 and 4-5 cm layers (presented here as average of both).
Nematodes were counted under a stereomicroscope, transferred to pure glycerol and mounted on slides (Somerfield and Warwick, 1996). All nematodes were identified to putative species (whenever possible) using the descriptions in Warwick et al. (1998) and Schmidt-Rhaesa, 2014), as well as the primary taxonomic literature. Nematodes were classified into feeding types following Moens and Vincx (1997). This scheme divides nematodes into six feeding types, i.e., microvores, ciliate feeders, deposit feeders, epigrowth feeders, facultative predators, and predators.
Statistical analyses
Analyses of univariate and multivariate data were conducted using the statistical routines in the multivariate statistical analysis software PRIMER v7 (Clarke and Gorley, 2015). PERMANOVA was used for analyses of nematode univariate and multivariate data as it does not rely on normally distributed data or balanced designs (Anderson et al., 2008). The following univariate parameters were quantified for each coring station: abundance (individuals 0.1 m-2), species richness (core-1), species diversity (Shannon diversity, H’10) and species evenness (J’). Univariate parameters were derived by averaging values of the two cores that were processed at each station, except for stations 42 and 71 where only one core was processed. Multivariate data were derived in the same way. Similarity matrices for univariate variables were built using Euclidean distance of untransformed data, and similarity matrices for multivariate data (nematode community structure) were built using the Bray-Curtis similarity measure of square root-transformed abundance data (Anderson et al., 2008; Anderson et al., 2011). The PERMANOVA was conducted using the single fixed factor “area” and distance from centre of seep area as covariate. P-values were obtained by 999 permutations. Pairwise PERMANOVA comparisons were conducted to identify which pair(s) of areas were responsible for the significant factor effect and the PERMDISP routine was used to test for differences in multivariate dispersion between the seep areas (Anderson et al., 2008). The SIMPER routine in PRIMER was used to identify the species contributing most to within-areasimilarity (75% similarity cut-off) and to among-area dissimilarity (40% dissimilarity cut-off) (Clarke and Warwick, 2001). Patterns in nematode community structure were visualised using a multidimensional (MDS) scaling ordination plot (Clarke and Warwick, 2001). In order to assess the level of heterogeneity in the structure of nematode communities among and within areas, the CLUSTER and SIMPROF routines in PRIMER were used to identify natural community groupings, with P set at 0.01 (Clarke et al., 2008). The SIMPER routine was then used to identify species responsible for within-group similarity (75% similarity cut-off).
Environmental variables (including sediment characteristics and methane concentrations) were compared among areas using PERMANOVA. The similarity matrix was built using Euclidean distance of normalised multivariate environmental data. As per the nematode analyses, the PERMANOVA was conducted using the single fixed factor “area”, P-values were obtained by 999 permutations, and SIMPER was used to identify the variables contributing most to among area dissimilarity.
Relationships between predictor variables and univariate and multivariate nematode community parameters were investigated using distance-based linear models (DistLMs) (Anderson et al., 2008). Because preliminary PERMANOVA with pairwise comparisons showed significant differences in nematode community structure among all three seep areas, separate DistLM analyses were conducted for each area. Predictor variables were checked for multicollinearity, which led to the removal of particle kurtosis, particle sorting, sand content and mud content from the list of predictor variables as they were highly correlated with other variables (r ≥ 0.9; Anderson et al., 2008). The remaining predictor variables were: mean particle size, particle skewness, %TOM, chl a, phaeo, chl a:phaeo, carbonate content, porewater sulphide concentration, porewater methane concentration and water column methane concentration. Water column methane concentration data from the nearest reading to the multicorer station were used (based on DTIS-mounted methane sensor), at a distance of 4-72 m away from the core location. As for the PERMANOVA, similarity matrices for univariate variables were built using Euclidean distance of untransformed data, and similarity matrices for multivariate data (nematode community structure) were built using the Bray-Curtis similarity measure of square root-transformed abundance data.
Results
Environment and sediment characteristics
There was a significant difference in environmental variables among the areas (PERMANOVA, P = 0.001). Pairwise comparisons were significant between all three areas (P = 0.05). Most (>50%) of the dissimilarity in environmental characteristics among the areas was due to sediment organic matter concentrations, water column and porewater methane concentrations, carbonates and pigment concentrations as well as sediment mean grain size (SIMPER, Supplement A). Methane concentrations in the water column decreased from the centre of each seep area towards the periphery (Figure 2). Methane concentrations in the water column were markedly greater at the Maungaroa (70-950 nmol L-1) and Urutī South areas (39-688 nmol L-1) than at the Glendhu area (4-208 nmol L-1) (Supplement B). Porewater methane concentrations were markedly lower than water column methane concentrations but showed the same general pattern of decreasing concentrations away from the centre of each seep area; the highest porewater methane concentrations were observed at Urutī South. Porewater sulphide concentrations decreased away from the centre of the Maungaroa seep area but peaked closer to the periphery at Glendhu and Urutī South. Sediment grain size characteristics were relatively similar across the three seep areas, consisting mostly of fine, medium and coarse silt particles (%silt = 87-97%, mean grain size = 12-27 μm) with relatively small proportions of sand particles (1-12%) (Supplement B). There was no obvious trend in grain size characteristics with distance from the seep centre at the Maungaroa area, however, mean grain size and particle sorting tended to be greater at or near the centre of the Glendhu and Urutī South areas (Figure 2); overall, mean grain size was slightly higher at the Glendhu area than at the other two areas. Sediment organic matter content (%TOM) was high and similar across all three areas (ca. 8-10%) but tended to be slightly higher at the Glendhu area and did not show any consistent trend with distance from the seep centres. Variability in carbonate content in the sediments was limited but tended to be lowest at the Maungaroa area, and to be slightly higher at or near the seep centres (Figure 2). Sediment chlorophyll a and phaeopigment concentrations were both markedly more elevated at the Maungaroa area than at the other two areas, but there was no trend in sediment pigment concentrations with distance from seep centres at any of the areas. The ratio of chlorophyll a to phaeopigment concentrations showed limited variability among the areas and no clear spatial trend was observed within areas (Figure 2).
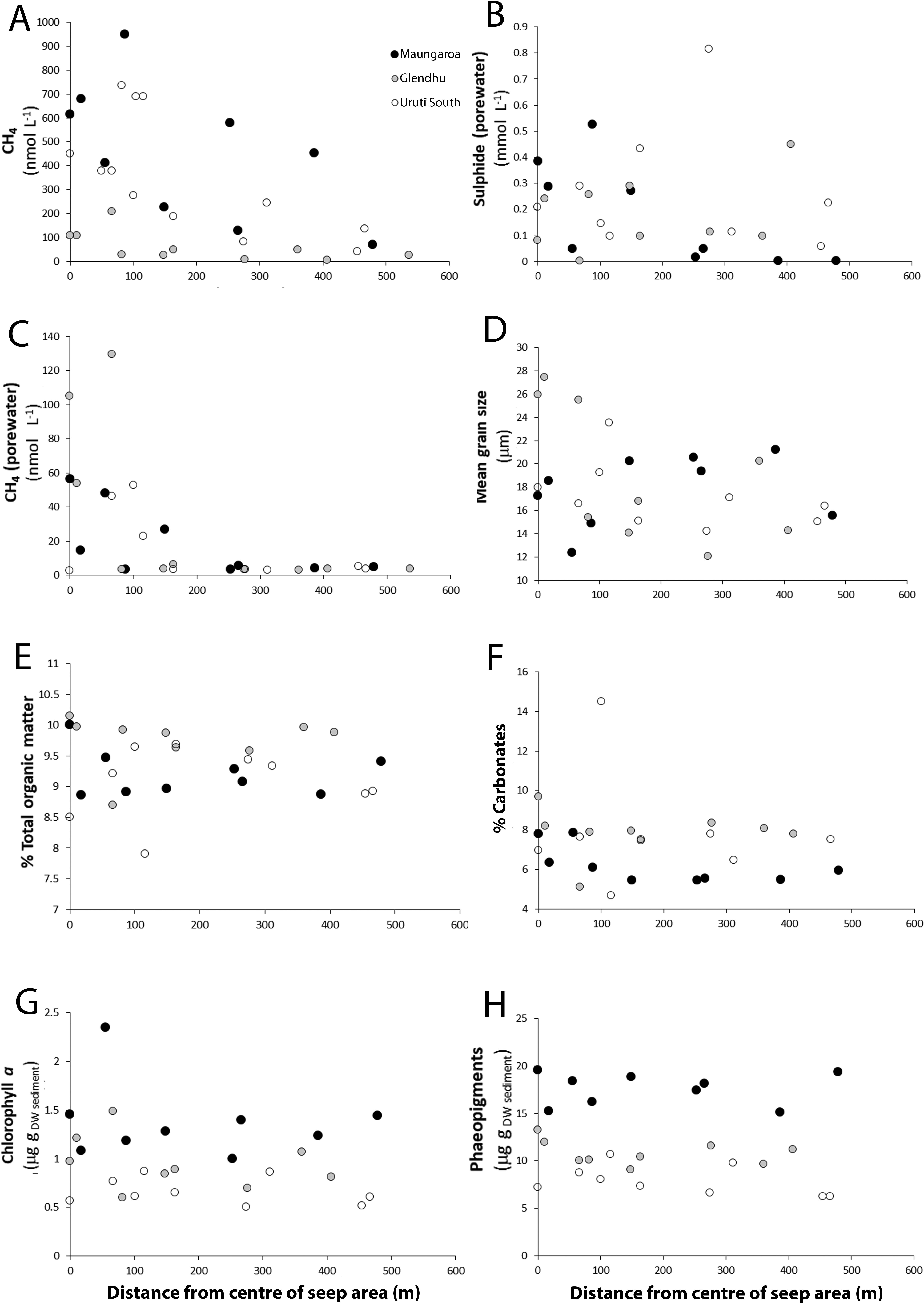
Figure 2. Relationship between distance from the centre of the seep areas and (A) dissolved methane concentration (seawater), (B) porewater sulphide concentration, (C) dissolved methane concentration (porewater), (D) sediment mean grain size, (E) organic matter content, (F) carbonate content, (G) chlorophyll a concentration, and (H) phaeopigment concentrations. Black filled circles = Maungaroa seep area; grey filled circles = Glendhu seep area; empty circles = Urutī South seep area.
Nematode communities
Values of univariate nematode community parameters were similar at all three study areas, and no significant difference in nematode abundance, species richness, diversity and evenness was observed among areas (PERMANOVA, P > 0.05; Supplement C). High nematode abundance values (> 1000 ind. 0.1 m2) were observed at (Maungaroa, Glendhu) or near (Urutī South) the centre of each of the seep area, with a steep decline away from the centre and uniformly low abundances in the seep periphery (>150-200 m away from seep centre; 190-681 ind. 0.1 m-2; Figure 3). Despite the similar trends at the three areas, nematode abundance in the seep periphery was noticeably greater at the Maungaroa area (421-681 ind. 0.1 m2) than at the Glendhu and Urutī South areas (190-379 ind. 0.1 m2), and the peak in nematode abundance at or near the seep centre was ca. 45% higher at the Maungaroa and Urutī South areas (maximum abundance ca. 2300 ind. 0.1 m-2 at both areas) compared to the Glendhu area (ca. 1600 ind. 0.1 m-2).
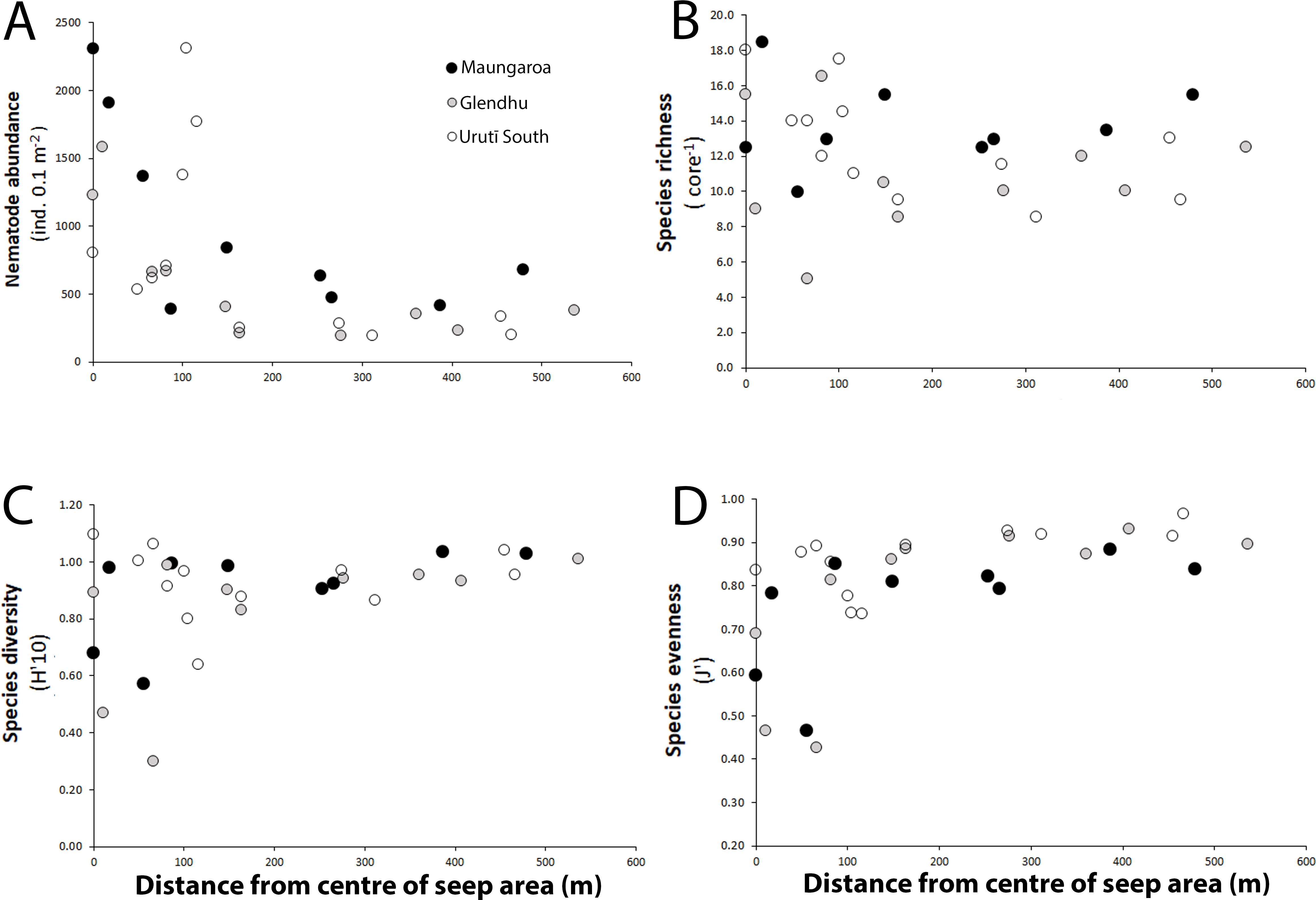
Figure 3. Relationship between distance from the centre of the seep areas and (A) nematode abundance, (B) species richness, (C) species diversity and (D) species evenness. Black filled circles = Maungaroa seep area; grey filled circles = Glendhu seep area; empty circles = Urutī South seep area.
Species richness ranged from 5 to 18 species per core across the study areas. No clear within-area trend in species richness was observed at the Maungaroa and Glendhu areas, however, values tended to be more variable near the seep centre (Figure 3). At the Urutī South area, species richness values were elevated near the seep centre relative to values in the seep periphery. Species diversity (H’) and evenness (J’) showed a different trend, with the lowest values observed near the seep centres but with substantial variation among locations, whereas values were uniformly high in the seep periphery of all areas (Figure 3).
