- Laboratório de Estudos Marinhos Aplicados – LEMA, Escola Politécnica, Universidade do Vale do Itajaí – UNIVALI, Itajaí, Brazil
In the deep-sea, abundant and diverse biological communities tend to occur in areas where combinations of geological, physical and biological processes locally enhance trophic-wide productivity, sustaining aggregations of consumers and top predators. These areas are ‘productivity hotspots’ and their delimitation should improve the effectiveness of area-based strategies designed to manage human activities and protect the deep-sea. We explored the premise that fishing operations in association with geomorphological features are effective surrogates for delimiting productivity hotspots distribution in the Brazilian Meridional Margin (BMM; 18°S - 35°S; 200 – 3300 m). We described along-slope and across-slope geoforms and related them with catch patterns of fishes, deep-sea shrimps, deep-sea crabs and squids in 23565 fishing hauls conducted between 2000 and 2007. Over 97% of the total catch was obtained in Santos (42.7%) and Pelotas (54.8%) sedimentary basins. In the former, 55.7% of all fishing hauls and 38.6% of the catch were obtained from one of 12 slope geoforms, which covered 22% of the available fishing area. In the Pelotas basin, a shelf break geoform covering 6% of the fishing area concentrated 29.5% of the fishing hauls and 30.2% of the catch. Best fit delta-lognormal Generalized Linear Models (explaining >50% of the total variance) highlighted the positive effect of shelf break embayments of Santos Basin in occurrence and abundance of the pool of species targeted by fisheries. Within these embayments, sectors incurvated and excavated by erosive action of the Brazil Current positively affected abundance and occurrences of fishes and deep-sea shrimps, respectively. The upper slope (300 – 500 m depths) were particularly favorable for fishes and squid concentrations, whereas the lower slope (600 – 800 m depths) increased the probability of deep-sea shrimp catches. We propose that the Santos Basin shelf break embayments host mesoscale and sub-mesoscale productivity hotspots, sustained by biophysical processes promoted by the Brazil Current flow, coupled with biological active transport of pelagic and demersal vertical migrators, some of them targeted by bottom fisheries. We advocate that these are unique features, in the otherwise oligotrophic SW Atlantic subtropical gyre, that should be taking into consideration in regional systematic conservation plans.
1 Introduction
Preserving marine ecosystems and the services they provide has called for conservation strategies focused on protecting biodiversity from the effects of human exploitation of living and non-living resources (Briscoe et al., 2016; Magris et al., 2020). To that end, these strategies have often relied on the effectiveness of area-based measures (e.g., marine protected areas - MPAs) to protect biodiversity ‘hotspots’, defined as areas where diversity is particularly rich, including rare and endemic species (Costello et al., 2022). While consistent with the strategies adopted in terrestrial ecosystem conservation, and likely useful for coastal ecosystems, protecting areas considered biodiversity hotspots may prove insufficient when it comes to the open ocean (Briscoe et al., 2016). In essence, that is because ecosystem services (including food provisioning) are not only maintained by biodiversity alone but by a combination of biophysical processes that result in productivity enhancement and energy transfer through the trophic web, that sustain rich and abundant communities (Mann and Lazier, 2005; Scales et al., 2014). As noted by Briscoe et al. (2016), ‘a strategy that focuses on protecting processes, patterns, and features that promote enhanced biological productivity in addition to biodiversity, will have a greater probability of including important conservation features’.
Deep-ocean habitats are facing growing human pressures originated from climate change and different forms of use of valuable living and non-living resources (Ramírez-Llodra et al., 2010; Levin and LeBris, 2015; Danovaro et al., 2017). Global warming has affected the deep-sea, which is gradually becoming warmer, more acidic, less oxygenated and even more food-limited (Thurber et al., 2014; Sweetman et al., 2017; Cheung et al., 2022). Simultaneously, human activities such as fisheries and mining seriously threaten structurally-complex biogenic habitats, including cold-water coral reefs, which host locally abundant and diverse communities on deep continental margins, seamounts and ridges, with little chances of recovery (Boschen et al., 2016; Clark et al., 2019; Carreiro-Silva et al., 2022). In that sense, deep-sea conservation has become of major concern around the globe (Barbier et al., 2014), raising important debates about cost-effective conservation strategies (e.g., Dunn et al., 2018).
The definition of priority conservation features and the delimitation of areas where these features could be more effectively protected, are critical steps for the development of deep-sea conservation strategies (Combes et al., 2021a). Deep-ocean basins are mostly oligotrophic, except for localized regions where combinations of topography, circulation, geological or biological processes create local phenomena that promote a relatively stable food supply and sustain abundant/rich communities (e.g., hydrothermal vents, food-falls, seamounts and others) (Genin, 2004; Ramirez-Llodra et al., 2010). These are ‘productivity hotspots’ in the deep-ocean that encompass trophic-wide aggregations of primary producers, secondary and tertiary consumers, and top predators, some of them targets of commercial fisheries (Levin and Dayton, 2009; Briscoe et al., 2016). Conservation strategies that prioritize regions identified as productivity hotspots, could favor the protection of unique biodiversity features while preserving current and future uses of the deep-sea.
