- 1Scottish Association for Marine Science, Argyll, Oban, United Kingdom
- 2Culture Collection of Algae and Protozoa (CCAP), Argyll, Oban, United Kingdom
- 3Shell Technology Center, Shell Exploration and Production Inc., Houston, TX, United States
Seaweed aquaculture is gaining traction globally as a solution to many climate issues. However, seaweeds themselves are also under threat of anthropogenically driven climate change. Here, we summarize climate-related challenges to the seaweed aquaculture industry, with a focus on the developing trade in the North Atlantic. Specifically, we summarize three main challenges: i) abiotic change; ii) extreme events; and iii) disease & herbivory. Abiotic change includes negative effects of ocean warming and acidification, as well as altered seasonality due to ocean warming. This can lower biomass yield and change biochemical composition of the seaweeds. Extreme events can cause considerable damage and loss to seaweed farms, particularly due to marine heatwaves, storms and freshwater inputs. Seaweed diseases have a higher chance of proliferating under environmentally stressful conditions such as ocean warming and decreased salinity. Herbivory causes loss of biomass but is not well researched in relation to seaweed aquaculture in the North Atlantic. Despite challenges, opportunities exist to improve resilience to climate change, summarized in three sections: i) future proof site selection; ii) advances in breeding and microbiome manipulation; and iii) restorative aquaculture. We present a case study where we use predictive modelling to illustrate suitable habitat for seaweed cultivation in the North Atlantic under future ocean warming. Notably, there was a large loss of suitable habitat for cultivating Alaria esculenta and Laminaria digitata. We show how selection and priming and microbe inoculates may be a cost-effective and scalable solution to improve disease- and thermal tolerance. Co-cultivation of seaweeds may increase both yield and biodiversity co-benefits. Finally, we show that aquaculture and restoration can benefit from collaborating on nursery techniques and push for improved legislation.
1 Introduction
Climate change is putting increased pressure on food production, creating a rising demand for sustainable aquaculture solutions (Subasinghe et al., 2009). Among the different avenues of aquaculture, seaweeds are a promising candidate. Seaweeds are capable of growing without the addition of nutrients or fertilizers and can be used in a multitude of downstream applications (Buschmann et al., 2017). In addition, seaweeds have great potential to increase sustainability of mariculture projects, such as in restorative aquaculture or integrated multitrophic aquaculture (IMTA; Duarte et al., 2022). Currently, almost all (97%) commercially grown seaweeds come from Asia, with China, Indonesia, and South Korea being the top three global producers (Sultana et al., 2023; Khan et al., 2024). However, the seaweed cultivation industry is growing in other parts of the world, including the North Atlantic (Figure 1). In 2024, a total of 1,240.86 KT wet weight of seaweed was produced annually by 139 companies, with the main species for cultivation being Saccharina latissima, Ulva sp. and Alaria esculenta (Figure 1, data sourced from www.phyconomy.net).
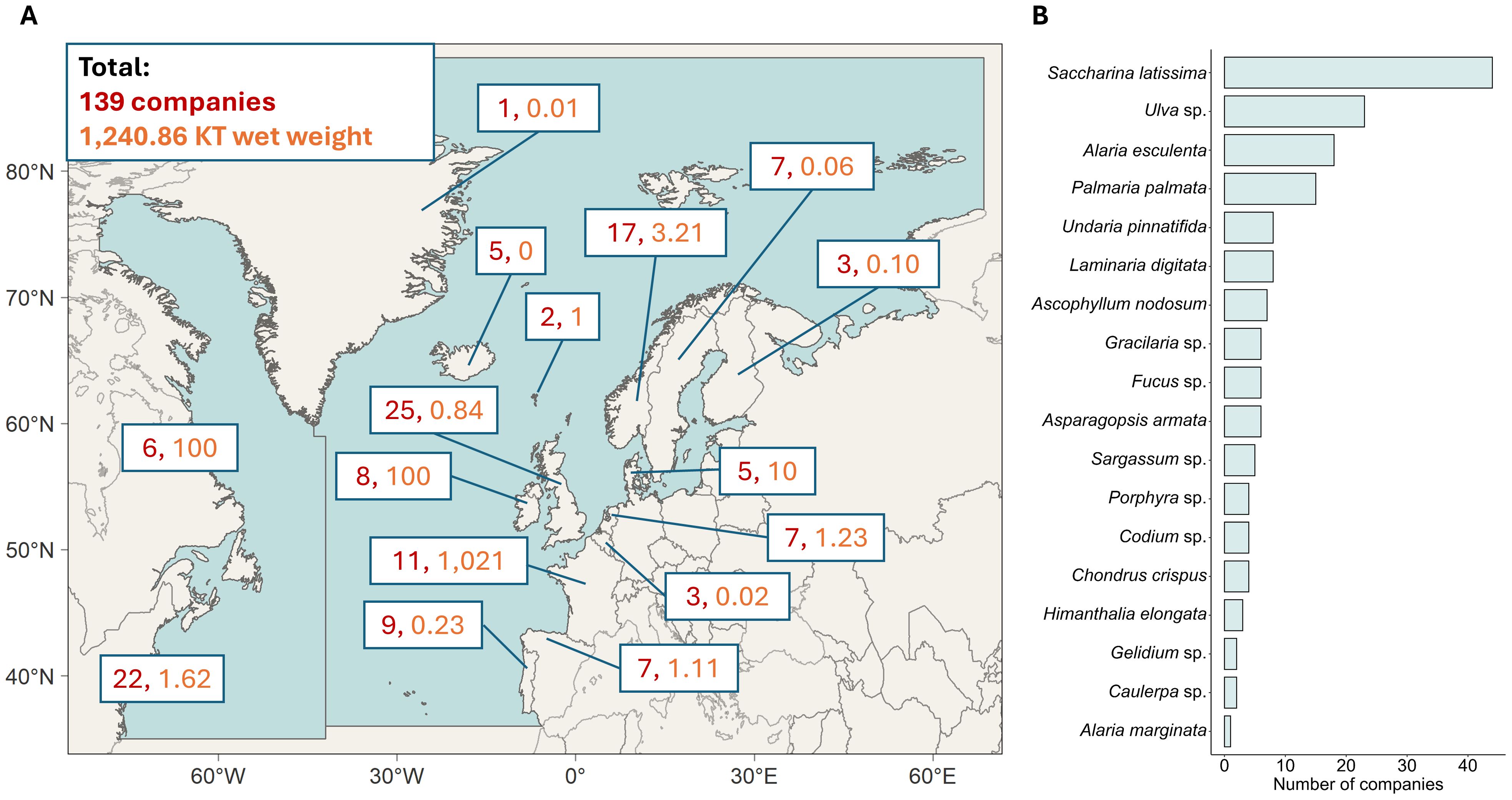
Figure 1. Production numbers and main species of seaweed cultivated in the North Atlantic seaweed industry. Data were taken from www.phyconomy.net in August 2024 and collated for visual presentation. Companies were only included if active seaweed growing took place in the North Atlantic (i.e. excluding any Pacific companies from Canada and the USA, and companies only practicing wild harvesting. Onshore cultivation and companies practicing both wild-harvesting and cultivation were included). (A) Map of the North Atlantic defined as area 21 and 27 of the FAO Major Fishing Areas, depicted by the two blue areas. In red is the number of seaweed cultivation companies per country, as well as the metric KT (kilo ton) of wet weight produced annually. (B) Main species grown in the North Atlantic seaweed industry, ordered by number of companies cultivating these species.
For seaweed aquaculture to expand in the North Atlantic, there are still many challenges that need to be addressed. Despite recognition as an emerging industry, there is a significant lack of seaweed policy and regulation in countries bordering the North Atlantic (Campbell et al., 2020; Naylor et al., 2021). Largely, seaweed policy is based on existing shellfish regulation, with some nations, such as Scotland and Norway, having started to develop independent seaweed aquaculture policies (Alexander et al., 2015; Wood et al., 2017). Seaweed cultivation requires large spatial areas for operations to be economically feasible, which is largely due to current market values for seaweeds and the costs associated with small-scale farming (Hughes and Black, 2016). Available space in the marine environment is highly contested, with potential solutions for avoiding conflict including the combination of seaweed with other aquaculture or marine-related industries, or offshore operations (Hughes and Black, 2016; Kim et al., 2017; Duarte et al., 2017). In addition, social licensing to build large scale seaweed farms in the North Atlantic may not yet be on par with other parts of the world (Billing et al., 2021), and the nursery phase of seaweed farming is still labor intensive and therefore costly.
Next to the economic, social, environmental and policy challenges of seaweed aquaculture (reviewed in for example Alexander et al., 2015; Campbell et al., 2019; Kerrison et al., 2015; Wood et al., 2017; Visch et al., 2023), there are concerns regarding seaweed aquaculture viability in relation to climate change threats. Climate stressors have long been recognized to affect natural seaweed habitats (e.g. Harley et al., 2012; Wernberg et al., 2023; Steneck et al., 2002; Schiel et al., 2004). At the same time, the commercial growth of seaweeds has been suggested as a solution to certain climate stressors, through carbon capture, pH buffering, waste-water remediation and offering a low-carbon alternative to certain products (reviewed in for example Yong et al., 2022; Sultana et al., 2023; Ross et al., 2023; Duarte et al., 2022). However, climate change stress will also have a profound effect on the seaweed growing industry (Chung et al., 2017). Climate change effects on the seaweed aquaculture industry have been reviewed for specific locations such as California (Kübler et al., 2021), the UK and Ireland (Callaway et al., 2012), the Gulf of Maine (Bricknell et al., 2021), Korea (Kim et al., 2019) and Norway (Stévant et al., 2017), as well as focusing on specific species such as tropical red seaweeds (Largo et al., 2017). This review focuses on the seaweed aquaculture species relevant to the North Atlantic (Figure 1). The species cultivated span across all three domains of seaweeds, therefore responses to climate change will differ. We summarize the main climate change challenges facing the seaweed aquaculture industry. At the same time, we address emerging opportunities and potential solutions to some of the challenges. As the focus is on the North Atlantic, most species discussed are of a temperate distribution. However, where relevant, examples might be provided from tropical seaweed aquaculture, particularly if the research is innovative and paves the way for technological improvements from which the temperate aquaculture industry may learn.
2 Challenges
Climate change is driving profound changes in seaweed ecosystems globally (Wernberg et al., 2023; Filbee-Dexter and Wernberg, 2018; Smale, 2020). Anthropogenic CO2 has been steadily rising since the start of the industrial era, which has driven multiple changes in the environment affecting marine ecosystems (Allen et al., 2009). The acceleration of carbon emissions has caused global atmospheric temperatures to rise, and a large proportion of that temperature increase is absorbed by the ocean, causing ocean temperatures to increase (Reichert et al., 2002; Bronselaer and Zanna, 2020; Goodwin et al., 2015). Ocean systems are also becoming more acidic due to increased absorption of atmospheric CO2 (Doney et al., 2009; Iida et al., 2021; Ma et al., 2023a). Through increased temperatures, weather patterns are shifting globally resulting in increased rainfall, which in turn can change salinity levels in coastal areas (Marsooli et al., 2019). Extreme events such as storms and marine heatwaves are also increasing in frequency and intensity (Coumou and Rahmstorf, 2012; Smale et al., 2019). The direct effects of ocean warming have other indirect consequences upon seaweed ecology and aquaculture, such as altered herbivory rates, species range shifts and the prevalence of disease (Vergés et al., 2014; Krumhansl et al., 2016; Gachon et al., 2010). All these ocean change factors represent risk and may influence seaweed aquaculture endeavors. The challenges they present are discussed here.
2.1 Challenge 1: abiotic change
2.1.1 Ocean warming
Increasing ocean temperatures have been identified as a major challenge to seaweed aquaculture industries, though it also may increase areas available for aquaculture (Largo et al., 2017; Chung et al., 2017). Global climate change is predicted to increase sea surface temperatures from an average 1.5°C to 3.5°C under low- and high-emission CMIP6 models, respectively (Kwiatkowski et al., 2020; IPCC, 2023). Since the latitudinal distribution of seaweed is largely constrained by temperature (Smale, 2020; Jayathilake and Costello, 2020), this could have a profound impact upon ecosystem ecology, as well as existing and future seaweed and IMTA enterprises (Chung et al., 2017). Temperature stress has effects on both the individual level, altering a seaweed’s morphology and biochemical profile (Eggert, 2012), and population level, including range shifts, genetic shifts, and decreased productivity of the whole ecosystem (Harley et al., 2012; Wernberg et al., 2023; Coleman et al., 2020a). However, how this may impact seaweed aquaculture remains uncertain and requires more detailed study and models to predict impacts.
Seaweeds have a multitude of mechanisms to acclimate, protect, or repair in response to temperature stress, among them adjusting cell membrane fluidity and production of a suite of enzymes to protect against intracellular reactive oxygen species (ROS) which may be formed in response to temperature stress (e.g. Eggert, 2012; Choo et al., 2004; Britton et al., 2020; Hammann et al., 2016) However, their upregulation will come at a metabolic cost. which reduces growth and net primary productivity (NPP) as temperature increases (Harley et al., 2012; Eggert, 2012). This has been observed in the northeast Atlantic, where net primary productivity (NPP) and biomass standing stock of Laminaria hyperborea was respectively 1.5 and 2.5 times greater in northern sites compared to the southernmost sites in the UK, across a temperature gradient of ~2.5°C (Smale et al., 2020). Overall trends show that NPP of seaweed systems is highest in temperate regions, where ocean temperatures are between 10-18°C (Pessarrodona et al., 2022). This indicates that ocean warming may shift areas of greatest NPP from temperate to arctic regions, which currently represent lower NPP rates. For higher NPP in seaweed farming, farms may thus be best placed in cooler thermal regions, or use species that have a higher NPP under increased temperatures. For example, the pseudo-kelp Saccorhiza polyschides has a higher mean NPP and a larger capacity to respond to thermal stress compared to Laminaria ochroleuca, in part due to its annual life cycle (Biskup et al., 2014). However, this does not take into account that NPP can become temperature acclimated (Davison et al., 1991; Kübler and Davison, 1995), or that photosynthetic rates may be adapted to local temperatures (King et al., 2020; Smolina et al., 2016). In addition, NPP can be influenced by other abiotic factors, e.g. light regimes or CO2, and biotic factors, e.g. life history stage or tissue type, which adds extra caution to extrapolating NPP measurements from limited data points (Franke et al., 2023; Veenhof et al., 2024).
Increased temperature can also alter the biochemical composition of seaweeds. As a majority of seaweeds are processed downstream for their primary and secondary metabolites (Buschmann et al., 2017), this can have a large impact on marketable products for the seaweed aquaculture industry. The effects of climate change on seaweed metabolites have recently been reviewed, and we refer to this body of work for further reading (Park et al., 2023). Briefly, the composition of carbohydrates, amino acids, and other metabolites can change under temperature stress potentially altering the overall nutritional composition of the seaweed (Park et al., 2023), which will likely have knock-on effects for human consumption (Shalders et al., 2022). Mixed reports show both no effect of temperature stress on the nutritional quality of seaweeds (Ecklonia radiata and Sargassum sp.; Shalders et al., 2023) or a decrease in nutritional quality with increased temperatures (Macrocystis pyrifera, Derbesia tenuissima; Lowman et al., 2022; Gosch et al., 2015), which could be due to separate stress tolerances between species. Research should address the changes in nutritional quality of seaweeds commonly grown for commercial aquaculture. This is particularly important as consistent biochemical composition of the seaweeds is key for delivering products to end-users such as the food- and feed industry (Park et al., 2023).
Range shifts of seaweeds induced by warming may change the species composition of natural seaweed populations, which may affect the total nutritional value of those seaweed environments (Shalders et al., 2023). Similarly, in the context of seaweed aquaculture, the species that are viable for culture and their nutritional content at one specific site may change with ocean warming. Or, the time of harvest may be shortened to earlier in the season from current operational farms. As such, projections of seaweed species’ distributions in a future ocean are crucial for marking locations suitable for seaweed aquaculture. Climate change has already resulted in the range shift of commercially important populations of seaweed. For instance, in North America, there has been a decline in S. latissima and Laminaria digitata populations on the southern range edge and in warming hotspots (Feehan et al., 2019; Filbee-Dexter et al., 2016). In Europe, shifts in seaweed distributions have also been reported, including poleward shifts in the cold-water species S. latissima and A. esculenta from Northern Europe (Moy and Christie, 2012; Simkanin et al., 2005). Warmer water affiliated L. ochroleuca and L. hyperborea have been reported to decline in Southern Europe (Piñeiro-Corbeira et al., 2018; Casado-Amezúa et al., 2019), but are expanding into Northern Europe (Schoenrock et al., 2019; Rinde et al., 2014). These examples indicate that site-specific consideration should be given to which species are currently suitable for aquaculture, and which species might offer more appropriate candidates for cultivation under future warming scenarios and predicted species range shifts.
2.1.2 Ocean acidification
An increase in atmospheric CO2 leads not only to ocean warming, but also to ocean acidification (OA). Rising atmospheric CO2 levels are tempered by oceanic uptake, removing approximately one third of all anthropogenic released carbon (Iida et al., 2021). Yet this uptake causes a shift in ocean carbonate chemistry (Doney et al., 2009; Kwiatkowski et al., 2020). As CO2 is absorbed by the oceans, it reacts with seawater to create carbonic acid, causing pH levels to decrease and thus making seawater overall more acidic (Raven et al., 2005). On average, anthropogenic emissions of greenhouse gases have caused pH to decrease in ocean surface seawater by around 0.1 since the beginning of the industrial era (Iida et al., 2021). Future estimates predict that oceanic pH could drop by another 0.2 - 0.3 units by the end of this century (IPCC, 2023). Coastal seas, where most seaweed aquaculture currently takes place, are more at risk of acidification than the open ocean. This is due to the multiple sources of CO2 and acidic sources that coastal seas are exposed to (such as river inputs, discharge, erosion runoff, etc.), compared with the well-buffered open ocean that is only significantly affected by atmospheric CO2 (Chan et al., 2017; Clements and Chopin, 2017).
Seaweeds are predicted to have a mixed response to OA as concentrations of dissolved CO2 increase (Roleda and Hurd, 2012). Calcifying seaweeds are expected to have a negative response toward acidification, yet few studies have examined the response of non-calcifying seaweeds dominating the aquaculture trade (Buschmann et al., 2017; Kim et al., 2017). For non-calcifying seaweeds, it is hypothesized that increased acidification may either have a neutral or a beneficial effect, especially if the seaweed does not use carbon concentrating mechanisms (CCM) as an active carbon uptake strategy (Kübler and Dudgeon, 2015). Britton et al. (2019) studied the effect of diel fluctuating pH levels (representative of coastal environments) on two seaweed species without CCMs. Effects were species-specific, where diel pH fluctuation reduced photosynthesis in the red seaweed Callophyllis lambertii, but increased OA benefited physiological rates. Conversely, another rhodophyte, Plocamium dilatum, showed no effects of pH fluctuations or OA. Other studies support the findings of species-specific responses to OA (Paine et al., 2023; Ho et al., 2021; Taise et al., 2023; van der Loos et al., 2019b) suggesting that its effects are not just dependent on the method of carbon acquisition, but also species-specific enzyme activity and natural pH fluctuations (Britton et al., 2019; van der Loos et al., 2019b).