A total of 3374 nematode specimens were identified. Of these, 96% were identified to species or morphospecies, while the rest of the specimens was identified to genus or family due to poor preservation or because they were juveniles. Overall, 56 genera were identified with Sabatieria the most common genus by far (accounting for 28% of all nematodes), followed by Rhabdodemania (10%), Paramesacanthion and Oncholaimus (8% each), Odontophora (6%) and Halalaimus (5%). All other genera represented 4% or less of the total. A total of 103 nematode species comprising 17 named species and 86 morphospecies were identified (Supplement D). A total of 56, 60 and 67 species were identified from the Maungaroa, Glendhu and Urutī South areas, respectively, with 26 species present across all three areas, 29 species found at two of the three areas and 48 species found at only one area. Thirteen species were only found at the Maungaroa area, including Odontophora B, the most common species at that area. A similar number of species (14) were found only at the Glendhu area, all of which were relatively uncommon or rare (average abundance < 3 ind. 0.1 m-2). Twenty-one species were found exclusively at the Urutī South area, including the relatively abundant Oncholaimus A, Dorylaimopsis nodderi and Deontostoma tridentum (average abundance > 10 ind. 0.1 m-2).
There was a significant difference in nematode species community structure among the seep areas (PERMANOVA, P = 0.0001), with all areas significantly different from each other (pairwise PERMANOVA, P < 0.02; Figure 4). The similarity between the nematode communities at the shallower Urutī South area and the two deeper Maungaroa and Glendhu areas was low (average similarity = 26% between each pair) relative to the similarity between the Maungaroa and Glendhu communities (35%). There was no significant difference in multivariate dispersion among the areas (PERMDISP, P > 0.1). A number of species accounted for a substantial proportion of within-area similarity across all areas, including Paramesacanthion A, Rhabdodemania zealandiaensis, Oncholaimus adustus and Odontanticoma A (Table 2. Among-area dissimilarity was mostly due to differences in the abundances of species, although as noted above some species were found only at one or two of the areas (Table 3). For example, Sabatieria bathycopia was present at all three areas, being most common at Glendhu, followed by Maungaroa then Urutī South, while Sabatieria articulata and Sabatieria balbutiens were most abundant at Urutī South, less abundant at Maungaroa, and absent from Glendhu.
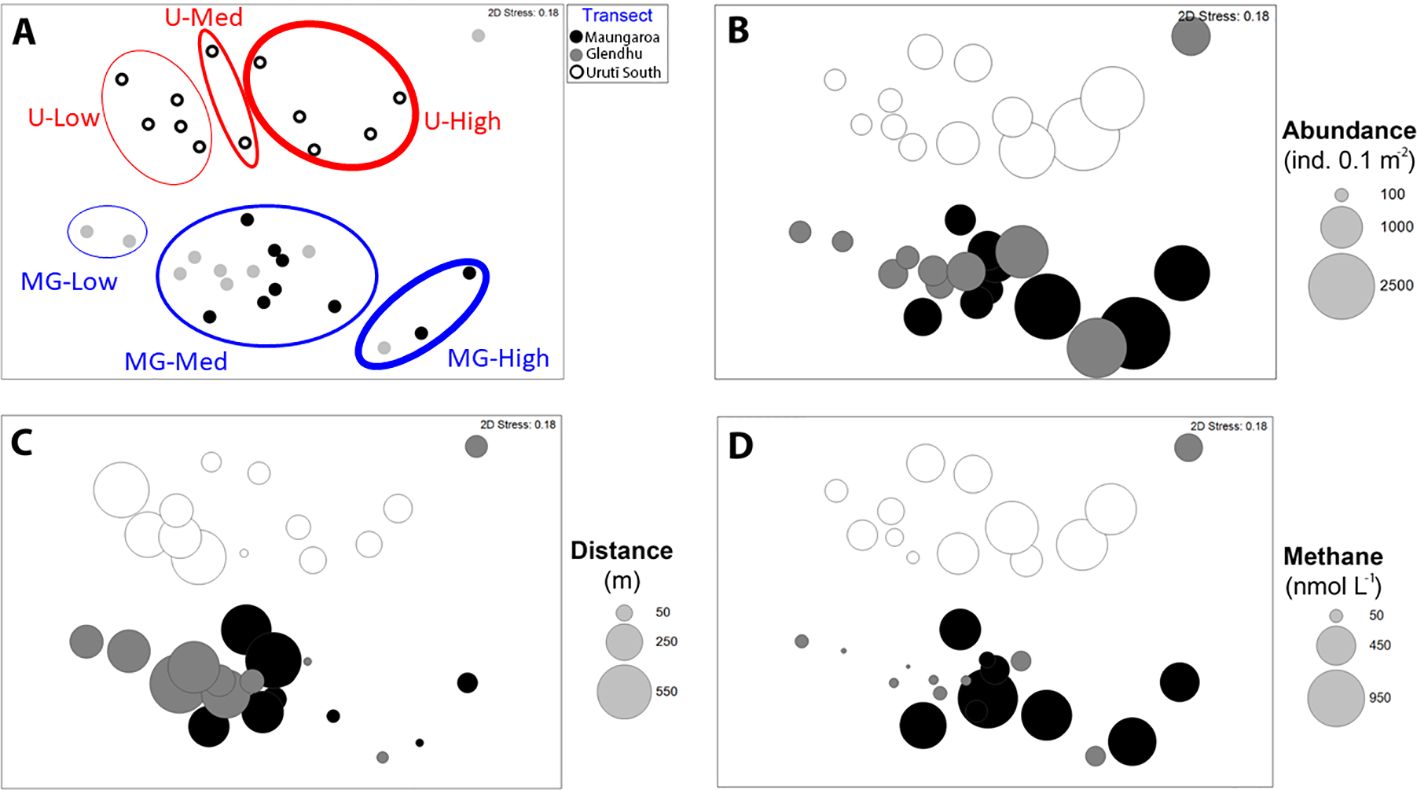
Figure 4. Two-dimensional multidimensional scaling (MDS) plot of square root-transformed nematode species abundance data at the Maungaroa (black filled circles), Glendhu (grey filled circles) and Urutī South (empty circles). The groups identified by SIMPROF are circled with blue (Uriti South groups) and blue lines (Maungaroa and Glendhu groups) (A). The size of the bubbles on (B), (C) and (D) indicate total nematode abundance, distance from the centre of the seep area, and methane concentration, respectively. U-High = Urutī South high abundance group; U-Med = Urutī South medium abundance group; U-Low = Urutī South low abundance group; Mg-High = Maungaroa & Glendhu high abundance group; MG-Med = Maungaroa and Glendhu medium abundance group; MG-Low = Maungaroa and Glendhu low abundance group.
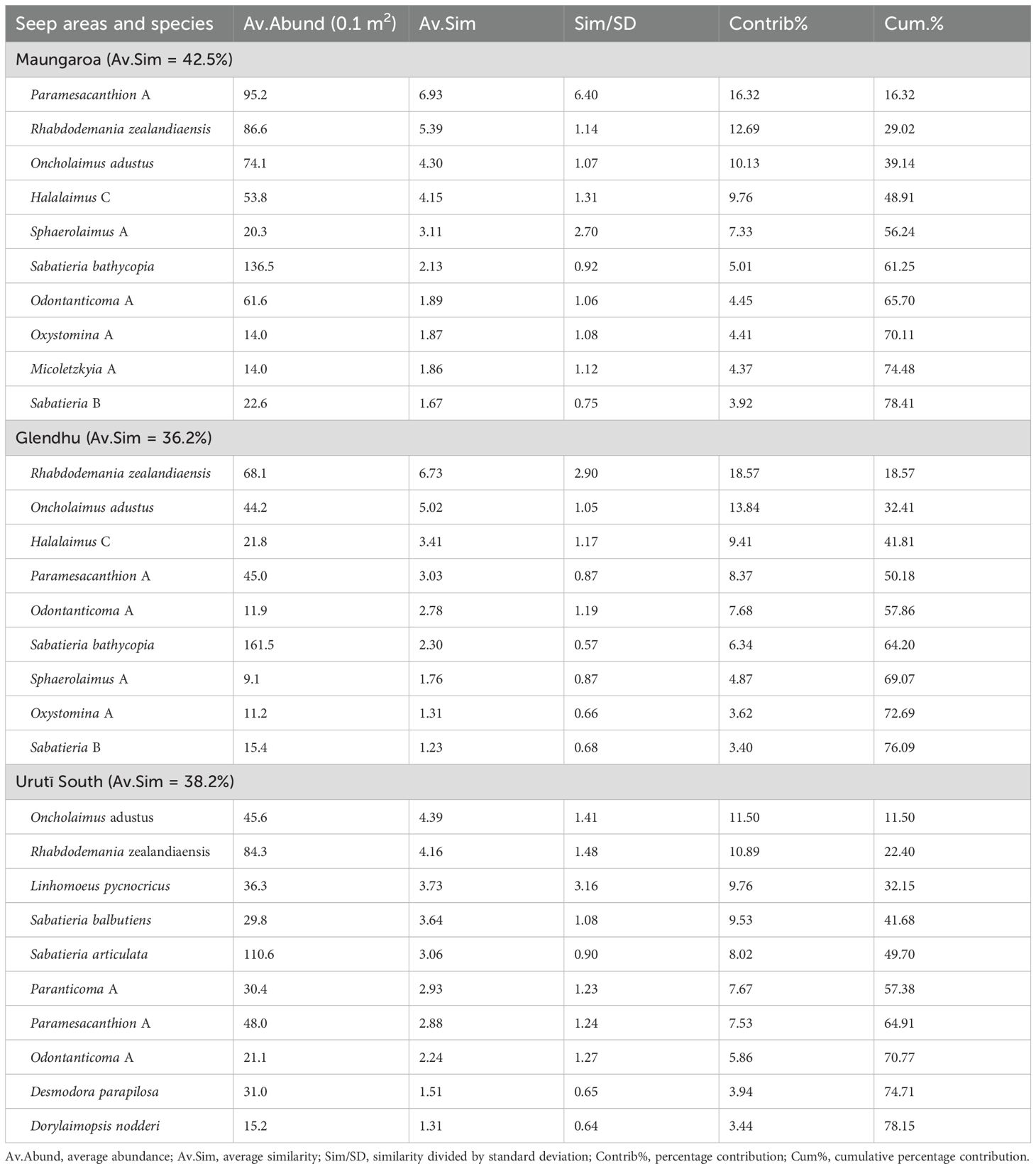
Table 2. Result of SIMPER analyses showing nematode species accounting for most of the within-site similarity at the Maungaroa, Glendhu and Urutī South seep areas.
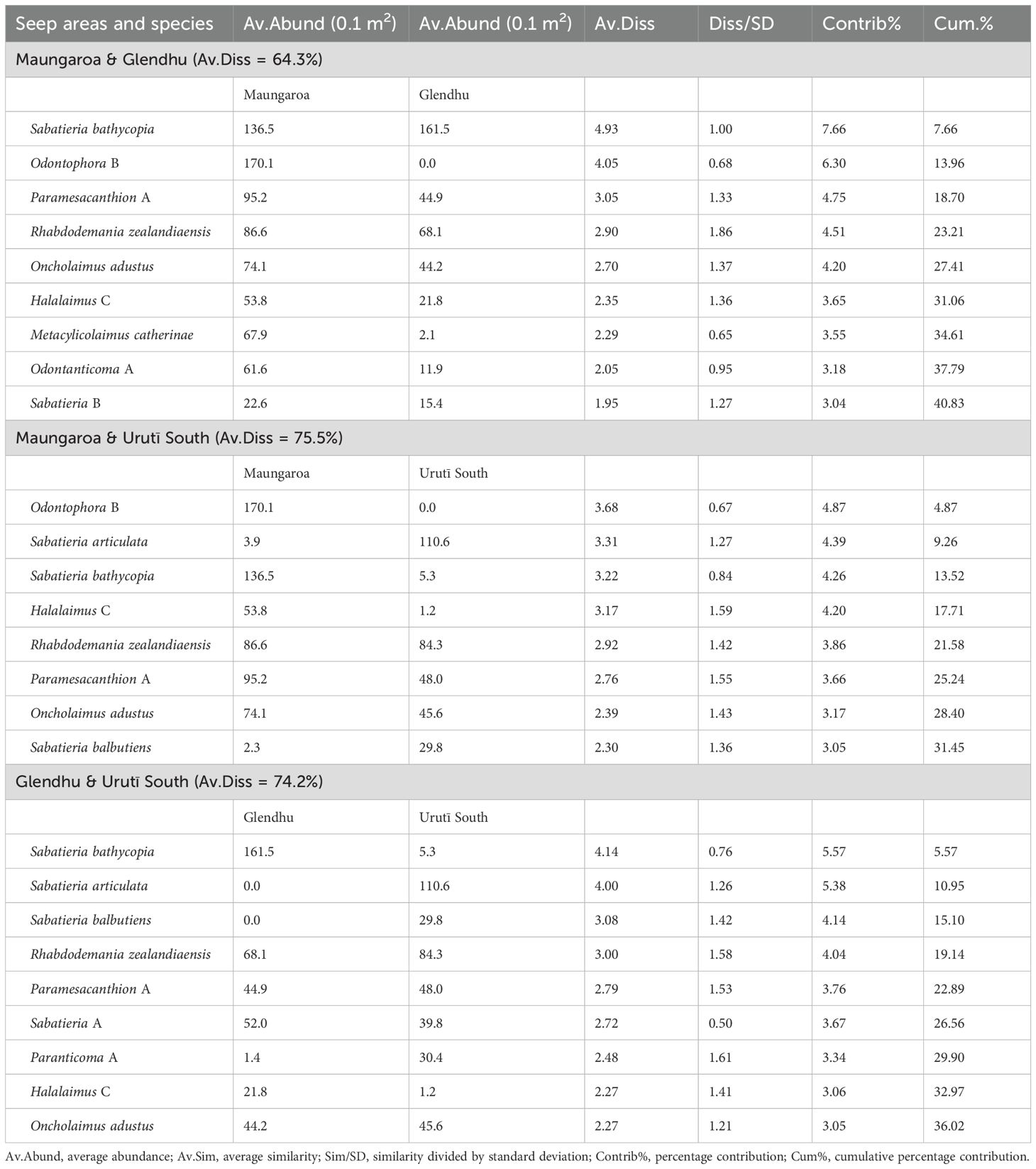
Table 3. Result of SIMPER analyses showing nematode species accounting for among-area dissimilarity among the Maungaroa, Glendhu and Urutī South seep areas.
The Cluster and SIMPROF analyses identified six groupings, leaving one sampling location (Glendhu area, station 42, represented by one core) unclustered (Figure 4). This sample was unusual in that only five species were found there (species richness ranged from 8.5 to 18.0 per core at the other locations), with most (82%) specimens identified as Sabatieria A. Three of the SIMPROF groups comprised samples from the Urutī South area, with the remaining three groups comprising a combination of Maungaroa and Glendhu samples or samples from Glendhu only. The Urutī South groups were: “U-High”, comprising samples with high nematode abundance (702-2310 ind. 0.1 m-2), “U-Med”, comprising samples with medium nematode abundance (534-801 ind. 0.1 m-2), and “U-Low”, comprising samples with low nematode abundance (190-330 ind. 0.1 m-2) (Table 4; Figure 5). The Maungaroa/Glendhu groups were: “MG-High” (1369-2310 ind. 0.1 m-2), “MG-Med” (232-1919 ind. 0.1 m-2) and “MG-Low” (190-211 ind. 0.1 m-2). The U-High group comprised samples located relatively near (67-116 m) to the centre of the Urutī South area, whereas the corresponding group MG-High comprised samples located at the centres (0-55 m) of the Maungaroa and Glendhu areas. These high abundance groups were characterised by low species evenness (J’ = 0.46-0.89) relative to the medium and low abundance groups at their respective areas (Table 4). Both U-Low and MG-Low comprised samples taken at least 164 m away from the centres of their respective areas and were characterised by relatively high species evenness (J’ = 0.89-0.96). Some species such as Rhabdodemania zealandiaensis and Oncholaimus adustus were relatively common across all or most of the groups, whereas others such as Metacylicolaimus catherinae and Linhomoeus pycnocricus characterised only one or two groups. Within-group similarity for group U-High was mainly due to high relative abundance of Sabatieria articulata, Rhabdodemania zealandiaensis and Desmodora parapilosa, whereas the MG-High group was mainly characterised by Sabatieria bathycopia, Paramesanthion A and Odontophora B. The groups U-Med and GM-Med were both characterised by high abundance of Oncholaimus adustus and Rhabdodemania zealandiaensis, however the remaining characterising species differed between the groups. Group U-Low was characterised by high relative abundance of Sabatieria balbutiens, Linhomoeus pycnocricus and Oncholaimus adustus whereas group MG-Low was mainly characterised by Oncholaimus adustus, Rhabdodemania zealandiaensis and Halalaimus C.