The spatial delimitation of productivity hotspots in the deep-ocean, as defined above, requires the characterization of localized biophysical processes and associated biological communities, usually achieved by integrated field studies (e.g., Kenchington et al., 2014). In poorly explored ocean basins, however, these studies tend to be insufficient to support conservation initiatives, justifying the use of alternative spatial analyses that can approximate the localization of potential productivity hotspots. Often, these analyses explore spatial patterns of regionally available surrogate environmental variables (e.g., Anderson et al., 2011; Misiuk and Brown, 2024), in particular those that describe seafloor geomorphic features and can be derived from bathymetry maps (Wilson et al., 2007; Harris et al., 2014; Walbridge et al., 2018). These terrain variables have been commonly used to describe deep-sea habitats and assess relevant drivers of deep-sea benthic species distribution (Yoklavich et al., 2000; Brown et al., 2011; Anderson et al., 2011; Misiuk and Brown, 2024). They can also delineate a variety of deep-sea geological features that originate from tectonic, sedimentary, oceanographic and biological processes (e.g., cold-water coral reef growth) interacting at multiple spatial and temporal scales (Blondel, 2001; Camerlenghi, 2018). Some geoforms are characterized by abrupt topographies (e.g. seamounts, canyons, shelf breaks), which modify the flow of deep-water masses and interpose the flux of particles suspended on oceanic pelagic layers, promoting mechanisms that spatially aggregate plankton, micronekton and their predators (e.g., Fernandez-Arkaya et al., 2017). These mechanisms operate mostly at intermediate depths (~200 – 400 m) by inducing (a) the upwelling/uplift of nutrient-rich deep waters up to the photic layer, strongly and persistently enough to sustain phytoplankton and zooplankton growth, and (b) the flow-driven processes of biomass accumulation in relatively small areas (Genin, 2004). High order consumers, including meso- and bathypelagic fishes, squids and deep-sea shrimps and crabs may couple their behavior and life history patterns with these biophysical processes, benefiting from the increased prey supply and forming stable patches of elevated density, which collectively typify deep productivity hotspots in space (Healey et al., 1990). If these organisms are commercially valuable (e.g., orange roughy, toothfishes, alfonsinos, aristeid shrimps, geryonid crabs and others) such patches tend to be exploited by fishing fleets, and sustain deep-water fisheries (Koslow et al., 2000; Clark, 2009; Company et al., 2012; Salois et al., 2023).
This study will explore the premise that historical records of fishing operations in association with deep abrupt topographies may operate as effective surrogates for oceanographic processes that define the spatial distribution of productivity hotspots. Also, because these areas include conservation features and food provisioning (i.e., exploitable biomass of fishing resources), their spatial delimitation is intended to support area-based management and conservation strategies in the deep-sea. The study will focus on the South American continental margin off Southeastern and Southern Brazil (SW Atlantic), here referred to as the Brazilian Meridional Margin (BMM, sensu Alberoni et al., 2019). The slope region is characterized by a complex topography and a variety of geological features that include sedimentary slopes, submarine canyons and pockmarks (Harris et al., 2014). In association with these features, distinctive benthic habitats and communities have been described, particularly cold-water coral banks, reefs and cold-seeps (see reviews in DeLeo et al., 2020; Kitahara et al., 2020; Shimabukuro et al., 2020). Bottom fisheries surged in this region in 2000, targeting concentrations of predatory demersal fish (e.g., the monkfish Lophius gastrophysus), crustaceans (Chaceon spp. and aristeid shrimps) and squids (Illex argentinus) (Perez et al., 2009a). For ten years, this activity affected over 10% of the available and previously untouched slope area of the BMM, being responsible for important biomass reductions of the main targeted stocks, and the increased mortality of noncommercial fauna including cold-water corals (Perez et al., 2013, 2020; Kitahara et al., 2020). After that period, fishing pressure on the slope grounds reduced to relatively low levels (Costa et al., 2024), but the region continued to be increasingly exposed to other anthropogenic pressures (Halpern et al., 2015), most notably, offshore exploration of oil and gas (Perez et al., 2020) and global warming [i.e., the region is under the influence of a major marine hotspot of the Atlantic Ocean (Franco et al., 2020)].
