- 1School of Ocean Sciences, China University of Geosciences, Beijing, China
- 2Key Laboratory of Polar Geology and Marine Mineral Resource (China University of Geosciences, Beijing), Ministry of Education, Beijing, China
- 3Key Laboratory of Marine Ecosystem and Biogeochemistry, Second Institute of Oceanography, Ministry of Natural Resources, Hangzhou, China
- 4National Deep Sea Center, Qingdao, China
The ecological implications of deep-sea mining, particularly the considerable discharge of suspended sediments during operational processes, have attracted substantial concerns. In order to reveal the metal regeneration dynamics in the polymetallic nodule area of the western Pacific Ocean, ex-situ sediment disturbance experiments were conducted on a research vessel. After two levels of regulated stirring disturbance were exerted for half an hour, the concentrations of 12 dissolved metals and physicochemical parameters, including Eh and pH, were monitored continuously in the overlying water for three days. Porewater samples were also collected at the starting and ending time of each experiment to detect the change of dissolved metal profiles within the sediments. The findings revealed that the sediment disturbance led to fluctuations in the concentrations of metals in the overlying water, with manganese exhibiting the most pronounced change at a coefficient of 208%. The temporal patterns of dissolved metal concentrations demonstrated a coherent behavior among certain metals, such as Li, V, Co, Ni, Rb, Mo, and Cs, which displayed an overall increase, ultimately surpassing the initial concentrations in the overlying water. In line with this, the metal concentration profiles in the porewater were also elevated. Spearman correlation analysis confirmed the synchronized behavior of these metals. The results suggested that the metals mobility might be governed by physicochemical factors in the overlying water. Moreover, the sedimentary features, such as grain size composition, and the morphological state of metals in sediments played pivotal roles in the differential responses of metal groups to sediment disturbance across stations. Conversely, the disturbance intensity was found to have a relatively minor impact on the dissolved metal behavior. The findings from the ex-situ experiments provided critical insights for predicting metal regeneration related to deep-sea mining, which are expected to be validated through rigorous monitoring protocols during future in-situ mining trials.
1 Introduction
Oceanic ferromanganese nodules are typical components of authigenic polymetallic deposits formed by the accretion of hydrated Mn and Fe hydroxide in deep ocean (Ren et al., 2024). They are scattered across the seafloor under diverse and often indistinct geological conditions, which have been identified as a promising alternative for strategic resources, particularly enriched in copper, nickel, and cobalt (Mero, 1965; Glasby, 1977; Amann, 1982; Petersen et al., 2016). Regional mapping showed that hydrogenetic nodules are distributed in the western and southern Pacific at low biological productivity sites, while diagenetic nodules are distributed in the eastern Pacific (Ren et al., 2024). Nonetheless, deep-sea mining operation on these ore deposits has profound impacts on the marine ecosystem (Amos and Roels, 1977; Ozturgut et al., 1978; Nath et al., 2001). The primary disruptions associated with deep-sea mining include (1) the wholesale excavation of surface sediments and the destruction of benthic communities, (2) localized elevations in suspended particulate matter, (3) crushing damage from tailings and overburden, and (4) enhanced toxicity resulting from metal release under high-pressure conditions (Li et al., 2006). When mining activities induce disturbances, elements in both solute and particulate forms may be introduced into the water column through the emergent plume, subsequent dispersal by ocean currents, and ultimate resettlement (Saulnier and Mucci, 2000). Under certain physicochemical conditions, dissolved metal elements may also be scavenged in the particulate or colloidal adsorption processes. These disturbances on bottom sediments can also trigger changes in geochemical attributes and biochemical conditions (Raghukumar et al., 2001), associated with the relocation of metals, which poses latent risks to benthic communities due to their toxicity and resistance to biodegradation (Caroppo et al., 2006; Duman et al., 2007; Varol and Sen, 2012; Drazen et al., 2020). Given the high mobility and bioavailability of these metals, their residence in the water column is critically concerning (Ankley et al., 1996).
Identifying the metal release linked to deep-sea sediment plumes is essential for fostering ecofriendly mining practices. Although the vertical distribution of porewater metals and their diffusion fluxes at the water–sediment interface in the polymetallic nodule area of western Pacific were investigated in our previous study (Yang et al., 2023), their response to sediment disturbance remained elusive. Since the 1970s, numerous experiments simulating deep-sea floor mining or sediment disturbance have been conducted (Burns et al., 1980; Thiel and Tiefsee-Umweltschutz, 2001; Volz et al., 2020), with the majority of research focusing on the impacts of substrate removal or deposition processes. According to the longest recolonization experiment to date, DISCOL, the potential short-term impacts of deep-sea mining activities on the geochemical system included the introduction of oxygen diffusion into previously hypoxic deposits, changes in redox conditions, and the acceleration of metal release and regeneration. The long-term impacts based on the observation over 26 years indicated that the recovery of highly active surface deposits could be protracted for millennia (Volz et al., 2020). However, the current findings are insufficient to provide validated data to evaluate the potential dispersion of toxic chemicals or nutrients associated with sediment disturbance during the mining operation. Recent numerical dispersion models to simulate the dispersion process of plumes during in-situ experiments as well as the concentration distribution of sediments over short durations (from several hours to months) suggested that plume dispersion may be limited by factors such as flocculation, background turbidity, and internal tides (Morato et al., 2022; Spearman et al., 2020). Nevertheless, the release and restoration of toxic chemicals in solution due to plume dispersion remain poorly understood.
In general, the increase in metal concentrations in the overlying water following sediment disturbance can be attributed to three primary factors: the release of pre-existing dissolved substances from the sediments, desorption from the sediment matrix, and the dissolution of suspended particles within the water column (Kalnejais et al., 2010). Previous ex-situ resuspension experiments conducted on contaminated coastal sediments have suggested that the released metals can be classified into distinct groups according to their properties, such as the dissolved or weakly adsorbed species, those bound within the crystalline lattice of minerals, or those incorporated in various amorphous solid compounds (Calmano et al., 1993). For example, metals with a high affinity for iron oxide [FeOOH(s)] are prone to be desorbed during the reduction of iron oxide. The environmental conditions encountered by suspended particles can also influence the processes of desorption or dissolution, such as scavenging processes during resuspension (Morin and Morse, 1999; Saulnier and Mucci, 2000; Kalnejais et al., 2010). The properties of overlying water, including salinity, pH, and redox conditions, in conjunction with sedimentation rates, ultimately govern the residence time of these metals in the water column (Martino et al., 2002; Kiratli and Ergin, 1996; Roberts, 2012).
In order to quantify the behavior of dissolved metals throughout the processes of suspension and resettlement, sediment disturbance experiments were conducted on board by using deep-sea sediments and bottom water derived from the polymetallic nodule area in the western Pacific. The study aims to elucidate (1) the short-term response of various metals in the overlying water and sediment porewater after the sediment disturbance and (2) the impacts of physicochemical condition change after the sediment disturbance on the behavior of these metals. The findings are anticipated to provide more definitive evidence to assess the potential ecological risk associated with deep-sea mining.
2 Materials and methods
2.1 Source of sediment samples
Sediments were derived from the undisturbed surface sediments from two box corer sampling stations (BC04 and BC06) during the investigation on the 56th COMRA cruise in September 2019. The stations were located in the intermountain basin of the Marcus-Wake Seamounts Cluster, Northwest Pacific, with information presented in Table 1. Ferromanganese nodules are well developed in the study area (Machida et al., 2016; Ren et al., 2022).
2.2 Procedure of the ex-situ sediment disturbance experiments
After over 2 L of overlying water was sampled from the box corer, the rest of the overlying water was removed. Polymetallic nodules were collected before four plastic tubes with sealed holes on the walls were used to collect sediment cores.
The four parallel sediment cores were separated into two groups. One group was used for the sediment disturbance experiments and measurement on the change of overlying water and porewater properties after the disturbance, while the other group was used for the measurement on the porewater and sedimentary properties before the experiment.
As shown in Figure 1, the two parallel sediment cores were fixed and associated with the stirring devices, which were set at different levels of sediment disturbance. In order to minimize the interference of the experimental device, part of the equipment below the surface water level was made of nonmetallic material. The propellers were located 3 cm above the sediment surface to generate turbulence within the sediment tubes. A total of 1 L of the collected overlying water was filtered through 0.45-μm mesh filters and slowly injected into each sediment tube, respectively. Before the stirring began, the two sediment tubes with overlying water were allowed to stand for 30 min for equilibration. Each set of experiment lasted for 3 days with no more interruption from stormy weather or other induced rocking disturbance in the interim.