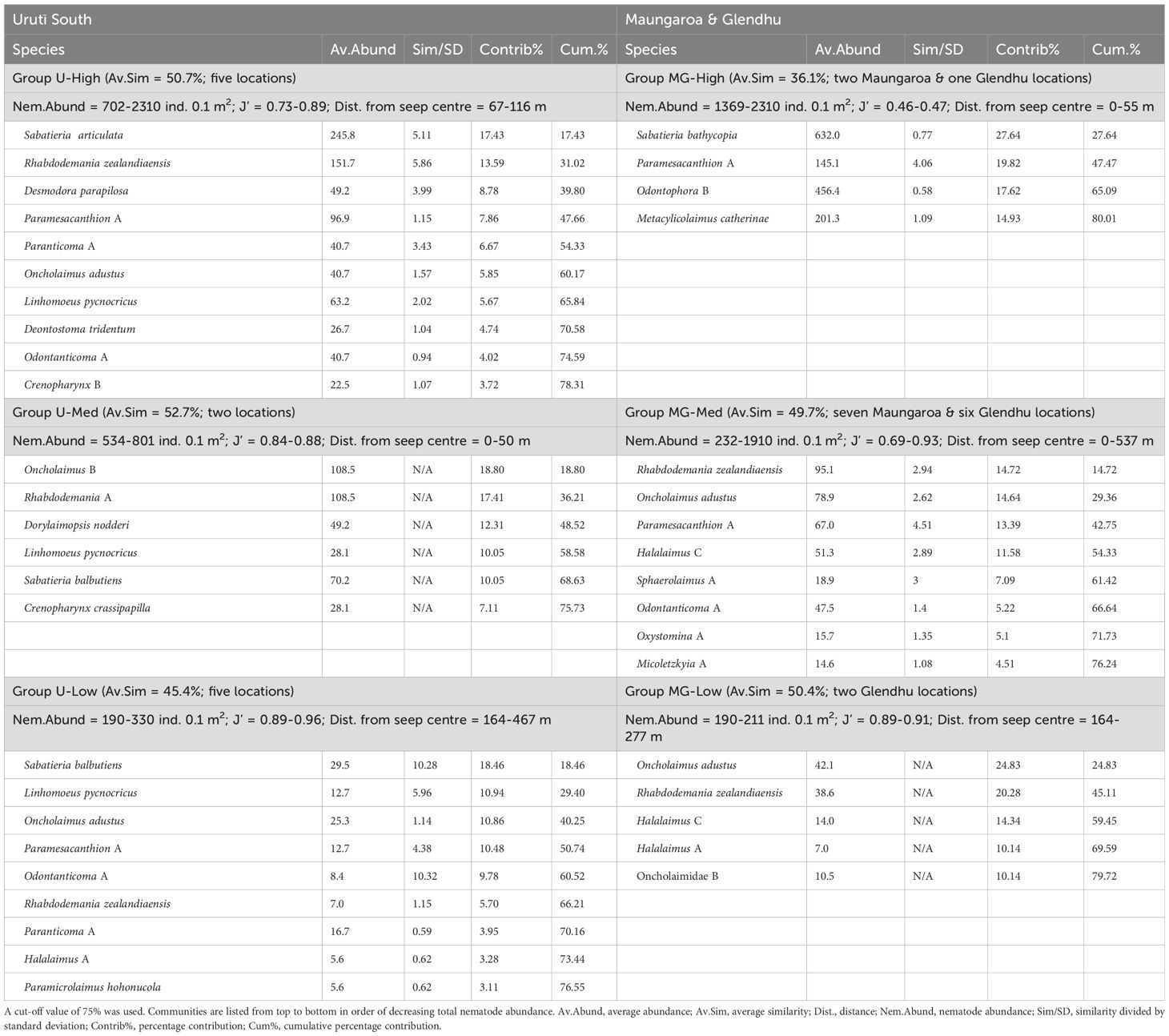
Table 4. Result of SIMPER analyses showing nematode species accounting for within-group similarity for each of the communities identified by SIMPROF analysis at the Urutī South (left-hand side columns) and Maungaroa and Glendhu areas (right-hand side columns).
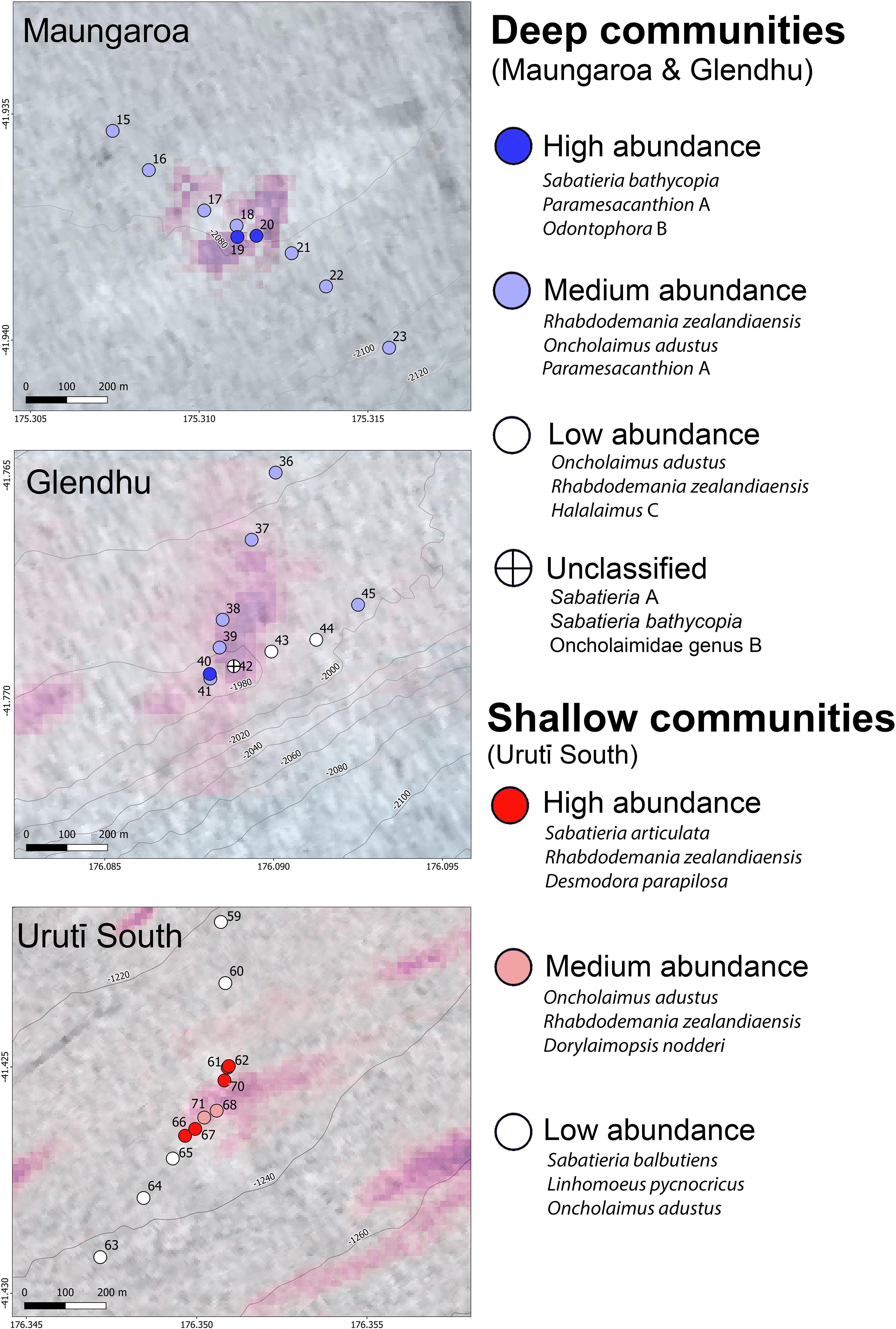
Figure 5. Spatial distribution of the six nematode communities (and one unclassified sample) identified by SIMPROF analysis at the Maungaroa, Glendhu and Urutī South seeps. The three species accounting for most of the within-community similarity (based on SIMPER analysis) are listed on the right. See Table 4 for detailed description of each group.
Nematode communities were dominated by deposit feeders and facultative predators, which together comprised over 70% of the community overall. There was substantial variability in the relative abundance of nematode feeding groups among the groups identified by the Cluster and SIMPROF analyses (Figure 6). Microvores, which include the common genus Halalaimus, tended to be more abundant at the deep Maungaroa and Glendhu areas than at the shallower Urutī South area. Average relative abundance of microvores was lowest (0-5% of total) in the high abundance groups (U-High and MG-High) but substantially higher (5-31%) in the U-Low, MG-Low and MG-Med groups. Deposit feeders, which included the highly abundant genus Sabatieria, were relatively abundant in all communities, averaging 33-59% of total abundance in the Urutī South communities, and 23-24, 10-46 and 13-82% in the MG-Low, MG-Med and MG High communities, respectively. Epigrowth feeders were present in low abundances in all the groups but tended to be somewhat more abundant in the Urutī South groups (0-16%) than in the deeper Mungaroa-Glendhu groups (0-6%, except for station 18, where epigrowth feeders represented 21% of total). Ciliate feeders were absent in 18 of the 30 cores we analysed and were relatively unabundant (¾8%) in most groups except for stations 19 and 20 (MG-High group) where they represented 22 and 65% of total abundance due to elevated abundances of Dorylaimopsis nodderi and Odontophora sp. B, respectively. Facultative predators were common in all groups, averaging 11-38% of total nematode abundance in the Urutī South samples. Among the Mungaroa-Glendhu groups, facultative predators were most abundant in the MG-Low group (52-62%), followed by the MG-Med (27-46%) and Mg-High groups (10-19%). Relative abundances of predators were relatively low (<10%) at most sites but reached values of 15-35% at some U-High, MG-High and MG-Med sites.
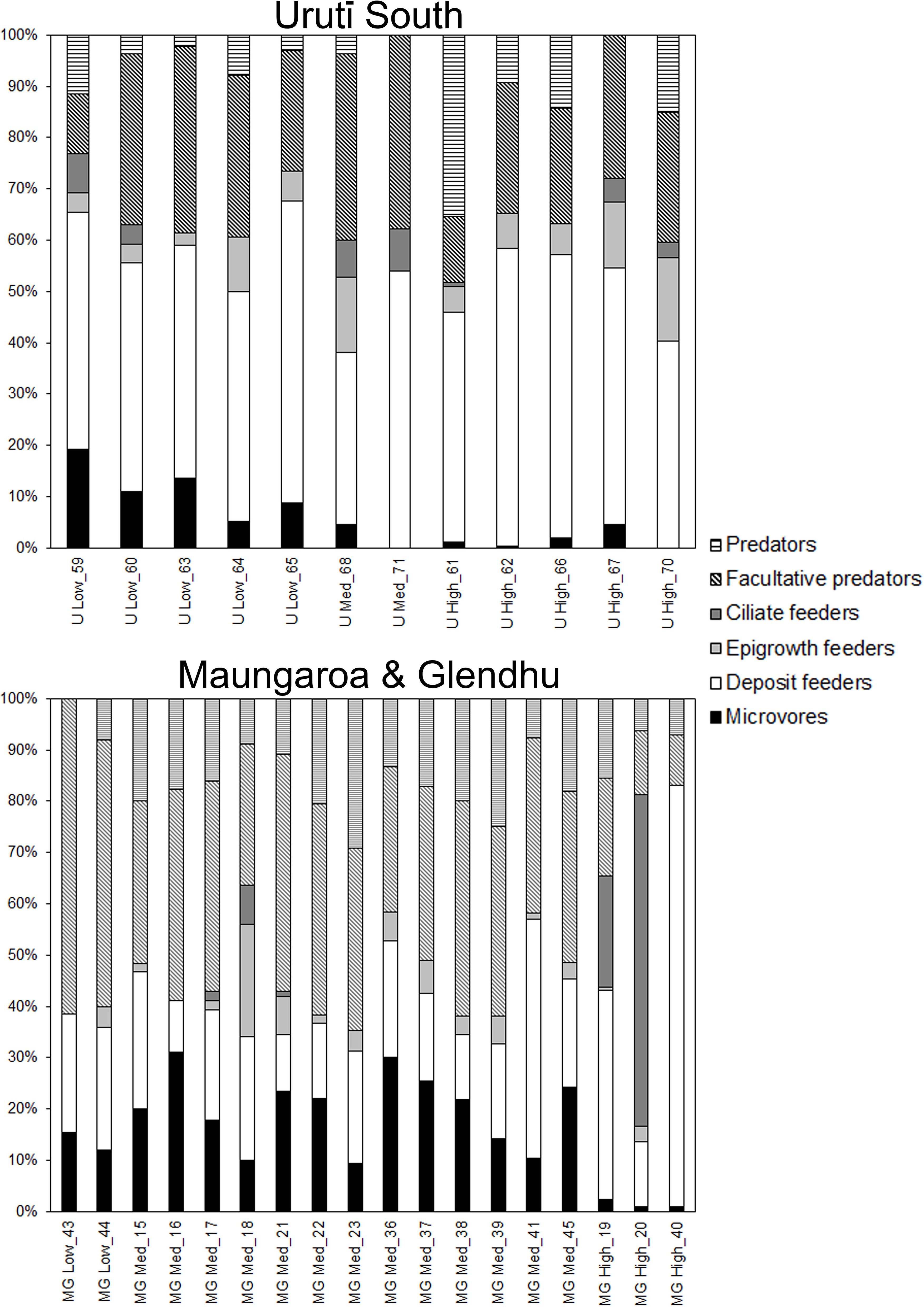
Figure 6. Relative abundance of nematode feeding groups at the Urutī South (top) and Maungaroa and Glendhu seep areas (bottom). U-High, Urutī South high abundance group; U-Med, Urutī South medium abundance group; U-Low, Urutī South low abundance group; Mg-High, Maungaroa & Glendhu high abundance group; MG-Med, Maungaroa and Glendhu medium abundance group; MG-Low, Maungaroa and Glendhu low abundance group.
Some trends were observed in the distribution of feeding groups in relation to distance from the centre of the seep areas (Figure 7). Deposit feeders, and to a lesser extent epigrowth feeders and ciliate feeders, tended to be most abundant at or near the centre of the seep areas, whereas microvores showed the opposite trend. No consistent trend was seen in the relative abundance of facultative predators and predators.