In addition, some degree of pH fluctuation happens naturally in many seaweed environments, caused by carbon cycling of the seaweeds. Dissolved inorganic carbon is taken up during the day, increasing the surrounding water pH, and decreases pH during the night as they release CO2 through respiration (Noisette et al., 2022). Organisms (including seaweeds themselves) which reside in these diel cycle systems are subjected to highly variable pH and CO2 concentrations that can be of a similar or larger magnitude to the near-future changes expected to occur due to OA (Frieder et al., 2012; Krause-Jensen et al., 2015). As such, these species may be less susceptible and more resilient toward ocean acidification.
2.1.3 Altered seasonality
Both growth and biochemical composition of seaweed species vary temporally. Growth is often determined according to season, such that it is mostly related to available daylight hours independent of other abiotic factors (Lüning, 1994, 1993). On the other hand, processes such as nutrient accumulation in seaweeds may be influenced by temperature, the type and concentration of water nutrient and other abiotic factors that vary seasonally (Rioux et al., 2009; Suresh Kumar et al., 2015). As such, ocean change may cause a mismatch between the optimal environmental conditions for biomass acquisition and intended biochemical composition at harvest time. For example, in cold-water species, such as kelps and fucoids, highest growth is achieved over winter and/or spring when daylight and SST increase, while growth diminishes in summer (Lüning, 1993). While daylength dictates growth, seasonal temperature influences lipid and fatty acid composition (Britton et al., 2021). Elevated water temperatures earlier in the season may thus shift the biochemical composition of harvested species, which will affect end-consumers if harvest time is kept similar, but may also influence the broader fisheries industry through trophic interactions (Shalders et al., 2022). This may be mitigated by shifting of harvest season to earlier in the season, which may offer a potential opportunity for a second harvest later in the season. Seasonal mismatches may also occur between spore production and the ideal conditions for microscopic life phases of seaweed to grow and recruit (Martins et al., 2017; Bartsch et al., 2013). While the commercial culture of gametophytes is generally achieved under controlled lab conditions, spores are often sourced from wild populations. Tracking optimum time frames for spore harvesting as seasons shift may thus be of relevance to future aquaculture projects (Veenhof et al., 2023).
The seasonal effects of temperature may also interact with effects from ocean acidification. OA can stimulate growth and nitrogen accumulation during warmer seasons in Gracilaria lemaneiformis and thus a shift of harvest period to later in the season may be beneficial in an acidifying ocean (Chen et al., 2018). Season can also determine whether OA exacerbates or mitigates the negative effect of ocean warming, which may have knock-on effects for time of harvest. For example, in Fucus vesiculosus, OA mitigate the effects of warming in spring and early summer, but the mitigating effect of OA on temperature stress ceased in high summer (Graiff et al., 2015). The results suggest that ocean acidification may impart benefits to temperature resilience in some seaweeds, but that these benefits are limited beyond certain temperature thresholds (24°C, F. vesiculosus), and at certain seasonal time-points (spring, F. vesiculosus).
Iodine is one of many biochemical components of seaweeds that can vary seasonally and with environmental conditions. Iodine is a key food supplement derived from seaweeds, but can be harmful to for example thyroid function when consumed in excess through seaweed consumption by both humans and animals (Farebrother et al., 2019). Iodine from seaweeds can also bio-accumulate in higher trophic levels, for instance in abalones in integrated aquaculture systems, leading to a risk of excess consumption (Xu et al., 2019). Increased temperature can increase iodine concentration in Ecklonia cava (Satoh et al., 2019). In contrast, iodine content increased in winter during colder conditions for L. digitata (Nitschke et al., 2018). Iodine content also significantly increased in monocultures of cultivated kelp as compared to wild stands of S. latissima (Roleda et al., 2018). In cultivation trials for S. latissima, early deployment (October) decreased iodine content as compared to late (January) deployment, demonstrating the clear influence of seasonality on iodine accumulation (Arlov et al., 2024). Currently, many available seaweed food products already contain more iodine per serving portion than is recommended by the Scientific Committee on Food (Aakre et al., 2021; Redway and Combet, 2023). As such, increased iodine content from shifts in season or time of harvest may cause increased risk of excess iodine intake. In addition, increased iodine may be excreted as volatile halocarbon compounds particularly under ocean warming scenarios, which can increase radiative forcing if released in large quantities (Keng et al., 2020). However, at current scale, Atlantic aquaculture is unlikely to pose a significant effect on global radiative forcing (Duarte et al., 2022).
2.2 Challenge 2: extreme events
2.2.1 Storms
Ocean warming has been linked to an increase in storm events and other extreme weather events (Meehl et al., 2000). There is evidence for the enhanced poleward movement of storms in the mid-latitudes due to the increase in atmospheric water vapor and strengthening of upper-level wind velocities (Tamarin-Brodsky and Kaspi, 2017; Wolf et al., 2020). This could increase the risk of severe winter storms over the mid-latitudes in Europe resulting in intense rainfall and stronger winds (Wolf et al., 2020). Changes in the strength of the North Atlantic Oscillation (NAO) towards the end of the 21st century may lead to regional differences in the frequency and intensity of storms. Storms and wind speeds over Central and Western Europe may increase in prevalence and strength, which have the potential to be more destructive to coastal systems (Wolf et al., 2020; Woollings et al., 2012). The North Atlantic coast of North America is also expected to see an increase in tropical storms and hurricanes due to increasing SSTs in the North Atlantic (Marsooli et al., 2019; Villarini and Vecchi, 2012). Increased storms can damage seaweed aquaculture infrastructure, which are often submerged floating longlines (Figure 2), resulting in economic losses and potential risk of marine pollution, affecting overall sustainability of a seaweed farm (Campbell et al., 2019).
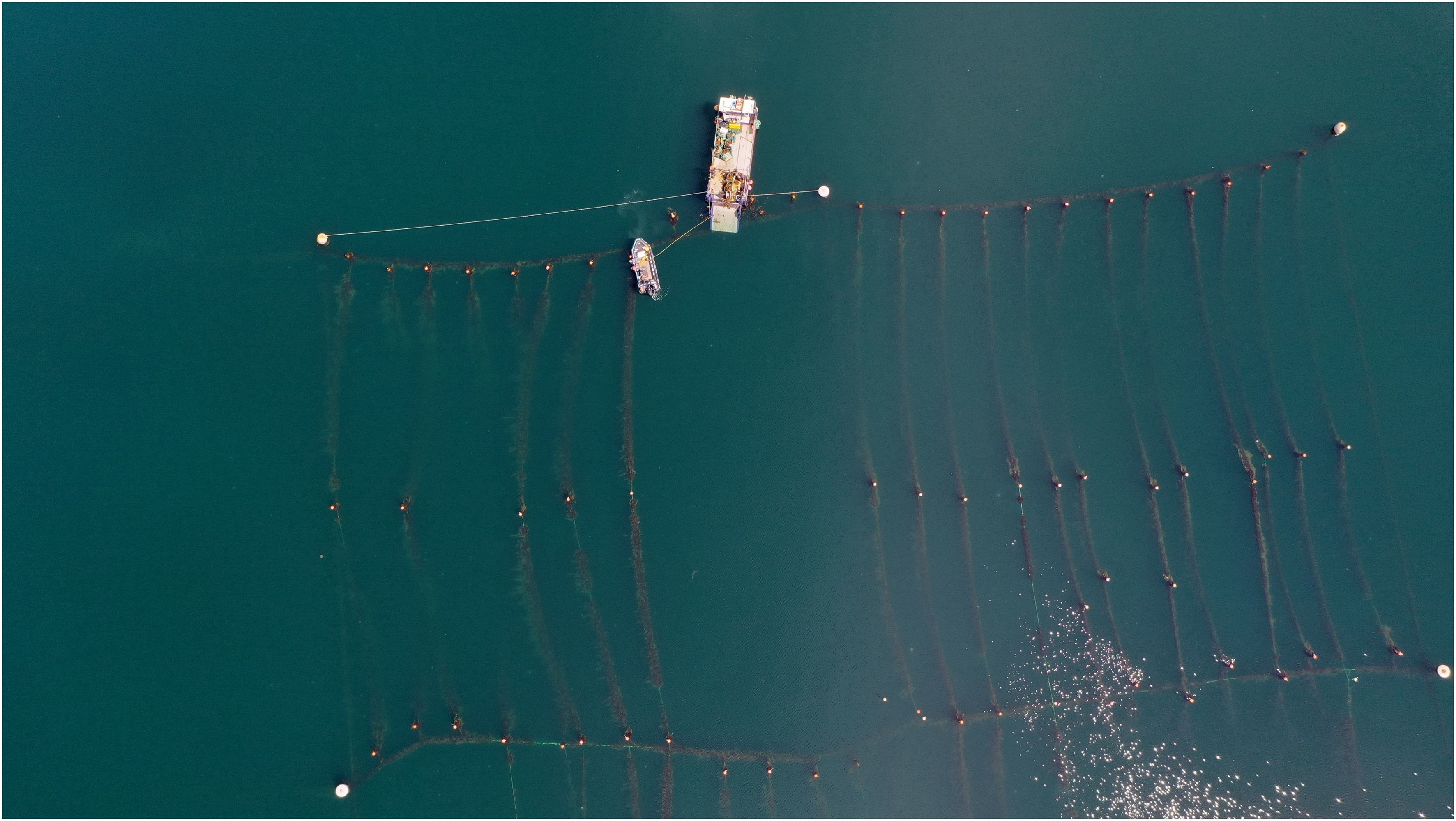
Figure 2. Harvest of sugar kelp (Saccharina latissima) at an experimental seaweed farm, Scotland. This farm utilizes the traditional submerged longline design. Photo credit: A. O’Dell (Scottish Association for Marine Science).
The biology of the seaweeds themselves can also be affected by increased storminess. The morphology of cultivated seaweed species (e.g. frond length and width) and the ability of their holdfast to stay secured may be affected by increased hydrodynamic forcing caused by the increase in storm frequency and strength. For example, the cultivated kelp species S. latissima generally grows longer and thinner in higher energy, exposed environments where stronger wave action and current strength occur (Peteiro and Freire, 2013). Deploying morphologically plastic crops in increasingly storm-affected environments could result in the direct loss of biomass, as well as compositional changes, due to ‘skinnier’ growth forms, generally better adapted to high wave energy (Koehl et al., 2008). It may be more desirable to cultivate species that are able to adapt to grow in high energy environments and maintain high biomass, such as the kelp species L. hyperborea and A. esculenta (Pedersen et al., 2012; Stamp, 2015; Smale and Vance, 2015).
Seasonal variations in the individual kelp biomass and surface area can decrease effects of wave action, whereby a loss in kelp tissue due to erosion or spore production results in less storm-generated drag so that the kelp is less prone to detachment and better able to withstand peak water velocities (de Bettignies et al., 2013, 2015). Peak water velocities and minimum individual biomass both occur over the autumn-winter season. This reflects the kelps adaptive response to severe hydrological impact. Kelp survival is also enhanced by a strong holdfast attachment to the substrate, although holdfast fatigue can occur over time with maturity (de Bettignies et al., 2015). Indeed, resilience to high wave-energy environments can change with life stage, where young plants and old plants are both at higher risk of dislodgement (Thomsen et al., 2004). Increased storms can thereby influence choice of deployment times, as optimal nutrient and light conditions for holdfast development in autumn in the Atlantic region coincide with periods of increased storms during which early life stages may become easily dislodged (Kerrison et al., 2015). Despite the increased risk of breakage through entanglement and drag, high wave-energy can increase NPP in certain species of kelps (Smale et al., 2016; Pedersen et al., 2012), though high energy storms in coastal areas can also reduce light availability through sediment turbidity, decreasing NPP (Franke et al., 2023).
2.2.2 Freshwater input and flooding
With increasing incidence of storms and cyclones also comes the increased risk of flooding and freshwater input to surface waters (Marsooli et al., 2019). Freshwater runoff from terrestrial systems can temporarily decrease the salinity in near-coastal waters and impact biodiversity (Gillanders and Kingsford, 2002). In polar regions, seasonal increase of ice melting may also contribute to freshwater influxes (IPCC, 2023; Timmermans and Marshall, 2020). Riverine runoff can also cause nutrient influxes as well as decreased light availability due to increased turbidity (Gillanders and Kingsford, 2002). All these factors may influence growth and performance of seaweeds and are thus relevant for seaweed aquaculture.
Lowered salinity can cause considerable damage to a seaweed crop, depending on the severity of the salinity fluctuation and the species involved. Euryhaline species with high tolerance for salinity changes such as Ulva sp. and red seaweeds including Gracilaria sp. could be good candidates for aquaculture near major river mouths (Glauco et al., 2024; Yu et al., 2013). However, many of the species currently targeted for aquaculture in the Atlantic, for instance many Laminarian species, have a lower tolerance for reduced salinity. For example, E. radiata kelp forests have been reported to decline as a result of increased rainfall and flooding off the Australian coast (Davis et al., 2022b). Adverse effects of reduced salinity can also be compounded by temperature or light stress (Monteiro et al., 2021; Diehl et al., 2020; Spurkland and Iken, 2011). Some kelp sporophytes endemic to the Arctic display a tolerance to low salinity (5-33 ppt) offering potential candidates for aquaculture in low salinity environments (Muth et al., 2021). Whilst under osmotic stress, brown algae can synthesize mannitol, a low molecular weight carbohydrate, which acts as an osmolyte and prevents damage from low salinities (Iwamoto and Shiraiwa, 2005; Diehl et al., 2023). Other compositional changes observed in brown seaweeds include a higher percentage of fermentable sugars (glucose and mannitol) due to low salinity in S. latissima and L. digitata as opposed to higher biomass and protein content at high salinity (Nielsen et al., 2016). The effect of salinity on biochemical composition varies with species and populations (Diehl et al., 2023), highlighting the need for further research in this area on aquaculture species.
Increase in frequency and severity of extreme weather is also predicted to cause deterioration in water quality in coastal areas, through enhanced runoff, flooding events and upwelling (Nazari-Sharabian et al., 2018). Though seaweeds can mitigate nutrient and pollutant increase through absorption, the increased variability in nutrient loading in coastal waters has also been directly linked to the establishment of invasive algal species in new areas (Incera et al., 2009; Bermejo et al., 2020). Nutrient loading can increase growth of faster growing invasive species on and near slow-growing cultivated species. This in turn leads to a reduced quantity of the biomass produced and a competition for nutrients, light and space (Pedersen and Borum, 1996). Flooding can cause epiphytes and diseases to decrease crop yields, which makes them unsuitable for harvest and consumption and may have consequences for food security (Behera et al., 2022; Ward et al., 2020). In addition to nutrient runoff and eutrophication, decreased clarity of seawater due to sediment discharge may majorly impact upon the production of seaweed farms, as it does in natural seaweed beds (Tait et al., 2021). This is especially relevant in more urbanized areas, where many river-linked systems have already experienced a lowering in water clarity, also called coastal darkening, such as the North Sea. Coastal darkening can reduce carbon acquisition up to 95% in kelps, which has major consequences for kelp farming in coastal areas (Blain et al., 2021). Research shows that despite a decrease in light availability, good crop yields may still be obtained in certain seaweed species due to greater nutrient availability (van der Molen et al., 2018).
2.2.3 Marine heatwaves
As well as driving an increase in average SST, climate change also contributes significantly to the increased frequency and intensity of marine heatwave events (defined as spikes of anomalous temperatures lasting at least five consecutive days) (IPCC, 2023; Sen Gupta et al., 2020). In the last century, marine heatwaves have doubled in intensity and duration (Oliver et al., 2018). These heatwaves can cause direct mortality of seaweeds and can favor the establishment of non-native or invasive species (Atkinson et al., 2020). Marine heatwaves have been directly linked to increased incidence and susceptibility to algal diseases, pests, and epiphytes, including the tropical bacterial disease ‘ice-ice’ in Kappaphycus sp. and Eucheuma spp (Largo et al., 2017). Ice-ice is a major disease of Kappaphycus and has been reported to have caused local losses on farms in Indonesia of up to $17,300–18,500 USD, and amounting to an estimated $100 million USD losses annually in the Philippines (Ward et al., 2020, 2022).
Short-term thermal stress may alter both productivity and biochemical composition of cultivated seaweeds. Short-term temperature stresses have been linked to reduced product quality in K. alvarezii, in particular in terms of the yield and characteristics of extracted carrageenan (Kumar et al., 2020). Likewise, brown seaweeds can reduce photosynthesis, protein and total fatty acid content in response to heatwaves (Britton et al., 2023; Nepper-Davidsen et al., 2019). On the other hand, certain species have shown no change in their biochemical composition in reaction to marine heatwaves (Shalders et al., 2023). Most research on marine heatwaves to-date has focused on the effects on natural seaweed populations (Smith et al., 2024, 2023; Smale et al., 2019), pointing to a knowledge gap on how marine heatwaves will affect chemical composition and productivity of farmed seaweed. These studies often mark warm-edge populations as most vulnerable to marine heatwaves, indicating that site selection should ideally be in the center range of the species of interest. Sudden loss of crops or disease outbreaks may be linked to marine heatwaves, but further evidence is required. In addition, seaweed farms are often located in sheltered areas which may be more exposed to localized surface warming, especially in areas of reduced tidal exchange. More fundamental and applied research is essential to enable technical and strategic mitigation strategies, such as lowering growing lines to cooler waters, to be proactively employed before marine heatwaves occur, thereby minimizing damage and economical loss to seaweed farms.
2.3 Challenge 3: disease and herbivory
2.3.1 Disease
Physiological impacts from the changes in temperature, salinity, and CO2 on seaweeds are often compounded by increased disease and pest susceptibility due to cumulative physiological stresses and reduced fitness (Largo et al., 2017; Qiu et al., 2019). Increased disease susceptibility can also be caused by environmental factors that disturb the microbiota that naturally occur on the seaweed, termed ‘dysbiosis’, which then leaves the seaweed vulnerable to invasion of pathogenic microbes (Egan and Gardiner, 2016). For example, decreased survival in the seaweed Delisea pulchra was caused by warmer waters, inducing stress and increasing its susceptibility to bacterial infection. This then led to an increased occurrence of bleaching events, which in turn further damaged and stressed the seaweed (Campbell et al., 2011).
Research on diseases in seaweed species has so far focused on species of aquaculture interest (Gachon et al., 2010; Ward et al., 2020). Of these, there is more research available on tropical species, such as Kappaphycus and Eucheuma species, including the widespread infection that commonly afflicts them, ‘ice-ice’ (Behera et al., 2022). Ice-ice presents a clear example of ocean-warming induced disease spread, as stock is more susceptible to infection with ice-ice during spikes of warming or heatwave events (Largo et al., 2017). For example, a significantly higher susceptibility to ice-ice (from 0 to 100% infection) following just one week of >30°C water temperatures was shown in lab studies of K. alvarezii (Largo et al., 1995). Similar patterns of association between disease outbreaks in Kappaphycus and Eucheuma sp. and heatwave events or spikes of low salinity have also been observed in natural populations and on cultivation lines (Pang et al., 2015; Ndawala et al., 2022).