Two levels of stirring speed of the propeller working for half an hour at each station were set to simulate the process of sediment disturbance. The experiment was based on the assumption that the shear stress at the sediment–water interface was proportional to the stirring speed, which was 100 ± 5 and 600 ± 5 rpm, respectively, both beyond the erosion threshold of shear stress.
After the regulated disturbance ended and standing for a while, the overlying water was sampled temporally by using a syringe connected with an acid-cleaned hose dipping in the overlying water but 5 cm above the turbid water layer. In the meantime, Eh and pH were doubly measured by using Eh and pH meters (InnoORP 100, InnopH, TWINNO), and the thickness of the turbid water layer was recorded after the propeller stopped. The sampling intervals were before the disturbance and after the disturbance for 15, 30, 60, 2, 6, 12, 24, and 72 h. The volume of each overlying water sample was 10 mL, with a total water volume accounting for approximately 10% of the overlying water volume. The overlying water samples were filtered through a 0.45-μm syringe filter membrane before storing them in acid-cleaned polyethylene centrifuge tubes below -20°C.
After the completion of sampling and monitoring in the overlying water, porewater samples were collected immediately by using Rhizon soil solution collectors (model CSS 5 cm, Rhizosphere), which were inserted into the sampling tube through the sidewall drainage holes for syringe vacuum collection. The sampling depth was approximately 20 cm below the sediment surface, with intervals of 1 cm in the upper layers and 2 to 3 cm in the lower layers. The porewater was pumped in vacuum syringes within 2 to 3 hours. Afterward, they were capped with no air residue and stored at -20°C in a refrigerator. For comparison in the physicochemical properties between stations before the experiments, the porewater was also sampled with one replicated sediment core with no experimental disturbance.
2.3 Sediment and porewater measurement
In the other parallel sediment core with no experimental disturbance, the sediment core was segmented and divided into three parts to measure the morphological partitioning of metals, total organic carbon content (TOC), and grain size composition. Beforehand, the profile of Eh and pH were doubly measured by inserting the probes into the sediments through the sealed holes on the side wall of the plastic tube.
The different extractable fractionations of metals were obtained by using the European Commission-proposed BCR sequential extraction procedure (Rauret et al., 2000). 1 g of sediment sample was placed into 50-mL PE tubes, following the four sequential steps of BCR protocol (Ates et al., 2020). A blank sample with no sediment was carried through the complete procedure with each batch of extraction. Finally, the four extracted states underwent individual measurements by the ICP-MS method. The standard lake sediment, BCR 701 (European Commission, Joint Research Centre), was used to check the accuracy of the fraction analysis. The concentration values of the first three fractions of BCR 701 demonstrated a good consistency with the reference values. In addition, the total recovery with the sequential extraction procedure was calculated, ranging from 93% to 108%. Notably, the weak-acid-soluble state was electrostatically adsorbed onto the surfaces of soil and sediment particles or sequestered within carbonate matrices, facilitating its straightforward release through ion exchange processes. The reducible state, on the other hand, primarily consisted of elements adsorbed by iron oxide, manganese oxide, and similar compounds, with their release contingent upon alterations in redox conditions. Elements in the oxidizable state were predominantly integrated with the active moieties of organic matter or sulfides, capable of being regenerated during oxidative transformations. Lastly, the residual state, unyielded by extraction, housed elements that remained steadfast, predominantly embodied within the silicate lattice (Tessier et al., 1979; Singh et al., 2005; Fan et al., 2002).
The dissolved elements (Li, V, Co, Ni, Rb, Mo, Cs, Cd, Mn, Cu, Zn, and Pb) in porewater samples and those extractable fractionations of metals in sediment samples were determined by using inductively coupled plasma-mass spectrometry (ICP-MS, NexlON300D), following the standard procedure of MNR (Ministry of Natural Resources, 2021). The standard reference solutions were IV-ICPMS-71 A, IV-ICPMS-71 B, and MSLI-10PPM (Inorganic Ventures, Inc.). Four concentration levels (5, 10, 50, and 100 μg/L) of the standard solutions with 2% HNO3 were used to fit the calibration curves. Repeated measurements on the standard of 10 μg/L were performed to control the relative error within ±5%. The reference materials, including freshwater SLRS-6 and seawater NASS-7, were used for quality control, with recoveries from 85% to 108%.
The total organic carbon content in sediment cores was determined by using the TOC Analyzer (vario TOC cube, Elememtar) according to the standard procedure. After the samples were weighed in silver cups, they were acidified with 1 mol/L HCl to remove carbonate and dried at 45°C before the measurement. The standard material GBW07430 (GSS-16) was used for accuracy control within ±<0.01%.
Grain size analysis for sediment cores was measured by using the Mastersizer 2000 instrument. Before the measurement, 30% (V/V) H2O2 was added to remove the organic matter from the sediment sample, and 1 mol/L HCl was added to remove the carbonate (shell fragments). The distribution in a graphic parameter of mean size (Mz) is described based on φ values in Equation (1) (McManus, 1988).
where D is the particle diameter (unit: μm). The higher the value of φ, the finer the grains in size.
The concentrations of SO42- and Cl- in porewater and overlying water were determined by ion chromatography (En-047). The measurement procedure followed the standard protocol (Ministry of Environmental Protection, 2016). Before the measurement, 1-mL water samples were diluted 10–100-fold with ultrapure water according to the concentration. The ultrapure water for blank samples and the standards were also measured repeatedly to ensure the accuracy of measurement. NH4+ concentration in porewater samples was determined by using a spectrophotometer (HACH DR2800). Based on duplicate measurement, the relative error of analysis was within ±5%.
3 Results
3.1 Comparison of the physicochemical environment properties of the stations
As shown in Table 2, the features of overlying water from the two different stations were similar before sediment disturbance. The Eh of BC04 was slightly lower than that of BC06; however, the pH was slightly higher. The experiments were carried out under room temperature and aerobic conditions.
As shown in Figure 2, the vertical tendencies of Eh and pH at the two stations were similar as well. The pH ranged between 7.26 and 8.24, with the maximum in the overlying water, and remained low in the deep. In contrast, the Eh ranged between 240 and 340 mV, with the minimum in the overlying water and surface sediment.
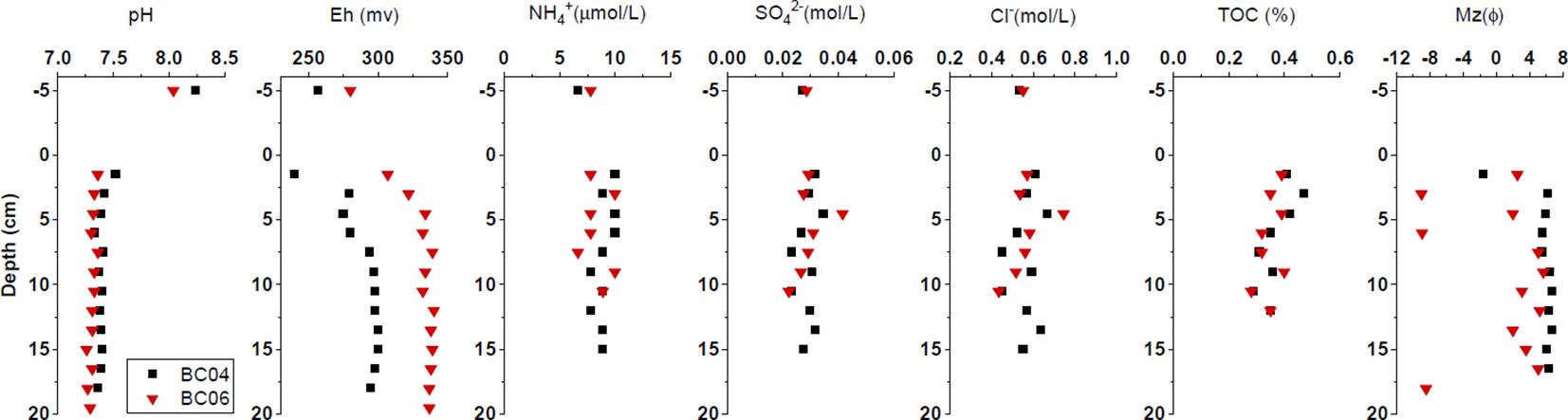
Figure 2. Vertical profiles of physicochemical parameters in porewater or sediments at stations BC04 and BC06.
The vertical trends of Cl- and SO42- and NH4+ concentrations in overlying water and porewater fluctuated similarly between stations (see Figure 2). The range was between 0.44 and 0.75 mol/L for Cl-, between 0.022 and 0.042 mol/L for SO42-, and between 6.7 and 10.0 μmol/L for NH4+. The maximum concentration of both Cl- and SO42- occurred at 5 cm below the sediment surface.