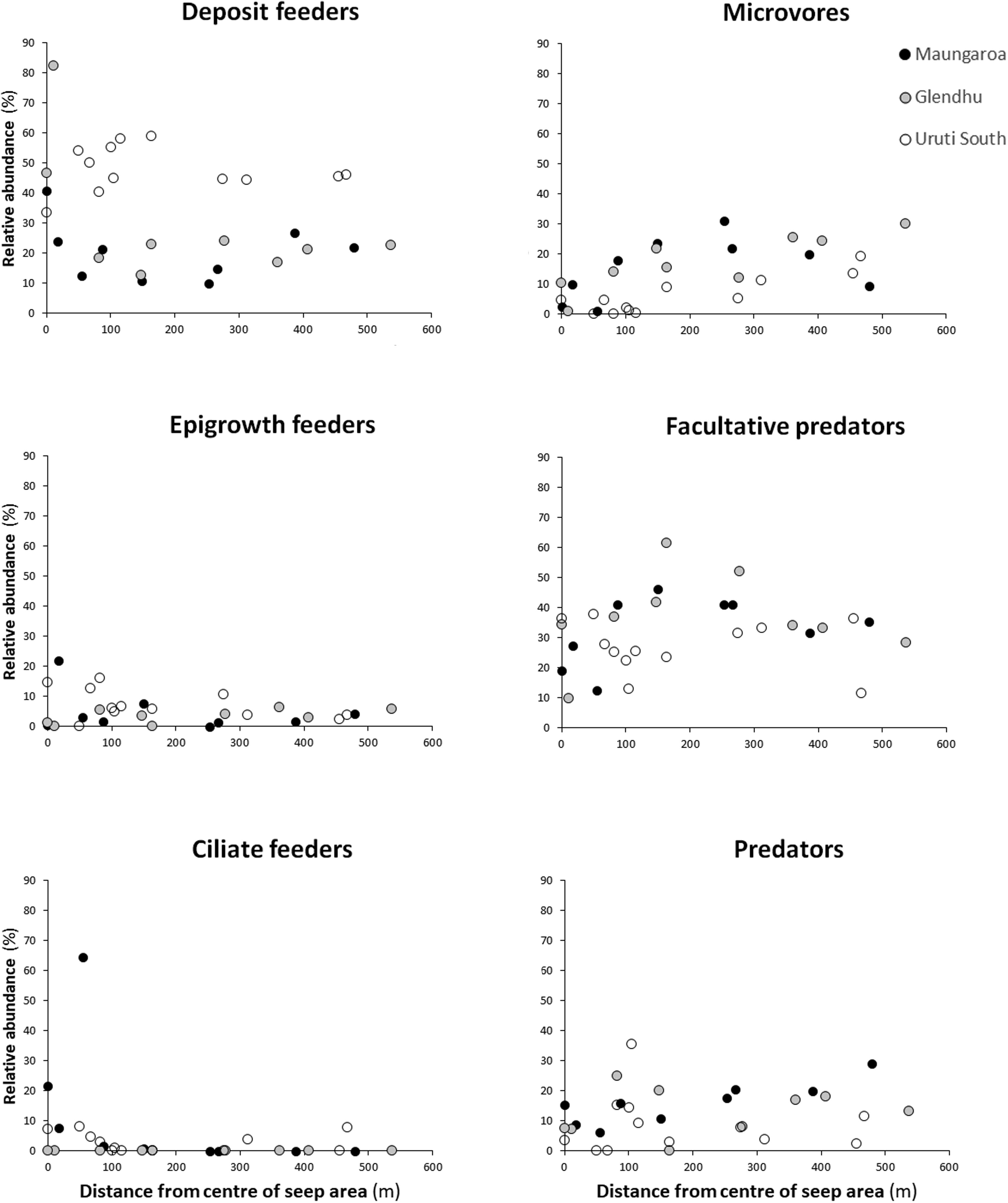
Figure 7. Relative abundance (%) of nematode feeding groups as a function of distance from the centre of seep areas. Black filled circles = Maungaroa seep area; grey filled circles = Glendhu seep area; empty circles = Urutī South seep area.
The results of DistLM analyses showed that nematode abundance was significantly and negatively correlated with distance from the centre of the seep areas at the Maungaroa and Glendhu areas (P < 0.05, R2 = 0.48-0.59), but not at the Urutī South area where abundance was highest some distance away from the centre (P < 0.05; Table 5; see Figure 3). Nematode abundance was also positively correlated with mean grain size at the Glendhu and Urutī South areas (P < 0.01, R2 = 0.68-0.83), positively correlated with sediment carbonate content at the Maungaroa area (P < 0.05, R2 = 0.58) and positively correlated with porewater methane concentrations at the Maungaroa and Urutī South areas (P<0.05, R2 = 0-37-0.60). Nematode species richness was not significantly correlated with any of the predictor variables at the Maungaroa and Urutī South areas (DistLM; P > 0.05). At the Glendhu area, species richness was positively correlated with sediment organic matter content and sediment carbonates content (P < 0.05, R2 = 0.54-0.57). Species evenness (J’) and diversity (H’10) were significantly and negatively correlated with proxies of food availability at all three areas, i.e., chlorophyll a and phaeopigment concentrations and sediment organic matter content, as well as sediment carbonates (P < 0.05, R2 = 0.45-0.83). Significant negative correlations were also observed with the ratio of chlorophyll a to phaeopigment (proxy for food quality, P < 0.05, R2 = 0.44-0.62) and porewater and water column methane concentrations (P < 0.05, R2 = 0.62-0.83). Nematode community structure was significantly correlated with a number of food availability proxies (porewater and water column methane concentration, pigment and organic matter concentrations, and/or distance from seep centre) and particle size variables (mean grain size and/or particle skewness), and/or carbonate content at all three areas. These significant correlations, however, were weaker (R2 = 0.21-0.44) than for the univariate variables (R2 = 0.45-0.83).
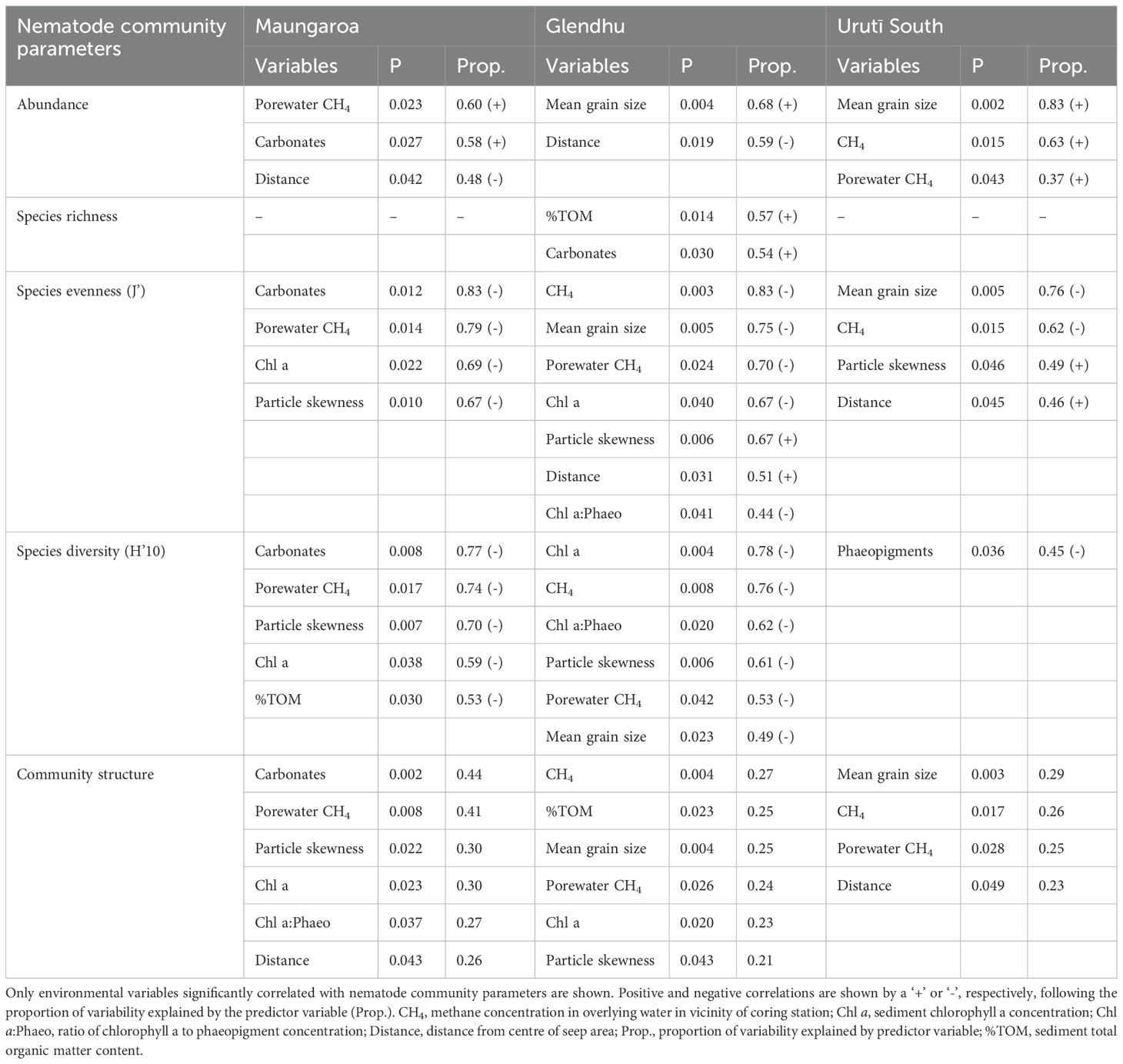
Table 5. Result of DistLM analyses showing the relationships between environmental variables and nematode community parameters.
Discussion
The present work was undertaken as part of a wider study to characterise the marine ecosystem of an area where gas hydrate extraction could occur in the future. This study is the first to characterise macrofaunal nematode communities of cold seeps and provides the first insight into the distribution of nematode species in deep-sea cold seep habitats of the New Zealand region. Our analyses have uncovered significant heterogeneity in the species structure and trophic make up of nematode communities across the three Hikurangi Margin seep areas, as well as within each of the areas, and show that this variability was linked to a range of proxies of food availability and sediment grain size variables. These results could be used for assessing the potential effects that could arise from disturbances that might relate to future gas hydrate extraction in the region.
Variability in environmental variables
The three seep areas on the Hikurangi Margin shared some similarities in environmental conditions despite one area (Urutī South at 12000 m) being substantially shallower than the other two (Glendhu and Maungaroa at ca. 2000 m), while also exhibiting some significant differences. One difference was the almost one order of magnitude lower methane concentrations in the water overlying the sediments at the Glendhu area relative to the Maungaroa and Urutī South areas. This trend among the three areas was also noted by Law et al. (2024) who suggested that the lower dissolved methane maximum at Glendhu may reflect that this area is characterised by a number of smaller dispersed seeps (Turco et al., 2022). Porewater methane concentrations showed the opposite pattern with the highest values observed at Glendhu. Estimated gas fluxes at the study areas overlap considerably and do not indicate substantially lower fluxes at Glendhu (1.6-4.3 × 106 kg yr-1) compared to Maungaroa (0.3-7.2 × 106 kg yr-1) or Urutī South (1.3-3.6 × 106 kg yr-1), with fluxes likely to be stable over time, although current estimates are likely affected by a range of methodological assumptions and uncertainties (Turco et al., 2022; Law et al., 2024). It is possible that the low methane concentrations in the overlying water observed at the Glendhu area reflects the effect of local currents or variation in the position of the samples relative to the areas of most active seepage rather than among-area difference in gas fluxes.
Despite the observed differences in methane concentrations observed among the study areas, we did not observe any obvious difference in sediment organic content among areas, indicating that sediment food availability for benthic fauna was similar. The three seep areas were all characterised by elevated organic matter concentrations ranging from 7.9 to 10.1% without obvious within-area trends. Similar elevated values of sediment organic matter content have been observed at other seeps (e.g., Seabrook et al., 2024), but values in Kaikōura Canyon, located south of Hikurangi Margin and characterised by the highest benthic biomass of any known non-chemosynthetic deep-sea environment (De Leo et al., 2010), are relatively low, ranging from 2.2 to 6.8% (Leduc et al., 2014). These high organic matter concentrations were observed across all seep areas we sampled, and as far as 250 m away from the seep centre, suggesting that the influence of methane seepage on organic matter availability extends to the edge of the areas and likely beyond. It is not clear how methane seepage may influence sediment organic matter concentrations at the edge of the areas up to 250 m or more away. Studies have found little if any difference in proxies of food quantity or quality (e.g., %C, %N, C:N) between seeps and adjacent non-seep sediments, perhaps due to high variation among seep macrohabitats (Levin et al., 2010; Ritt et al., 2012). Inputs of chemosynthetic carbon may not necessarily be reflected in sediment organic matter concentrations due to high turnover and consumption rates by infauna (Nascimento et al., 2012). The ratio of chlorophyll a to phaeopigment concentration, which gives an indication of the freshness (i.e., lability) of phytodetrital food sources, did not show any clear within-area spatial patterns, but this is not unexpected as pigment concentrations reflect inputs from surface primary productivity rather than in situ chemosynthesis. Among-area variability in pigment concentrations was observed, with elevated chlorophyll a and phaeopigment concentrations at the Maungaroa area relative to the Glendhu and Urutī South areas, indicating greater supply of phytodetrital food sources at the former. The Maungaroa area is located to the southwest of the other two areas, and is the area closest to the Subtropical Front, a region associated with heightened primary productivity (Bradford-Grieve et al., 1997; Murphy et al., 2001).
Sediments at the three seep areas were characterised by similar grain size distributions dominated by fine, medium and coarse silt particles. At Glendhu and Urutī South, however, mean sediment particle size tended to be highest at or near the centre of the area and was significantly and positively correlated with methane concentrations in the water column (DistLM, P < 005, R2 = 0.71 and 0.78, respectively). Seepage can affect sediment grain size distribution through mechanical disturbance by bubbling gas which may remove the finer particles or reduce their deposition (Luth et al., 1999; Dando and Hovland, 1992). Coarser particles may also result from microbially mediated authigenic carbonate production via in situ production of relatively large carbonate accretions or through the erosion of nearby carbonate mounds (Bourque et al., 2017). Carbonate concentrations tended to be most elevated at or near the seep centres, which aligns with localised precipitation of authigenic carbonates from anaerobic methane oxidation.
Nematode abundance, species richness and diversity
There were no significant difference in nematode abundance, species richness, diversity and evenness among the three study areas, which may reflect the lack of any obvious difference in sediment organic matter, carbonate content or sediment grain size among them. However, a consistent spatial pattern in nematode abundance was observed within all the study seep areas on the Hikurangi Margin: abundance was highest at or near the seep centre, decreased steeply away from the centre and was low in the periphery (> 150-200 m from the centre). These spatially consistent patterns clearly reflect the influence of methane seepage, which appears to be limited to the inner 150-200 m radius of each area on Hikurangi Margin, on nematode abundance via input of chemosynthetic food sources. However, sediment organic matter concentrations were elevated both in the centres and peripheries of all three study areas, similar levels of food availability across the methane seepage gradient. This seemingly contradictory observation, both in the present study and previous studies (Levin et al., 2010; Ritt et al., 2012), could indicate that organic matter quality (lability) rather than quantity is the most important factor influencing nematode abundance (e.g., Cook et al., 2000). Areas of methane seepage at or near the seep centres were associated with nematode abundance up to ca. 6, 8 and 12 times higher at the Maungaroa, Glendhu and Urutī South areas, respectively, compared to values at their respective peripheries. Similar differences in macro-infaunal abundance have been found in several studies of continental slope seeps and adjacent non-seep sediments, with 2 to 10-fold greater macroinfaunal abundance in the former relative to the latter (Robinson et al., 2004; Bernardino and Smith, 2010; Levin et al., 2010; Menot et al., 2010; Ritt et al., 2012; Bourque et al., 2017; Campanya-Llovet and Snelgrove, 2018; Washburn et al., 2018).
Although the same spatial trend was observed at all three areas, we also saw somewhat elevated nematode abundances in the periphery of the Maungaroa seep area (421-681 ind. 0.1 m2) compared to the nematode abundance in the periphery of the other two areas (190-379 ind. 0.1 m2). This contrast is consistent with the elevated pigment concentrations observed throughout the Maungaroa area, which likely reflects its proximity to the highly productive Subtropical Front (Bradford-Grieve et al., 1997; Murphy et al., 2001). Among-area difference in peak nematode abundance at or near the centres of the seep areas were also observed, with a low value observed at Glendhu (1580 ind. 0.1m2) relative to Maungaroa and Urutī South (2310 ind. 0.1 m2 at both areas). It is not clear whether this difference reflects small-scale spatial patchiness in nematode abundance or lower food availability of photo- and/or chemosynthetic origins at the Glendhu area. There were no clear indications of low sediment food availability at the former area, although relatively low water column methane concentrations may reflect lower flux rates and therefore less chemosynthetic primary production.