There is less available knowledge on prevalent diseases and their interactions with environmental factors in temperate species currently used in Atlantic aquaculture (but see Ward et al., 2020). White spot disease in S. japonica causes blisters and white spots on the front, and can decrease iodine and crude protein content by ~20%, as well as lower photosynthetic pigment concentrations and daily growth rates (Wang et al., 2021). Green rotten disease meanwhile strikes early, mostly affecting S. japonica juveniles (Li et al., 2020). Cataloguing different pathogens has mainly focused on bacteria and fungi. Epiphytes and viruses may also cause considerable damage and need further research attention (Behera et al., 2022; Matsson et al., 2019). Creative solutions need to be developed to minimize the threat of crop diseases under ocean change and foster collaboration. A fantastic, albeit short-lived, example of this was the web-portal where farmers can report seaweed disease and send samples found on their farms (Strittmatter et al., 2022). Lessons can be learned from some of the problems encountered with tropical seaweed aquaculture, where the extensive use of cloning has reduced the gene pool and is thought to have lowered disease resistance (Valero et al., 2017). However, solutions can also be found in tropical aquaculture, for example, usage of mixed crops which can enhance resilience to diseases (Pang et al., 2015).
2.3.2 Herbivory
Grazing is a well-known mechanism controlling the range and productivity of natural seaweed beds (Ling et al., 2015; Vergés et al., 2016; Dayton et al., 1984). Despite extensive attention on the effects of grazing on wild seaweed populations, little is known about the effects of herbivory on commercially grown seaweeds in the North Atlantic (Behera et al., 2022). As with diseases, most of the knowledge is concentrated around the tropical species Kappaphycus and Eucheuma. Grazing of these cultivated species can lead to tissue damage, and thus crop loss (Mantri et al., 2017), but also an increased risk of further infection (Tan et al., 2020). Grazing can trigger the seaweeds defense mechanisms, which in turn can change the biochemical composition of the crop and may lower the nutritional or palatable quality of the products (Cruz-Rivera and Villareal, 2006; Toth et al., 2007). As an example, many brown seaweeds increase phlorotannin content as a reaction to grazing (Pavia and Toth, 2000; Taylor et al., 2002).
In the context of climate change, there are certain factors which may exacerbate grazing activity on commercial farms. Topicalization is the movement of tropical species into temperate habitats, where they can cause substantial damage to seaweed beds (Vergés et al., 2016). Tropical fish can cause more extensive damage than native species, as they are adapted to feed continuously and are able to remove large portions (60 - 97%) from seaweed systems daily (Hay and Fenical, 1988). In tropical reef systems, this maintains a balance between coral and seaweed abundance (Bellwood and Fulton, 2008). However, in temperate reef environments, this mode of grazing can often be unsustainable and detrimental to seaweed populations (Vergés et al., 2016; Bennett et al., 2015). Atlantic fish assemblages are shifting towards more warm water affiliated species (Chust et al., 2024; Horta e Costa et al., 2014). The inclusion of large-scale farms in these temperate environments may allow tropical grazers to flourish in these regions under ocean warming, potentially acting as initial foothold habitats for invasive species and resulting in spillover to natural seaweed populations. In addition, metabolic theory predicts that increased temperature increases oxygen consumption (Gillooly et al., 2001), which can lead to increased consumption in some grazers (Leung et al., 2021; O’Connor, 2009; Carey et al., 2016). Chemical defense mechanisms against grazers may also be reduced under warming and acidified conditions (Kinnby et al., 2021). Compounding factors such as these may exacerbate grazing impact on seaweed farms under ocean change.
Grazing by herbivorous fish in temperate aquaculture systems has already been observed on S. latissima and U. pinnatifida (Peteiro and Freire, 2012). The types of grazers that may affect seaweed farms will depend on the location of the farm. With inshore farms, it is expected that similar grazers to those of natural seaweed beds will interact with the farmed seaweed, both macrograzers (e.g. fish and sea urchins) and mesograzers (small crustaceans and gastropods). However, with the introduction of open ocean seaweed farming, novel interactions between grazers, epifauna and the farmed seaweed may occur. Currently, there are very few offshore, open ocean farms in operation but there is considerable interest given the spatial scale needed to make seaweed farming more economically viable (Visch et al., 2023; Bak et al., 2020). It will be vital to thoroughly research any potential interactions harmful for either the farmed seaweed or the environment at large before open ocean aquaculture ventures are carried out at scale.
3 Opportunities
As the effects of climate change become more severe, there is a greater drive for researching potential solutions and adaptations in seaweeds to changing climate conditions. With increasingly sophisticated oceanographic and climate modelling, future conditions at potential cultivation sites may be more accurately predicted to assist in the selection of seaweed aquaculture sites and species with changing oceans in mind. In the era of ‘omics’ approaches, there are opportunities in breeding and trait selection for climate resilience, as well as enhancing performance through targeted microbe treatments (Li et al., 2023; Kim et al., 2017). And finally, the capacity and motivation to restore natural seaweed habitats has increased with the public awareness that many seaweed ecosystems are under threat from climate change (Eger et al., 2023). Many individual restoration projects have sprung up worldwide in recent years, however technical challenges remain in terms of scale and feasibility (Coleman et al., 2020b). Here we highlight the benefits from closer collaboration between restoration and aquaculture ventures and investigate the use of restorative approaches in aquaculture beneficial to both industry and the environment it depends on.
3.1 Opportunity 1: ‘future-proof’ site selection
Site selection is the first barrier to overcome when starting a new seaweed cultivation operation. The environmental conditions of the site must include the suitable range for the chosen species over the cultivation cycle in order to ensure adequate crop quantity and quality (Kerrison et al., 2015). This will include seawater temperature, salinity, nutrient and light levels, as well as prevalent current and wave regime. However, environmental conditions are set to shift in response to climate change, which will affect not only existing aquaculture operations, but also the siting of future developments. With careful consideration, sites and species can be selected with an eye on future climate conditions.
3.1.1 Site considerations in a changing climate
Current cultivation sites in the North Atlantic are often positioned in naturally sheltered, coastal and estuarine environments to facilitate operations and minimize wave and storm damage. The physical resilience of aquaculture infrastructure and gear (such as anchored lines or mooring systems) must be modified to withstand increased loading and mechanical failure due to storm surge damage such as increased wave current velocities, high winds and large waves (Bricknell et al., 2021). For example, infrastructure designs may be optimized to dissipate wave energy and take into consideration local geomorphology and hydrology to select sheltered sites at peak storm surge timings. As such, a farm site can even protect the shoreline from damaging wave action and increase coastal resilience (Zhu et al., 2021). However, the selection of such sites is not straightforward. With increased storms, increased flooding is expected to affect tide-surges in estuarine and coastal systems, for which there is currently a lack of accurate modelling capacity (Bricknell et al., 2021). Resolving these issues through improved modelling may greatly improve spatial planning of seaweed farms with consideration given to future storm and flooding events.
Another important consideration with regard to increased flooding is the increased influx of freshwater and decreased salinity, which can have detrimental effects on seaweed beds (Davis et al., 2022b). Choosing sites with higher vertical mixing and/or upwelling may better help mitigate against the impact of osmotic stress, as they restore salinity to ambient levels. Alternatively, cultivation of seaweed at greater depths, with adjustable depth control, or on offshore sites may resolve some of these issues as salinity is more stable at greater depths and offshore (Stammer et al., 2021). Light may become limited at greater depths, though successful cultivation and greater depths have been reported from pilot off-shore farms as water clarity often improves offshore (Bak et al., 2020).
Increasing average SST, as well as increased occurrences of marine heatwaves, may make sites unsuitable for the cultivation of certain species in the near future. However, suitable management plans can help to mitigate negative outcomes. Marine heatwaves can be forecast with reasonable accuracy up to one year in advance (Jacox et al., 2022). Digital resources, such as www.marineheatwaves.org, which can be used for real-time monitoring and future prediction of marine heatwaves, are becoming an increasingly powerful tool for preventative strategies and policies. For example, both the United States and Australia recently implemented nationwide marine heatwave briefings designed to aid the shellfish aquaculture industry in mitigating damage (Hobday et al., 2023). As the seaweed aquaculture industry uses controlled environment nursery systems for seed-line production, operations may choose to deploy later in the season if heatwaves are predicted to occur at the time of deployment. This delayed deployment however comes at the cost of greater risks of autumn storms and increased light limitation during the critical early grow-out stage.
3.1.2 Future range shifts of Atlantic commercial species
With increasingly sophisticated models of historic and future natural kelp distributions, a clearer picture emerges of where net gain/loss in kelp biomass will occur under long-term ocean warming (e.g. Krumhansl et al., 2016; Davis et al., 2022a; Goldsmit et al., 2021; Gouvêa et al., 2024; Assis et al., 2024, 2022). However, analysis of range shifts that focus specifically on commercially important species in the context of seaweed aquaculture remain scarce (but see Assis et al., 2018; Wilson et al., 2019). We, therefore, present a case study where we project future distributions based on thermal niche of five commercially important species in the North Atlantic; L. digitata, A. esculenta, S. latissima, L. hyperborea and Palmaria palmata. Projections use the ssp370 medium-high warming scenario and project to a near-future of 2070 (methods fully described in García Molinos et al. (2016); see also Supplementary Material for clarification).
Climate velocity trajectory (CVTs, Figure 3) models show projected losses at warm edges of species ranges and gains at cold edges. Together, these approximate the simple predictions of shifts in the isotherms corresponding to thermal limits. Losses at warm edges were projected to be severe for some species. They include a complete loss by 2070 of L. hyperborea and S. latissima from northern Spain (Figures 3C, E), reduction in range in the same area for P. palmata (Figure 3D), and extensive loss of range in southwest Britain, Ireland, and France for A. esculenta and L. digitata (Figures 3A, B). Importantly, the projected distributions indicate that large parts of the UK, Ireland and North America will be unsuitable for growing A. esculenta by 2070, and parts of Spain and France unsuitable for S. latissima, both species being currently favored in Atlantic aquaculture (Figure 1). A shift in the species considered for aquaculture to more thermally tolerant species, for example L. ochroleuca and S. polyschides, may mitigate some of these losses (Casado-Amezúa et al., 2019).
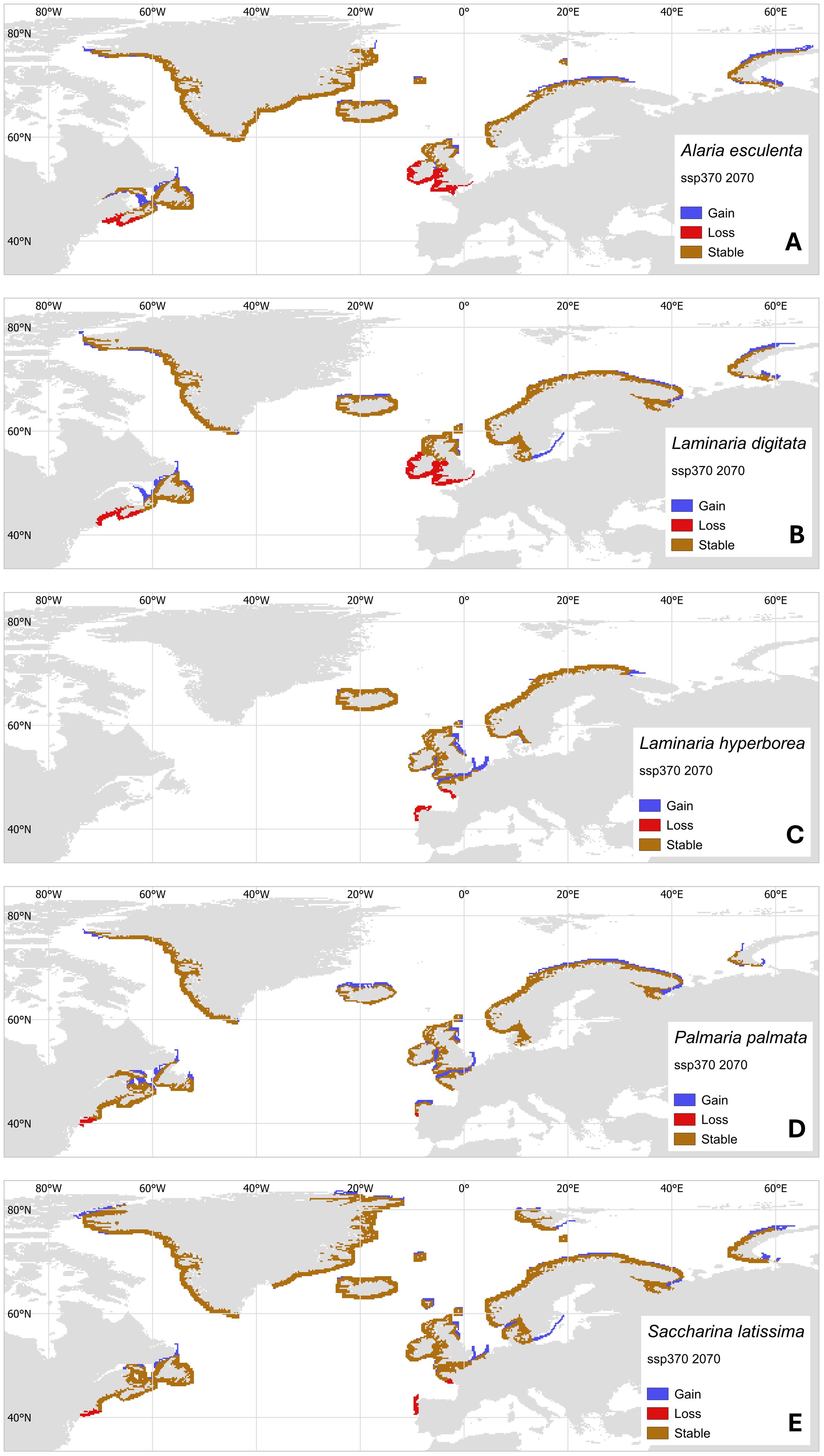
Figure 3. Projected geographical distributions of North Atlantic aquaculture species by 2070 under the medium-high ssp370 warming scenario from shifts in isotherms from present-day range locations. Maps show projected changes for (A) Alaria esculenta, (B) Laminaria digitata, (C) Laminaria hyperborea, (D) Palmaria palmata, and (E) Saccharina latissima. New areas of habitat (Gain, blue) are where conditions become climatically suitable, while habitats lost (Loss, red) are where future temperatures are likely to exceed maximum baseline temperatures within the distribution range. Stability (brown) is indicated where populations persist.
CVT models suggest that all species examined would have newly suitable areas for growth at the cold edges of their distributions. Range expansions in the sugar kelp S. latissima and winged kelp A. esculenta may occur in the Russian Arctic, but less area appears suitable in Greenland and the Canadian Arctic. Aquaculture activity will not be limited by considerations of population connectivity and available rocky substrate, although use of species in newly thermally suitable areas may be limited by other factors. Most importantly among these are water clarity, salinity fluxes and the extreme seasonality of light availability, which may be a constraint for poleward expansion of cold-temperate species of seaweed (Filbee-Dexter et al., 2019) and thus cultivation of seaweed in these regions. For example, Laminaria solidungula is endemic to the Arctic, though kelps common in the Atlantic and suited for aquaculture such as S. latissima, A. esculenta, and L. digitata grow in the Arctic as well (Wiencke and Amsler, 2012; Filbee-Dexter et al., 2019). Generally, if a kelp is a seasonal anticipator (starting growth and reproduction under short-day conditions in winter and early spring, anticipating summer conditions) they may fare well under Arctic conditions, where long daylight coincides with low nutrient conditions (Wiencke and Amsler, 2012; Kain, 1989). This growth under suboptimal light conditions is facilitated by the storing of carbon acquired during summer periods, in the form of laminarian and/or lipids, thus potentially changing biochemical composition compared to temperate kelps (Scheschonk et al., 2019; Olischläger et al., 2014). Many kelps growing in the North Atlantic originated in the Pacific with multiple crossings of the Arctic occurring in their evolutionary past (Starko et al., 2019), while recent genomic data shows that kelps persisted through several periods of glaciation (Bringloe et al., 2022). This indicates that North Atlantic kelps may already be adapted to grow under future polar conditions. Trials with commonly cultured species under darkness would be beneficial to understand the constraints of expanding seaweed aquaculture into the Arctic as more areas become continuously free of sea ice.
3.2 Opportunity 2: advances in breeding & microbiome manipulation
3.2.1 Breeding and hybridization
Advances in genomic research on seaweeds has greatly expanded the toolkit available for breeding desirable traits in seaweeds. So far, many of the breeding efforts in seaweeds have been focused on increased biomass and growth, and most available knowledge is on the few species grown in large quantities in Asia, predominantly of the genera Gracilaria, Porphyra, Saccharina, Undaria and Ulva (Patwary et al., 2021). Through a mixture of self-fertilization, cross breeding between populations, and selection of well-performing offspring, strains of S. japonica and U. pinnatifida now exist in China and Korea that yield far higher growth and dry biomass weights than in early cultivar lines (Li et al., 2016a; Shan et al., 2016; Li et al., 2016b). The number of available seaweed genomes for commercially grown species has also increased rapidly in the past decade offering platforms for further genetic breeding programs (Wang et al., 2020; Nelson et al., 2024). A recent leap in sequencing effort has made a further 110 seaweed genomes publicly available, spanning 105 different species (Nelson et al., 2024). Among cultivated seaweeds, several have been successfully genetically modified to express recombinant proteins, including S. japonica, U. pinnatifida, K. alvarezii, Porphyra yezoensis and Ulva lactuca (Trujillo et al., 2024). Recently, CRISPR-Cas9 has been used to successfully gene edit Ectocarpus and S. japonica gametophytes which were able to produce sporophytes, paving the way for further gene-editing studies (Shen et al., 2023; Badis et al., 2021). The use of transcriptomics, metabolomics and proteomics are still in their early stages for most seaweeds compared to terrestrial crop species (Patwary et al., 2021). However, exciting advances have been made in recent years which offer potential solutions to the challenges presented by climate change, which are discussed below.
With increasing pressure from climate change, there has been an increased effort to discover and characterize environmentally resilient strains of seaweeds for cultivation. One major advance in this area has been the identification of the molecular basis of stress responses in several species of seaweed, such as heat shock proteins (HSPs) (Hammann et al., 2016; Eggert, 2012; Smolina et al., 2016). HSPs are key players in stress response in land plants and protect cells from damage due to heat (and other) stress (Timperio et al., 2008). Stress-related transcriptomic studies have mostly been carried out on red seaweeds, paving the way to understanding the molecular basis for stress resilience in algae more widely. For example, P. yezoensis displays upregulation of HSP under increased temperature stress (Sun et al., 2015). More recently, transcriptomic analysis showed that thermal resilience is higher among outbred crosses of L. digitata, which was underpinned by differentially expressed genes (Liesner et al., 2022). Interestingly, whilst inbred and outbred crosses performed similarly physiologically, the underlying protein expressions were different, indicating a divergent metabolic pathway to cope with temperature stress (Liesner et al., 2022). Such use of transcriptomics in breeding experiments under heat stress provides invaluable data that may be further used in targeted molecular breeding.