Figure 2 also shows similar profiles of the TOC in sediments, ranging between 0.28% and 0.47% and decreasing with depth at both stations. The mean grain size (Mz) at station BC04 was coarse in the surface sediment and remained fine below the depth of 2 cm, while the variation in mean grain size (Mz) at station BC06 was more vivid, coarser above the depth of 5 cm as well as below the depth of 17 cm. A more fluctuant hydrodynamic condition was suggested in station BC06 in comparison with station BC04.
The morphological partitioning of metals often reflected the mobility and bioavailability of metals in sediments. Figure 3 shows that the compositions in different states were relatively stable with depths and similar for specific metals at both stations. The predominant state was the residual for Li, V, Rb, Mo, and Cs, with an extremely high proportion (>85%), suggesting very limited mobility and bioavailability of these metals during the resuspension process. Moreover, Li had a relatively higher proportion in the oxidable state, which was potentially bound to organic matter.
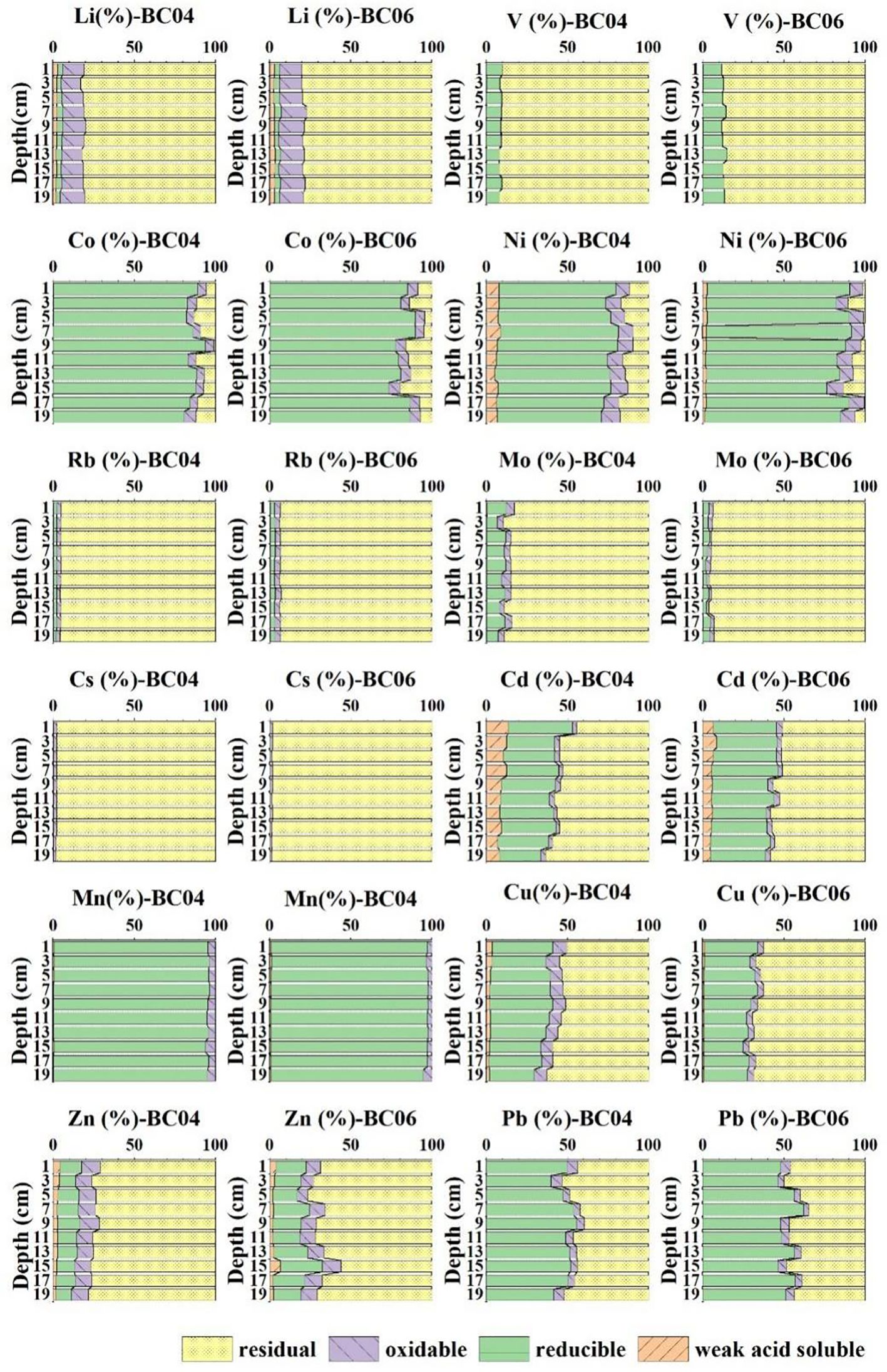
Figure 3. Partitioning of metals (Li, V, Co, Ni, Rb, Mo, Cd, Cs, Mn, Cu, Zn, and Pb) in sediments, including weakly acid-soluble, reducible, oxidable, and residual states.
Meanwhile, the proportion in the reducible state in sediments was relatively high for Co, Ni, Mn, Cd, Cu, and Pb, with a reducible/oxidable ratio of more than 9:1. The high content of the reducible state, especially for Co, Ni, and Mn, also indicated the potential increase of their mobility and bioavailability with the decrease of redox.
The proportion of the weakly acid soluble in all metals was extremely low. Meanwhile, the slight increase with depth in the residual state proportion for many metals also indicated the decrease of metal immobilization at both stations.
3.2 Temporal variation of physicochemical properties in the overlying water after the sediment disturbance
Similar fluctuating trends of Eh and pH were found under different levels of disturbance at both stations (see Figure 4). After the sediment disturbance terminated, Eh increased in the first 30 min and remained stable before decreasing 12 h later at station BC04. In contrast, the Eh value increased rapidly in the first 15 min and remained stable before decreasing 6 h later at station BC06. At both stations, the Eh values were high, ranging between 230 and 330 mV. Moreover, the Eh after the stirring disturbance under 600 rpm was generally higher than that under 100 rpm.
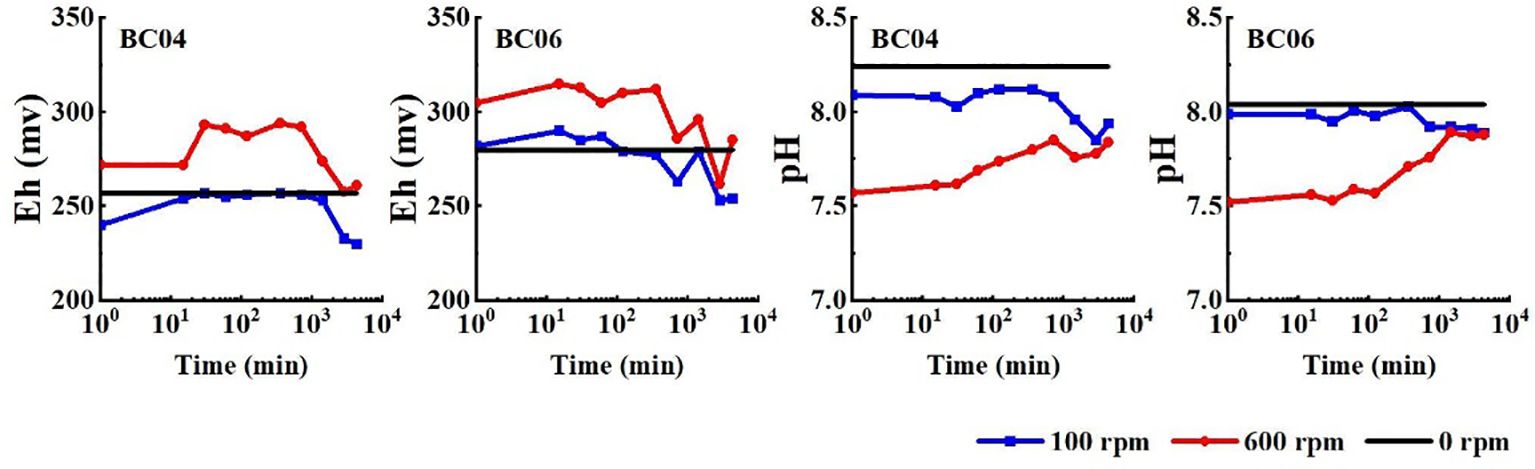
Figure 4. Temporal changes in pH and Eh in the overlying water at different disturbance levels and stations. Time intervals include 0 min (before the disturbance started) and 15, 30, 60, 120, 360, 720, 1,440, 2,880, and 4,320 min after the disturbance.