Based on these cumulative human pressures and environmental sensitivity criteria (e.g., biodiversity representation, population connectivity and threatened species), priority areas for implementing new MPAs have been proposed within the BMM slope region (Magris et al., 2020; Costa, 2024). However, no concrete initiatives in that direction exist to date, but only management measures and regulations placed on slope fishing and oil and gas exploration activities (Perez et al., 2020). The delimitation of productivity hotspots could inform area-based management and conservation strategies, such as the implementation of MPA networks along the slope region (Costa, 2024), the limitation of bottom fishing areas (Perez et al., 2009a) and the exclusion of ecologically significant regions from the areas leased for deep-sea oil and gas exploration (Bernardino and Sumida, 2017). These strategies, and others, are expected to emerge in the context of the recently established Brazilian Marine Spatial Planning process (Gerhardinger et al., 2019), in which areas defined as deep-sea productivity hotspots could be particularly useful to develop conservation plans in the oceanic sectors of Brazilian EEZ. Around the globe, criteria defining deep-sea MPAs location, both within and outside EEZs, have gradually incorporated ecosystem productivity as a conservation feature (e.g., Briscoe et al., 2016; Visali et al., 2020) and some high-seas MPAs proposed under the BBNJ framework are productivity hotspots, e.g., the Costa Rica Dome, the South Tasman Sea, the Emperor Seamount Chain, the Mascarene Plateau, and the Gulf of Guinea (PEW, 2024). Despite the availability of data on habitats and biodiversity in these areas, their selection and spatial delimitation have relied on mapping environmental variables that best represented desired conservation features. Because habitat and biodiversity descriptions in the BMM slope region are scarce and scattered (Sumida et al., 2020) using the same approach, as proposed in this study, seems necessary and timely.
2 Materials and methods
2.1 Study area and deep-sea fisheries
The Brazilian Meridional Margin (BMM) is the southernmost sector of Brazilian Continental Margin extending from the Espírito Santo State (~20°S) to the Brazilian EEZ border with Uruguay (~34°S) (Alberoni et al., 2019) (Figure 1). It is part of the East South America divergent/passive margin, which includes four sedimentary basins: Espírito Santo, Campos, Santos and Pelotas (Mohriak, 2003). The continental shelf is narrow at Espírito Santo and Campos basins (~40 km), widening south of 21°S, across the arc-shaped margin of Santos Basin (up to 250 km-wide). The Pelotas Basin extend to the south of 28°30’S with a continental shelf 125 km-wide, on average. The continental shelf in the BMM breaks at 80 to 180 m depths from which a generally gentle slope extends down to approximately 2000 m (Martins and Coutinho, 1981). The shelf-slope transition