Huang et al. (2022) trialed genomic selection in kelp breeding in S. latissima, where genotyped gametophytes were used to grow sporophytes, which were then evaluated for desirable traits such as increased wet and dry weight. The next breeding cycle used genetic selection to perform optimal crosses with gametophytes containing beneficial traits as defined by genotyping. Genetic selection at the gametophyte stage was successful which resulted in higher yields (weight, length) in farmed sporophytes, the effects of which increased over several breeding cycles, indicating genetic gain (Huang et al., 2023). Some roadblocks to successful use of genetic selection in seaweed breeding still remain, e.g. difficulties in upscaling bulk cultures of gametophytes, improving spore survival, and in inducing spore release of desirable sporophytes to start the next breeding cycle (Huang et al., 2022). If these roadblocks can be overcome, genetic selection may be useful in the selection of climate stress resilient strains for future deployment. Furthermore, legislation around breeding and genetic modification in kelps is not yet well-defined in many countries, and should be underpinned by knowledge on genetic variety in local populations, as well as the scale of genetic impact from farm to wild populations (Goecke et al., 2020).
Whilst many of these advances can help make seaweed farms more resilient to ocean change, care should be taken to not negatively impact genetic diversity in natural seaweed beds (Campbell et al., 2019; Hu et al., 2023). The widespread use of clonal monocultures, as well as threats from climate change, can result in the loss of wild genetic resources that underpin climate resilience (Goecke et al., 2020; Coleman et al., 2020a; Valero et al., 2017). Efforts to map wild genetic diversity in seaweed species of cultivation interest are improving (e.g. Fouqueau et al., 2024), alongside efforts to biobank and preserve wild genetic resources for future use (Wade et al., 2020; Brakel et al., 2021). Taking note of genetic diversity within farms may not only help in preserving genetic diversity of wild populations but can also enhance resilience of the farmed species to climate change. Through hybridization, which may preserve genetic diversity, physiological performance under stress can be increased (Goecke et al., 2020; Hu et al., 2023). This has been shown in multiple kelp species (e.g. Martins et al., 2019; Murúa et al., 2021; Hara and Akiyama, 1985) and Porphyra (Kim, 2011). Interestingly, fecundity in M. pyrifera gametophytes appears influenced by the degree of relatedness, as well as population of origin (Camus et al., 2021; Solas et al., 2024). This indicates that interpopulation breeding may also benefit productivity at the nursery stage, depending on the population of origin.
3.2.2 Priming of early life history stages
Another promising avenue for advancing stress tolerance of broodstock is through priming the early life history stages of seaweed with sublethal levels of stress, so that the subsequent adult generation is more resilient to that stressor (Jueterbock et al., 2021). As this can be done without the need for inbreeding or performing outcrosses, this does not increase risk of genetic depression in either farmed or natural populations. The molecular basis for priming is relatively well established in agriculture practices, where this technique is routinely used to enhance crop stress resilience (Liu et al., 2022a). Exposure to heat stress, for example, triggers certain genes to switch on, which is retained in later life stages through epigenetic modification such as methylation (Liu et al., 2022a). However, as seaweeds often have several life history phases, the basis of passing on ‘stress memory’ diverges from that of land plants. Recently, cold-priming of L. digitata gametophytes was shown to improve thermal resilience in the sporophyte generation, which is thought to be a result of epigenetic modification (Gauci et al., 2022). Increased methylation under both cold and warm temperature stress has been found in G. lemaneiformis (Peng et al., 2018). In S. latissima, methylation patterns were associated with culturing conditions, and differed significantly from field samples, as well as differing between populations of origin, showing the importance of environment in determining methylation patterns (Scheschonk et al., 2023). In S. japonica, heat stress caused an increase of methylation, which in turn regulated genes connected to heat stress response such as the production of HSPs (Liu et al., 2023a). Cross-stressor use of priming has also proven effective in A. esculenta, where high light doses during early cultivation decreased the thermal stress response of sporophytes (Martins et al., 2022). These results indicate that priming may be an effective and relatively easy to achieve method of increasing thermal resilience in seaweed stocks used for aquaculture. However, the effect of priming has only been tested in gametophytes and juvenile sporophytes. Whether the increased resilience to temperature stress from priming carries over into adult cultivated sporophytes remains to be tested.
3.2.3 Microbiome manipulation in the nursery stage
There is increasing research interest in the role of microbiota on the physiology and ecology of the seaweed host. The microbial community and the host, together termed the holobiont, can be considered as one functional entity responding and adapting to environmental change (Egan et al., 2013; van der Loos et al., 2019a). As microbes have short generation spans, they can be of use in accelerating adaptation to environmental change in the host organism and, as such, have received attention in the context of climate change adaptability of seaweeds (Eger et al., 2022; Wood et al., 2019). As early as the 1980s, research on Ulva sp. showed abnormal development of morphological characteristics when changing the epibiotic community associated with the Ulva host (Provasoli and Pintner, 1980). Since then, research has expanded to characterize, identify and isolate beneficial strains of microorganisms involved in seaweed growth and disease resistance. A recent review by Li et al. (2023) outlines a pathway for using microbiota manipulation for improving the seaweed aquaculture industry. Here, we focus on some studies that have the potential to increase climate change resilience of cultivated species.
Disease resistance is one major pathway in which microbiota can be exploited to increase climate change resilience of cultivated seaweed species. For example, identification of a bacterial strain (Phaeobacter sp. BS52) that protects against opportunistic harmful microbial invasion causing bleaching in Delisea pulchra (using the model pathogen Aquimarina sp. AD1) shows a potential pathway of enhancing disease resistance through manipulation of microbial communities (Li et al., 2022a). As D. pulchra is more susceptible to pathogens under elevated temperature, the addition of BS52 may enhance its resilience to ocean warming. Moreover, the beneficial effects of BS52 were applicable to a non-native host, Agarophyton vermiculophyllum, where inoculation worked better in reducing harmful effects of a bleaching disease as compared to its native microbiota (Li et al., 2022b). In S. japonica, differences in associated microbiota between healthy and infected juvenile sporophytes offer the potential for developing microbial inoculates to enhance resistance against white bleaching disease, which has a damaging effect on the nursery stages of this cultivated kelp (Ling et al., 2022). Based on this, a beneficial strain of bacteria was isolated (Vibrio alginolyticus X-2) which increased S. japonica’s immune response and disease resistance via changing the transcriptome and metabolome of inoculated juvenile sporophytes (Ma et al., 2023b).
Inoculation of early life history stages may be an effective way of improving overall disease and climate resilience in cultivated species, as this can be done in vivo in nursery facilities, and whole broodstocks can be treated at once. There are, however, some significant knowledge gaps in how effectively the microbiota transfer from one life stage to the next. Recently, some indication was found that the parent microbiota transfers to the gametophyte in M. pyrifera, as there was a significant effect of population of origin on the microbiome of gametophyte cultures (Osborne et al., 2023). In addition, strains were identified (within the Mesorhizobium genus) that were associated with increased biomass acquisition in the sporophyte stage (Osborne et al., 2023). Contrastingly, Davis et al. (2023) found little to no transference of nursery gametophyte microbes to the out-planted sporophytes of A. marginata and S. latissima. Instead, species, time of year and source microbiota influenced the associated microbiota on cultivated sporophytes (Davis et al., 2023). Recruitment of microbial communities in cultivated Sargassum fusiforme seedlings was also mostly governed by stochastic processes (Liu et al., 2023b).
There is increased evidence that the microbiota of the seaweed holobiont plays a role in the response of the host to thermal stress. The ability to maintain stable microbiota through host selection under thermal stress was linked to invasiveness in G. vermiculophylla (Bonthond et al., 2023). Thermal stress also changes the microbiota of Cystoceira compressa and E. radiata, among other species, which subsequently affects both growth and photosynthetic capability (Qiu et al., 2019; Mancuso et al., 2023). However, direct evidence for microbial inoculation increasing thermal resilience is still lacking. Juveniles of Dictyota dichotoma did not perform better when inoculated with a mix of naturally occurring microbiota, neither did the inoculum affect the epibiota (Delva et al., 2023). However, this may have been due to the sourcing of the inoculum, which was taken from seawater at the same temperature as the lower thermal treatment. This shows that ample opportunity still exists to examine the potential of enhanced stress tolerance in seaweeds via microbiota manipulation, and conflicting results point to knowledge gaps defining the underlying mechanisms of the role of microbiota in seaweed stress resilience.
3.3 Opportunity 3: restorative aquaculture
While seaweed aquaculture production continues to accelerate, there is an increasing awareness of the simultaneous threat to natural seaweed beds from changing climate. Initiatives to restore natural seaweed ecosystems have developed worldwide in the last few decades (e.g. Eger et al., 2023; Vergés et al., 2020; Chung et al., 2013). However, large-scale restoration projects are still rare. There are some success stories, for example active restoration of 500-800 hectare of seaweed habitat in Korea and Japan (Eger et al., 2020), 8500 hectares of macrophyte habitat (also including seagrass) in China (Liu et al., 2022b) and the protection of 30,000 hectares of kelp habitat for rewilding in the English Channel (Williams et al., 2022). While these are all steps in the right direction, large scale restoration will need significant investment of both time and monetary funds, as well as technological advances in growing and breeding seaweeds (Eger et al., 2020). There is an opportunity for collaboration between aquaculture and restoration projects, as investment and technical advances will be more likely to develop in the aquaculture sector. This synergy is required so that maximum benefits can be derived from restorative approaches to seaweed aquaculture, which will be discussed in this section.
3.3.1 Co-culture
Improving resilience in both aquaculture and restoration projects may be boosted by using co-culture techniques rather than growing one target species for restoration and cultivation. There are not many studies available yet which examine a more ecosystem-based approach to growing seaweed, such as is being trialed in agriculture with the use of restorative and permaculture practices (Corrigan et al., 2022). However, there are some studies that indicate that diversifying crops grown in aquaculture facilities can increase yield. For example, co-culture of Kappaphycus sp. with Euchema denticulatum increased resistance against ice-ice during the summer months, when chances of infection rise with ocean temperatures (Pang et al., 2015). In their study, co-culturing was achieved by alternating longlines growing one species each, and infection rates of ice-ice and the epiphyte Neosiphonia savatieri were reduced from ~80% in individually cultured species, to ~14% in co-culture (Pang et al., 2015). Furthermore, the co-culture of cultivated seaweeds with species that are non-palatable to grazers such as Caulerpa and Halimeda spp. also decreased grazing of Gracilaria sp. by herbivores (Ganesan et al., 2006). Co-culture may thus increase resilience to ocean-warming induced disease, while also increasing the potential increasing derived biodiversity benefits of a seaweed farm by increasing macroalgal diversity.
This concept may be extended to co-culture with species of shellfish or finfish, which has been more widely researched. Integrated multitrophic aquaculture (IMTA) may have further knock-on benefits for the local system it is placed in by absorbing nutrients and dissolved CO2 (Duarte et al., 2022; Kim et al., 2017; Ross et al., 2023). This lowers pH locally and provides a chemical refuge for marine calcifiers such as shellfish, limiting low saturation levels of aragonite (ΩArag) and thus lowering the risk of shell dissolution (Fernández et al., 2019; Falkenberg et al., 2021). In turn, the shellfish provide additional nutrients (e.g. nitrate, urea, phosphate) for seaweed growth, thus increasing the potential for large-scale macroalgal cultivation and providing economic benefits for both the seaweed and shellfish farm. However, the pH-buffering capacity of seaweeds is highly species-specific and depends on the local community structure and prevailing hydrodynamic conditions (Ricart et al., 2023). Some studies show a significant increase in pH, O2 and ΩArag in seaweed farms which can increase shellfish growth, as well as large fluctuations in pH hypothesized to help shellfish adapt to acidification (Xiao et al., 2021; Li et al., 2021; Young et al., 2022). Others have found no benefits derived from co-culturing shellfish with several species of seaweed (Leal et al., 2024). Overall, more research is required in this area, particularly regarding the cumulative effect of OA and warming on seaweed performances. Often the negative impact of ocean warming outweighs any beneficial effect of acidification (Graba-Landry et al., 2018; Britton et al., 2020; Wahl et al., 2020). In addition, a better understanding is needed for the co-culture of shellfish and seaweeds regarding target species and productivity rates and their interactions with local environmental factors.
3.3.2 Restoration and cultivation co-benefits
Besides bio-buffering in a commercial IMTA context, seaweed farms may also be used as a pH buffering strategy in naturally occurring ecosystems which rely on calcifying species (e.g. coral reefs, maerl beds, oyster reefs). These species are most vulnerable to OA as their structural integrity is threatened by lowered oceanic pH (Doney et al., 2009). For example, seaweed farming partially mitigated OA in a coral reef ecosystem, but mitigation success (in terms of maximum increase in pH and ΩArag) depended on the optimum location, size, seaweed density and harvesting strategy of the farm (Mongin et al., 2016). To the best of our knowledge, this is the only study that has investigated benefits of acidification in the context of coral reefs, but the projected co-benefits certainly warrant further research.
Other ways in which restoration and aquaculture industries can benefit from collaboration is through knowledge sharing and generating funds to achieve successful restoration. Certainly, one of the main roadblocks to many restoration initiatives is lack of funding, as well as technical knowledge and facilities for cultivating seaweeds on a large scale (Eger et al., 2020). The aims of aquaculture and restoration industries are distinct: where aquaculture may be concerned mostly with increasing biomass and composition of the product, restoration is interested in successful transference of ecosystem-wide benefits. There are, however, some areas in which these two industries overlap. For example, both industries will benefit from climate-proof solutions to cultivation, maintaining genetic variety in biobanks and cost-effective technologies for large-scale deployment. In particular, the maintenance and provision of seedstock by commercial scale nurseries to support restoration has the potential to accelerate restoration scale and success (Filbee-Dexter et al., 2022). Currently, many restoration projects propagate their own seedstock derived from small source populations or transplanted adult individuals, which is both costly and diminishes the chances of success through lack of genetic resilience (Eger et al., 2022). Commercial scale nurseries have the knowledge and skills to increase resilience and genetic diversity in their broodstock, which will benefit restoration by increasing robustness to climate change. Recent biobanking initiatives focusing on Atlantic species are paving the way for preserving genetically diverse broodstock (e.g. the SeaStrains initiative by the Global Seaweed Coalition, and several biobanks like Biobancos (Portugal), the Seaweed Nursery at the Scottish Association for Marine Science (UK), and CCAP (UK)). Another factor that can hinder both aquaculture and restoration initiatives is permitting, social license and legislation (Eger et al., 2022; Wood et al., 2017). A more integrated push from both restoration and aquaculture industries may increase the speed and efficacy in which the necessary legislative changes for both coastal cultivation and restoration are achieved.
4 Conclusion
Globally, seaweed aquaculture is currently a major contributor (~50%) to ocean-based aquaculture production and has the potential to expand further as the need for sustainable food and materials increases (FAO, 2022). The seaweed industry, however, faces key challenges from ongoing climate change. In this review we have summarized some of the major challenges that the industry is facing through climate change stressors. On the other hand, we have highlighted opportunities for increasing resilience and sustainable development. These findings are summarized in Figure 4. Whilst some of the challenges are considerable, the strengths and opportunities highlighted in this review outnumber the weaknesses and threats, emphasizing the great potential of the seaweed industry as a sustainable industry. In recent years, there has been a lot of media attention on seaweed as a solution to many climate issues, creating a ‘seaweed hype’. To deliver on its promise however, technological difficulties and practical challenges pertaining to thermal tolerance, genetic diversity, scalability and disease resistance must be overcome. Here we have summarized research efforts that can provide a solution to some of these hurdles, and we hope to inspire further research in the three areas of opportunity: ‘Future-proof’ site selection, development of selective breeding and microbe inoculations for increased resilience, and taking a restorative or ecosystem approach to seaweed aquaculture. In the face of climate change, seaweed aquaculture offers a globally sustainable solution to some of the most pressing challenges related to food security and environmental stress.
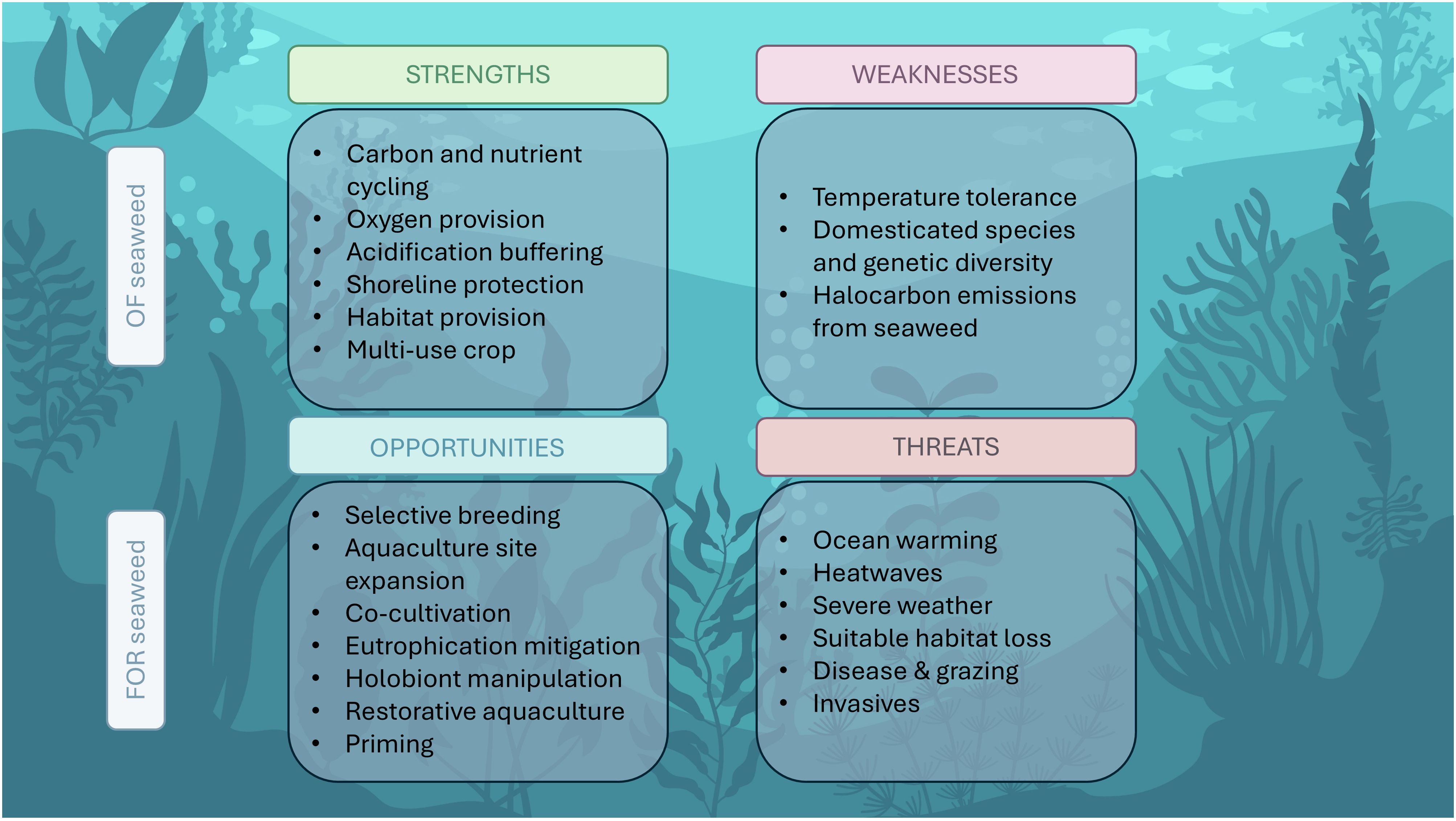
Figure 4. Summary of strengths and weaknesses of seaweed cultivation in the context of climate change, and the opportunities and challenges facing the seaweed industry.