The trend of the pH values after sediment disturbance was also distinct under different stirring levels but similar at both stations. The variation of pH ranged between 7.8 and 8.2, showing a stronger decrease under the higher level of disturbance but gradually returning to the original level in the end of the experiments. In contrast, the pH at a low level of disturbance remained stable but decreased slightly with time after the disturbance terminated. The final pH values under both levels of disturbance were similar but lower than the initial pH at both stations.
3.3 Concentration variation of dissolved metals in the overlying water after the sediment disturbance
As shown in Figure 5, the concentrations of dissolved metals in the overlying water generally changed with time. According to their response to the disturbance, most metal concentrations increased gradually or fluctuated vividly, with final concentrations in the overlying water higher than the original concentrations. The elevated concentrations were higher under 600 rpm than under 100 rpm, such as Li, V, Co, Ni, Rb, Mo, Cs, and Cd. Other metals, such as Mn and Zn, were characterized as the metal concentrations below the initial concentration after the disturbance, fluctuating and finally returning to the initial concentrations. Although Cu and Pb demonstrated to be relatively stable after the disturbance, their concentrations could be also agitated at an early stage with a higher concentration under 600 rpm at station BC04. Moreover, the high scavenging capacity of suspended particles was also confirmed for metals such as Mn, Zn, Cu, and Pb, whose concentrations could decline below pre-disturbance levels or return to the equilibrium rapidly. When considering the resilience to the sediment disturbance, these metals performed better in comparison with the first group of metals.
Spearman correlation analysis on the temporal concentrations of dissolved metals in the overlying water demonstrated that highly positive correlations occurred between metals such as Li, V, Co, Ni, Rb, Mo, Cs, and Cu, especially under 100-rpm disturbance at BC04 and 600-rpm disturbance at BC06 (See Figures 6 and 7). In contrast, metals such as Mn, Zn, and Pb showed an insignificant correlation with the metals above. The exception was Cd, which was positively correlated with the first group of metals under 100-rpm disturbance at BC04 but negatively correlated with them under 100-rpm disturbance at BC06. Moreover, the temporal pH values were significantly negatively correlated with the concentrations of metals such as Li, Rb, Mo, Cs, and Cu under 100-rpm disturbance at BC06 but positively correlated with Cd under 600 rpm at BC04. It is worth noting that the temporal Eh showed an obviously negative correlation with Mn under 100/600-rpm disturbance at BC06, reflecting a high redox sensitivity of Mn.
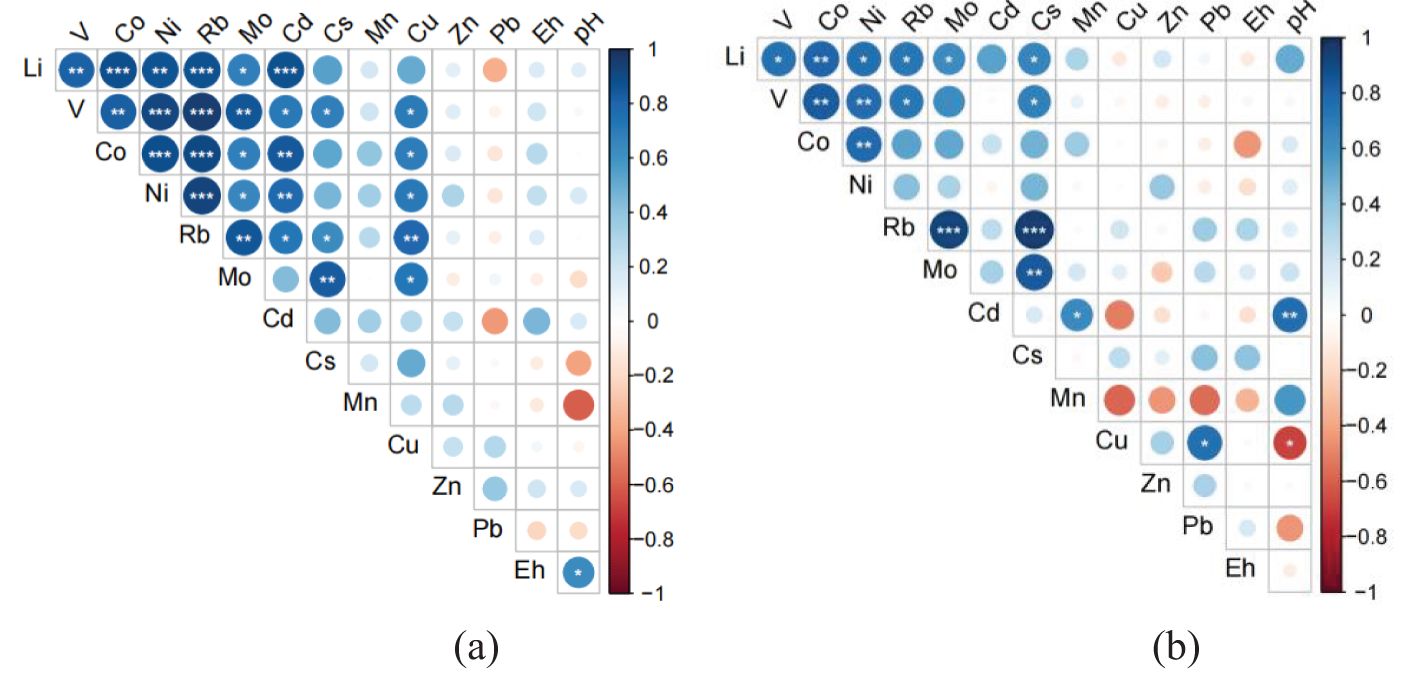
Figure 6. Spearman correlations between metal elements of different disturbance intensities at station BC04. (A) 100-rpm disturbance, (B) 600-rpm disturbance. The number of “*” denotes different significance levels.
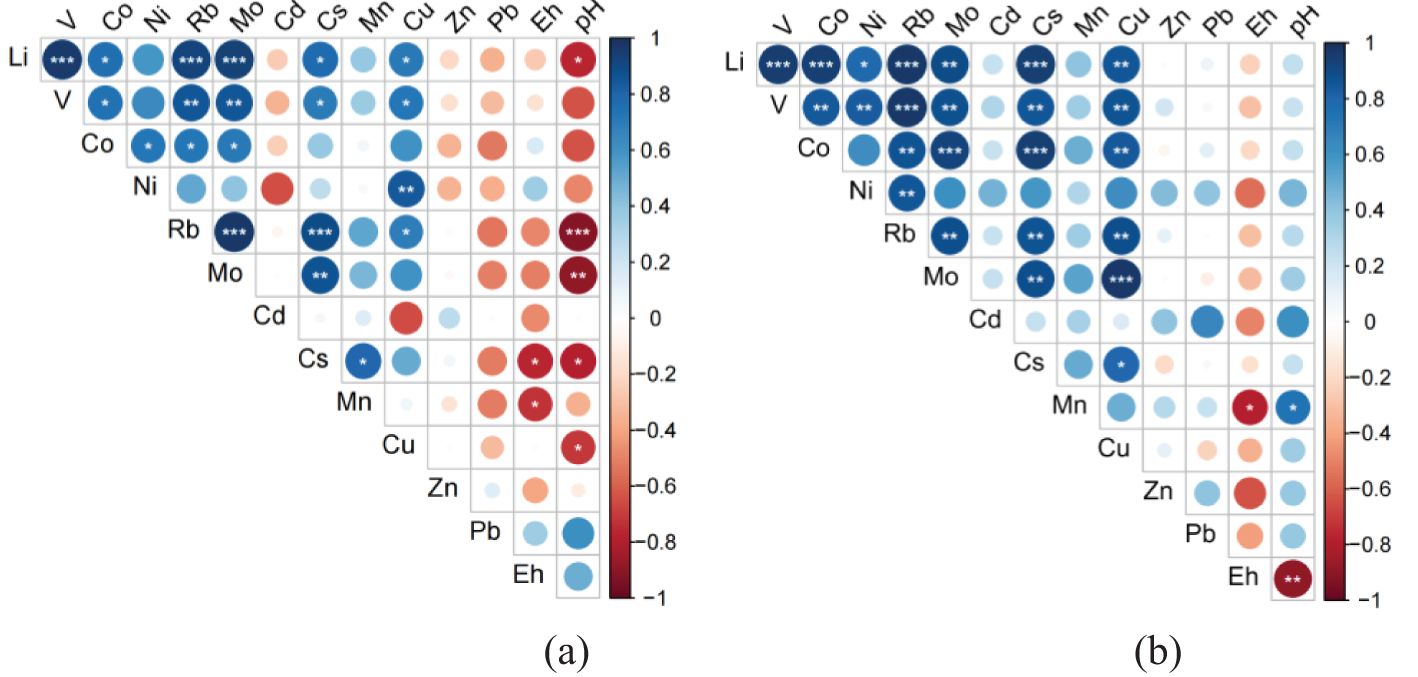
Figure 7. Spearman correlations between metal elements of different disturbance intensities at station BC06. (A) 100-rpm disturbance, (B) 600-rpm disturbance. The number of “*” denotes different significance levels.