Species richness did not exhibit clear patterns at any of the areas but tended to be most variable near the seep centres, whereas species diversity and evenness were lowest at or near the seep centres and elevated in the periphery. Elevated infaunal abundance in seeps is often associated with lower diversity and/or evenness relative to adjacent non-seep sediments (Dando et al., 1991; Bernardino and Smith, 2010; Menot et al., 2010; Ritt et al., 2012; Bourque et al., 2017; Campanya-Llovet and Snelgrove, 2018; Macheriotou et al., 2021) although this pattern is not always clear (Shirayama and Ohta, 1990; Levin et al., 2010). Decreased infaunal diversity and dominance by one or a few species in seeps is often observed and is likely a function of competitive displacement by species able to exploit the abundant food resources and increased stress from low oxygen and toxic sulphides, which only a limited number of taxa are able to withstand (Sahling et al., 2002; Levin et al., 2003; Levin, 2005). Among the infauna, nematodes appear to be particularly well-adapted to cope with high sulphide and low oxygen conditions, with a small number of opportunistic species able to exploit the abundant food resources present at seeps (Steichen et al., 1996; Van Gaever et al., 2006; Vanreusel et al., 2010a). It is not entirely clear how some nematode species cope with the toxic seep conditions, but some documented adaptations include ovoviviparous reproduction, storage of sulphur in intracellular inclusions, and a distribution restricted to the topmost layer of sediments (Thiermann et al., 2000; Van Gaever et al., 2006). Unlike macro- and megafauna associated with seeps, there is limited evidence of associations with ecto- or endosymbiotic bacteria in nematodes of deep-sea cold seep environments, although they have been documented in shallow water seeps (Dando et al., 1991; Jensen et al., 1992).
Comparisons between our results and published data are limited by the relatively small number of studies on deep-sea macrofaunal nematodes and the range of mesh sizes used by different authors (250 to 500 μm). The impact of mesh size on estimates of macrofaunal nematode abundance was demonstrated in a study of macrofaunal nematodes of cold seeps on the Norwegian margin (732-1263 m depth) by Decker et al. (2012), who found nematode abundances ranging from 4 to 1015 ind. 0.1 m-2 when using a 250 μm mesh, which are similar, though not as high, as our estimates of nematode abundance at the Hikurangi margin seep sites based on a 300 μm mesh. The latter authors, however, found much lower abundances (0 to 56 ind. 0.1 m-2) when using a 500 μm mesh. In the eastern Mediterranean, macrofaunal nematode abundance based on a 250 μm mesh reached 928 ind. 0.1 m-2 at the Napoli mud volcano and 2612 ind. 0.1 m-2 at the Amsterdam mud volcano, respectively (Ritt et al., 2012), which is similar to the peak abundances (1580-2310 ind. 0.1 m-2) we observed in at our study sites. The nematode abundances we observed are high relative to abundances reported by Sharma and Bluhm (2011) from the Arctic deep-sea Canada Basin. The latter authors used a 250 μm mesh to quantify the abundance of macrofaunal nematodes in box-corer samples. Mean nematode abundance was highest at their Chukchi Sea slope sites (898-1945 m depth; 400 to 515 ind. 0.1 m2), which is within the range of values we observed in samples from the periphery of the seeps at similar depths. However, mean nematode abundances at the other twelve sites sampled by Sharma and Bluhm (2011) at 640 to 3850m depth were much lower and did not exceed 110 ind. 0.1 m2. A study by Gunton et al. (2016) on the macrofaunal nematodes of the deep Whittard Canyon and adjacent slope (3500 m depth, NE Atlantic) found relatively low mean abundances ranging from ca. 15 to 45 ind. 0.1 m2 at slope and canyon sites. These low values likely reflect the relatively coarse mesh size (500 μm) used by the latter authors. Hunter et al. (2013) found higher macrofaunal abundance (ca. 190 ind. 0.1 m2) at two sites in the Whittard Canyon at similar depths, which likely reflects the use of a finer (250 μm) mesh size than Gunton et al. (2016). The nematode abundances observed by Hunter et al. (2013) are at the lower end of values we observed at the periphery of our Hikurangi Margin seep areas, which is likely due to our seep sites being located at shallower depths (ca. 1225-2100 vs 3500 m). A study on Black Sea shelf cold seeps and adjacent non-seep sites based on box-corer samples (60-150 m water depth, 250 μm mesh size) showed nematodes to be the most dominant macrofaunal taxon with similar abundance at both seep and non-seep sites ranging from 129 to 653 ind. 0.1m2 (Luth et al., 1999), which is within the range of nematode abundances we observed at the periphery of the Hikurangi Margin seep areas. Considering the water depth of the seep sites investigated in the present study, the macrofaunal nematode abundances we observed appear to be relatively high even at the periphery of the seeps where the influence of methane seepage is expected to be negligible. To our knowledge, the peak in abundance observed at the centres of the Maungaroa and Urutī South areas (ca. 2300 ind. 0.1 m2) are higher than any published values for macrofaunal nematode. These peak macrofaunal nematode abundance values are of similar magnitude to total macrofaunal abundance (macrofauna sensu stricto Hessler and Jumars, 1974) from some of the most abundant deep-sea seep infaunal communities documented to date (Levin et al., 2003; Levin, 2005; Levin and Mendoza, 2007; Robinson et al., 2004). The macrofaunal ampharetid polychaete communities described by Thurber et al. (2013) from Hikurangi Margin at 662-1172 m depth are characterised by some of the highest seep macrofaunal densities recorded anywhere (300 μm mesh, 5700 ind. 0.1m2; see Figure 5 in Thurber et al., 2013) but nematodes were not included in their faunal counts. Our findings provides further evidence for the presence of a highly abundant macrofaunal community associated with Hikurangi Margin seep environments; our data, as well as data from deep-sea cold seep habitats on the Norwegian margin (Decker et al., 2012) and mud volcanoes in the deep eastern Mediterranean Sea (Ritt et al., 2012), also show that macrofaunal nematode abundances can be of similar magnitude or greater than all other macrofaunal taxa combined (macrofauna sensu stricto) when a relatively fine mesh is used (250 or 300 μm).
Nematode communities
While there were no differences in univariate community metrics among the Hikurangi Margin seep areas, we found significant differences in nematode community structure among all three areas. However, the SIMPROF results showed that most of heterogeneity in community structure was due to (1) differences between the shallow Urutī South area and deeper Maungaroa and Glendhu areas, and (2) differences among nematode communities of high, medium and low abundance associated with area-specific gradients in methane seepage. The Maungaroa and Glendhu areas shared the same high and medium abundance nematode communities. This high abundance community was located at the centre of both seep areas and was characterised by a small number of dominant species, i.e., Sabatieria bathycopia, Paramesacanthion A, Odontophora B and Metacylicolaimus catherinae. The genus Sabatieria is often associated with organic-rich muddy sediments low in oxygen (Vanreusel, 1991; Vincx et al., 1990) and has been reported as a common or dominant genus in studies of meiofauna associated with cold seeps (Dando et al., 1991; Van Gaever et al., 2009a) and mud volcanoes (Van Gaever et al., 2006). This genus, however, is also found in less food-rich environments and is common or dominant in meio- and macrofaunal samples from open slope environments (Soetaert and Heip, 1995; Muthumbi et al., 2004; Sharma and Bluhm, 2011). Sabatieria bathycopia is a relatively large species (>2 mm body length) originally described from an organic matter-rich site at 1240 m depth on Chatham Rise, located southeast of Hikurangi Margin (Leduc, 2013). The dominance of this species at the centres of the Maungaroa and Glendhu seep areas is consistent with a preference for sediments rich in organic matter. Paramesacanthion and Metacylicolaimus are typically less abundant or absent in meiofauna samples. These genera were not abundant in the macrofaunal nematode material from the deep Arctic and Gulf of Mexico (Sharma et al., 2011; Sharma and Bluhm, 2011) but each represented about 20% of total nematodes identified in a study of macrofaunal nematodes of the Whittard Canyon (Gunton et al., 2016). Very little is known about the ecology of Metacylicolaimus, however, like other species of the genus, M. catherinae is among the largest free-living marine nematode taxa (body length 15-19 mm) and is equipped with a spacious buccal cavity with teeth and is likely able to prey on other nematodes or other meiofauna (Leduc, 2023a). Paramesacanthion is a more common genus, and like other genera of the family Thoracostomopsidae, is thought to have predatory feeding habits (Jensen, 1987). Odontophora is a nematode genus characterised by a spacious buccal cavity with eversible teeth and has been classified as a non-selective feeder (Wieser, 1953) and ciliate feeder (Moens and Vincx, 1997). Representatives of this genus are mostly found in coastal sediments but also occurs in continental slope environments (Vitiello, 1971). Odontophora was recorded in Canadian Basin of the Arctic Ocean (Sharma and Bluhm, 2011) but to our knowledge no association between this nematode genus and seep habitats has been documented to date. High abundance of this typically shallow water genus at 2000 m depth could reflect the atypically high food availability characterising the Hikurangi Margin seep environments. It should be noted that although this species accounts for a substantial proportion of within-group similarity for the high nematode abundance found at the Maungaroa and Glendhu sites, it was only present at the Maungaroa area.
The medium abundance communities found at both Maungaroa and Glendhu areas, and the low abundance community found at the Glendhu area, were characterised by the same two species, i.e., Rhabdodemania zealandiaensis and Oncholaimus adustus, which together accounted for 30-45% of within group similarity. These two species were common at all three areas and present in all but three and five of the Hikurangi Margin cores we examined, respectively. The presence of these species across a relatively wide range of conditions spanning low to high food availability at both shallow and deep locations suggests that these are generalist species able to thrive in a wide range of environments. Rhabdodemania zealandiaensis also occurs on Chatham Rise, a submarine ridge southeast of Hikurangi Margin, hinting at wide distribution of this species in the region (Leduc, 2023a). Both R. zealandiaensis and O. adustus are characterised by large buccal cavity with teeth and have been classified as omnivores-carnivores in the scheme of Wieser (1953) and as facultative predators by Moens and Vincx (1997), meaning that they can potentially exploit a wide range of food sources ranging from detrital material to microalgae and nematode prey. The predator species Paramesacanthion A and Sphaerolaimus A are also important components of the medium abundance nematode community at Maungaroa and Glendhu, likely reflecting the still sufficiently high abundance of nematode and other prey some distance away from the centre of the highly productive seep areas. Two species of Halalaimus, a microbial feeding genus most commonly found on the lower continental slope and abyssal plain, are characteristic of the low abundance nematode community at the Glendhu area, and are indicative of more typical, lower productivity deep-sea conditions.
The high abundance community at Urutī South was dominated by Sabatieria articulata, a species belonging to the same genus as the dominant species characterising the high abundance community at Glendhu and Maungaroa (Sabatieria bathycopia). Sabatieria articulata was recently described by Fu et al. (2019) from subsurface (1-5 cm) sediments in the Conway Trough at 491-570 m water depth. The Conway Trough is a sedimentary basin immediately adjacent to the highly productive Kaikoura Canyon (ca. 260 km to the south of the study seep areas), and like the latter is thought to be characterised by high food availability. Thus both S. bathycopia and S. articulata appear to have a preference for organically rich environments, but with S. bathycopia typically occurring at somewhat deeper locations (ca. 1200-2100 m water depth) than S. articulata (ca. 500-1200 m). Desmodora parapilosa was another species characterising the high abundance nematode community at Urutī South. Species of this genus are known to be associated with cold seep and mud volcanoes where they can be highly abundant (Van Gaever et al., 2009a; Vanreusel et al., 2010b; Pape et al., 2011; Zeppilli et al., 2011; Macheriotou et al., 2021). Desmodora species, including Desmodora parapilosa, are often characterised by the presence of ectosymbiotic bacteria and/or protists on their cuticle (Blome and Riemann, 1987; Bernhard et al., 2000; Leduc, 2023b), however, the nature of this symbiosis has not yet been investigated. Desmodora is characterised by a buccal cavity with teeth and is classified as an epistrate/epigrowth feeder (Wieser, 1953; Moens and Vincx, 1997), meaning that they can feed on freshly settled microalgae or bacteria attached on the surface of sediment particles or potentially their own cuticle. Rhabdodemania zealandiaensis and Oncholaimus adustus were common species characterising the three communities at Urutī South, with the former species most common in the high and medium abundance communities, and the latter species most common at the medium and low abundance communities. As noted earlier, these species were widely distributed at all three Hikurangi Margin areas where they can potentially exploit a wide range of food sources. Dorylaimopsis nodderi, another common species in the medium abundance community at Urutī South, was originally described from Chatham Rise at 350 m water depth (Leduc et al., 2012). This species possesses a spacious buccal cavity with eversible teeth and has been classified as a non-selective feeder (Wieser, 1953) and ciliate feeder (Moens and Vincx, 1997). Linhomoeus pycnocricus, a non-selective deposit feeder, was a characterising species of the low abundance community. This species, like others in the family Linhomoeidae, is characterised by sometimes abundant dark inclusions in the intestine wall (Leduc, 2023b). These inclusions have been found in a number of marine nematode taxa living in organic rich and low oxygen environments and may act to sequester toxic compounds such as H2S from the environment (Nicholas et al., 1987; Thiermann et al., 1994). The high abundance of this species in the low abundance community found on the periphery of the Urutī South seep area could suggest the presence of reducing conditions. This species has also been found at a shallow (54 m) continental shelf site in the same region (D. Leduc, unpublished data).
Halalaimus and Sabatieria were the most species-rich genera with each represented by six species. The average abundance of Halalaimus, which is generally considered a deep-sea genus particularly abundant in abyssal plains (Miljutin and Miljutina, 2016), was higher at the deeper Maungaroa and Glendhu areas (67 and 39 ind. 0.1m-2) than at the shallow Urutī South area (14 ind. 0.1 m-2). In contrast, the average abundance of Sabatieria, a genus most commonly found in coastal, shelf and continental slope environments (Soetaert and Heip, 1995), was very similar across the areas (196-245 ind 0.1 m-2); however, as noted above there were marked differences in the composition and relative abundances of the different Sabatieria species. Two of the Sabatieria species were present at all three areas (Sabatieria sp. A and S. bathycopia) but with marked differences in relative abundance, and the other species present at Maungaroa and one of the other two areas. These results show that an analysis focusing on nematode genera, as is commonly done in ecological studies of deep-sea nematodes, would have underestimated the heterogeneity in community structure we observed in our species level analyses. However, despite our focus on species, we did not find evidence of seep endemics with the majority of common species occurring at both the centre and periphery of the areas and/or in other deep-sea environments, which is consistent with previous investigations of seep nematodes (Vanreusel et al., 2010b).
Differences in the relative abundance of nematode feeding groups among the SIMPROF-identified communities suggest that there are functional differences in addition to the structural differences discussed above. The shallower Urutī South communities were characterised by greater relative abundances of epigrowth feeders (e.g., Desmodora parapilosa) than the deeper Mungaroa and Glendhu communities, perhaps reflecting greater availability of fresh microalgal detritus at shallower depths. Deposit feeders (e.g., Sabatieria species., Linhomoeus pycnocricus) were generally more abundant at Urutī South while microvores (e.g., Halalaimus species) and facultative predators (e.g., Oncholaimus adustus) were more abundant at the Maungaroa and Glendhu areas, suggesting that seep food webs may vary depending on water depth. The high abundance communities at Urutī South- and Maungaroa/Glendhu were both characterised by low relative abundances of microvores (<2%), indicating that few nematodes specialise in exploiting bacterial food sources at Hikurangi Margin seeps. The high relative abundance of deposit feeders (50-58%) in these communities suggests instead that a broader diet, which may include detritus, bacteria and/or small protists (Moens and Vincx, 1997), is a more successful strategy. Facultative predators, predators and ciliate feeders were also relatively abundant in the high abundance communities, suggesting that macrofaunal nematodes exploit a wide range of food sources at seeps. Overall, our findings are consistent with previous studies of nematode communities at deep-sea cold seeps, which typically find that deposit feeders are the most abundant feeding group (Vanreusel et al., 2010b; Pape et al., 2011). Stable isotope studies suggest that deposit feeding nematodes associated with cold seeps feed on chemosynthetically derived carbon via either direct consumption of sulphur-oxidising bacteria in the sediment (Spies and Des Marais, 1983; Van Gaever et al., 2006; Van Gaever et al., 2009b) or consumption of dissolved organic matter released upon bacterial lysis (Jensen, 1987). However, in contrast to previous studies (Vanreusel et al., 2010b), we have found that predators can be relatively abundant at seeps (up to 17% of total), which may be due to differences in ecology between the macrofaunal (this study) and meiofaunal nematode size fractions (previous studies).