region is characterized by a sinusoidal morphology, with a series of embayments (Duarte and Viana, 2016). Within these embayments the upper slope is gentle and interrupted by terraces (Figure 1) formed by (a) the deposition of sediment drifts by along-slope flowing Brazil Current (BC), and (b) the across-slope sediment transport in association with sea level transgression/regression processes during the late Quaternary (Viana, 2002; de Mahiques et al., 2007; Alves, 2010; Duarte and Viana, 2016; Lobo et al., 2024). The shelf break and slope are also carved by canyons and scars of downslope sedimentary displacement (Alberoni et al., 2019). The slope is connected to the Brazil Basin through the São Plateau (21 - 29°S) that extends over 400,000 km2, from 2000 to 4500 m depths.
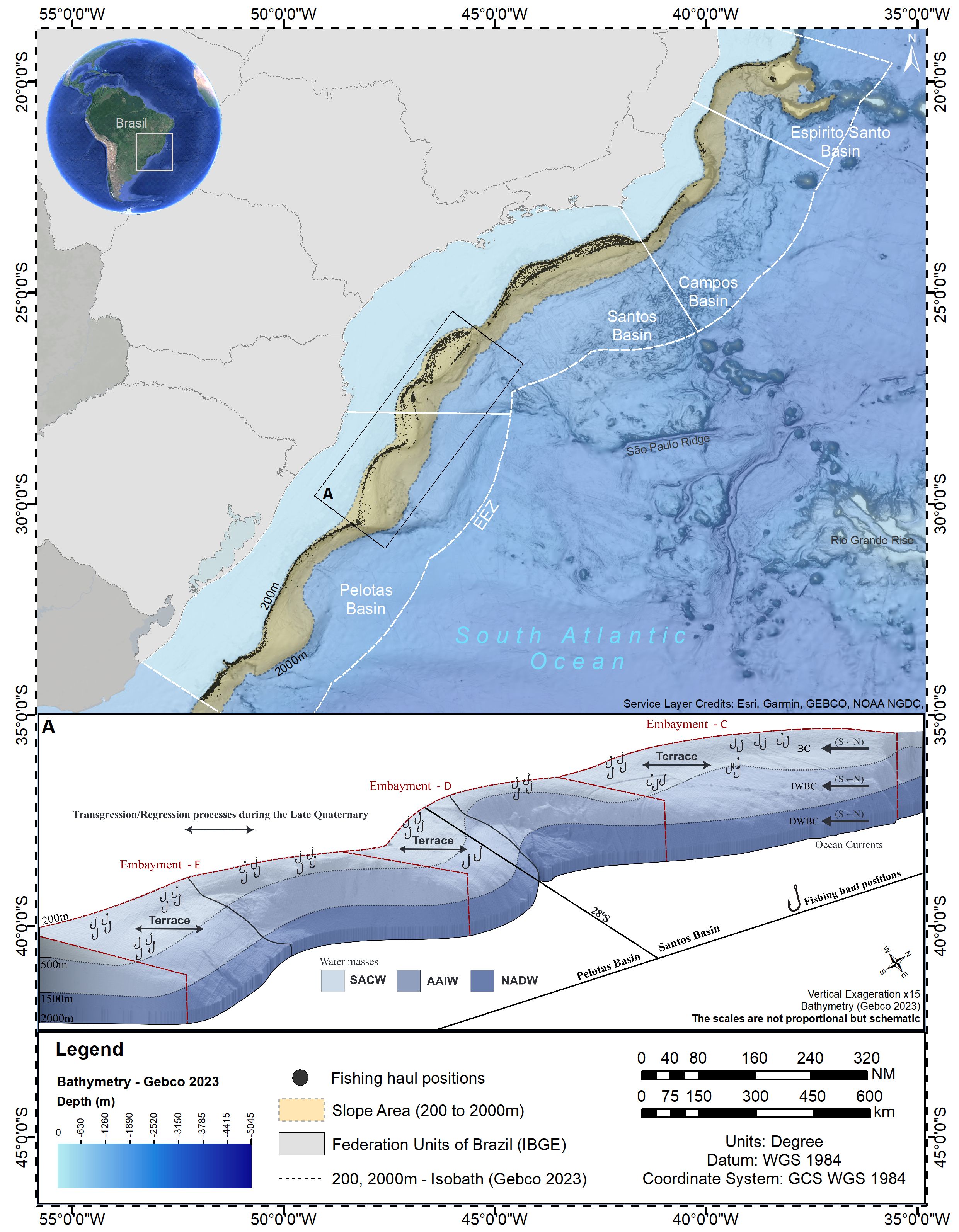
Figure 1. Slope area of the Brazilian Meridional Margin (200 – 2000 m depths), SW Atlantic. Insert A depicts a 3-D representation of slope region within box A, indicating geological features and selected fishing haul positions (hooks).
The BMM is influenced by two western boundary current systems of the South Atlantic; the Brazil Current (BC) and the Deep Western Boundary Current (DWBC) (Figure 1). At the northernmost sector of the BMM (~21°S), the BC extends from the surface to the upper slope (350 – 500m) transporting the Tropical Water and the South Atlantic Central Water (SACW) towards the south, at speeds reaching 0.7 m/s (Silveira et al., 2020). Intermediate waters masses that flow towards the BMM between 500 and 1500 m depths bifurcate at the Santos Basin (23 - 28°S) where (a) the Antarctic Intermediate Water (AAIW) and the Upper Circumpolar Water originate the equatorward flowing Intermediate Western Boundary Current, and (b) the AAIW is added to the BC southward transport, which becomes deeper mostly south of 28°S (Silveira et al., 2020). Below 1500 m, the DWBC carries the North Atlantic Deep Water (NADW), flowing from the Northwest Atlantic southward along the BMM lower slope with velocities up to 0.2 m/s. At its southernmost reach (38° - 40°S) the BC collides with cold and nutrient-rich subantarctic waters carried northwards by the Malvinas Current (the Brazil-Malvinas Confluence), and retroflects eastwards over the South Atlantic Basin (Brandini et al., 2000; Artana et al., 2019). Particularly relevant in the context of fishing activity, is the BC flow on the upper slope, which is affected by the along-margin shelf break sinusoidal morphology, defining zones of acceleration/deceleration of current flow that lead to erosion/deposition of sediment drifts (Figure 1) (Viana, 2002; Alves, 2010). Also, the shear of the BC with the seafloor in these zones produce changes in along-margin pressure gradients which, in association with the development of cyclonic meanders and eddies, induce shelf break and upper slope uplift of the SACW (Campos et al., 2000; Palma and Matano, 2009; Combes et al., 2021b, 2023).