Author contributions
RV: Conceptualization, Visualization, Writing – original draft, Writing – review & editing. MB: Conceptualization, Data curation, Formal analysis, Writing – review & editing, Visualization. AH: Conceptualization, Funding acquisition, Writing – review & editing. KM: Conceptualization, Writing – review & editing. MR: Conceptualization, Writing – review & editing. AT: Conceptualization, Writing – review & editing. JF: Conceptualization, Writing – review & editing. MS: Conceptualization, Funding acquisition, Supervision, Writing – review & editing.
Funding
The author(s) declare financial support was received for the research, authorship, and/or publication of this article. This review was funded by Shell Global Solutions International BV and the EU H2020 project ASTRAL [grant agreement No. 863034].
Conflict of interest
Author JF was employed by the company Shell Exploration and Production Inc.
The remaining authors declare that the research was conducted in the absence of any commercial or financial relationships that could be construed as a potential conflict of interest.
The authors declare that this study received funding from Shell Global Solutions International BV. The funder had the following involvement in the study: review of the article and the decision to submit it for publication.
Publisher’s note
All claims expressed in this article are solely those of the authors and do not necessarily represent those of their affiliated organizations, or those of the publisher, the editors and the reviewers. Any product that may be evaluated in this article, or claim that may be made by its manufacturer, is not guaranteed or endorsed by the publisher.
Supplementary material
The Supplementary Material for this article can be found online at: https://www.frontiersin.org/articles/10.3389/fmars.2024.1483330/full#supplementary-material
References
Aakre I., Solli D. D., Markhus M. W., Mæhre H. K., Dahl L., Henjum S., et al. (2021). Commercially available kelp and seaweed products–valuable iodine source or risk of excess intake? Food Nutr. Res. 65, 7584. doi: 10.29219/fnr.v65.7584
Alexander K. A., Potts T. P., Freeman S., Israel D., Johansen J., Kletou D., et al. (2015). The implications of aquaculture policy and regulation for the development of integrated multi-trophic aquaculture in Europe. Aquaculture 443, 16–23. doi: 10.1016/j.aquaculture.2015.03.005
Allen M. R., Frame D. J., Huntingford C., Jones C. D., Lowe J. A., Meinshausen M., et al. (2009). Warming caused by cumulative carbon emissions towards the trillionth tonne. Nature 458, 1163–1166. doi: 10.1038/nature08019
Arlov Ø, Nøkling-Eide K., Aarstad O. A., Jacobsen S. S., Langeng A.-M., Borrero-Santiago A. R., et al. (2024). Variations in the chemical composition of Norwegian cultivated brown algae Saccharina latissima and Alaria esculenta based on deployment and harvest times. Algal. Res. 78, 103421. doi: 10.1016/j.algal.2024.103421
Assis J., Araújo M. B., Serrão E. A. (2018). Projected climate changes threaten ancient refugia of kelp forests in the North Atlantic. Global Change Biol. 24, e55–e66. doi: 10.1111/gcb.2018.24.issue-1
Assis J., Fragkopoulou E., Gouvêa L., Araújo M. B., Serrão E. A. (2024). Kelp forest diversity under projected end-of-century climate change. Diversity Distrib. 30, e13837. doi: 10.1111/ddi.13837
Assis J., Serrão E. A., Duarte C. M., Fragkopoulou E., Krause-Jensen D. (2022). Major expansion of marine forests in a warmer arctic. Front. Mar. Sci. 9, 850368. doi: 10.3389/fmars.2022.850368
Atkinson J., King N. G., Wilmes S. B., Moore P. J. (2020). Summer and winter marine heatwaves favor an invasive over native seaweeds. J. Phycol. 56, 1591–1600. doi: 10.1111/jpy.13051
Badis Y., Scornet D., Harada M., Caillard C., Godfroy O., Raphalen M., et al. (2021). Targeted CRISPR-Cas9-based gene knockouts in the model brown alga Ectocarpus. New Phytol. 231, 2077–2091. doi: 10.1111/nph.v231.5
Bak U. G., Gregersen Ó, Infante J. (2020). Technical challenges for offshore cultivation of kelp species: lessons learned and future directions. Botanica Marina. 63, 341–353. doi: 10.1515/bot-2019-0005
Bartsch I., Vogt J., Pehlke C., Hanelt D. (2013). Prevailing sea surface temperatures inhibit summer reproduction of the kelp Laminaria digitata at Helgoland (North Sea). J. Phycol. 49, 1061–1073. doi: 10.1111/jpy.2013.49.issue-6
Behera D. P., Ingle K. N., Mathew D. E., Dhimmar A., Sahastrabudhe H., Sahu S. K., et al. (2022). Epiphytism, diseases and grazing in seaweed aquaculture: A comprehensive review. Rev. Aquacult. 14, 1345–1370. doi: 10.1111/raq.12653
Bellwood D. R., Fulton C. J. (2008). Sediment-mediated suppression of herbivory on coral reefs: Decreasing resilience to rising sea-levels and climate change? Limnol. Oceanogr. 53, 2695–2701. doi: 10.4319/lo.2008.53.6.2695
Bennett S., Wernberg T., Harvey E. S., Santana-Garcon J., Saunders B. J. (2015). Tropical herbivores provide resilience to a climate-mediated phase shift on temperate reefs. Ecol. Lett. 18, 714–723. doi: 10.1111/ele.2015.18.issue-7
Bermejo R., Macmonagail M., Heesch S., Mendes A., Edwards M., Fenton O., et al. (2020). The arrival of a red invasive seaweed to a nutrient over-enriched estuary increases the spatial extent of macroalgal blooms. Mar. Environ. Res. 158, 104944. doi: 10.1016/j.marenvres.2020.104944
Billing S.-L., Rostan J., Tett P., Macleod A. (2021). Is social license to operate relevant for seaweed cultivation in Europe? Aquaculture 534, 736203. doi: 10.1016/j.aquaculture.2020.736203
Biskup S., Bertocci I., Arenas F., Tuya F. (2014). Functional responses of juvenile kelps, Laminaria ochroleuca and Saccorhiza polyschides, to increasing temperatures. Aquat. Bot. 113, 117–122. doi: 10.1016/j.aquabot.2013.10.003
Blain C. O., Hansen S. C., Shears N. T. (2021). Coastal darkening substantially limits the contribution of kelp to coastal carbon cycles. Global Change Biol. 27, 5547–5563. doi: 10.1111/gcb.v27.21
Bonthond G., Neu A.-K., Bayer T., Krueger-Hadfield S. A., Künzel S., Weinberger F. (2023). Non-native hosts of an invasive seaweed holobiont have more stable microbial communities compared to native hosts in response to thermal stress. Ecol. Evol. 13, e9753. doi: 10.1002/ece3.v13.1
Brakel J., Sibonga R. C., Dumilag R. V., Montalescot V., Campbell I., Cottier-Cook E. J., et al. (2021). Exploring, harnessing and conserving marine genetic resources towards a sustainable seaweed aquaculture. Plants. People. Planet. 3, 337–349. doi: 10.1002/ppp3.10190
Bricknell I. R., Birkel S. D., Brawley S. H., Van Kirk T., Hamlin H. J., Capistrant-Fossa K., et al. (2021). Resilience of cold water aquaculture: a review of likely scenarios as climate changes in the Gulf of Maine. Rev. Aquacult. 13, 460–503. doi: 10.1111/raq.12483
Bringloe T. T., Fort A., Inaba M., Sulpice R., Ghriofa C. N., Mols-Mortensen A., et al. (2022). Whole genome population structure of North Atlantic kelp confirms high-latitude glacial refugia. Mol. Ecol. 31, 6473–6488. doi: 10.1111/mec.v31.24
Britton D., Craig Mundy C. J., Mcallister J. (2023). “Nutritional quality of kelp as a key driver of commercial abalone productivity,” in Technical report series (Hobart, Tasmania: University of Tasmania: Institute for Marine and Antarctic Studies).
Britton D., Mundy C. N., Mcgraw C. M., Revill A. T., Hurd C. L. (2019). Responses of seaweeds that use CO2 as their sole inorganic carbon source to ocean acidification: differential effects of fluctuating pH but little benefit of CO2 enrichment. ICES. J. Mar. Sci. 76, 1860–1870. doi: 10.1093/icesjms/fsz070
Britton D., Schmid M., Noisette F., Havenhand J. N., Paine E. R., Mcgraw C. M., et al. (2020). Adjustments in fatty acid composition is a mechanism that can explain resilience to marine heatwaves and future ocean conditions in the habitat-forming seaweed Phyllospora comosa (Labillardière) C.Agardh. Global Change Biol. 26, 3512–3524. doi: 10.1111/gcb.15052
Britton D., Schmid M., Revill A. T., Virtue P., Nichols P. D., Hurd C. L., et al. (2021). Seasonal and site-specific variation in the nutritional quality of temperate seaweed assemblages: implications for grazing invertebrates and the commercial exploitation of seaweeds. J. Appl. Phycol. 33, 603–616. doi: 10.1007/s10811-020-02302-1
Bronselaer B., Zanna L. (2020). Heat and carbon coupling reveals ocean warming due to circulation changes. Nature 584, 227–233. doi: 10.1038/s41586-020-2573-5
Buschmann A. H., Camus C., Infante J., Neori A., Israel Á., Hernández-González M. C., et al. (2017). Seaweed production: overview of the global state of exploitation, farming and emerging research activity. Eur. J. Phycol. 52, 391–406. doi: 10.1080/09670262.2017.1365175
Callaway R., Shinn A. P., Grenfell S. E., Bron J. E., Burnell G., Cook E. J., et al. (2012). Review of climate change impacts on marine aquaculture in the UK and Ireland. Aquat. Conserv.: Mar. Freshw. Ecosyst. 22, 389–421. doi: 10.1002/aqc.2247
Campbell A. H., Harder T., Nielsen S., Kjelleberg S., Steinberg P. D. (2011). Climate change and disease: bleaching of a chemically defended seaweed. Global Change Biol. 17, 2958–2970. doi: 10.1111/j.1365-2486.2011.02456.x
Campbell I., Kambey C. S. B., Mateo J. P., Rusekwa S. B., Hurtado A. Q., Msuya F. E., et al. (2020). Biosecurity policy and legislation for the global seaweed aquaculture industry. J. Appl. Phycol. 32, 2133–2146. doi: 10.1007/s10811-019-02010-5
Campbell I., Macleod A., Sahlmann C., Neves L., Funderud J., Øverland M., et al. (2019). The environmental risks associated with the development of seaweed farming in Europe - Prioritizing key knowledge gaps. Front. Mar. Sci. 6. doi: 10.3389/fmars.2019.00107
Camus C., Solas M., Martínez C., Vargas J., Garcés C., Gil-Kodaka P., et al. (2021). Mates matter: Gametophyte kinship recognition and inbreeding in the giant kelp, Macrocystis pyrifera (Laminariales, Phaeophyceae). J. Phycol. 57, 711–725. doi: 10.1111/jpy.13146
Carey N., Harianto J., Byrne M. (2016). Sea urchins in a high-CO2 world: partitioned effects of body size, ocean warming and acidification on metabolic rate. J. Exp. Biol. 219, 1178–1186. doi: 10.1242/jeb.136101
Casado-Amezúa P., Araújo R., Bárbara I., Bermejo R., Borja Á., Díez I., et al. (2019). Distributional shifts of canopy-forming seaweeds from the Atlantic coast of Southern Europe. Biodivers. Conserv. 28, 1151–1172. doi: 10.1007/s10531-019-01716-9
Chan F., Barth J. A., Blanchette C. A., Byrne R. H., Chavez F., Cheriton O., et al. (2017). Persistent spatial structuring of coastal ocean acidification in the California Current System. Sci. Rep. 7, 2526. doi: 10.1038/s41598-017-02777-y
Chen B., Zou D., Du H., Ji Z. (2018). Carbon and nitrogen accumulation in the economic seaweed Gracilaria lemaneiformis affected by ocean acidification and increasing temperature. Aquaculture 482, 176–182. doi: 10.1016/j.aquaculture.2017.09.042
Choo K.-S., Snoeijs P., Pedersén M. (2004). Oxidative stress tolerance in the filamentous green algae Cladophora glomerata and Enteromorpha ahlneriana. J. Exp. Mar. Biol. Ecol. 298, 111–123. doi: 10.1016/j.jembe.2003.08.007
Chung I. K., Oak J. H., Lee J. A., Shin J. A., Kim J. G., Park K.-S. (2013). Installing kelp forests/seaweed beds for mitigation and adaptation against global warming: Korean Project Overview. ICES. J. Mar. Sci. 70, 1038–1044. doi: 10.1093/icesjms/fss206
Chung I. K., Sondak C. F. A., Beardall J. (2017). The future of seaweed aquaculture in a rapidly changing world. Eur. J. Phycol. 52, 495–505. doi: 10.1080/09670262.2017.1359678
Chust G., Villarino E., Mclean M., Mieszkowska N., Benedetti-Cecchi L., Bulleri F., et al. (2024). Cross-basin and cross-taxa patterns of marine community tropicalization and deborealization in warming European seas. Nat. Commun. 15, 2126. doi: 10.1038/s41467-024-46526-y
Clements J. C., Chopin T. (2017). Ocean acidification and marine aquaculture in North America: potential impacts and mitigation strategies. Rev. Aquacult. 9, 326–341. doi: 10.1111/raq.2017.9.issue-4
Coleman M. A., Minne A. J. P., Vranken S., Wernberg T. (2020a). Genetic tropicalisation following a marine heatwave. Sci. Rep. 10, 12726. doi: 10.1038/s41598-020-69665-w
Coleman M. A., Wood G., Filbee-Dexter K., Minne A. J. P., Goold H. D., Vergés A., et al. (2020b). Restore or redefine: Future trajectories for restoration. Front. Mar. Sci. 7, 237. doi: 10.3389/fmars.2020.00237
Corrigan S., Brown A. R., Ashton I. G. C., Smale D. A., Tyler C. R. (2022). Quantifying habitat provisioning at macroalgal cultivation sites. Rev. Aquacult. 14, 1671–1694. doi: 10.1111/raq.12669
Coumou D., Rahmstorf S. (2012). A decade of weather extremes. Nat. Climate Change 2, 491–496. doi: 10.1038/nclimate1452
Cruz-Rivera E., Villareal T. A. (2006). Macroalgal palatability and the flux of ciguatera toxins through marine food webs. Harmful. Algae. 5, 497–525. doi: 10.1016/j.hal.2005.09.003
Davis K. M., Zeinert L., Byrne A., Davis J., Roemer C., Wright M., et al. (2023). Successional dynamics of the cultivated kelp microbiome. J. Phycol. 59, 538–551. doi: 10.1111/jpy.13329
Davis T. R., Champion C., Coleman M. A. (2022a). Ecological interactions mediate projected loss of kelp biomass under climate change. Diversity Distrib. 28, 306–317. doi: 10.1111/ddi.13462
Davis T. R., Larkin M. F., Forbes A., Veenhof R. J., Scott A., Coleman M. A. (2022b). Extreme flooding and reduced salinity causes mass mortality of nearshore kelp forests. Estuarine. Coast. Shelf. Sci. 275, 107960. doi: 10.1016/j.ecss.2022.107960
Davison I. R., Greene R. M., Podolak E. J. (1991). Temperature acclimation of respiration and photosynthesis in the brown alga Laminaria saccharina. Mar. Biol. 110, 449–454. doi: 10.1007/BF01344363
Dayton P. K., Currie V., Gerrodette T., Keller B. D., Rosenthal R., Tresca D. V. (1984). Patch dynamics and stability of some California kelp communities. Ecol. Monogr. 54, 253–289. doi: 10.2307/1942498
de Bettignies T., Wernberg T., Lavery P. S., Vanderklift M. A., Gunson J. R., Symonds G., et al. (2015). Phenological decoupling of mortality from wave forcing in kelp beds. Ecology 96, 850–861. doi: 10.1890/13-2365.1
de Bettignies T., Wernberg T., Lavery P. S., Vanderklift M. A., Mohring M. B. (2013). Contrasting mechanisms of dislodgement and erosion contribute to production of kelp detritus. Limnol. Oceanogr. 58, 1680–1688. doi: 10.4319/lo.2013.58.5.1680
Delva S., De Baets B., Baetens J. M., De Clerck O., Stock W. (2023). No bacterial-mediated alleviation of thermal stress in a brown seaweed suggests the absence of ecological bacterial rescue effects. Sci. Total. Environ. 876, 162532. doi: 10.1016/j.scitotenv.2023.162532
Diehl N., Karsten U., Bischof K. (2020). Impacts of combined temperature and salinity stress on the endemic Arctic brown seaweed Laminaria solidungula J. Agardh. Polar. Biol. 43, 647–656. doi: 10.1007/s00300-020-02668-5
Diehl N., Steiner N., Bischof K., Karsten U., Heesch S. (2023). Exploring intraspecific variability – biochemical and morphological traits of the sugar kelp Saccharina latissima along latitudinal and salinity gradients in Europe. Front. Mar. Sci. 10, 995982. doi: 10.3389/fmars.2023.995982
Doney S. C., Fabry V. J., Feely R. A., Kleypas J. A. (2009). Ocean acidification: The other CO2 problem. Annu. Rev. Mar. Sci. 1, 169–192. doi: 10.1146/annurev.marine.010908.163834
Duarte C. M., Bruhn A., Krause-Jensen D. (2022). A seaweed aquaculture imperative to meet global sustainability targets. Nat. Sustainabil. 5, 185–193. doi: 10.1038/s41893-021-00773-9
Duarte C. M., Wu J., Xiao X., Bruhn A., Krause-Jensen D. (2017). Can seaweed farming play a role in climate change mitigation and adaptation? Front. Mar. Sci.4, 100. doi: 10.3389/fmars.2017.00100
Egan S., Gardiner M. (2016). Microbial dysbiosis: Rethinking disease in marine ecosystems. Front. Microbiol. 7, 00991. doi: 10.3389/fmicb.2016.00991
Egan S., Harder T., Burke C., Steinberg P., Kjelleberg S., Thomas T. (2013). The seaweed holobiont: understanding seaweed–bacteria interactions. FEMS Microbiol. Rev. 37, 462–476. doi: 10.1111/1574-6976.12011
Eger A. M., Aguirre J. D., Altamirano M., Arafeh-Dalmau N., Arroyo N. L., Bauer-Civiello A. M., et al. (2023). The Kelp Forest Challenge: A collaborative global movement to protect and restore 4 million hectares of kelp forests. J. Appl. Phycol. 36, 951–964. doi: 10.1007/s10811-023-03103-y
Eger A. M., Marzinelli E. M., Christie H., Fagerli C. W., Fujita D., Gonzalez A. P., et al. (2022). Global kelp forest restoration: past lessons, present status, and future directions. Biol. Rev. 97, 1449–1475. doi: 10.1111/brv.12850
Eger A. M., Vergés A., Choi C. G., Christie H., Coleman M. A., Fagerli C. W., et al. (2020). Financial and institutional support are important for large-scale kelp forest restoration. Front. Mar. Sci. 7, 811. doi: 10.3389/fmars.2020.535277
Eggert A. (2012). “Seaweed responses to temperature,” in Seaweed Biology: Novel Insights into Ecophysiology, Ecology and Utilization. Eds. Wiencke C., Bischof K. (Springer Berlin Heidelberg, Berlin, Heidelberg).