Within area variability in nematode community structure was mainly correlated with food availability variables and also sediment grain size. These results are consistent with the SIMPROF results which identified communities characterised by low, medium and high abundances distributed along gradients in methane seepage. Sediment grain size variables may directly affect nematode community structure through their influence on habitat heterogeneity or food source partitioning (Etter and Grassle, 1992; Leduc, 2012). Alternatively, the relationship between community structure and sediment granulometry may reflect within-area variability in grain size associated with gradients in methane seepage, with coarser mean grain size in areas with high methane flux due to authigenic carbonate production or mechanical disturbance by bubbling gas removing finer particles (Luth et al., 1999; Bourque et al., 2017).
Implications of potential disturbance from gas hydrate extraction
Impacts on the abundance, diversity and structure of benthic communities by conventional oil/gas exploratory drilling and production have been demonstrated for the deep sea, although these impacts are typically observable over relatively small spatial extents (see review by Cordes et al., 2016). Drilling for gas hydrate is likely to take place directly above the hydrate resource, but because extraction can be via directional drilling, the well-head can and will most likely be located away from active seep areas on the seafloor surface. Therefore, while drilling will locally impact ‘background’ benthic communities on the Hikurangi Margin, there is no expectation that drilling infrastructure and products (well-head, cables, drilling rig/platform anchors if used, drilling cutting, etc.) will directly physically disturb the seep habitat and associated communities. However, gas hydrate extraction processes could impact these communities indirectly.
If effective gas production is established, it may reduce or potentially cause cessation of
free-gas methane supply to the seafloor seep (Oluwunmi et al., 2023). The correlation observed in this study between the abundance of nematodes and methane seepage, and associated organic matter, at the centre of the seep areas suggests that any reduction in fluid flux resulting from future hydrate extraction would lead to changes in community structure at seep areas. A potential reduction in the observed elevated levels of abundance could also have implications for nematode communities elsewhere if these high abundance sites act as a ‘hotspot’ source of new recruits and help maintain population connectivity on the margin (Edmunds, 2021). Drilling may also cause free gas to flow through alternative outlets on the seafloor (Oluwunmi et al., 2023). In this case, it is possible that new seep locations with potentially different magnitude of methane supply will cause the development of new seep-related communities. However, while there are indications that seep-related megafaunal communities may take a potentially long time to reach a fully ‘mature’ structure on initiation of new seepage (Bowden et al., 2013), nematode communities will likely establish relatively quickly given that relative abundance, not species composition (including no seep-endemic species), is the primary structural component of the nematode communities observed at the centre of seep areas on the Hikurangi Margin. Abundance in deep-sea nematode communities has been shown to respond to alterations in organic matter availability within weeks to months (e.g., Soltwedel et al., 2018).
The depressurisation involved in the extraction of gas hydrate can cause ‘sand’ production at the seafloor due to the physical degradation of the substrate structure (Moridis and Reagan, 2007). There are means by which this ‘sand’ production can be reduced, for example, downhole sand screens/filters. However, some sediment will necessarily build up as an unconsolidated formation around the screens, and the use of screens may lead to formation of ‘wormholes’, which increase substrate permeability and can act as sites of erosion (Kurihara et al., 2008). Physical disturbance from the deposition of sediment in the vicinity of the well-head will affect ‘background communities’ but not seep communities if the well-head is located away from the seep habitat. The results of this study showed that nematode community structure across the study areas was related to sediment grain size, and therefore it is likely that any changes in sediment structure caused by hydrate extraction will result in concomitant changes in community structure. Studies elsewhere in the deep sea have shown that disturbance-related changes in sediment grain size do affect nematode community structure (e.g., Hasemann et al., 2020). Furthermore, depending on the physical characteristics of the produced ‘sand’, this sediment could be mobilised by local bottom currents and be dispersed from the well-head area to nearby active seafloor seepage areas. Elevations of suspended sediment above background levels can have detrimental effects for benthic fauna, and the ultimate deposition of these dispersed sediments, if above particular depths, can also impact benthic communities (Hendrick et al., 2016). Thus, it is reasonable to predict that any sediment mobilised by the drilling and transported to seep areas will similarly affect the abundance and diversity of nematode communities, although such impacts may be short term for nematodes (Schratzberger et al., 2000).
Extraction of hydrates will alter the structural integrity of the seafloor substrate, leading to subsidence around the well and compression of substrate in immediate region of the well (Jin et al., 2019; Lin and Hsieh, 2020; Zhu et al., 2023). ‘Background’ communities in the vicinity of the well-head will be impacted by such substrate alterations. Up to 8 m subsidence has been observed in large gas reservoirs with no aquifer support (Jin et al., 2019; Lin and Hsieh, 2020; Zhu et al., 2023). However, aquifer support is expected in the configurations investigated for the potential gas hydrate extraction on the Hikurangi Margin (Oluwunmi et al., 2023). Nonetheless, if subsidence does occur, it could cause lateral displacement if the geometry of the slope and sediments were disposed to it. Such lateral displacement could, considering the location of the hydrate deposits on the Hikurangi Margin, trigger slope failure, submarine landslides and turbidity flows (Crutchley et al., 2016). Such disturbance would occur downslope and therefore is unlikely to impact seep-related communities. Physical disturbance from the consequences of slope failure will initially negatively impact the ‘background’ communities, causing major initial losses of benthic fauna followed by a period of community recovery that could take years to decades or potentially cause a shift to an alternative stable state if the disturbance is significant enough (Hecker, 1982). However, studies of meiofaunal response to turbidity flow disturbance indicates that nematode communities are likely to recover from even these large-scale disturbances within a few years (Bigham et al., 2024).
In addition to the most obvious potential impacts from gas hydrate drilling to benthic ecosystems noted above, there is also the possibility of other effects. For example, water is produced from the degradation of hydrate (Moridis and Reagan, 2007; Merey and Longinos, 2018; Zhang et al., 2023) and, depending on the chemical characteristics of this water, could have an impact on benthic communities if this water is disposed of at site and near the seabed. Heating at the well-head and of the extraction pipes could potentially be implemented to counter the freezing that occurs from secondary hydrate formation. The use of inhibitors, such as ethanol, can also be used to counter the freezing (Oluwunmi et al., 2015; Koh et al., 2016). The freezing, heating and/or use of inhibitors could potentially cause local impacts to benthic communities. In addition, there is always the possibility of a ‘blow-out’ event, although a hydrate gas blow-out is considered unlikely, as the reaction is endothermic and would act to shut-off the flow. A free-gas well blow-out is also considered a low-risk event, but it is possible. Sampling to date shows that very limited liquid hydrocarbons are produced during free-gas production, so an oil blow-out would not happen on the scale of and impact to benthic communities of the Horizon Deepwater at Macondo in the Gulf of Mexico (Schwing et al., 2020).
Clearly, the above is a very limited qualitative assessment of the likely impacts to benthic ecosystems that could result from gas hydrate exploitation. A full, and ideally quantitative, ecological risk assessment would need to be followed should the decision be made to proceed towards commercial extraction of gas hydrate on the Hikurangi Margin (Kaikkonen et al., 2018). Such a risk assessment should include the assessment of potential impacts from other human-related activities in the region. For example, assessing the potential cumulative impact from fishing within bottom trawling depth (e.g., Urutī South area) will be necessary given the indications of chronic impact to seep-associated communities by trawling disturbance on the Hikurangi Margin (Bowden et al., 2013). Following risk assessment, management options to mitigate the potential environmental effects from gas hydrate extraction will need to be identified (Ellis et al., 2017). Such mitigation measures can include the implementation of protected areas. In this regard it is worth finally noting that the results of this study showed that the macrofaunal nematode communities at the three different seep areas were different, principally varying with water depth. These differences in community structure will need to be reflected in any spatial management options considered (i.e., have protected sites at different depths and locations on the Hikurangi Margin).
Data availability statement
The original contributions presented in the study are included in the article/Supplementary Material. Further inquiries can be directed to the corresponding author.
Ethics statement
The manuscript presents research on animals that do not require ethical approval for their study.
Author contributions
DL: Conceptualization, Data curation, Investigation, Methodology, Writing – original draft, Writing – review & editing. AR: Conceptualization, Investigation, Methodology, Supervision, Writing – original draft, Writing – review & editing. SS: Data curation, Methodology, Writing – original draft, Writing – review & editing. DB: Data curation, Methodology, Writing – original draft, Writing – review & editing. AT: Investigation, Writing – original draft, Writing – review & editing. JH: Data curation, Methodology, Writing – original draft, Writing – review & editing. CL: Conceptualization, Data curation, Investigation, Methodology, Writing – original draft, Writing – review & editing. OP: Writing – original draft, Writing – review & editing. BW: Writing – original draft, Writing – review & editing. AM: Formal analysis, Methodology, Writing – review & editing.
Funding
The author(s) declare financial support was received for the research, authorship, and/or publication of this article. Funding was provided by the National Institute of Water & Atmospheric Research (NIWA)’s Oceans Centre Research Programme ‘Protecting Marine Biodiversity and the HYDEE research programme for which we thank the funding agencies: Ministry for Business, Innovation and Employment (MBIE) (project C05X1708) and NIWA (under sub-contract GNSMBIE00079). A portion of this work was supported by US National Science Foundation Award 2046800 to ART.
Acknowledgments
We are grateful Grace Frontin-Rollet (NIWA) for sediment sample processing. We thank the science party participants of voyage TAN1904 and the officers and crew of RV Tangaroa, and the programme leaders of the HYDEE project for their unstinting support of the ecosystem research, first Gareth Crutchley and latterly Jess Hillman (both Institute of Geological and Nuclear Sciences, New Zealand). We thank Paul Oluwumni for discussions regarding the potential impacts of hydrate extraction. We are grateful to two reviewers for providing constructive criticisms on the manuscript draft.
Conflict of interest
The authors declare that the research was conducted in the absence of any commercial or financial relationships that could be construed as a potential conflict of interest.
The author(s) declared that they were an editorial board member of Frontiers, at the time of submission. This had no impact on the peer review process and the final decision.
Publisher’s note
All claims expressed in this article are solely those of the authors and do not necessarily represent those of their affiliated organizations, or those of the publisher, the editors and the reviewers. Any product that may be evaluated in this article, or claim that may be made by its manufacturer, is not guaranteed or endorsed by the publisher.
Supplementary material
The Supplementary Material for this article can be found online at: https://www.frontiersin.org/articles/10.3389/fmars.2024.1486305/full#supplementary-material
References
Anderson M. J., Crist T. O., Chase J. M., Vellend M., Inouye B. D., Freestone, et al. (2011). Navigating the multiple meanings of β diversity: a road map for the practicing ecologist. Ecol. Lett. 14, 19–28. doi: 10.1111/j.1461-0248.2010.01552.x
Anderson M. J., Gorley R. N., Clarke K. R. (2008). PERMANOVA+ for PRIMER: guide to software and statistical methods (Plymouth: PRIMER-E).
Baco A. R., Rowden A. A., Levin L. A., Smith C. R., Bowden D. A. (2010). Initial characterization of cold seep faunal communities on the New Zealand Hikurangi margin. Mar. Geology 272, 251–259. doi: 10.1016/j.margeo.2009.06.015
Baldrighi E., Manini E. (2015). Deep-sea meiofauna and macrofauna diversity and functional diversity: are they related? Mar. Biodiversity 45, 469–488. doi: 10.1007/s12526-015-0333-9
Bernardino A. F., Levin L. A., Thurber A. R., Smith C. R. (2012). Comparative composition, diversity and trophic ecology of sediment macrofauna at vents, seeps and organic falls. PloS One 7, e33515. doi: 10.1371/journal.pone.0033515
Bernardino A. F., Smith C. R. (2010). Community structure of infaunal macrobenthos around vestimentiferan thickets at the San Clemente cold seep, NE Pacific. Mar. Ecol. 31, 608–621. doi: 10.1111/j.1439-0485.2010.00389.x
Bernhard J. M., Buck K. R., Farmer M. A., Bowsers S. S. (2000). The Santa Barbara Basin is a symbiosis oasis. Nature 405, 77–80. doi: 10.1038/47476
Bigham K. T., Leduc D., Rowden A. A., Bowden D. A., Nodder S. D., Orpin A. R. (2024). Recovery of deep-sea meiofauna community in Kaikoura Canyon following an earthquake-triggered turbidity flow. PeerJ 12, 217367. doi: 10.7717/peerj.17367
Blome D., Riemann F. (1987). A sediment accumulation on females of the free-living marine nematode Desmodora schulzi. Helgolander Meeresuntersuchungen 41, 113–119. doi: 10.1007/BF02365102
Bourque J. R., Robertson C. M., Brooke S., Demopoulos A. W. J. (2017). Macrofaunal communities associated with chemosynthetic habitats from the U.S. Atlantic margin: A comparison among depth and habitat types. Deep-Sea Res. II 137, 42–55. doi: 10.1016/j.dsr2.2016.04.012
Bowden D. A., Rowden A. A., Thurber A. R., Baco A. R., Levin L. A., Smith C. R. (2013). Cold seep epifaunal communities on the Hikurangi Margin, New Zealand: Composition, succession, and vulnerability to human activities. PloS One 8, e76869. doi: 10.1371/journal.pone.0076869
Bradford-Grieve J. M., Chang F. H., Gall M., Pickmere S., Richards F. (1997). Size-fractionated phytoplankton standing stocks and primary production during austral winter and spring 1993 in the Subtropical Convergence region near New Zealand. New Z. J. Mar. Freshw. Res. 31, 201–224. doi: 10.1080/00288330.1997.9516759
Campanya-Llovet N., Snelgrove P. V. R. (2018). Fine-scale infaunal community and food web patch mosaic from Barkley methane hydrates (British Columbia, Canada): The role of food quality. Deep-Sea Res. I 140, 186–195. doi: 10.1016/j.dsr.2018.06.009
Charrier B. R., Ingels J., Danielson S. L., Mincks S. L. (2023). Infaunal community structure, diversity, and functions in Pacific-Arctic shelf sediments: a comparison of meiofaunal- and macrofaunal-sized nematodes. Mar. Ecol. Prog. Ser. 720, 95–116. doi: 10.3354/meps14397
Childress J. J., Fisher C. R., Brooks J. M., Kennicutt M. C., Bidigare R., Anderson A. E. (1986). A methanotrophic marine molluscan (Bivalvia, Mytiliidae) symbiosis: mussels fueled by gas. Science 233, 1306. doi: 10.1126/science.233.4770.1306
Clarke K., Gorley R. (2015). PRIMER v7: user manual/tutorial 3rd ed (Plymouth, United Kingdom: Primer-E Ltd).
Clarke K. R., Somerfield P. J., Gorley R. N. (2008). Testing of null hypotheses in explanatory community analyses: similarity profiles and biota-environment linkages. J. Exp. Mar. Biol. Ecol. 366, 56–69. doi: 10.1016/j.jembe.2008.07.009
Clarke K. R., Warwick R. M. (2001). Change in marine communities: an approach to statistical analysis and interpretation. 2nd ed. (Plymouth: PRIMER-E Ltd.).