Demersal fishing expanded to slope regions of the BMM in the late 1990’s, a process that involved both the national trawl fleet and foreign fishing vessels authorized to fish in Brazilian waters (see review in Perez et al., 2009a). The former generally occupied the shelf break and upper slope (100 – 500 m depths), aiming at multispecies finfish and shellfish concentrations that included the argentine hake (Merluccius hubbsi), the codling (Urophycis mystacea), the monkfish (Lophius gastrophysus), and the argentine squid (Illex argentinus). The foreign fleet started to operate in 1999 and concentrated in the BMM, along Campos, Santos and Pelotas Basins and across the upper and lower slope regions (Figure 1; Supplementary Figure 1). The upper slope included multispecies trawling and gillnet operations targeting the monkfish. The lower slope (500 – 1000 m depths), was initially explored by pot fishing vessels aiming at the red crab Chaceon notialis in the southern Pelotas Basin (south of 33°S) and the royal crab Chaceon ramosae in the Santos Basins. By the end of 2002 a foreign trawl fleet occupied the lower slope targeting valuable concentrations of three deep-sea shrimp species: Aristaeopsis edwardsiana (scarlet shrimp), Aristaeomorpha foliacea (giant red shrimp), and Aristeus antillensis (alistado shrimp). Operations reached 1100 m depths but most profitable concentrations were limited to a narrow bathymetric band between 700 – 750 m isobaths. Slope exploration by deep-sea shrimp trawlers started by productive grounds of the Santos Basin, expanding, after 2005, to the northern Pelotas Basin and Campos Basin (Dallagnolo et al., 2009). By 2008 this fishery also was interrupted, ending the foreign deep-sea fishing episode in Brazil (Perez et al., 2009a).
2.2 Geomorphometry
For the purpose of this study, the slope region of the BMM was delimited by the 200 and 3359 m isobaths and the latitudes 19.00°S and 35.24°S (Figure 1), including the southern sector of Espírito Santo, Campos, Santos and Pelotas Basins. A description of the seabed morphology and a classification of geoforms in the BMM slope region was conducted using a Digital Bathymetric Model (GEBCO Compilation Group, 2021) with 490 m cell-size. This dataset was filtered to remove spurious depth records and submitted to an interpolation procedure to fill in the removed values. Subsequently, a low-pass filter was applied to smooth the data, reduce local variation and remove noise. Bathymetry data were transformed into secondary-derived terrain variables using the algorithm package Benthic Terrain Modeler (BTM) (Walbridge et al., 2018) contained in ArcMap Desktop 10.7.1. A general description of the seafloor morphology combined the visual analysis of the terrain variables that expressed surface gradients (slope, aspect northness, aspect eastness, flow direction), relative depth (Bathymetric Position Index – BPI) and surface rugosity (Vector Roughness Measurement – VRM) (Wilson et al., 2007; Walbridge et al., 2018). A seabed classification procedure was conducted using BTM’s Classify Benthic Terrain tool, which operates with bathymetry and two terrain variables: BPI (broad and fine) and slope. BPI is a neighborhood analysis function of the mean depth around each cell in the Digital Bathymetric Model. Positive values correspond to features and regions that are higher than the surrounding area (e.g., ridges); negatives values represent depressions of the seafloor. Bathymetric profiles of BPI-broad (annulus = 300 pixels ~ 36900 m), BPI-fine (annulus = 60 pixels ~ 7380 m) and slope were extracted from a series of sections across the shelf-slope gradient and graphically analyzed in order to identify maximum and minimum values for visually identified geoforms (classes) (Erdey-Heydorn, 2008). A classification table summarizing these upper and lower limits was used as input data for semi-automated classification of new adjusted classes by BTM’s Classify Benthic Terrain tool. A final spatial configuration of the resulting classes was obtained after a supervised interactive procedure, in which the seabed classification was conducted repeatedly after visual analysis of the adjusted classes and their spatial limits. In each model run, the upper and lower limits of variables in the classification table were fine-tuned until the spatial configuration contained a spatially persistent set of classes, which were also coherent with other terrain variables.
2.3 Fishing data analysis
Analyzed fishing data included 23656 fishing hauls of the foreign fishing fleet monitored by observers between 2000 and 2007 in the BMM. These included bottom trawls (70.2% of total hauls), gillnet settings (16.2%) and pot settings (13.7%) (Table 1). Records of each fishing haul included date, geographic position of the start and the end of the haul, and biomass (in kg) of all non-discarded species. The analysis considered the recorded catches of all species targeted by fishing operations: upper slope trawl (monkfish, argentine hake, codling and argentine squid), lower slope trawl (scarlet shrimp, giant red shrimp and alistado shrimp), gillnet fishing (monkfish) and pot fishing (red crab, royal crab). Centroids of fishing hauls (midpoint position between positions of the start and the end of the hauls) were plotted on the Digital Bathymetric Model of the BMM (GEBCO Compilation Group, 2021). The sum of fishing hauls and catches (in kg) obtained within each seabed feature (classes) were compared to the surface area (km2) contained within each feature.