Falkenberg L. J., Scanes E., Ducker J., Ross P. M. (2021). Biotic habitats as refugia under ocean acidification. Conserv. Physiol. 9, coab077. doi: 10.1093/conphys/coab077
FAO (2022). The state of world fisheries and aquaculture 2022. Towards Blue Transformation (Rome: FAO).
Farebrother J., Zimmermann M. B., Andersson M. (2019). Excess iodine intake: sources, assessment, and effects on thyroid function. Ann. New York. Acad. Sci. 1446, 44–65. doi: 10.1111/nyas.2019.1446.issue-1
Feehan C. J., Grace S. P., Narvaez C. A. (2019). Ecological feedbacks stabilize a turf-dominated ecosystem at the southern extent of kelp forests in the Northwest Atlantic. Sci. Rep. 9, 7078. doi: 10.1038/s41598-019-43536-5
Fernández P. A., Leal P. P., Henríquez L. A. (2019). Co-culture in marine farms: macroalgae can act as chemical refuge for shell-forming molluscs under an ocean acidification scenario. Phycologia 58, 542–551. doi: 10.1080/00318884.2019.1628576
Filbee-Dexter K., Feehan C. J., Scheibling R. E. (2016). Large-scale degradation of a kelp ecosystem in an ocean warming hotspot. Mar. Ecol. Prog. Ser. 543, 141–152. doi: 10.3354/meps11554
Filbee-Dexter K., Wernberg T. (2018). Rise of turfs: a new battlefront for globally declining kelp forests. BioScience 68, 64–76. doi: 10.1093/biosci/bix147
Filbee-Dexter K., Wernberg T., Barreiro R., Coleman M. A., De Bettignies T., Feehan C. J., et al. (2022). Leveraging the blue economy to transform marine forest restoration. J. Phycol. 58, 198–207. doi: 10.1111/jpy.13239
Filbee-Dexter K., Wernberg T., Fredriksen S., Norderhaug K. M., Pedersen M. F. (2019). Arctic kelp forests: Diversity, resilience and future. Global Planet. Change 172, 1–14. doi: 10.1016/j.gloplacha.2018.09.005
Fouqueau L., Reynes L., Blanfuné A., Mauger S., Assis J. (2024). Seascape genetic study on Laminaria digitata underscores the critical role of sampling schemes. Mar. Ecol. Prog. Ser. 740, 23–42. doi: 10.3354/meps14640
Franke K., Matthes L. C., Graiff A., Karsten U., Bartsch I. (2023). The challenge of estimating kelp production in a turbid marine environment. J. Phycol. 59, 518–537. doi: 10.1111/jpy.13327
Frieder C. A., Nam S. H., Martz T. R., Levin L. A. (2012). High temporal and spatial variability of dissolved oxygen and pH in a nearshore California kelp forest. Biogeosciences 9, 3917–3930. doi: 10.5194/bg-9-3917-2012
Gachon C. M., Sime-Ngando T., Strittmatter M., Chambouvet A., Kim G. H. (2010). Algal diseases: spotlight on a black box. Trends Plant Sci. 15, 633–640. doi: 10.1016/j.tplants.2010.08.005
Ganesan M., Thiruppathi S., Sahu N., Rengarajan N., Veeragurunathan V., Jha B. (2006). In situ observations on preferential grazing of seaweeds by some herbivores. Current Science, 1256–1260.
García Molinos J., Halpern B. S., Schoeman D. S., Brown C. J., Kiessling W., Moore P. J., et al. (2016). Climate velocity and the future global redistribution of marine biodiversity. Nat. Climate Change 6, 83–88. doi: 10.1038/nclimate2769
Gauci C., Bartsch I., Martins N., Liesner D. (2022). Cold thermal priming of Laminaria digitata (Laminariales, Phaeophyceae) gametophytes enhances gametogenesis and thermal performance of sporophytes. Front. Mar. Sci. 9, 862923. doi: 10.3389/fmars.2022.862923
Gillanders B. M., Kingsford M. J. (2002). Impact of changes in flow of freshwater on estuarine and open coastal habitats and the associated organisms. Oceanogr. Mar. Biol.: Annu. Rev. 40, 233–309.
Gillooly J. F., Brown J. H., West G. B., Savage V. M., Charnov E. L. (2001). Effects of size and temperature on metabolic rate. Science 293, 2248–2251. doi: 10.1126/science.1061967
Glauco F., He P., Chen Z. (2024). Combine effects of multiple environmental factors on growth and nutrient uptake of euryhaline seaweed growth in integrated multitrophic aquaculture systems. Algal. Res. 77, 103347. doi: 10.1016/j.algal.2023.103347
Goecke F., Klemetsdal G., Ergon Å. (2020). Cultivar development of kelps for commercial cultivation—Past lessons and future prospects. Front. Mar. Sci. 7, 110. doi: 10.3389/fmars.2020.00110
Goldsmit J., Schlegel R. W., Filbee-Dexter K., Macgregor K. A., Johnson L. E., Mundy C. J., et al. (2021). Kelp in the Eastern Canadian Arctic: Current and future predictions of habitat suitability and cover. Front. Mar. Sci. 8, 742209. doi: 10.3389/fmars.2021.742209
Goodwin P., Williams R. G., Ridgwell A. (2015). Sensitivity of climate to cumulative carbon emissions due to compensation of ocean heat and carbon uptake. Nat. Geosci. 8, 29–34. doi: 10.1038/ngeo2304
Gosch B. J., Lawton R. J., Paul N. A., De Nys R., Magnusson M. (2015). Environmental effects on growth and fatty acids in three isolates of Derbesia tenuissima (Bryopsidales, Chlorophyta). Algal. Res. 9, 82–93. doi: 10.1016/j.algal.2015.02.022
Gouvêa L., Fragkopoulou E., Legrand T., Serrão E. A., Assis J. (2024). Range map data of marine ecosystem structuring species under global climate change. Data Brief 52, 110023. doi: 10.1016/j.dib.2023.110023
Graba-Landry A., Hoey A. S., Matley J. K., Sheppard-Brennand H., Poore A. G. B., Byrne M., et al. (2018). Ocean warming has greater and more consistent negative effects than ocean acidification on the growth and health of subtropical macroalgae. Mar. Ecol. Prog. Ser. 595, 55–69. doi: 10.3354/meps12552
Graiff A., Bartsch I., Ruth W., Wahl M., Karsten U. (2015). Season exerts differential effects of ocean acidification and warming on growth and carbon metabolism of the seaweed Fucus vesiculosus in the Western Baltic Sea. Front. Mar. Sci. 2, 00112. doi: 10.3389/fmars.2015.00112
Hammann M., Wang G., Boo S. M., Aguilar-Rosas L. E., Weinberger F. (2016). Selection of heat-shock resistance traits during the invasion of the seaweed Gracilaria vermiculophylla. Mar. Biol. 163, 104. doi: 10.1007/s00227-016-2881-3
Hara M., Akiyama K. (1985). Heterosis in growth of Undaria pinnatifida (Harvey) Suringar. Bull. Tohoku. Regional. Fisheries. Res. Lab. 47, 47–50.
Harley C. D. G., Anderson K. M., Demes K. W., Jorve J. P., Kordas R. L., Coyle T. A., et al. (2012). Effects of climate change on global seaweed communities. J. Phycol. 48, 1064–1078. doi: 10.1111/j.1529-8817.2012.01224.x
Hay M. E., Fenical W. (1988). Marine plant-herbivore interactions: The ecology of chemical defense. Annu. Rev. Ecol. Syst. 19, 111–145. doi: 10.1146/annurev.es.19.110188.000551
Ho M., Mcbroom J., Bergstrom E., Diaz-Pulido G. (2021). Physiological responses to temperature and ocean acidification in tropical fleshy macroalgae with varying affinities for inorganic carbon. ICES. J. Mar. Sci. 78, 89–100. doi: 10.1093/icesjms/fsaa195
Hobday A. J., Burrows M. T., Filbee-Dexter K., Holbrook N. J., Sen Gupta A., Smale D. A., et al. (2023). With the arrival of El Niño, prepare for stronger marine heatwaves. Nature 621, 38–41. doi: 10.1038/d41586-023-02730-2
Horta e Costa B., Assis J., Franco G., Erzini K., Henriques M., Gonçalves E. J., et al. (2014). Tropicalization of fish assemblages in temperate biogeographic transition zones. Mar. Ecol. Prog. Ser. 504, 241–252. doi: 10.3354/meps10749
Hu Z.-M., Shan T.-F., Zhang Q.-S., Liu F.-L., Jueterbock A., Wang G., et al. (2023). Kelp breeding in China: Challenges and opportunities for solutions. Rev. Aquacult. 16, 855–871. doi: 10.1111/raq.12871
Huang M., Robbins K. R., Li Y., Umanzor S., Marty-Rivera M., Bailey D., et al. (2023). Genomic selection in algae with biphasic lifecycles: A Saccharina latissima (sugar kelp) case study. Front. Mar. Sci. 10, 1040979. doi: 10.3389/fmars.2023.1040979
Huang M., Robbins K. R., Li Y., Umanzor S., Marty-Rivera M., Bailey D., et al. (2022). Simulation of sugar kelp (Saccharina latissima) breeding guided by practices to accelerate genetic gains. G3 Genes|Genomes|Genetics. 12, jkac003. doi: 10.1093/g3journal/jkac003
Hughes A. D., Black K. D. (2016). Going beyond the search for solutions: understanding trade-offs in European integrated multi-trophic aquaculture development. Aquacult. Environ. Interact. 8, 191–199. doi: 10.3354/aei00174
Iida Y., Takatani Y., Kojima A., Ishii M. (2021). Global trends of ocean CO2 sink and ocean acidification: an observation-based reconstruction of surface ocean inorganic carbon variables. J. Oceanogr. 77, 323–358. doi: 10.1007/s10872-020-00571-5
Incera M., Olabarria C., Troncoso J. S., López J. (2009). Response of the invader Sargassum muticum to variability in nutrient supply. Mar. Ecol. Prog. Ser. 377, 91–101. doi: 10.3354/meps07866
IPCC (2023). Climate Change 2023: Synthesis Report. Contribution of Working Groups I, II and III to the Sixth Assessment Report of the Intergovernmental Panel on Climate Change (Geneva, Switzerland: IPCC).
Iwamoto K., Shiraiwa Y. (2005). Salt-regulated mannitol metabolism in algae. Mar. Biotechnol. 7, 407–415. doi: 10.1007/s10126-005-0029-4
Jacox M. G., Alexander M. A., Amaya D., Becker E., Bograd S. J., Brodie S., et al. (2022). Global seasonal forecasts of marine heatwaves. Nature 604, 486–490. doi: 10.1038/s41586-022-04573-9
Jayathilake D. R. M., Costello M. J. (2020). A modelled global distribution of the kelp biome. Biol. Conserv. 252, 108815. doi: 10.1016/j.biocon.2020.108815
Jueterbock A., Minne A. J. P., Cock J. M., Coleman M. A., Wernberg T., Scheschonk L., et al. (2021). Priming of marine macrophytes for enhanced restoration success and food security in future oceans. Front. Mar. Sci. 8, 658485. doi: 10.3389/fmars.2021.658485
Kain J. M. (1989). The seasons in the subtidal. Br. Phycol. J. 24, 203–215. doi: 10.1080/00071618900650221
Keng F. S.-L., Phang S.-M., Abd Rahman N., Leedham Elvidge E. C., Malin G., Sturges W. T. (2020). The emission of volatile halocarbons by seaweeds and their response towards environmental changes. J. Appl. Phycol. 32, 1377–1394. doi: 10.1007/s10811-019-02026-x
Kerrison P. D., Stanley M. S., Edwards M. D., Black K. D., Hughes A. D. (2015). The cultivation of European kelp for bioenergy: Site and species selection. Biomass Bioenergy 80, 229–242. doi: 10.1016/j.biombioe.2015.04.035
Khan N., Sudhakar K., Mamat R. (2024). Macroalgae farming for sustainable future: Navigating opportunities and driving innovation. Heliyon 10, e28208. doi: 10.1016/j.heliyon.2024.e28208
Kim N.-G. (2011). Culture study on the hybrid by interspecific crossing between Porphyra pseudolinearis and P. dentata (Bangiales, Rhodophyta), two dioecious species in culture. Algae 26, 79–86. doi: 10.4490/algae.2011.26.1.079
Kim B.-T., Brown C. L., Kim D.-H. (2019). Assessment on the vulnerability of Korean aquaculture to climate change. Mar. Policy 99, 111–122. doi: 10.1016/j.marpol.2018.10.009
Kim J. K., Yarish C., Hwang E. K., Park M., Kim Y. (2017). Seaweed aquaculture: cultivation technologies, challenges and its ecosystem services. Algae 32, 1–13. doi: 10.4490/algae.2017.32.3.3
King N. G., Moore P. J., Pessarrodona A., Burrows M. T., Porter J., Bue M., et al. (2020). Ecological performance differs between range centre and trailing edge populations of a cold-water kelp: implications for estimating net primary productivity. Mar. Biol. 167, 1–12. doi: 10.1007/s00227-020-03743-5
Kinnby A., Toth G. B., Pavia H. (2021). Climate change increases susceptibility to grazers in a foundation seaweed. Front. Mar. Sci. 8, 688406. doi: 10.3389/fmars.2021.688406
Koehl M. A. R., Silk W. K., Liang H., Mahadevan L. (2008). How kelp produce blade shapes suited to different flow regimes: A new wrinkle. Integr. Comp. Biol. 48, 834–851. doi: 10.1093/icb/icn069
Krause-Jensen D., Duarte C. M., Hendriks I. E., Meire L., Blicher M. E., Marbà N., et al. (2015). Macroalgae contribute to nested mosaics of pH variability in a subarctic fjord. Biogeosciences 12, 4895–4911. doi: 10.5194/bg-12-4895-2015
Krumhansl K. A., Okamoto D. K., Rassweiler A., Novak M., Bolton J. J., Cavanaugh K. C., et al. (2016). Global patterns of kelp forest change over the past half-century. Proc. Natl. Acad. Sci. 113, 13785–13790. doi: 10.1073/pnas.1606102113
Kübler J. E., Davison I. R. (1995). Thermal acclimation of light-use characteristics of Chondrus crispus (Rhodophyta). Eur. J. Phycol. 30, 189–195. doi: 10.1080/09670269500650971
Kübler J. E., Dudgeon S. R. (2015). Predicting effects of ocean acidification and warming on algae lacking carbon concentrating mechanisms. PloS One 10, e0132806. doi: 10.1371/journal.pone.0132806
Kübler J. E., Dudgeon S. R., Bush D. (2021). Climate change challenges and opportunities for seaweed aquaculture in California, the United States. J. World Aquacult. Soc. 52, 1069–1080. doi: 10.1111/jwas.12794
Kumar Y. N., Poong S.-W., Gachon C., Brodie J., Sade A., Lim P.-E. (2020). Impact of elevated temperature on the physiological and biochemical responses of Kappaphycus alvarezii (Rhodophyta). PloS One 15, e0239097. doi: 10.1371/journal.pone.0239097
Kwiatkowski L., Torres O., Bopp L., Aumont O., Chamberlain M., Christian J. R., et al. (2020). Twenty-first century ocean warming, acidification, deoxygenation, and upper-ocean nutrient and primary production decline from CMIP6 model projections. Biogeosciences 17, 3439–3470. doi: 10.5194/bg-17-3439-2020
Largo D. B., Chung I. K., Phang S.-M., Gerung G. S., Sondak C. F. A. (2017). “Impacts of climate change on Eucheuma-Kappaphycus Farming,” in Tropical Seaweed Farming Trends, Problems and Opportunities. Developments in Applied Phycology. Eds. Hurtado A. Q., Critchley A. T., Neish I. C. (Springer International Publishing, Cham).