As shown in Table 3, most variation coefficients were less than 50%, while very few of them could be over 100%, such as Mn and Zn. Those metals, including Li, V, Ni, Rb, Mo, Mn, Cu, Zn, and Pb, demonstrated a higher variation at the BC04 station in comparison with that under the same stirring disturbance level at BC06. The result coincided with the grain size composition of the two stations (see Figure 2). The coarser the mean grain size of the sediments, the less desorption of metals after the disturbance. Moreover, many metals, such as Li, V, Co, Ni, and Mn, demonstrated a higher variation under 100-rpm than that under 600-rpm stirring disturbance at station BC04 but demonstrated a reverse trend at station BC06. Very few metals, such as Rb and Zn, demonstrated a higher variation under lower disturbance levels at both stations, while Mo and Pb demonstrated a reverse trend at both stations. In brief, the impact of the disturbance level on the desorption and dissolution process for different metals is not consistent at different stations.

Table 3. Variation coefficient (%) of metal concentrations in the overlying water under different stirring disturbances at stations BC04 and BC06.
3.4 Vertical redistribution of dissolved metals in porewater along the depth
As shown in Figure 8, the vertical distributions of most metal concentrations in porewater before the disturbance were similar, with the maximum concentration in the subsurface layer. After the disturbance, almost all metal concentrations in porewater under both disturbance levels increased, and the subsurface peaks disappeared. Due to the continuum between the porewater and overlying water, the redistribution of dissolved metals in porewater, such as Li, V, Co, Ni, Rb, Mo, Cs, and Cd, indicated that metal regeneration could be triggered in sediments. The peak concentrations of many dissolved metals at the depth of 15–20 cm below the sediment surface, especially after the higher level of disturbance, might reflect the activation of metal regeneration in the deep layer.
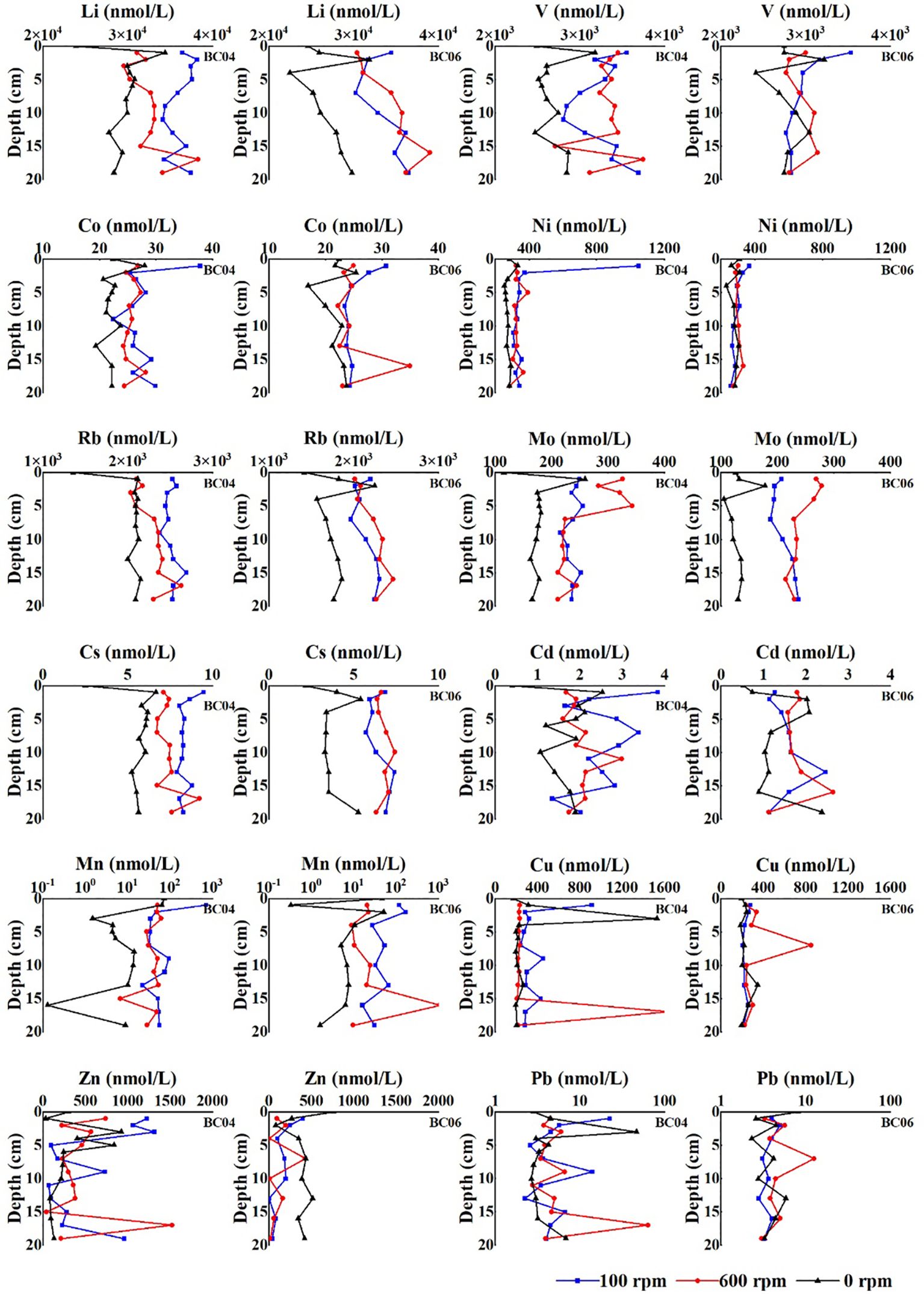
Figure 8. Vertical redistribution of dissolved metals in porewater before and after the experiments.
4 Discussion
4.1 The influence of physicochemical background and disturbance intensity on the geochemical response of metals to the sediment disturbance
Marine sediments serve as both sinks and sources for the exchange of elements with the overlying water column, which can pose significant environmental hazards and elevated ecological risks (Zhong et al., 2006). Despite the release of dissolved metals into the porewater and overlying water being obvious during the experiments, the temporal change of most metal concentrations in the overlying water was concerted and fluctuating for balance in the end of the experiments. Previous research indicated that many external and internal factors have impacts on the element exchange between different phases. For example, sediments are generally categorized into oxic, suboxic, and anoxic based on the diagenetic sequence of terminal electron acceptors. The vertical profiles of Eh exceeding 300 mV, coupled with the predominance of the residual state for many metals in sediments, suggested the oxic sedimentary condition at both stations (see Figure 2). Moreover, the alteration in physicochemical properties, such as pH and Eh after the disturbance, also confirmed the oxic condition in overlying water during the resuspension and resettling processes, which partially accounted for the synchronized metal behaviors.
Recent focus on the impacts of deep-sea mining was posed on the release of precipitates like Mn–Fe oxyhydroxides and mobile sediment constituents (Koschinsky et al., 2001; Volz et al., 2018; Stratmann et al., 2018). The mimetic experiments have confirmed that Mn and Fe oxides and oxyhydroxides are pivotal phases in scavenging many dissolved heavy metals (Koschinsky et al., 2003; Grybos et al., 2007). The preferential scavenging order for metals from seawater is typically Mn oxides > Fe oxides > silicate minerals (Takematsu, 1979). Additionally, many laboratory experiments have suggested that the resuspension of oxic to suboxic surface sediments into the bottom water is rapidly followed by the scavenging of dissolved heavy metals through various mechanisms—for example, hydrated cations such as Mn2+, Co2+, and Ni2+ are preferentially adsorbed or ion-exchanged onto the negatively charged surfaces of Mn oxides, whereas oxyanions and neutrally or negatively charged complexes, such as HVO4— and MoO4—, tend to associate with neutral to slightly positively charged amphoteric Fe oxyhydroxide particles. Other hydrated cations or labile cationic chloro-complexes, such as Cu2+, Zn2+, and PbCl+, can be scavenged in both phases (Koschinsky et al., 2003).