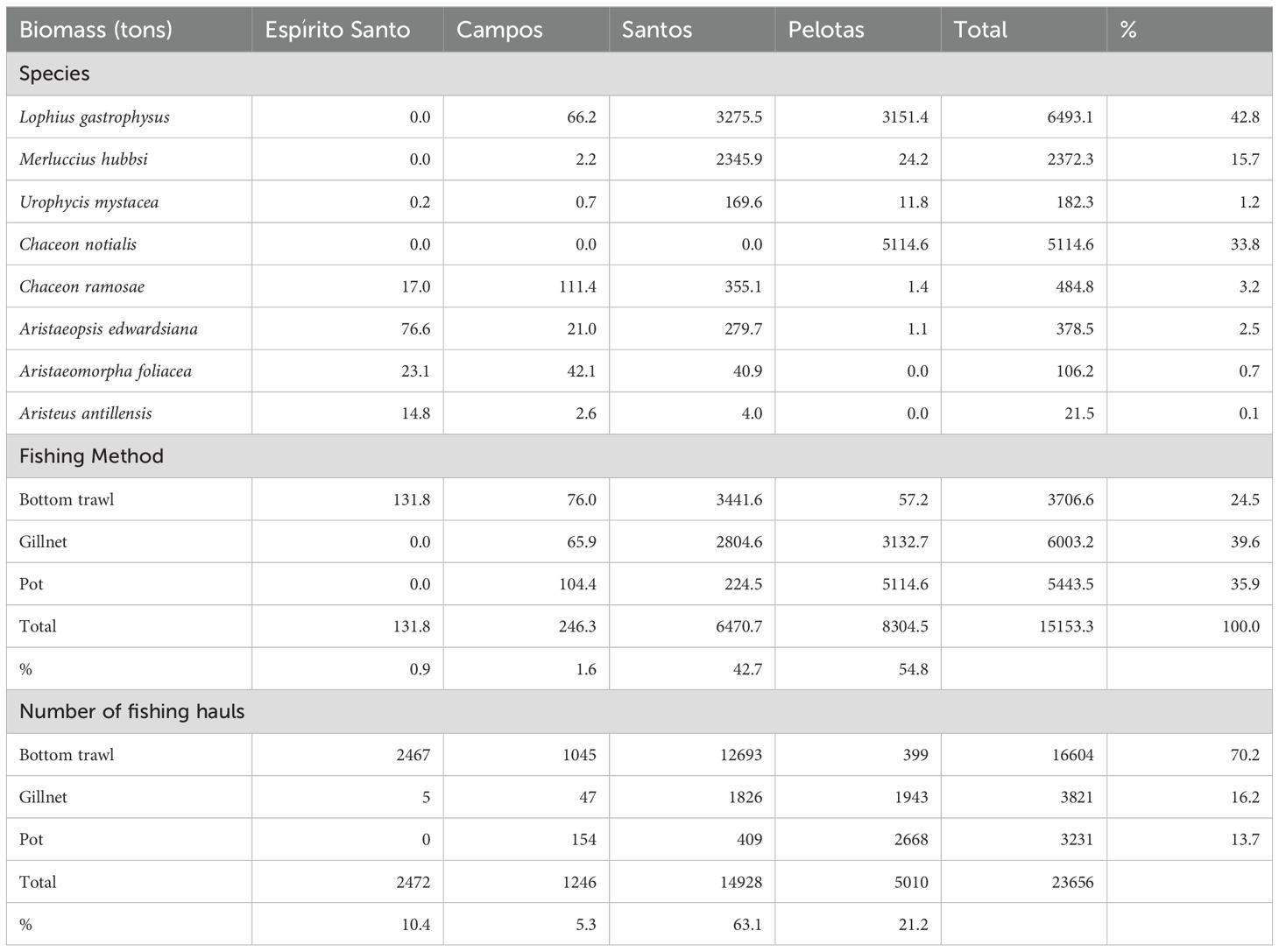
Table 1. Summary of demersal fishing operations conducted on the slope regions of the Brazilian Meridional Margin between 2000 and 2007.
A quantitative analysis was conducted to assess the effect of along-slope and across-slope geoforms on the spatial variability of species occurrence and abundance, expressed as catch-per-unit-effort (CPUE), of all catches combined and by taxonomic groups: fishes, deep-sea shrimps, deep-sea crabs and squids. The identification of productivity hotspots resulted from the analysis focusing on all groups of megafauna, however, the analyses of fisheries data by taxonomic groups was considered necessary because: (a) variable responses among taxonomic groups could better inform about the robustness of productivity hotspots, and (b) the spatial patterns of the total catches combined could be affected by the different fishing gear/fishing fleets that targeted almost exclusive taxonomic groups (see below). In that sense, because fishing gear and taxonomic groups were correlated, they were not included as explanatory variables in the quantitative analyses.
The fishing area was divided in 1.9 x 1.9 km pixels, and, in each of them, the CPUE was computed dividing the total amount of catch (in kg) by the total amount of hauls of all fishing gear combined. CPUE, expressed as average catch per haul, followed a skewed zero-inflated distribution, which required the use of a Delta-Lognormal Generalized Linear Model (GLM). This kind of hurdle model (Cragg, 1971) allows modeling the probability of observing zero catches as a function of the explanatory variables separately from the model fitted for the non-zero catches (Lo et al., 1992; Maunder and Punt, 2004). The general form of the delta model was
where w is the probability of observing a zero for the response variable (CPUE) and f(y) is a model of the mean of the non-zero values in the CPUE (Maunder and Punt, 2004). For the purpose of this analyses, the proportion of positive CPUEs (1 – w) was fitted using a binomial GLM with a logit link function, and the positive CPUEs were fitted using a lognormal GLM.