Largo D. B., Fukami K., Nishijima T., Ohno M. (1995). Laboratory-induced development of the ice-ice disease of the farmed red algae Kappaphycus alvarezii and Eucheuma denticulatum (Solieriaceae, Gigartinales, Rhodophyta). J. Appl. Phycol. 7, 539–543. doi: 10.1007/BF00003940
Leal P. P., Uribe D., Henríquez-Antipa L. A., Jiménez C., Hormazábal L., Cascales E.-K. (2024). Evaluating the ability of macroalgae to create a chemical refuge for bivalves under ocean acidification conditions in closed-environment experiments. J. Appl. Phycol. 36, 1561–1575. doi: 10.1007/s10811-023-03163-0
Leung J. Y. S., Russell B. D., Coleman M. A., Kelaher B. P., Connell S. D. (2021). Long-term thermal acclimation drives adaptive physiological adjustments of a marine gastropod to reduce sensitivity to climate change. Sci. Total. Environ. 771, 145208. doi: 10.1016/j.scitotenv.2021.145208
Li J., Majzoub M. E., Marzinelli E. M., Dai Z., Thomas T., Egan S. (2022a). Bacterial controlled mitigation of dysbiosis in a seaweed disease. ISME. J. 16, 378–387. doi: 10.1038/s41396-021-01070-1
Li J., Pang S., Shan T., Su L. (2020). Changes of microbial community structures associated with seedlings of Saccharina japonica at early stage of outbreak of green rotten disease. J. Appl. Phycol. 32, 1323–1327. doi: 10.1007/s10811-019-01975-7
Li J., Weinberger F., De Nys R., Thomas T., Egan S. (2023). A pathway to improve seaweed aquaculture through microbiota manipulation. Trends Biotechnol. 41, 545–556. doi: 10.1016/j.tibtech.2022.08.003
Li J., Weinberger F., Saha M., Majzoub M. E., Egan S. (2022b). Cross-host protection of marine bacteria against macroalgal disease. Microbial. Ecol. 84, 1288–1293. doi: 10.1007/s00248-021-01909-2
Li J., Zhang W., Ding J., Xue S., Huo E., Ma Z., et al. (2021). Effect of large-scale kelp and bivalve farming on seawater carbonate system variations in the semi-enclosed Sanggou Bay. Sci. Total. Environ. 753, 142065. doi: 10.1016/j.scitotenv.2020.142065
Li X., Zhang Z., Qu S., Liang G., Sun J., Zhao N., et al. (2016a). Improving seedless kelp (Saccharina japonica) during its domestication by hybridizing gametophytes and seedling-raising from sporophytes. Sci. Rep. 6, 21255. doi: 10.1038/srep21255
Li X. J., Zhang Z. Z., Qu S. C., Liang G. J., Zhao N., Sun J., et al. (2016b). Breeding of an intraspecific kelp hybrid Dongfang no. 6 (Saccharina japonica, Phaeophyceae, Laminariales) for suitable processing products and evaluation of its culture performance. J. Appl. Phycol. 28, 439–447. doi: 10.1007/s10811-015-0562-0
Liesner D., Pearson G. A., Bartsch I., Rana S., Harms L., Heinrich S., et al. (2022). Increased heat resilience of intraspecific outbred compared to inbred lineages in the kelp laminaria digitata: physiology and transcriptomics. Front. Mar. Sci. 9, 838793. doi: 10.3389/fmars.2022.838793
Ling F., Egan S., Zhuang Y., Chang L., Xiao L., Yang Q., et al. (2022). Epimicrobiome shifts with bleaching disease progression in the brown seaweed Saccharina japonica. Front. Mar. Sci. 9, 865224. doi: 10.3389/fmars.2022.865224
Ling S., Scheibling R., Rassweiler A., Johnson C., Shears N., Connell S., et al. (2015). Global regime shift dynamics of catastrophic sea urchin overgrazing. Philos. Trans. R. Soc. B., 370, 20130269. doi: 10.1098/rstb.2013.0269
Liu H., Able A. J., Able J. A. (2022a). Priming crops for the future: rewiring stress memory. Trends Plant Sci. 27, 699–716. doi: 10.1016/j.tplants.2021.11.015
Liu F., Zhang P., Liang Z., Yuan Y., Liu Y., Wu Y. (2023a). The global dynamic of DNA methylation in response to heat stress revealed epigenetic mechanism of heat acclimation in Saccharina japonica. J. Phycol. 59, 249–263. doi: 10.1111/jpy.13305
Liu S., Zhou X., Zeng C., Frankstone T., Cao L. (2022b). Characterizing the development of Sea ranching in China. Rev. Fish. Biol. Fisheries. 32, 783–803. doi: 10.1007/s11160-022-09709-8
Liu W., Zou H., Wu S., Li N., Pang Q., Yan X. (2023b). Growth promotion of Sargassum fusiforme by epiphytic microbes is dependent on the extent of interspecific interactions of the microbial community. Sci. Total. Environ. 897, 165449. doi: 10.1016/j.scitotenv.2023.165449
Lowman H. E., Emery K. A., Dugan J. E., Miller R. J. (2022). Nutritional quality of giant kelp declines due to warming ocean temperatures. Oikos 2022, e08619. doi: 10.1111/oik.v2022.i7
Lüning K. (1993). Environmental and internal control of seasonal growth in seaweeds. Hydrobiologia 260, 1–14. doi: 10.1007/BF00048997
Lüning K. (1994). When do algae grow? The third Founders’ lecture. Eur. J. Phycol. 29, 61–67. doi: 10.1080/09670269400650501
Ma D., Gregor L., Gruber N. (2023a). Four decades of trends and drivers of global surface ocean acidification. Global Biogeochem. Cycles. 37, e2023GB007765. doi: 10.1029/2023GB007765
Ma M., Zhuang Y., Chang L., Xiao L., Lin Q., Qiu Q., et al. (2023b). Naturally occurring beneficial bacteria Vibrio alginolyticus X-2 protects seaweed from bleaching disease. mBio 14, e00065–e00023. doi: 10.1128/mbio.00065-23
Mancuso F. P., Morrissey K. L., De Clerck O., Airoldi L. (2023). Warming and nutrient enrichment can trigger seaweed loss by dysregulation of the microbiome structure and predicted function. Sci. Total. Environ. 879, 162919. doi: 10.1016/j.scitotenv.2023.162919
Mantri V. A., Eswaran K., Shanmugam M., Ganesan M., Veeragurunathan V., Thiruppathi S., et al. (2017). An appraisal on commercial farming of Kappaphycus alvarezii in India: success in diversification of livelihood and prospects. J. Appl. Phycol. 29, 335–357. doi: 10.1007/s10811-016-0948-7
Marsooli R., Lin N., Emanuel K., Feng K. (2019). Climate change exacerbates hurricane flood hazards along US Atlantic and Gulf Coasts in spatially varying patterns. Nat. Commun. 10, 3785. doi: 10.1038/s41467-019-11755-z
Martins N., Barreto L., Bartsch I., Bernard J., Serrão E. A., Pearson G. A. (2022). Daylength influences reproductive success and sporophyte growth in the Arctic kelp species Alaria esculenta. Mar. Ecol. Prog. Ser. 683, 37–52. doi: 10.3354/meps13950
Martins N., Pearson G. A., Gouveia L., Tavares A. I., Serrao E. A., Bartsch I. (2019). Hybrid vigour for thermal tolerance in hybrids between the allopatric kelps Laminaria digitata and L. pallida (Laminariales, Phaeophyceae) with contrasting thermal affinities. Eur. J. Phycol. 54, 548–561. doi: 10.1080/09670262.2019.1613571
Martins N., Tanttu H., Pearson G. A., Serrão E. A., Bartsch I. (2017). Interactions of daylength, temperature and nutrients affect thresholds for life stage transitions in the kelp Laminaria digitata (Phaeophyceae). Botanica Marina. 60, 109–121. doi: 10.1515/bot-2016-0094
Matsson S., Christie H., Fieler R. (2019). Variation in biomass and biofouling of kelp, Saccharina latissima, cultivated in the Arctic, Norway. Aquaculture 506, 445–452. doi: 10.1016/j.aquaculture.2019.03.068
Meehl G. A., Zwiers F., Evans J., Knutson T., Mearns L., Whetton P. (2000). Trends in extreme weather and climate events: Issues related to modeling extremes in projections of future climate change. Bull. Am. Meteorol. Soc. 81, 427–436. doi: 10.1175/1520-0477(2000)081<0427:TIEWAC>2.3.CO;2
Mongin M., Baird M. E., Hadley S., Lenton A. (2016). Optimising reef-scale CO2 removal by seaweed to buffer ocean acidification. Environ. Res. Lett. 11, 034023. doi: 10.1088/1748-9326/11/3/034023
Monteiro C., Li H., Diehl N., Collén J., Heinrich S., Bischof K., et al. (2021). Modulation of physiological performance by temperature and salinity in the sugar kelp Saccharina latissima. Phycol. Res. 69, 48–57. doi: 10.1111/pre.12443
Moy F. E., Christie H. (2012). Large-scale shift from sugar kelp (Saccharina latissima) to ephemeral algae along the south and west coast of Norway. Mar. Biol. Res. 8, 309–321. doi: 10.1080/17451000.2011.637561
Murúa P., Patiño D. J., Müller D. G., Westermeier R. (2021). Sexual compatibility in giant kelp gametophytes: inter-cultivar hybridization is average between parents but excels under harsher conditions. J. Appl. Phycol. 33, 3261–3275. doi: 10.1007/s10811-021-02506-z
Muth A. F., Bonsell C., Dunton K. H. (2021). Inherent tolerance of extreme seasonal variability in light and salinity in an Arctic endemic kelp (Laminaria solidungula). J. Phycol. 57, 1554–1562. doi: 10.1111/jpy.13187
Naylor R. L., Hardy R. W., Buschmann A. H., Bush S. R., Cao L., Klinger D. H., et al. (2021). A 20-year retrospective review of global aquaculture. Nature 591, 551–563. doi: 10.1038/s41586-021-03308-6
Nazari-Sharabian M., Ahmad S., Karakouzian M. (2018). Climate change and eutrophication: a short review. Engineering. Technol. Appl. Sci. Res. 8, 3668–3672. doi: 10.48084/etasr.2392
Ndawala M. A., Msuya F. E., Cabarubias J. P., Kambey C. S. B., Buriyo A. S., Mvungi E. F., et al. (2022). Effect of biosecurity practices and diseases on growth and carrageenan properties of Kappaphycus alvarezii and Eucheuma denticulatum cultivated in Zanzibar, Tanzania. J. Appl. Phycol. 34, 3069–3085. doi: 10.1007/s10811-022-02835-7
Nelson D. R., Mystikou A., Jaiswal A., Rad-Menendez C., Preston M. J., De Boever F., et al. (2024). Macroalgal deep genomics illuminate multiple paths to aquatic, photosynthetic multicellularity. Mol. Plant 17, 747–771. doi: 10.1016/j.molp.2024.03.011
Nepper-Davidsen J., Andersen D. T., Pedersen M. F. (2019). Exposure to simulated heatwave scenarios causes long-term reductions in performance in Saccharina latissima. Mar. Ecol. Prog. Ser. 630, 25–39. doi: 10.3354/meps13133
Nielsen M. M., Manns D., D’este M., Krause-Jensen D., Rasmussen M. B., Larsen M. M., et al. (2016). Variation in biochemical composition of Saccharina latissima and Laminaria digitata along an estuarine salinity gradient in inner Danish waters. Algal. Res. 13, 235–245. doi: 10.1016/j.algal.2015.12.003
Nitschke U., Walsh P., Mcdaid J., Stengel D. B. (2018). Variability in iodine in temperate seaweeds and iodine accumulation kinetics of Fucus vesiculosus and Laminaria digitata (Phaeophyceae, Ochrophyta). J. Phycol. 54, 114–125. doi: 10.1111/jpy.2018.54.issue-1
Noisette F., Pansch C., Wall M., Wahl M., Hurd C. L. (2022). Role of hydrodynamics in shaping chemical habitats and modulating the responses of coastal benthic systems to ocean global change. Global Change Biol. 28, 3812–3829. doi: 10.1111/gcb.v28.12
O’Connor M. I. (2009). Warming strengthens an herbivore–plant interaction. Ecology 90, 388–398. doi: 10.1890/08-0034.1
Olischläger M., Iñiguez C., Gordillo F. J. L., Wiencke C. (2014). Biochemical composition of temperate and Arctic populations of Saccharina latissima after exposure to increased pCO2 and temperature reveals ecotypic variation. Planta 240, 1213–1224. doi: 10.1007/s00425-014-2143-x
Oliver E. C. J., Donat M. G., Burrows M. T., Moore P. J., Smale D. A., Alexander L. V., et al. (2018). Longer and more frequent marine heatwaves over the past century. Nat. Commun. 9, 1324. doi: 10.1038/s41467-018-03732-9
Osborne M. G., Molano G., Simons A. L., Dao V., Ong B., Vong B., et al. (2023). Natural variation of Macrocystis pyrifera gametophyte germplasm culture microbiomes and applications for improving yield in offshore farms. J. Phycol. 00, 1–16. doi: 10.1111/jpy.13320
Paine E. R., Britton D., Schmid M., Brewer E. A., Diaz-Pulido G., Boyd P. W., et al. (2023). No effect of ocean acidification on growth, photosynthesis, or dissolved organic carbon release by three temperate seaweeds with different dissolved inorganic carbon uptake strategies. ICES. J. Mar. Sci. 80, 272–281. doi: 10.1093/icesjms/fsac221
Pang T., Liu J., Liu Q., Li H., Li J. (2015). Observations on pests and diseases affecting a eucheumatoid farm in China. J. Appl. Phycol. 27, 1975–1984. doi: 10.1007/s10811-014-0507-z
Park E., Yu H., Lim J.-H., Hee Choi J., Park K.-J., Lee J. (2023). Seaweed metabolomics: A review on its nutrients, bioactive compounds and changes in climate change. Food Res. Int. 163, 112221. doi: 10.1016/j.foodres.2022.112221
Patwary Z. P., Paul N. A., Nishitsuji K., Campbell A. H., Shoguchi E., Zhao M., et al. (2021). Application of omics research in seaweeds with a focus on red seaweeds. Briefings Funct. Genomics 20, 148–161. doi: 10.1093/bfgp/elab023
Pavia H., Toth G. B. (2000). Inducible chemical resistance to herbivory in the brown seaweed Ascophyllum nodosum. Ecology 81, 3212–3225. doi: 10.1890/0012-9658(2000)081[3212:ICRTHI]2.0.CO;2
Pedersen M. F., Borum J. (1996). Nutrient control of algal growth in estuarine waters. Nutrient limitation and the importance of nitrogen requirements and nitrogen storage among phytoplankton and species of macroalgae. Mar. Ecol. Prog. Ser. 142, 261–272. doi: 10.3354/meps142261
Pedersen M. F., Nejrup L. B., Fredriksen S., Christie H., Norderhaug K. M. (2012). Effects of wave exposure on population structure, demography, biomass and productivity of the kelp Laminaria hyperborea. Mar. Ecol. Prog. Ser. 451, 45–60. doi: 10.3354/meps09594
Peng C., Sui Z., Zhou W., Hu Y., Mi P., Jiang M., et al. (2018). Analysis of DNA methylation of Gracilariopsis lemaneiformis under temperature stress using the methylation sensitive amplification polymorphism (MSAP) technique. J. Ocean. Univ. China 17, 623–631. doi: 10.1007/s11802-018-3426-9
Pessarrodona A., Assis J., Filbee-Dexter K., Burrows M. T., Gattuso J.-P., Duarte C. M., et al. (2022). Global seaweed productivity. Sci. Adv. 8, eabn2465. doi: 10.1126/sciadv.abn2465
Peteiro C., Freire Ó. (2012). Observations on fish grazing of the cultured kelps Undaria pinnatifida and Saccharina latissima (Phaeophyceae, Laminariales) in Spanish Atlantic waters. Aquacult. Aquarium. Conserv. Legislation. 5, 189–196.
Peteiro C., Freire Ó. (2013). Biomass yield and morphological features of the seaweed Saccharina latissima cultivated at two different sites in a coastal bay in the Atlantic coast of Spain. J. Appl. Phycol. 25, 205–213. doi: 10.1007/s10811-012-9854-9
Piñeiro-Corbeira C., Barreiro R., Cremades J., Arenas F. (2018). Seaweed assemblages under a climate change scenario: Functional responses to temperature of eight intertidal seaweeds match recent abundance shifts. Sci. Rep. 8, 12978. doi: 10.1038/s41598-018-31357-x
Provasoli L., Pintner I. J. (1980). Bacteria induced polymorphism in an axenic laboratory strain of Ulva lactuca (chlorophyceae)1. J. Phycol. 16, 196–201. doi: 10.1111/j.1529-8817.1980.tb03019.x
Qiu Z., Coleman M. A., Provost E., Campbell A. H., Kelaher B. P., Dalton S. J., et al. (2019). Future climate change is predicted to affect the microbiome and condition of habitat-forming kelp. Proc. R. Soc. B. 286, 20181887. doi: 10.1098/rspb.2018.1887
Raven J., Caldeira K., Elderfield H., Hoegh-Guldberg O., Liss P., Riebesell U., et al. (2005). Ocean acidification due to increasing atmospheric carbon dioxide (London: The Royal Society).
Redway M. L., Combet E. (2023). Seaweed as food: survey of the UK market and appraisal of opportunities and risks in the context of iodine nutrition. Br. Food J. 125, 3601–3622. doi: 10.1108/BFJ-01-2023-0024
Reichert B. K., Schnur R., Bengtsson L. (2002). Global ocean warming tied to anthropogenic forcing. Geophys. Res. Lett. 29, 20–1-20-4. doi: 10.1029/2001GL013954
Ricart A. M., Honisch B., Fachon E., Hunt C. W., Salisbury J., Arnold S. N., et al. (2023). Optimizing marine macrophyte capacity to locally ameliorate ocean acidification under variable light and flow regimes: Insights from an experimental approach. PloS One 18, e0288548. doi: 10.1371/journal.pone.0288548
Rinde E., Christie H., Fagerli C. W., Bekkby T., Gundersen H., Norderhaug K. M., et al. (2014). The influence of physical factors on kelp and sea urchin distribution in previously and still grazed areas in the NE Atlantic. PloS One 9, e100222. doi: 10.1371/journal.pone.0100222
Rioux L.-E., Turgeon S. L., Beaulieu M. (2009). Effect of season on the composition of bioactive polysaccharides from the brown seaweed Saccharina longicruris. Phytochemistry 70, 1069–1075. doi: 10.1016/j.phytochem.2009.04.020
Roleda M. Y., Hurd C. L. (2012). “Seaweed responses to ocean acidification,” in Seaweed Biology. Eds. Wiencke C., Bischof K. (Springer, Berlin, Heidelberg).