The collective response of metals to the anthropogenic disturbances during the experiments also offered potential insights on their shared characteristics. In terms of their chemical properties, redox-sensitive elements such as V, Mo, and Cd exhibit pronounced responses to variations in oxygen concentrations (Morford and Emerson, 1999). The behavior of Mo, V, Ni, Co, and Cu was also found to be linked to Mn cycling (Paul et al., 2018; Morford et al., 2005), whereas the behavior of Cd was closely associated with Zn (Reddy and DeLaune, 2008; Calmano et al., 1993). Similarly, the concerted behavior among the dissolved metals such as Li, V, Co, Ni, Rb, Mo, and Cs (see Figures 6, 7) also indicated their involvement in similar scavenging processes with Mn and Fe oxides (Koschinsky et al., 2001). In contrast, metals like Cu, Pb, and Zn, with a high proportion of extractable states in sediments, were prone to be released into the overlying water during the resuspension process and rapidly scavenged following the disturbance. It coincided with the breakdown of SEM, which was rapid under oxidizing conditions, coupled with a decrease in pH. However, the rapid recovery of pH also effectively regulated the regeneration of heavy metals (Hong, 2009; Novikau and Lujaniene, 2022; Calmano et al., 1993). Although air exposure likely influenced the behavior of metals in our experimental setup, elements with a high proportion in residual state in sediments, such as V, Mo, Rb, and Cs, demonstrated similar behaviors when subjected to disturbance experiments conducted within a nitrogen-filled glove box (Shi et al., 2023), owing to the prevailing high redox sedimentary condition in both experimental configurations.
In addition, microorganisms can also accelerate the degradation of organic matter and enhance specific metal release during the resuspension process (Lors et al., 2004). The influence of microorganisms was not considered in the experiments owing to the low concentration of TOC in sediments and the short experiment period. However, as regards to a large scale for deep-sea mining, the structure and functional compositions of microbial communities associated with their change in sediments and overlying water must be evaluated in the future for their influence on metal behaviors during the resuspension and resettling processes.
In the context of environmental impacts of commercial deep-sea mining, the particle concentration effect was suggested to be an external factor influencing metal behavior predictions (Jones et al., 2017). The resuspended particles in the overlying water, including their concentration and size distribution, are critical in regulating metal regeneration processes (Trefry et al., 2014; Cantwell et al., 2008; Ma et al., 2019). Most metals have a propensity to resettle within fine particles, which was supported by various experiments and investigations (Ma et al., 2019; Cantwell et al., 2008; Trefry et al., 2014). However, the influence of disturbance intensity on particle concentration effects was not a decisive factor in the metal release process in our study—for instance, larger fluctuations in dissolved metals, such as Mn and Zn, were observed in the overlying water under low disturbance intensity (Table 3). However, a more pronounced fluctuation in metal concentrations within the overlying water was observed at both disturbance levels at station BC04, signifying the influence of sediment composition on metal release across various stations. Furthermore, the distinct temporal patterns in physicochemical characteristics observed under different disturbance levels (see Figure 4) provided an explanation for the significantly negative correlation between pH and the concentrations of metals such as Li, Rb, Mo, and Cs at the 100-rpm disturbance level and the significantly positive correlation between pH and metal concentrations including those of Cd, Zn, and Mn at 600 rpm at station BC06. Accordingly, the indirect effects of disturbance intensity on the scavenging capacity of suspended particles were enforced via the media of pH.
4.2 Potential toxic impacts of the dissolved metals after sediment disturbance
Identifying the potential impact of toxic metals released during deep-sea disturbance is a formidable challenge in assessing the ecological risks posed by deep-sea mining activities (Hauton et al., 2017). Benthic organisms take up metals through multiple pathways, including absorption through skin and gills and ingestion of metal-contaminated sediments or food, yielding both acute and chronic toxic effects (Warren, 1981). The bioavailable metal fraction and the mobility of metals in the environment could be determined by the BCR sequential extraction procedure (Rauret et al., 2000). Thereby, SEM appears to be a good estimate of heavy metal bioavailability, which was applied for a large number of potentially toxic elements over a wide range (Bacon and Davidson, 2008), not only for the prediction of metal behavior under sediment disturbance but also for the potential contamination risk from the human and natural standpoint (Stohs and Bagchi, 1995; Ayala et al., 2014). In our study, Li, V, Rb, Mo, and Cs mainly existed in the residual state, indicating a lower probability of mobility and lower bioavailability and ecological risk. In contrast, those elements, such as Co, Ni, Mn, Cu, and Cd, with a higher fraction in the extractable state in sediments indicated a higher risk of biotoxicity. Moreover, Hauton et al. (2017) reported that redox-active metals, such as Co and Ni, can catalyze the formation of reactive oxygen and nitrogen species that can bind with lipids and cause lipid peroxidation and damage to cell membranes (Stohs and Bagchi, 1995; Ayala et al., 2014). As a large amount of Ni and Co exists in deep seafloor polymetallic nodules on the Northwest Pacific seafloor, the potential environmental risks of metal release during deep-sea mining activities (Hein et al., 1987) should be assessed based on the dissolution of Ni and Co into the water column.
In addition, attention has been given to the potential impacts of metal toxicity changes under deep-sea conditions with high pressure and low temperature during deep-sea mining activities. Scientists used toxicogenomics and the biodynamic model to evaluate metal mixture toxicity and identify associated mechanisms (Rainbow, 2007; Wu et al., 2016). To date, the combined effects of temperature and pressure have rarely been taken into account in modifying the metal tolerance of organisms (Carvalho et al., 1998; Fetters et al., 2016; Hauton et al., 2017), which should be explored based on the potential release of heavy metals, such as Cd, Cu, etc.
5 Conclusion
Sediment disturbance experiments were carried out to simulate the dissolved metal behavior with plumes aroused by deep-sea mining operations. The concentration of 12 dissolved metals in the overlying water was monitored during the resuspension and resettlement processes. According to their behavior, the 12 metals could be divided into different groups. Most metals, such as Li, V, Co, Ni, Rb, Mo, Cs, and Cd, showed a gradual increase, with the final concentrations being higher than the original concentrations. Others, such as Mn, Cu, Zn, and Pb, fluctuated vividly in the beginning but tended to return to equilibrium in the end of the experiment. Many internal and external factors have impacts on the concerted behavior of metals. The variation coefficients of metal concentration in the overlying water were more influenced by the sediment texture of the stations than by the level of disturbance intensities. Moreover, the concentrations of most metals in the porewater increased following both levels of disturbance, suggesting that the enrichment of those dissolved metals in the overlying water was partially due to the regeneration of metals within the sediment matrix. The results provide valuable evidence for predicting the concerted behavior of metals under the disturbance of deep-sea mining activities in the polymetallic nodule area of western Pacific. Nevertheless, our current understanding of the environmental impacts of the deep seafloor is far from sufficient. In-situ experiments are urgently needed to simulate the resuspension and resettling processes under a larger disturbance scale on the deep seafloor with high pressure, low temperature, and bottom current environments.
Data availability statement
The raw data supporting the conclusions of this article will be made available by the authors, without undue reservation.
Author contributions
JY: Conceptualization, Data curation, Funding acquisition, Investigation, Methodology, Writing – original draft, Writing – review & editing, Validation. ZX: Formal analysis, Validation, Visualization, Writing – original draft. BL: Funding acquisition, Writing – review & editing, Project administration. DS: Data curation, Investigation, Writing – review & editing. CW: Data curation, Funding acquisition, Methodology, Resources, Writing – review & editing. LH: Data curation, Methodology, Writing – review & editing. JX: Funding acquisition, Writing – review & editing. WZ: Data curation, Writing – review & editing, Resources. CS: Data curation, Funding acquisition, Writing – review & editing.
Funding
The author(s) declare financial support was received for the research, authorship, and/or publication of this article. This research work was supported by the National Key R&D Program of China (2022YFC2803902, 2023YFC2811400, and SQ2021YFC2800022) and the China Ocean Mineral Resources Research and Development Association (DY135-E2-2-3).
Acknowledgments
We thank the crews of the 56th cruises of the Chinese Research expedition in the Pacific Ocean for their onboard assistance.
Conflict of interest
The authors declare that the research was conducted in the absence of any commercial or financial relationships that could be construed as a potential conflict of interest.
Publisher’s note
All claims expressed in this article are solely those of the authors and do not necessarily represent those of their affiliated organizations, or those of the publisher, the editors and the reviewers. Any product that may be evaluated in this article, or claim that may be made by its manufacturer, is not guaranteed or endorsed by the publisher.