The explanatory variables included in both models were all categorical, comprising along-margin and across-margin geoforms (Table 2, Figures 2, 3). The explanatory variable ‘slope-profile’ described seabed morphology across the slope. It comprised four categories (levels) formed by grouping negative (depressions) and positive (crests) classes obtained by the seabed classification procedure (Figure 3). A series of ‘embayments’ that are arc-shaped sectors of the shelf break, characterized the along-margin morphology (delimited by boxes A to F, Table 2, Figure 2A). Within these embayments, the ‘E-contour’ variable described the embayment curvature, which included the ‘protrusion’ and the ‘incurvate’ sectors (Table 2, Figure 2B), and the ‘E-profile’ variable described the embayment depth profile, which included the ‘deposition’ and the ‘erosion’ sectors (Table 2, Figure 2C). Because the Campos and Espírito Santo Basins were less explored by fishing fleets and differ in morphology and complexity (see results), the quantitative analyses were limited to the Santos and Pelotas basins.
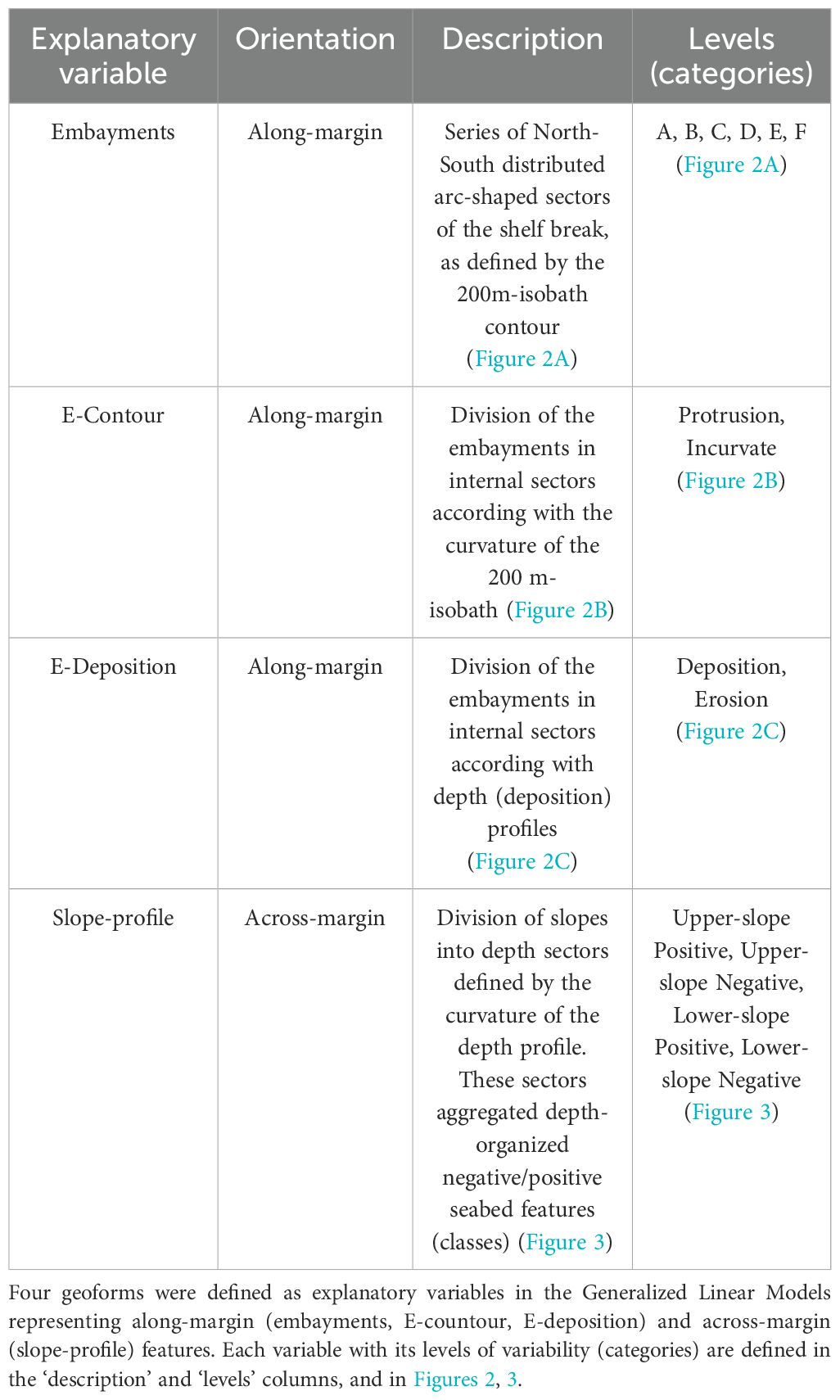
Table 2. Summary of geoforms considered to explain the spatial variability of deep-sea fauna in the Brazilian Meridional Margin.