Cline J. D. (1969). Spectrophotometric determination of hydrogen sulfide in natural waters. Limnology Oceanography 14, 454–458. doi: 10.4319/lo.1969.14.3.0454
Cook A. A., Lambshead P. J. D., Hawkins L. E., Mitchell N., Levin L. A. (2000). Nematode abundance at the oxygen minimum zone in the Arabian Sea. Deep-Sea Res. II 47, 75–85. doi: 10.1016/S0967-0645(99)00097-1
Cordes E. E., Bergquist D. C., Fisher C. R. (2009). Macro-ecology of Gulf of Mexico cold seeps. Ann. Rev. Mari. Sci. 1, 143–168. doi: 10.1146/annurev.marine.010908.163912
Cordes E. E., Jones D. O. B., Schlacher T. A., Amon D. J., Bernardino A. F., Brooke S., et al. (2016). Environmental impacts of the deep-water oil and gas industry: a review to guide management strategies. Front. Environ. Sci. 4. doi: 10.3389/fenvs.2016.00058
Crutchley G. J., Mountjoy J. J., Davy B. W., Hillman J. I. T., Watson S., Stewart L. C., et al. (2018). Gas hydrate systems of the southern Hikurangi Margin, Aotearoa, New Zealand. (Lower Hutt (NZ: GNS Science), 83. GNS Science report 2018/40. doi: 10.21420/73WW-1W83
Crutchley G. J., Mountjoy J. J., Pecher I. A., Gorman A. R., Henrys S. A. (2016). “Submarine slope instabilities coincident with shallow gas hydrate systems: insights from New Zealand examples,” in Submarine mass movements and their consequences: 7th International Symposium, Wellington, New Zealand, 2015 Nov 1–4, 401–409 (Cham (CH): Springer International Publishing). doi: 10.1007/978-3-319-20979-1_40
Dando P. R., Austen M. C., Burke R. A., Kemdall M. A., Kennicutt M. C. II, Judd A. C., et al. (1991). Ecology of a North Sea pockmark with an active methane seep. Mar. Ecol. Prog. Ser. 70, 49–63. doi: 10.3354/meps070049
Dando P. R., Bussmann I., Niven S. J., O’Hara S. C. M., Schmaljohann R., Taylor L. J. (1994). A methane seep area in the Skagerrak, the habitat of the pogonophore Siboglinum pseidoni and the bivalve mollusc Thyasira sarsi. Mar. Ecol. Prog. Ser. 107, 157–167. doi: 10.3354/meps107157
Dando P. R., Hovland M. (1992). Environmental effects of submarine seeping natural gas. Continental Shelf Res. 12, 1197–1207. doi: 10.1016/0278-4343(92)90079-Y
Decker C., Morineaux M., Van Gaver S., Caprais J. C., Lichtschlag A., Gauthier O., et al. (2012). Habitat heterogeneity influences cold-seep macrofaunal communities within and among seeps along the Norwegian margin. Part 1: macrofaunal community structure. Mar. Ecol. 33, 205–230. doi: 10.1111/j.1439-0485.2011.00503.x
De Leo F. C., Smith C. R., Rowden A. A., Bowden D. A., Clark M. R. (2010). Submarine canyons: hotspots of benthic biomass and productivity in the deep sea. Proc. R. Soc. B 1695, 2783–2792. doi: 10.1098/rspb.2010.0462
Demopoulos A. W. J., Bourque J. R., Durkin A., Cordes E. E. (2018). The influence of seep habitats on sediment macrofaunal biodiversity and functional traits. Deep-Sea Res. I 142, 77–93. doi: 10.1016/j.dsr.2018.10.004
Edmunds P. J. (2021). Recruitment hotspots and bottlenecks mediate the distribution of corals on a Caribbean reef. Biol. Lett. 17, 20210149. doi: 10.1098/rsbl.2021.0149
Eleftheriou A., Moore D. C. (2005). “Macrofauna techniques,” in Methods for the study of marine benthos. Eds. Eleftheriou A., McIntyre A. (Blackwell Publishing, Oxford), 160–228. doi: 10.1002/9780470995129.ch5
Ellis J. I., Clark M. R., Rouse H. L., Lamarche G. (2017). Environmental management frameworks for offshore mining: the New Zealand approach. Mar. Policy 84, 178–192. doi: 10.1016/j.marpol.2017.07.004
Etter R. J., Grassle J. F. (1992). Patterns of species diversity in the deep sea as a function of sediment particle size diversity. Nature 360. 576–578. doi: 10.1038/360576a0
Fiala-Medioni A., Boulegue J., Ohta S., Felbeck H., Marriotti A. (1993). Source of energy sustaining the Calyptogena populations from deep trenches in subduction zones off Japan. Deep-Sea Res. I 40, 1241–1258. doi: 10.1016/0967-0637(93)90136-Q
Fu S., Leduc D., Zhao Z. Q. (2019). Two new and one known deep-sea Comesomatidae Filipje species (Nematoda: Araeolaimida) from New Zealand’s continental margin. Mar. Biodiversity 49, 1931–1949. doi: 10.1007/s12526-019-00955-x
Greinert J., Lewis K. B., Bialas J., Pecher I. A., Rowden A. A., Bowden D. A., et al. (2010). Methane seepage along the Hikurangi Margin, New Zealand: Overview of studies in 2006 and 2007 and new evidence from visual, bathymetric and hydroacoustic investigations. Mar. Geology 272, 6–25. doi: 10.1016/j.margeo.2010.01.017
Guilini K., Levin L. A., Vanreusel A. (2012). Cold seep and oxygen minimum zone associated sources of margin heterogeneity affect benthic assemblages, diversity and nutrition at the Cascadian margin (NE Pacific Ocean). Prog. Oceanography 96, 77–92. doi: 10.1016/j.pocean.2011.10.003
Gunton L. M., Bett B. J., Gooday A. J., Glover A. G., Vanreusel A. (2016). Macrofaunal nematodes of the deep Whittard Canyon (NE Atlantic): assemblages characteristics and comparison with polychaetes. Mar. Ecol. 38, e12408. doi: 10.1111/maec.12408
Hasemann C., Mokievsky V., Sablotny B., Tekman M. B., Soltwedel T. (2020). Effects of sediment disturbance on deep-sea nematode communities: Results from an in-situ experiment at the arctic LTER observatory HAUSGARTEN. J. Exp. Mar. Biol. Ecol. 533. doi: 10.1016/j.jembe.2020.151471
Hauquier F., Ingels J., Gutt J., Raes M., Vanreusel A. (2011). Characterisation of the nematode community of a low-activity seep in the recently ice-free shelf Larsen B area, eastern Antarctic Peninsula. PloS One 6, e22240. doi: 10.1371/journal.pone.0022240
Hecker B. (1982). “Possible benthic fauna and slope instability relationships,” in Marine slides and other mass movements. Eds. Saxov S., Nieuwenhuis J. K. (Springer US, Boston (MA), 335–347. doi: 10.1007/978-1-4613-3362-3_20
Hecker B., Paul A. (1979). “Abyssal community structure of the benthic infauna of the Eastern Equatorial Pacific: DOMES sites A, B and C,” in Marine geology and oceanography of the Pacific Manganese nodule province. Eds. Bischoff J., Piper D. (Springer, US), 287–308. doi: 10.1007/978-1-4684-3518-4_8
Heip C., Vincx M., Vranken G. (1985). The ecology of marine nematodes. Oceanography Mar. Biol. Annu. Rev. 23, 399–489.
Hendrick V. J., Hutchison Z. L., Last K. S. (2016). Sediment burial intolerance of marine macroinvertebrates. PloS One 11, 0149114 doi: 10.1371/journal.pone.0149114
Hessler R. R., Jumars P. A. (1974). Abyssal community analysis from replicate box cores in central north Pacific. Deep-Sea Res. 21, 185–209. doi: 10.1016/0011-7471(74)90058-8
Hunter W. R., Jamieson A., Huvenne V. A. I., Witte U. (2013). Sediment community response to marine vs terrigenous organic matter in a submarine canyon. Biogeosciences 10, 67–80. doi: 10.5194/bg-10-67-2013
Jensen P. (1987). Feeding ecology of free-living aquatic nematodes. Mar. Ecol. Prog. Ser. 35, 187–196. doi: 10.3354/meps035187
Jensen P., Aagaard I., Burke R. A., Dando P. R., Jorgensen N. O., Kuijpers A., et al. (1992). [amp]]lsquo;Bubbling reefs’ in the Kattegat: submarine landscapes of carbonate-cemented rocks support a diverse ecosystem at methane seeps. Mar. Ecol. Prog. Ser. 83, 103–112. doi: 10.3354/meps083103
Jin G., Lei H., Xu T., Liu L., Xin X., Zhai. H., et al. (2019). Seafloor subsidence induced by gas recovery from a hydrate-bearing sediment using multiple well system. Mar. Petroleum Geology 107, 438–450. doi: 10.1016/j.marpetgeo.2019.05.008
Jones A. T., Greinert J., Bowden D. A., Klaucke I., Petersen C. J., Netzeband G. L, et al. (2010). Acoustic and visual characterisation of methane-rich seabed seeps at Omakere Ridge on the Hikurangi Margin, New Zealand. Mar. Geology 272, 154–169. doi: 10.1016/j.margeo.2009.03.008
Kaikkonen L., Venesjärvib R., Nygårdc H., Kuikkaa S. (2018). Assessing the impacts of seabed mineral extraction in the deep sea and coastal marine environments: Current methods and recommendations for environmental risk assessment. Mar. pollut. Bull. 135, 1183–1197. doi: 10.1016/j.marpolbul.2018.08.055
Klaucke I., Weinrebe W., Petersen C. J., Bowden D. (2010). Temporal variability of gas seeps offshore New Zealand: Multi-frequency geoacoustic imaging of the Wairarapa area, Hikurangi margin. Mar. Geology 272, 49–58. doi: 10.1016/j.margeo.2009.02.009
Koh D. Y., Kang H., Lee J. W., Park Y., Kim S. J., Lee J., et al. (2016). Energy-efficient natural gas hydrate production using gas exchange. Appl. Energy 162, 114–130. doi: 10.1016/j.apenergy.2015.10.082
Kurihara M., Funatsu K., Ouchi H., Masuda Y., Yasuda M., Yamamoto K., et al. (2008). “Analysis of the JOGMEC/NRCan/Aurora Mallik gas hydrate production test through numerical simulation,” in Proceedings of the 6th International Conference on Gas Hydrates (ICGH 2008), Vancouver, British Columbia, 2008 Jul 6–10.
Law C. S., Collins C., Marriner A., Bury S. J., Brown J., Rickard G. (2024). Dispersion and fate of methane emissions from cold seeps on Hikurangi Margin, New Zealand. Front. Earth Sci. 12. 1354388. doi: 10.3389/feart.2024.1354388
Leduc D. (2012). Deep-sea nematodes (Comesomatidae) from the Southwest Pacific Ocean: five new species and three new species records. Eur. J. Taxonomy 24, 1–12. doi: 10.5852/ejt.2012.24
Leduc D. (2013). Seven new species and one new species record of Sabatieria (Nematoda: Comesomatidae) from the continental slope of New Zealand. Zootaxa 3693, 1–35. doi: 10.11646/zootaxa.3693.1.1
Leduc D. (2023a). Six new species of free-living nematodes (Nematoda: Enoplida) from deep-sea cold seeps on Hikurangi Margin, New Zealand. PeerJ 11, e14867. doi: 10.7717/peerj.14867
Leduc D. (2023b). New nematode species and genera (Nematoda: Chromadorea) from cold seeps on Hikurangi Margin, New Zealand. Eur. J. Taxonomy 856, 1–45. doi: 10.5852/ejt.2023.856.2025
Leduc D., Rowden A. A., Clark M. R., Bowden D. A., Thurber A. R. (2016). Limited differences among habitats in deep-sea macro-infaunal communities off New Zealand: implications for their vulnerability to anthropogenic disturbance. Mar. Ecol. 37, 845–866. doi: 10.1111/maec.12363
Leduc D., Rowden A. A., Nodder S. D., Berkenbusch K., Probert P. K., Hadfield M. G. (2014). Unusually high food availability in Kaikoura canyon linked to distinct deep-sea nematode community. Deep-Sea Res. I 104, 310–318. doi: 10.1016/j.dsr2.2013.06.003
Leduc D., Rowden A. A., Probert P. K., Pilditch C. A., Nodder S. D., Vanreusel A., et al. (2012). Further evidence for the effect of particle-size diversity on deep-sea benthic biodiversity. Deep-Sea Res. I 63. 164–169. doi: 10.1016/j.dsr.2011.10.009
Levin L. A. (2005). Ecology of cold seep sediments: Interactions of fauna with flow, chemistry and microbes. Oceanography Mar. Biology: Annu. Rev. 43, 1–46. doi: 10.1201/9781420037449.ch1
Levin L. A., Mendoza G. F. (2007). Community structure and nutrition of deep methane-seep macrobenthos from the North Pacific (Aleutian) Margin and the Gulf of Mexico (Florida Escarpment). Mar. Ecol. 28, 131–151. doi: 10.1111/j.1439-0485.2006.00131.x
Levin L. A., Mendoza G. F., Gonzalez J. P., Thurber A. R., Cordes E. E. (2010). Diversity of bathyal macrofauna on the northeastern Pacific margin: the influence of methane seeps and oxygen minimum zones. Mar. Ecol. 31, 94–110. doi: 10.1111/j.1439-0485.2009.00335.x
Levin L. A., Michener R. (2002). Isotopic evidence of chemosynthesis-based nutrition of macrobenthos: the lightness of being at Pacific methane seeps. Limnology Oceanography 47, 1336–1345. doi: 10.4319/lo.2002.47.5.1336
Levin L. A., Ziebis W., Mendoza G., Growney V., Tryon M., Brown K., et al. (2003). Spatial heterogeneity of macrofauna at northern California methane seeps: the influence of sulfide concentration and fluid flow. Mar. Ecol. Prog. Ser. 265, 123–139. doi: 10.3354/meps265123
Lin T.-K., Hsieh B.-Z. (2020). Prevention of seabed subsidence of Class-1 gas hydrate deposits via CO2-EGR: a numerical study with coupled geomechanics-hydrate reaction-multiphase fluid flow model. Energies. 13, 1579. doi: 10.3390/en13071579
Luth C., Luth U., Gebruk A. V., Thiel H. (1999). Methane gas seepage along the oxic/anoxic gradients in the Black Sea: Manifestations, biogenic sediment compounds and preliminary results on benthic ecology. Mar. Ecol. 20, 221–249. doi: 10.1046/j.1439-0485.1999.t01-1-00073.x
Macheriotou L., Rigaux A., Olu K., Zeppilli D., Derycke S., Vanreusel A. (2021). Deep-sea nematodes of the Mozambique Channel: Evidence of random community assembly dynamics in seep sediments. Front. Mar. Sci. 8. doi: 10.3389/fmars.2021.549834
Magen C., Lapham L. L., Pohlman J. W., Marshall K., Bosman S., Casso M., et al. (2014). A simple headspace equilibration method for measuring dissolved methane. Limnology Oceanography Methods 12, 637–650. doi: 10.4319/lom.2014.12.637
Menot L., Galeron J., Olu K., Caprais J. C., Crassous P., Khripounoff A., et al. (2010). Spatial heterogeneity of macrofaunal communities in and near a giant pockmark area in the deep Gulf of Guinea. Mar. Ecol. 31, 78–93. doi: 10.1111/j.1439-0485.2009.00340.x
Merey Ş., Longinos S. N. (2018). Numerical simulations of gas production from Class 1 hydrate and Class 3 hydrate in the Nile Delta of the Mediterranean Sea. J. Natural Gas Sci. Eng. 52, 248–266. doi: 10.1016/j.jngse.2018.01.001
Miljutin D. M., Miljutina M. A. (2016). Review of Acantholaimus Allgé(Nematoda: Chromadoridae), a genus of marine free-living nematodes, with a tabular key to species. Nematology 18, 537–558. doi: 10.1163/15685411-00002976
Moens T., Vincx M. (1997). Observations on the feeding ecology of estuarine nematodes. J. Mar. Biol. Assoc. United Kingdom 77, 211–227. doi: 10.1017/S0025315400033889
Moridis G. J., Reagan M. T. (2007). “Strategies for gas production from oceanic Class 3 hydrate accumulations,” in Offshore Technology Conference, Houston, Texas, 2007 Apr 30 – May 3. OTC–18865-MS. doi: 10.4043/18865-MS
Murphy R. J., Pinkerton M. H., Richardson K. M., Bradford-Grieve J. M., Boyd P. W. (2001). Phytoplankton distributions around New Zealand derived from SeaWiFS remotely-sensed ocean colour data. New Z. J. Mar. Freshw. Res. 35, 343–362. doi: 10.1080/00288330.2001.9517005
Muthumbi A. W., Vanreusel A., Duineveld G., Soetaert K., Vincx M. (2004). Nematode community structure along the continental slope off the Kenyan Coast, Western Indian Ocean. Int. Rev. Hydrobiology 89, 188–205. doi: 10.1002/iroh.200310689
Nascimento F. J. A., Naslund J., Elmgren R. (2012). Meiofauna enhances organic matter mineralization in soft sediment ecosystems. Limnology Oceanography 57, 338–346. doi: 10.4319/lo.2012.57.1.0338
Nicholas W. L., Goodchild D. J., Stewart A. (1987). The mineral composition of intracellular includions in nematodes from thiobiotic mangrove mudflats. Nematologica 33, 167–179. doi: 10.1163/187529287X00308
Oluwunmi P. A., Finney A. R., Rodger P. M. (2015). Molecular dynamics screening for new kinetic inhibitors of methane hydrate. Can. J. Chem. 93, 1043–1049. doi: 10.1139/cjc-2015-0003
Oluwunmi P. A., Reagan M. T., Pecher I. A., Archer R. A., Moridis G. J., Rowden A. A., et al. (2023). Possible influx of seawater during gas production from unsealed gas hydrate reservoirs has economic and environmental implications. Energy Fuels 37, 18843–18854. doi: 10.1021/acs.energyfuels.3c02963
Pape E., Bezerra T. N., Vanneste H., Heeschen K., Moodley L., Leroux F., et al. (2011). Community structure and feeding preference of nematodes associated with methane seepage at the Darwin mud volcano (Gulf of Cadiz). Mar. Ecol. Prog. Ser. 438, 71–83. doi: 10.3354/meps09278
Paull C. K., Hecker B., Commeau R., Freeman-Lynde R. P., Neumann C., Corso W. P., et al. (1984). Biological communities at the Florida Escarpment resemble hydrothermal vent taxa. Science 226, 965–967. doi: 10.1126/science.226.4677.965
Paull C. K., Jull A. J. T., Tolin L. J., Linick T. (1985). Stable isotope evidence for chemoautotrophy in an abyssal seep community. Nature 317, 709–711. doi: 10.1038/317709a0
Pavithran S., Ingole B. S., Nanajkar M., Raghukumar C., Nath B. N., Valsangkar A. B. (2009). Composition of macrobenthos from the Central Indian Ocean basin. J. Earth System Sci. 118, 689–700. doi: 10.1007/s12040-009-0051-4
Pecher I., Crutchley G., Kröger K. F., Hillman J., Mountjoy J., Coffin R., et al. (2022). “New Zealand’s gas hydrate systems,” in World atlas of submarine gas hydrates incontinental margins. Eds. Mienert J., Berndt C., Tréhu A. M., Camerlenghi A., Liu C.-S. (Springer International Publishing, Cham (CH), 415–424. doi: 10.1007/978-3-030-81186-0_35
Pecher I. A., Henrys S. A. (2003). “Potential gas reserves in gas hydrate sweet spots on the Hikurangi Margin,” in (Institute of Geological & Nuclear Sciences science report; 2003/23) (Institute of Geological & Nuclear Sciences, New Zealand. Lower Hutt (NZ), 32.