References
Amann H. (1982). Technological trends in ocean mining. Phil. Trans. R. Soc. Lond. A. 307, 377–403. doi: 10.1098/rsta.1982.0118
Amos A. F., Roels O. A. (1977). Environmental aspects of manganese nodule mining. Mar. Policy 1, 156–163. doi: 10.1016/0308-597X(77)90050-1
Ankley G., Toro D. D., Hansen D., Berry W. (1996). Assessing the ecological risk of metals in sediments. Environ. Toxicol. Chem. 15, 2053–2055. doi: 10.1002/etc.5620151201
Ates A., Demirel H., Mergul N. (2020). Risk assessment and chemical fractionation of heavy metals by BCR sequential extraction in soil of the Sapanca Lake Basin, Turk. Pol. J. Environ. Stud. 29, 1523–1533. doi: 10.15244/pjoes/101609
Ayala A., Muñoz M. F., Argüelles S. (2014). Lipid peroxidation: production, metabolism, and signaling mechanisms of malondialdehyde and 4-hydroxy-2-nonenal. Oxid. Med. Cell. Longevity 2014, 360438. doi: 10.1155/2014/360438
Bacon J. R., Davidson C. M. (2008). Is there a future for sequential chemical extraction? Analyst 133, 25–46. doi: 10.1039/b711896a
Burns R. E., Erickson B., Lavelle J. W., Ozturgut E. (1980). Observations and measurements during the monitoring of deep ocean manganese nodule mining test in the North Pacific, April-May 1978, US Department of Commerce, NOAA technical Memorandum, ERL MESA-47.
Calmano W., Hong J., Förstner U. (1993). Binding and mobilization of heavy metals in contaminated sediments affected by pH and redox potential. Water Sci. Technol. 28, 223–235. doi: 10.2166/wst.1993.0622
Cantwell M. G., Burgess R. M., King J. W. (2008). Resuspension of contaminated field and formulated reference sediments Part I: Evaluation of metal release under controlled laboratory conditions. Chemosphere 73, 1824–1831. doi: 10.1016/j.chemosphere.2008.08.007
Caroppo C., Stabili L., Aresta M., Corinaldesi C., Danovaro R. (2006). Impact of heavy metals and PCBs on marine picoplankton. Environ. Toxicol. 21, 541–551. doi: 10.1002/tox.20215
Carvalho P. S. M., Zanardi E., Buratini S. V., Lamparelli M. C., Martins M. C. (1998). Oxidizing effect on metal remobilization and daphia similis toxicity from a Brazilian reservoir sediment suspension. Water Res. 32 (1), 193–199. doi: 10.1016/S0043-1354(97)00186-3
Drazen J. C., Smith C. R., Gjerde K. M., Haddock S. H.D., Carter G. S., Choy C.A., et al. (2020). Midwater ecosystems must be considered when evaluating environmental risks of deep-sea mining. Proc. Natl. Acad. Sci. 117, 17455–17460. doi: 10.1073/pnas.2011914117
Duman F., Aksoy A., Demirezen D. (2007). Seasonal variability of heavy metals in surface sediment of lake Sapanca. Turkey Environ. Monit. Assess. 133, 277–283. doi: 10.1007/s10661-006-9580-3
Fan W., Wang W.-X., Chen J., Li X., Yen Y.-F. (2002). Cu, Ni, and Pb speciation in surface sediments from a contaminated bay of northern China. Mar. pollut. Bull. 44 (8), 820–826. doi: 10.1016/s0025-326x(02)00069-3
Fetters K. J., Costello D. M., Hammerschmidt C. R., Burton G. A. Jr (2016). Toxicological effects of short-term resuspension of metal-contaminated freshwater and marine sediments. Environ. Toxicol. Chem. 35, 676–686. doi: 10.1002/etc.3225
Glasby G. P. (1977). “Marine manganese deposits,” in Elsevier oceanography series 15 (Elsevier, Adsterdam), 523 pp.
Grybos M., Davranche M., Gruau G., Petitjean P. (2007). Is trace metal release in wetland soils controlled by organic matter mobility or Fe-oxyhydroxides reduction? J. Colloid Interface Sci. 314, 490–501. doi: 10.1016/j.jcis.2007.04.062
Hauton C., Brown A., Thatje S. C., Mestre N. C., Bebianno M. J., Martins I., et al. (2017). Identifying toxic impacts of metals potentially released during deep-sea mining—A synthesis of the challenges to quantifying risk. Front. Mar. Sci. doi: 10.3389/fmars.2017.00368
Hong Y. (2009). Experimental and mathematical investigation of dynamic availability of metals in sediment [dissertation], (Austin: The University of Texas at Austin), P 7.
Jones D. O. B., Kaiser S., Sweetman A. K., Smith C. R., Menot L., Vink A., et al. (2017). Biological responses to disturbance from simulated deep-sea polymetallic nodule mining. PloS One 12, e0171750. doi: 10.1371/journal.pone.0171750
Kalnejais L. H., Martin W. R., Bothner M. H. (2010). The release of dissolved nutrients and metals from coastal sediments due to resuspension. Mar. Chem. 121, 224–235. doi: 10.1016/j.marchem.2010.05.002
Kiratli N., Ergin M. (1996). Partitioning of heavy metals in surface Black Sea sediments. Appl. Geochemistry 11, 775–788. doi: 10.1016/S0883-2927(96)00037-6
Koschinsky A., Gaye-Haake B., Arndt C., Maue G., Spitzy A., Winkler A., et al. (2001). Experiments on the influence of sediment disturbances on the biogeochemistry of the deep-sea environment. Deep Sea Res. Part II: Topical Stud. Oceanography 48 (17-18), 3629–3651. doi: 10.1016/S0967-0645(01)00060-1
Koschinsky A., Winkler A., Fritsche U. (2003). Importance of different types of marine particles for the scavenging of heavy metals in the deep-sea bottom water. Appl. Geochemistry 18, 693–710. doi: 10.1016/S0883-2927(02)00161-0
Li T., Wang D., Zhang B., Liu H., Tang H. (2006). Characterization of the phosphate adsorption and morphology of sediment particles under simulative disturbing conditions. J. Hazardous Materials 137, 1624–1630. doi: 10.1016/j.jhazmat.2006.04.051
Lors C., Tiffreau C., Laboudigue A. (2004). Effects of bacterial activities on the release of heavy metals from contaminated dredged sediments. Chemosphere 56, 619–630. doi: 10.1016/j.chemosphere.2004.04.009
Ma T., Sheng Y., Meng Y., Sun J. (2019). Multistage remediation of heavy metal contaminated river sediments in a mining region based on particle size. Chemosphere 225, 83–92. doi: 10.1016/j.chemosphere.2019.03.018
Machida S., Fujinaga K., Ishii T., Nakamura K., Hirano N., Kato Y. (2016). Geology and geochemistry of ferromanganese nodules in the Japanese Exclusive Economic Zone around Minamitorishima Island. Geochemical J. 50, 1–17. doi: 10.2343/geochemj.2.0419
Martino M., Turner A., Nimmo M., Millward G. E. (2002). Resuspension, reactivity and recycling of trace metals in the Mersey estuary. Mar. Chem. 77, 171–186. doi: 10.1016/S0304-4203(01)00086-X
Mero J. L. (1965). “The mineral resources of the sea,” in Elsevier Oceanography Series, 1 (Elsevier Publishing Company, Amsterdam/London/New York), 312 pp, ISBN: ISBN 978-0-444-40394-0.
Ministry of Environmental Protection. (2016). “Water quality-determination of inorganic anions (F-、Cl-、NO2-、Br-、NO3-、PO43-、SO32-、SO42-)-ion chromatography,” in National Environmental Protection Standards of the People's Republic of China. HJ84. (Beijing: China Environmental Science Press).
Ministry of Natural Resources. (1921). “Methods for analysis of groundwater quality—part 80: determination of forty element (lithium,rubidium,cesium, etc) concentrations—by inductively coupled plasma mass spectrometry,” in People’s Republic of China, Industry Standards, Geology CN-DZ, DZ/T 0064.80-2021.
Morato T., Juliano M., Pham C. K., Carreiro-Silva M., Martins I and Colaço A. (2022). Modelling the dispersion of seafloor massive sulphide mining plumes in the mid atlantic ridge around the azores. Front. Mar. Sci. 9. doi: 10.3389/fmars.2022.910940
Morford J. L., Emerson S. (1999). The geochemistry of redox sensitive trace metals in sediments. Geochimica Cosmochimica Acta 63, 1735–1750. doi: 10.1016/S0016-7037(99)00126-X
Morford J. L., Emerson S. R., Breckel E. J., Kim S. H. (2005). Diagenesis of oxyanions (V, U, Re, and Mo) in porewaters and sediments from a continental margin. Geochimica Cosmochimica Acta 69, 5021–5032. doi: 10.1016/j.gca.2005.05.015
Morin J., Morse J. W. (1999). Ammonium release from resuspended sediments in the Laguna Madre estuary. Mar. Chem. 65, 97–110. doi: 10.1016/S0304-4203(99)00013-4
Nath B. N., Parthiban G., Sankar S. J. (2001). Sediment redistribution during simulated benthic disturbance and its implications on deep seabed mining. Deep-Sea Res. Part II 48, 3363–3380. doi: 10.1016/S0967-0645(01)00046-7
Novikau R., Lujaniene G. (2022). Adsorption behaviour of pollutants: Heavy metals, radionuclides, organic pollutants, on clays and their minerals (raw, modified and treated): A review. J. Environ. Manage. 309, 114685. doi: 10.1016/j.jenvman.2022.114685
Ozturgut E., Anderson G. C., Burns R. E., Lavelle J. W., Swift S. A. (1978). “Deep ocean mining of manganese nodules in North Pacific Ocean,” in Pre-mining environmental conditions and anticipated mining effects. NOAA-TM-ERL MESA-33 (National Oceanic and Atmospheric Administration, USA), 138 pp.