Roleda M. Y., Skjermo J., Marfaing H., Jónsdóttir R., Rebours C., Gietl A., et al. (2018). Iodine content in bulk biomass of wild-harvested and cultivated edible seaweeds: Inherent variations determine species-specific daily allowable consumption. Food Chem. 254, 333–339. doi: 10.1016/j.foodchem.2018.02.024
Ross F. W. R., Boyd P. W., Filbee-Dexter K., Watanabe K., Ortega A., Krause-Jensen D., et al. (2023). Potential role of seaweeds in climate change mitigation. Sci. Total. Environ. 885, 163699. doi: 10.1016/j.scitotenv.2023.163699
Satoh Y., Wada S., Hisamatsu S. I. (2019). Seasonal variations in iodine concentrations in a brown alga (Ecklonia cava Kjellman) and a seagrass (Zostera marina L.) in the northwestern Pacific coast of central Japan. J. Oceanogr. 75, 111–117. doi: 10.1007/s10872-018-0479-8
Scheschonk L., Becker S., Hehemann J. H., Diehl N., Karsten U., Bischof K. (2019). Arctic kelp eco-physiology during the polar night in the face of global warming: a crucial role for laminarin. Mar. Ecol. Prog. Ser. 611, 59–74. doi: 10.3354/meps12860
Scheschonk L., Bischof K., Kopp M. E. L., Jueterbock A. (2023). Differences by origin in methylome suggest eco-phenotypes in the kelp Saccharina latissima. Evol. Appl. 16, 262–278. doi: 10.1111/eva.13382
Schiel D. R., Steinbeck J. R., Foster M. S. (2004). Ten years of induced ocean warming causes comprehensive changes in marine benthic communities. Ecology 85, 1833–1839. doi: 10.1890/03-3107
Schoenrock K. M., O’callaghan T., O’callaghan R., Krueger-Hadfield S. A. (2019). First record of Laminaria ochroleuca Bachelot de la Pylaie in Ireland in Béal an Mhuirthead, county Mayo. Mar. Biodivers. Records. 12, 9. doi: 10.1186/s41200-019-0168-3
Sen Gupta A., Thomsen M., Benthuysen J. A., Hobday A. J., Oliver E., Alexander L. V., et al. (2020). Drivers and impacts of the most extreme marine heatwaves events. Sci. Rep. 10, 19359. doi: 10.1038/s41598-020-75445-3
Shalders T. C., Champion C., Benkendorff K., Davis T., Wernberg T., Morris S., et al. (2023). Changing nutritional seascapes of kelp forests. Front. Mar. Sci. 10, 1197468. doi: 10.3389/fmars.2023.1197468
Shalders T. C., Champion C., Coleman M. A., Benkendorff K. (2022). The nutritional and sensory quality of seafood in a changing climate. Mar. Environ. Res. 176, 105590. doi: 10.1016/j.marenvres.2022.105590
Shan T. F., Pang S. J., Li J., Gao S. Q. (2016). Breeding of an elite cultivar Haibao No. 1 of Undaria pinnatifida (Phaeophyceae) through gametophyte clone crossing and consecutive selection. J. Appl. Phycol. 28, 2419–2426. doi: 10.1007/s10811-015-0748-5
Shen Y., Motomura T., Ichihara K., Matsuda Y., Yoshimura K., Kosugi C., et al. (2023). Application of CRISPR-Cas9 genome editing by microinjection of gametophytes of Saccharina japonica (Laminariales, Phaeophyceae). J. Appl. Phycol. 35, 1431–1441. doi: 10.1007/s10811-023-02940-1
Simkanin C., Power A. M., Myers A., Mcgrath D., Southward A., Mieszkowska N., et al. (2005). Using historical data to detect temporal changes in the abundances of intertidal species on Irish shores. J. Mar. Biol. Assoc. United. Kingdom. 85, 1329–1340. doi: 10.1017/S0025315405012506
Smale D. A. (2020). Impacts of ocean warming on kelp forest ecosystems. New Phytol. 225, 1447–1454. doi: 10.1111/nph.v225.4
Smale D. A., Burrows M. T., Evans A. J., King N., Sayer M. D. J., Yunnie A. L. E., et al. (2016). Linking environmental variables with regional-scale variability in ecological structure and standing stock of carbon within UK kelp forests. Mar. Ecol. Prog. Ser. 542, 79–95. doi: 10.3354/meps11544
Smale D. A., Pessarrodona A., King N., Burrows M. T., Yunnie A., Vance T., et al. (2020). Environmental factors influencing primary productivity of the forest-forming kelp Laminaria hyperborea in the northeast Atlantic. Sci. Rep. 10, 12161. doi: 10.1038/s41598-020-69238-x
Smale D. A., Vance T. (2015). Climate-driven shifts in species’ distributions may exacerbate the impacts of storm disturbances on North-east Atlantic kelp forests. Mar. Freshw. Res. 67, 65–74. doi: 10.1071/MF14155
Smale D. A., Wernberg T., Oliver E. C. J., Thomsen M., Harvey B. P., Straub S. C., et al. (2019). Marine heatwaves threaten global biodiversity and the provision of ecosystem services. Nat. Climate Change 9, 306–312. doi: 10.1038/s41558-019-0412-1
Smith K. E., Aubin M., Burrows M. T., Filbee-Dexter K., Hobday A. J., Holbrook N. J., et al. (2024). Global impacts of marine heatwaves on coastal foundation species. Nat. Commun. 15, 5052. doi: 10.1038/s41467-024-49307-9
Smith K. E., Burrows M. T., Hobday A. J., King N. G., Moore P. J., Sen Gupta A., et al. (2023). Biological impacts of marine heatwaves. Annu. Rev. Mar. Sci. 15, 119–145. doi: 10.1146/annurev-marine-032122-121437
Smolina I., Kollias S., Jueterbock A., Coyer J. A., Hoarau G. (2016). Variation in thermal stress response in two populations of the brown seaweed, Fucus distichus, from the Arctic and subarctic intertidal. R. Soc. Open Sci. 3, 150429. doi: 10.1098/rsos.150429
Solas M., Correa R. A., Barría F., Garcés C., Camus C., Faugeron S. (2024). Assessment of local adaptation and outbreeding risks in contrasting thermal environments of the giant kelp, Macrocystis pyrifera. J. Appl. Phycol. 36, 471–483. doi: 10.1007/s10811-023-03119-4
Spurkland T., Iken K. (2011). Salinity and irradiance effects on growth and maximum photosynthetic quantum yield in subarctic Saccharina latissima (Laminariales, Laminariaceae). Botanica Marina. 54, 355–365. doi: 10.1515/bot.2011.042
Stammer D., Martins M. S., Köhler J., Köhl A. (2021). How well do we know ocean salinity and its changes? Prog. Oceanogr. 190, 102478. doi: 10.1016/j.pocean.2020.102478
Stamp T. (2015). “Alaria esculenta forest with dense anemones and crustose sponges on extremely exposed infralittoral bedrock,” in Marine life Information Network: Biology and Sensitivity Key Information Reviews. Eds. Tyler-Walters H., Hiscock K. (Marine Biological Association of the United Kingdom, Plymouth).
Starko S., Soto Gomez M., Darby H., Demes K. W., Kawai H., Yotsukura N., et al. (2019). A comprehensive kelp phylogeny sheds light on the evolution of an ecosystem. Mol. Phylogenet. Evol. 136, 138–150. doi: 10.1016/j.ympev.2019.04.012
Steneck R. S., Graham M. H., Bourque B. J., Corbett D., Erlandson J. M., Estes J. A., et al. (2002). Kelp forest ecosystems: biodiversity, stability, resilience and future. Environ. Conserv. 29, 436–459. doi: 10.1017/S0376892902000322
Stévant P., Rebours C., Chapman A. (2017). Seaweed aquaculture in Norway: recent industrial developments and future perspectives. Aquacult. Int. 25, 1373–1390. doi: 10.1007/s10499-017-0120-7
Strittmatter M., Murúa P., Arce P., Perrineau M.-M., Gachon C. (2022). My seaweed looks weird: a community web portal to accelerate pathogen discovery in seaweeds. Appl. Phycol. 3, 300–305. doi: 10.1080/26388081.2022.2059783
Subasinghe R., Soto D., Jia J. (2009). Global aquaculture and its role in sustainable development. Rev. Aquacult. 1, 2–9. doi: 10.1111/j.1753-5131.2008.01002.x
Sultana F., Wahab M. A., Nahiduzzaman M., Mohiuddin M., Iqbal M. Z., Shakil A., et al. (2023). Seaweed farming for food and nutritional security, climate change mitigation and adaptation, and women empowerment: A review. Aquacult. Fisheries. 8, 463–480. doi: 10.1016/j.aaf.2022.09.001
Sun P., Mao Y., Li G., Cao M., Kong F., Wang L., et al. (2015). Comparative transcriptome profiling of Pyropia yezoensis (Ueda) M.S. Hwang & H.G. Choi in response to temperature stresses. BMC Genomics 16, 463. doi: 10.1186/s12864-015-1586-1
Suresh Kumar K., Ganesan K., Subba Rao P. V. (2015). Seasonal variation in nutritional composition of Kappaphycus alvarezii (Doty) Doty—an edible seaweed. J. Food Sci. Technol. 52, 2751–2760. doi: 10.1007/s13197-014-1372-0
Taise A., Krieger E., Bury S. J., Cornwall C. E. (2023). Physiological responses of Caulerpa spp. (with different dissolved inorganic carbon physiologies) to ocean acidification. New Z. J. Bot. 19, 1–25. doi: 10.1080/0028825X.2023.2289432
Tait L. W., Thoral F., Pinkerton M. H., Thomsen M. S., Schiel D. R. (2021). Loss of Giant Kelp, Macrocystis pyrifera, driven by marine heatwaves and exacerbated by poor water clarity in New Zealand. Front. Mar. Sci. 8, 721087. doi: 10.3389/fmars.2021.721087
Tamarin-Brodsky T., Kaspi Y. (2017). Enhanced poleward propagation of storms under climate change. Nat. Geosci. 10, 908–913. doi: 10.1038/s41561-017-0001-8
Tan T.-T., Song S.-L., Poong S.-W., Ward G. M., Brodie J., Lim P.-E. (2020). The effect of grazing on the microbiome of two commercially important agarophytes, Gracilaria firma and G. salicornia (Gracilariaceae, Rhodophyta). J. Appl. Phycol. 32, 2549–2559. doi: 10.1007/s10811-020-02062-y
Taylor R. B., Sotka E., Hay M. E. (2002). Tissue-specific induction of herbivore resistance: seaweed response to amphipod grazing. Oecologia 132, 68–76. doi: 10.1007/s00442-002-0944-2
Thomsen M. S., Wernberg T., Kendrick G. A. (2004). The effect of thallus size, life stage, aggregation, wave exposure and substratum conditions on the forces required to break or dislodge the small kelp Ecklonia radiata. Botanica Marina. 47, 454–460. doi: 10.1515/BOT.2004.068
Timmermans M.-L., Marshall J. (2020). Understanding Arctic Ocean circulation: A review of ocean dynamics in a changing climate. J. Geophys. Res.: Oceans. 125, e2018JC014378. doi: 10.1029/2018JC014378
Timperio A. M., Egidi M. G., Zolla L. (2008). Proteomics applied on plant abiotic stresses: Role of heat shock proteins (HSP). J. Proteomics 71, 391–411. doi: 10.1016/j.jprot.2008.07.005
Toth G. B., Karlsson M., Pavia H. (2007). Mesoherbivores reduce net growth and induce chemical resistance in natural seaweed populations. Oecologia 152, 245–255. doi: 10.1007/s00442-006-0643-5
Trujillo E., Monreal-Escalante E., Ramos-Vega A., Angulo C. (2024). Macroalgae: Marine players in vaccinology. Algal. Res. 78, 103392. doi: 10.1016/j.algal.2024.103392
Valero M., Guillemin M.-L., Destombe C., Jacquemin B., Gachon C. M., Badis Y., et al. (2017). Perspectives on domestication research for sustainable seaweed aquaculture. Perspect. Phycol. 4, 33–46. doi: 10.1127/pip/2017/0066
van der Loos L. M., Eriksson B. K., Falcão Salles J. (2019a). The macroalgal holobiont in a changing sea. Trends Microbiol. 27, 635–650. doi: 10.1016/j.tim.2019.03.002
van der Loos L. M., Schmid M., Leal P. P., Mcgraw C. M., Britton D., Revill A. T., et al. (2019b). Responses of macroalgae to CO2 enrichment cannot be inferred solely from their inorganic carbon uptake strategy. Ecol. Evol. 9, 125–140. doi: 10.1002/ece3.2019.9.issue-1
van der Molen J., Ruardij P., Mooney K., Kerrison P., O’connor N. E., Gorman E., et al. (2018). Modelling potential production of macroalgae farms in UK and Dutch coastal waters. Biogeosciences 15, 1123–1147. doi: 10.5194/bg-15-1123-2018
Veenhof R. J., Champion C., Dworjanyn S. A., Shalders T. C., Coleman M. A. (2023). Reproductive phenology of the kelp Ecklonia radiata at its Australian warm range edge and the influence of environmental factors. Mar. Freshw. Res. 74, 928–940. doi: 10.1071/MF22259
Veenhof R. J., Coleman M. A., Champion C., Dworjanyn S. A., Venhuizen R., Kearns L., et al. (2024). Novel high-throughput oxygen saturation measurements for quantifying the physiological performance of macroalgal early life stages. J. Phycol, 1–12 doi: 10.1111/jpy.13489
Vergés A., Campbell A. H., Wood G., Kajlich L., Eger A. M., Cruz D., et al. (2020). Operation Crayweed: Ecological and sociocultural aspects of restoring Sydney’s underwater forests. Ecol. Manage. Restor. 21, 74–85. doi: 10.1111/emr.12413
Vergés A., Doropoulos C., Malcolm H. A., Skye M., Garcia-Pizá M., Marzinelli E. M., et al. (2016). Long-term empirical evidence of ocean warming leading to tropicalization of fish communities, increased herbivory, and loss of kelp. Proc. Natl. Acad. Sci. 113, 13791–13796. doi: 10.1073/pnas.1610725113
Vergés A., Steinberg P. D., Hay M. E., Poore A. G. B., Campbell A. H., Ballesteros E., et al. (2014). The tropicalization of temperate marine ecosystems: climate-mediated changes in herbivory and community phase shifts. Proc. R. Soc. B. 281, 20140846. doi: 10.1098/rspb.2014.0846
Villarini G., Vecchi G. A. (2012). Twenty-first-century projections of North Atlantic tropical storms from CMIP5 models. Nat. Climate Change 2, 604–607. doi: 10.1038/nclimate1530
Visch W., Layton C., Hurd C. L., Macleod C., Wright J. T. (2023). A strategic review and research roadmap for offshore seaweed aquaculture—A case study from southern Australia. Rev. Aquacult. 15, 1467–1479. doi: 10.1111/raq.12788
Wade R., Augyte S., Harden M., Nuzhdin S., Yarish C., Alberto F. (2020). Macroalgal germplasm banking for conservation, food security, and industry. PloS Biol. 18, e3000641. doi: 10.1371/journal.pbio.3000641
Wahl M., Werner F. J., Buchholz B., Raddatz S., Graiff A., Matthiessen B., et al. (2020). Season affects strength and direction of the interactive impacts of ocean warming and biotic stress in a coastal seaweed ecosystem. Limnol. Oceanogr. 65, 807–827. doi: 10.1002/lno.11350
Wang W., Li X., Liang G., Zhao N., Shi L., Yang G. (2021). Description of a white spot disease of field cultivated kelp (Saccharina japonica) and evaluation of its influences on the growth and phytochemical contents. Aquat. Bot. 173, 103414. doi: 10.1016/j.aquabot.2021.103414
Wang X., Yao J., Zhang J., Duan D. (2020). Status of genetic studies and breeding of Saccharina japonica in China. J. Oceanol. Limnol. 38, 1064–1079. doi: 10.1007/s00343-020-0070-1
Ward G. M., Faisan J. P. Jr., Cottier-Cook E. J., Gachon C., Hurtado A. Q., Lim P. E., et al. (2020). A review of reported seaweed diseases and pests in aquaculture in Asia. J. World Aquacult. Soc. 51, 815–828. doi: 10.1111/jwas.12649
Ward G. M., Kambey C. S. B., Faisan J. P. Jr., Tan P.-L., Daumich C. C., Matoju I., et al. (2022). Ice-Ice disease: An environmentally and microbiologically driven syndrome in tropical seaweed aquaculture. Rev. Aquacult. 14, 414–439. doi: 10.1111/raq.12606
Wernberg T., Thomsen M. S., Baum J. K., Bishop M. J., Bruno J. F., Coleman M. A., et al. (2023). Impacts of climate change on marine foundation species. Annu. Rev. Mar. Sci. 16, 13–59. doi: 10.1146/annurev-marine-042023-093037
Wiencke C., Amsler C. D. (2012). “Seaweeds and their communities in polar regions,” in Seaweed Biology: Novel Insights into Ecophysiology, Ecology and Utilization. Eds. Wiencke C., Bischof K. (Springer Berlin Heidelberg, Berlin, Heidelberg).
Williams C., Rees S., Sheehan E. V., Ashley M., Davies W. (2022). Rewilding the sea? A rapid, low cost model for valuing the ecosystem service benefits of kelp forest recovery based on existing valuations and benefit transfers. Front. Ecol. Evol. 10, 642775. doi: 10.3389/fevo.2022.642775
Wilson K. L., Skinner M. A., Lotze H. K. (2019). Projected 21st-century distribution of canopy-forming seaweeds in the Northwest Atlantic with climate change. Diversity Distrib. 25, 582–602. doi: 10.1111/ddi.2019.25.issue-4
Wolf J., Woolf D., Bricheno L. (2020). Impacts of climate change on storms and waves relevant to the coastal and marine environment around the UK. MCCIP. Sci. Rev. 2020, 132–157. doi: 10.14465/2020.arc07.saw
Wood D., Capuzzo E., Kirby D., Mooney-Mcauley K., Kerrison P. (2017). UK macroalgae aquaculture: What are the key environmental and licensing considerations? Mar. Policy 83, 29–39. doi: 10.1016/j.marpol.2017.05.021
Wood G., Marzinelli E. M., Coleman M. A., Campbell A. H., Santini N. S., Kajlich L., et al. (2019). Restoring subtidal marine macrophytes in the Anthropocene: trajectories and future-proofing. Mar. Freshw. Res. 70, 936–951. doi: 10.1071/MF18226
Woollings T., Gregory J. M., Pinto J. G., Reyers M., Brayshaw D. J. (2012). Response of the North Atlantic storm track to climate change shaped by ocean–atmosphere coupling. Nat. Geosci. 5, 313–317. doi: 10.1038/ngeo1438
Xiao X., Agustí S., Yu Y., Huang Y., Chen W., Hu J., et al. (2021). Seaweed farms provide refugia from ocean acidification. Sci. Total. Environ. 776, 145192. doi: 10.1016/j.scitotenv.2021.145192
Xu D., Brennan G., Xu L., Zhang X. W., Fan X., Han W. T., et al. (2019). Ocean acidification increases iodine accumulation in kelp-based coastal food webs. Global Change Biol. 25, 629–639. doi: 10.1111/gcb.2019.25.issue-2
Yong W. T. L., Thien V. Y., Rupert R., Rodrigues K. F. (2022). Seaweed: A potential climate change solution. Renewable Sustain. Energy Rev. 159, 112222. doi: 10.1016/j.rser.2022.112222
Young C. S., Sylvers L. H., Tomasetti S. J., Lundstrom A., Schenone C., Doall M. H., et al. (2022). Kelp (Saccharina latissima) mitigates coastal ocean acidification and increases the growth of North Atlantic bivalves in lab experiments and on an oyster farm. Front. Mar. Sci. 9, 881254. doi: 10.3389/fmars.2022.881254
Yu C.-H., Lim P.-E., Phang S.-M. (2013). Effects of irradiance and salinity on the growth of carpospore-derived tetrasporophytes of Gracilaria edulis and Gracilaria tenuistipitata var liui (Rhodophyta). J. Appl. Phycol. 25, 787–794. doi: 10.1007/s10811-012-9960-8
Keywords: seaweed aquaculture, climate change, breeding, ocean warming, salinity, restorative aquaculture, site selection, omics
Citation: Veenhof RJ, Burrows MT, Hughes AD, Michalek K, Ross ME, Thomson AI, Fedenko J and Stanley MS (2024) Sustainable seaweed aquaculture and climate change in the North Atlantic: challenges and opportunities. Front. Mar. Sci. 11:1483330. doi: 10.3389/fmars.2024.1483330
Received: 19 August 2024; Accepted: 01 October 2024;
Published: 22 October 2024.
Edited by:
Samuel Starko, University of Victoria, CanadaCopyright © 2024 Veenhof, Burrows, Hughes, Michalek, Ross, Thomson, Fedenko and Stanley. This is an open-access article distributed under the terms of the Creative Commons Attribution License (CC BY). The use, distribution or reproduction in other forums is permitted, provided the original author(s) and the copyright owner(s) are credited and that the original publication in this journal is cited, in accordance with accepted academic practice. No use, distribution or reproduction is permitted which does not comply with these terms.
*Correspondence: Reina J. Veenhof, cmVpbmEudmVlbmhvZkBzYW1zLmFjLnVr