Portail M., Olu K., Escobar-Briones E., Caprais J. C., Menot L., Waeles M., et al. (2015). Comparative study of vent and seep macrofaunal communities in the Guaymas Bason. Biogeosciences 12, 5455–5479. doi: 10.5194/bg-12-5455-2015
Portnova D., Mokievsky V., Soltwedel T. (2011). Nematode species distribution patterns at the Hakon Mosby Mud Volcano (Norwegian Sea). Mar. Ecol. 32, 24–41. doi: 10.1111/j.1439-0485.2010.00403.x
Ritt B., Desbruyeres D., Caprais J. C., Gauthier O., Ruffine L., Buscail R., et al. (2012). Seep communities from two mud volcanoes in the deep Mediterranean Sea: faunal composition, spatial patterns and environmental control. Mar. Ecol. Prog. Ser. 466, 93–119. doi: 10.3354/meps09896
Robinson C. A., Bernhard J. M., Levin L. A., Mendoza G. F., Blanks J. K. (2004). Surficial hydrocarbon seep infauna from the Blake Ridge (Atlantic Oceam) and the Gulf of Mexico (690-2240 m). Mar. Ecol. 25, 313–336. doi: 10.1111/j.1439-0485.2004.00034.x
Rosli N., Leduc D., Rowden A. A., Clark M. R., Probert P. K., Berkenbusch K., et al. (2016). Differences in meiofauna communities with sediment depth are greater than habitat effects on the New Zealand continental margin: implications for vulnerability to anthropogenic disturbance. PeerJ 4, e2154. doi: 10.7717/peerj.2154
Rosli N., Leduc D., Rowden A. A., Probert P. K., Clark M. R. (2018). Regional and sediment depth differences in nematode community structure greater than between habitats on the New Zealand margin: Implications for vulnerability to anthropogenic disturbance. Prog. Oceanography 160, 26–52. doi: 10.1016/j.pocean.2017.11.006
Sahling H., Galkin S. V., Salyuk A., Greinert J., Foerstel H., Piepenburg D., et al. (2003). Depth-related structure and ecological significance of cold seep communities: a case study from the Sea of Okhotsk. Deep-Sea Res. I 50, 1391–1409. doi: 10.1016/j.dsr.2003.08.004
Sahling H., Rickert D., Lee R. W., Linke P., Suess E. (2002). Macrofaunal community structure and sulfide flux and gas hydrate deposits from the Cascadia convergent margin. Mar. Ecol. Prog. Ser. 231, 121–138. doi: 10.3354/meps231121
Schmidt-Rhaesa A. (2014). z Vol. Volume 2 (Gastrotricha, Cycloneuralia, 542 Gnathifera: Nematoda. De Gruyter), 759.
Schratzberger M., Rees H. L., Boyd S. E. (2000). Effects of simulated deposition of dredged material on structure of nematode assemblages: the role of burial. Mar. Biol. 136, 519–530. doi: 10.1007/s002270050712
Schwing P. T., Montagna P. A., Joye S. B., Paris C. B., Cordes E. E., McClain C. R., et al. (2020). A synthesis of deep benthic faunal impacts and resilience following the deepwater horizon oil spill. Front. Mar. Sci. 7. doi: 10.3389/fmars.2020.560012
Seabrook S., Bumberger T., Butterfield D., Roe K., Cummings M., Crawford R., et al. (2024). Ubiquitous but unique: Water depth and oceanographic attributes shape methane seep communities. Limnology Oceanography 69, 1218–1232. doi: 10.1002/lno.12564
Sharma J., Baguley J., Bluhm B. A., Rowe G. (2011). Do meio- and microbenthic nematodes differ in community composition and body weight trends with depth? PloS One 6, e14491. doi: 10.1371/journal.pone.0014491
Sharma J., Bluhm B. A. (2011). Diversity of larger free-living nematodes from macrobenthos (>250 μm) in the Arctic deep-sea Canada Basin. Mar. Biodivers. 41, 455-465. doi: 10.1007/s12526-010-0060-1
Shirayama Y., Ohta S. (1990). Meiofauna in cold-seep community off Hatsushima, Central Japan. J. Oceanographical Soc. Japan 46, 118–124. doi: 10.1007/BF02123438
Soetaert K., Heip C. (1995). Nematode assemblages of deep-sea and shelf break sites in the North Atlantic and Mediterranean Sea. Mar. Ecol. Prog. Ser. 125, 171–183. doi: 10.3354/meps125171
Soltwedel T., Guilini K., Sauter E., Schewe I., Hasemann C. (2018). Local effects of large food-falls on nematode diversity at an arctic deep-sea site: Results from an in situ experiment at the deep-sea observatory HAUSGARTEN. J. Exp. Mar. Biol. Ecol. 502, 129–141. doi: 10.1016/j.jembe.2017.03.002
Somerfield P. J., Warwick R. M. (1996). Meiofauna in marine pollution monitoring programmes: a laboratory manual. Ministry of Agriculture, Fisheries, and Food, Lowestoft.
Spies R., Des Marais D. (1983). Natural isotope study of trophic enrichment of marine benthic communities by petroleum seepage. Mar. Biol. 73, 67–71. doi: 10.1007/BF00396286
Steichen D. J., Holbrook S. J., Osenberg C. W. (1996). Distribution and abundance of benthic and demersal macrofauna within a natural hydrocarbon seep. Mar. Ecol. Prog. Ser. 138, 71–82. doi: 10.3354/meps138071
Thiermann F., Windoffer R., Giere O. (1994). Selected meiofauna around shallow water hydrothermal vents off Milos (Greece): ecological and ultrastructural aspects. Vie Milieu 44, 215–226.
Thiermann F., Vismann B., Giere O. (2000). Sulphide tolerance of the marine nematode Oncholaimus campylocercoides: A result of internal sulphur formation? Mar. Ecol. Prog. Ser. 193, 251–259. doi: 10.3354/meps193251
Thurber A. R., Kroger K., Neira C., Wiklund H., Levin L. A. (2010). Stable isotope signatures and methane use by New Zealand cold seep benthos. Mar. Geology 272, 260.269. doi: 10.1016/j.margeo.2009.06.001
Thurber A. R., Levin L. A., Rowden A. A., Sommer S., Linke P., Kroger K. (2013). Microbes, macrofauna, and methane: A novel seep community fueled by aerobic methanotrophy. Limnology Oceanography 58, 1640–1656. doi: 10.4319/lo.2013.58.5.1640
Turco F., Ladroit Y., Watson S. J., Seabrook S., Law C. S., Crutchley G. J., et al. (2022). Estimates of methane release from gas seeps at the southern Hikurangi Margin, New Zealand. Front. Earth Sci. 10. doi: 10.3389/feart.2022.834047
Van Gaever S., Galeron J., Sibuet M., Vanreusel A. (2009a). Seep-sea habitat heterogeneity influence on meiofaunal communities in the Gulf of Guinea. Deep-Sea Res. II 56, 2259–2269. doi: 10.1016/j.dsr2.2009.04.008
Van Gaever S., Moodley L., Pasotti F., Houtekamer M., Middelburg J. J., Danovaro R., et al. (2009b). Trophic specialisation of metazoan meiofauna at the Hakon Mosby Mud Volcano: fatty acid biomarker isotope evidence. Mar. Biol. 156, 1289–1296. doi: 10.1007/s00227-009-1170-9
Van Gaever S., Moodley L., de Beer D., Vanreusel A. (2006). Meiobenthos at the Arctic Hakon Mosby Mud Volcano, with a parental-caring nematode thriving in sulphide-rich sediments. Mar. Ecol. Prog. Ser. 321, 143–155. doi: 10.3354/meps321143
Vanreusel A. (1991). Ecology of free-living marine nematodes in the Voordelta (Southern Bight of the North Sea). II. Habitat preferences dominant Species. Nematologica 37, 343–359. doi: 10.1163/187529291X00349
Vanreusel A., De Groote A., Gollner S., Bright M. (2010b). Ecology and Biogeography of free-living nematodes associated with chemosynthetic environments in the deep sea: A Review. PloS One 5, e12449. doi: 10.1371/journal.pone.0012449
Vanreusel A., Fonseca G., Danovaro R., da Silva M. C., Esteves A. M., Ferrero T., et al. (2010a). The contribution of deep-sea macrohabitat heterogeneity to global nematode diversity. Mar. Ecol. 31, 6–20. doi: 10.1111/j.1439-0485.2009.00352.x
Vincx M., Meire P., Heip C. (1990). The distribution of nematodes communities in the Southern Bight of the North Sea. Cahiers Biologie Mar. 31, 107–129.
Vitiello P. (1971). Nématodes libres marins des vases profondes du Golfe de Lion III. Monhysterida Araeolaimida Desmodorida. Thetys 2, 647–690.
Warwick R. M., Platt H. M., Somerfield P. J. (1998). Free living marine nematodes. Part III. Monhysterids 53 296.(Cambridge University Press, Synopses of the Brithsh Fauna (New Series)Cambridge).
Washburn T. W., Demopoulos A. W. J., Montagna P. A. (2018). Macrobenthic infaunal communities associated with deep-sea hydrocarbon seeps in the northern Gulf of Mexico. Mar. Ecol. 39, e12508. doi: 10.1111/maec.12508
Watson S. J., Mountjoy J. J., Barnes P. M., Crutchley G. J., Lamarche G., Higgs B., et al. (2020). Focused fluid seepage related to variations in accretionary wedge structure, Hikurangi margin, New Zealand. Geology 48, 56–61. doi: 10.1130/G46666.1
Wieser W. (1953). Die Beziehung zwischen Mundho«hlengestalt, Erna«hrungsweise und Vorkommen bei freilebenden marinen Nematoden. Arkiv fur Zoologie 2, 439–484.
Zeppilli D., Leduc D., Fontanier C., Fontaneto D., Fuchs S., Gooday A. J., et al. (2018). Characteristics of meiofauna in extreme marine ecosystems: a review. Mar. Biodiversity 48, 35–71. doi: 10.1007/s12526-017-0815-z
Zeppilli D., Mea M., Corinaldesi C., Danovaro R. (2011). Mud volcanoes in the Mediterranean Sea are hot spots of exclusive meiobenthic species. Prog. Oceanography 91, 260–272. doi: 10.1016/j.pocean.2011.01.001
Zhang J., Moridis G. J., Blasingame T. A. (2023). Effect of geomechanics and of grid discretization on the predictions of production from natural hydrate deposits and of the associated geomechanical system response. Gas Sci. Eng. 112, 204942. doi: 10.1016/j.jgsce.2023.204942
Keywords: macrofauna, infauna, community ecology, continental slope, nematode species
Citation: Leduc D, Rowden AA, Seabrook S, Bowden DA, Thurber AR, Halliday J, Law CS, Pereira OS, Whitten BG and Marriner A (2025) Distribution and environmental drivers of macrofaunal nematode communities across gradients of methane seepage at cold seeps on Hikurangi Margin (New Zealand) and potential implications of disturbance from gas hydrate extraction. Front. Mar. Sci. 11:1486305. doi: 10.3389/fmars.2024.1486305
Received: 25 August 2024; Accepted: 03 December 2024;
Published: 15 January 2025.
Edited by:
Maria Cristina Gambi, National Institute of Oceanography and Applied Geophysics, ItalyReviewed by:
Maickel Armenteros, National Autonomous University of Mexico, MexicoDaniel Pech, El Colegio de la Frontera Sur, Mexico
Copyright © 2025 Leduc, Rowden, Seabrook, Bowden, Thurber, Halliday, Law, Pereira, Whitten and Marriner. This is an open-access article distributed under the terms of the Creative Commons Attribution License (CC BY). The use, distribution or reproduction in other forums is permitted, provided the original author(s) and the copyright owner(s) are credited and that the original publication in this journal is cited, in accordance with accepted academic practice. No use, distribution or reproduction is permitted which does not comply with these terms.
*Correspondence: Daniel Leduc, RGFuaWVsLkxlZHVjQG5pd2EuY28ubno=