Paul S., Gaye B., Haeckel M., Kasten S., Koschinsky A. (2018). Biogeochemical regeneration of a nodule mining disturbance site: trace metals, DOC and amino acids in deep-sea sediments and porewaters. Front. Mar. Sci. 5. doi: 10.3389/fmars.2018.00117
Petersen S., Krätschell A., Augustin N., Jamieson J., Hein J. R., Hannington M. D. (2016). News from the seabed – Geological characteristics and resource potential of deep-sea mineral resources. Mar. Policy 70, 175–187. doi: 10.1016/j.marpol.2016.03.012
Raghukumar C., Bharathi P. A. L., Ansari Z. A., Nair S., Ingole B., Sheelu G., et al. (2001). Bacterial standing stock, meiofauna and sediment-nutrient characteristics: Indicators of benthic disturbance in the Central Indian Basin. Deep-Sea Res. Part II 48, 3381–3399. doi: 10.1016/S0967-0645(01)00047-9
Rainbow P. S. (2007). Trace metal bioaccumulation: Models, metabolic availability and toxicity. Environ. Int. 33, 576–582. doi: 10.1016/j.envint.2006.05.007
Rauret G., López-Sánchez J. F., Sahuquillo A., Barahona E., Lachica M., Ure A. M., et al. (2000). Application of a modified BCR sequential extraction (three-step) procedure for the determination of extractable trace metal contents in a sewage sludge amended soil reference material (CRM 483), complemented by a three-year stability study of acetic acid and EDTA extractable metal content. J. Environ. Monit. 2, 228–233. doi: 10.1039/b001496f
Reddy K. R., DeLaune R. D. (2008). Biogeochemistry of Wetlands: Science and Applications. 1st ed (Boca Raton: CRC Press). doi: 10.1201/9780203491454
Ren J., He G., Deng X., Deng X., Yang Y., Yao H., et al. (2022). Metallogenesis of Co-rich ferromanganese nodules in the northwestern Pacific: Selective enrichment of metallic elements from seawater. Ore Geology Rev. 143, 104778. doi: 10.1016/j.oregeorev.2022.104778
Ren J., He G., Yang Y., Yu M., Deng Y., Pang Y., et al. (2024). Ultraselective enrichment of trace elements in seawater by Co-rich ferromanganese nodules. Global Planetary Change 239, 104498. doi: 10.1016/j.gloplacha.2024.104498
Roberts D. A. (2012). Causes and ecological effects of resuspended contaminated sediments (RCS) in marine environments. Environ. Int. 40, 230–243. doi: 10.1016/j.envint.2011.11.013
Saulnier I., Mucci A. (2000). Trace metal remobilization following the resuspension of estuarine sediments: Saguenay Fjord, Canada. Appl. Geochemistry 15, 191–210. doi: 10.1016/S0883-2927(99)00034-7
Shi P., Yang J., Sun D., Wang C. (2023). A simulation from offsite disturbance experiments on the metal resuspension process in the seafloor of the western Pacific. Chemosphere 311, 137042. doi: 10.1016/j.chemosphere.2022.137042
Singh K., Mohan D., Singh V., Malik A. (2005). Studies on distribution and fractionation of heavy metals in Gomti river sediments - A tributary of the Ganges, India. J. Hydrology 312, 14–27. doi: 10.1016/j.jhydrol.2005.01.021
Spearman J., Taylor J., Crossouard N., Cooper A., Turnbull M., Manning A., et al. (2020). Measurement and modelling of deep sea sediment plumes and implications for deep sea mining. Sci. Rep. 10, 5075. doi: 10.1038/s41598-020-61837-y
Stohs S. J., Bagchi D. (1995). Oxidative mechanisms in the toxicity of metal ions. Free Radical Biol. Med. 18, 321–336. doi: 10.1016/0891-5849(94)00159-h
Stratmann T., Mevenkamp L., Sweetman A., Vanreusel A., Oevelen D. (2018). Has phytodetritus processing by an abyssal soft-sediment community recovered 26 years after an experimental disturbance? Front. Mar. Sci. 5. doi: 10.3389/fmars.2018.00059
Takematsu N. (1979). Sorption of transition metals on manganese and iron oxides, and silicate minerals. J. Oceanographical Soc. Japan 35, 36–42. doi: 10.1007/BF02108280
Tessier A., Campbell P., Bisson M. (1979). Sequential extraction procedure for the speciation of particular trace elements. Environ. Technol. 15 (7), 844–851. doi: 10.1021/ac50043a017
Thiel H., Tiefsee-Umweltschutz F. (2001). Evaluation of the environmental consequences of polymetallic nodule mining based on the results of the TUSCH Research Association. Deep-Sea Res. II 48, 3433–3452. doi: 10.1016/S0967-0645(01)00051-0
Trefry J. H., Trocine R. P., Cooper L. W., Dunton K. H. (2014). Trace metals and organic carbon in sediments of the northeastern Chukchi Sea. Deep Sea Res. Part II: Topical Stud. Oceanography 102, 18–31. doi: 10.1016/j.dsr2.2013.07.018
Varol M., Sen B. (2012). Assessment of nutrient and heavy metal contamination in surface water and sediments of the upper Tigris River, Turkey. Catena 92, 1–10. doi: 10.1016/j.catena.2011.11.011
Volz J., Haffert L., Haeckel M., Koschinsky A., Kasten S. (2020). Impact of small-scale disturbances on geochemical conditions, biogeochemical processes and element fluxes in surface sediments of the eastern Clarion-Clipperton Zone, Pacific Ocean. Biogeosciences 17, 1113–1131. doi: 10.5194/bg-17-1113-2020
Volz J. B., Mogollón J. M., Geibert W., Arbizu P. M., Koschinsky A., Kasten S. (2018). Natural spatial variability of depositional conditions, biogeochemical processes and element fluxes in sediments of the eastern Clarion-Clipperton Zone, Pacific Ocean. Deep Sea Res. Part I: Oceanographic Res. Papers 140, 159–172. doi: 10.1016/j.dsr.2018.08.006
Warren L. J. (1981). Contamination of sediments by lead, zinc and cadmium: A review. Environ. Pollut. Ser. B Chem. Phys. 2, 401–436. doi: 10.1016/0143-148X(81)90037-9
Wu X., Cobbina S., Mao G., Xu H., Zhang Z., Yang L. (2016). A review of toxicity and mechanisms of individual and mixtures of heavy metals in the environment. Environ. Sci. pollut. Res. 23, 8244–8259. doi: 10.1007/s11356-016-6333-x
Yang J., Nie H., Sun D., Wang C. (2023). Environmental controls on the distribution of metals in porewater and their diffusion fluxes at the sediment-water interface of the western Pacific. Appl. Geochemistry 148, 105520. doi: 10.1016/j.apgeochem.2022.105520
Keywords: deep-sea mining, metal regeneration, sediment disturbance, polymetallic nodules, Western Pacific
Citation: Yang J, Xing Z, Liu B, Sun D, Wang C, Han L, Xia J, Zhang W and Song C (2024) Metal regeneration during an ex-situ disturbance experiment on deep-sea sediments from the polymetallic nodule area of western Pacific. Front. Mar. Sci. 11:1480906. doi: 10.3389/fmars.2024.1480906
Received: 14 August 2024; Accepted: 23 September 2024;
Published: 24 October 2024.
Edited by:
Jiangbo Ren, Guangzhou Marine Geological Survey, ChinaReviewed by:
Huan Zhang, University of Hamburg, GermanyFenlian Wang, Guangzhou Marine Geological Survey, China
Copyright © 2024 Yang, Xing, Liu, Sun, Wang, Han, Xia, Zhang and Song. This is an open-access article distributed under the terms of the Creative Commons Attribution License (CC BY). The use, distribution or reproduction in other forums is permitted, provided the original author(s) and the copyright owner(s) are credited and that the original publication in this journal is cited, in accordance with accepted academic practice. No use, distribution or reproduction is permitted which does not comply with these terms.
*Correspondence: Juan Yang, eWFuZ2p1YW5AY3VnYi5lZHUuY24=