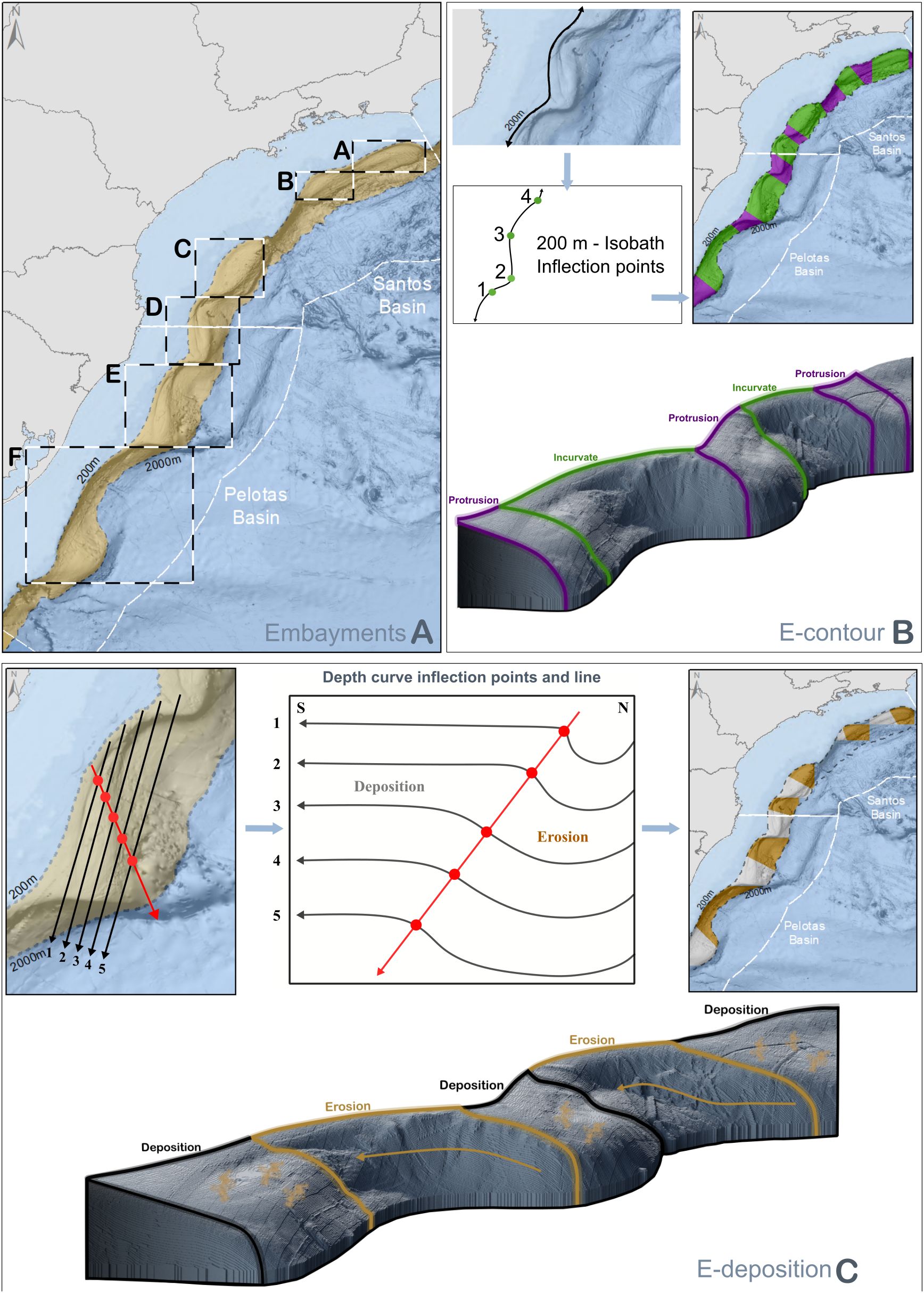
Figure 2. Spatial delimitation of geoforms of the Brazilian Meridional Margin (Santos and Pelotas Basins) affecting deep-sea demersal catches. These geoforms are also described in Table 2. (A) Boxes A to F delimit shelf break embayments along the BMM; north/south and east/west limits of the boxes were defined by the 200 - 2000m isobaths; (B) Embayment contour (E-contour), protrusions and incurvate sectors of the embayments were delimited by inflection points in the contour of the 200 m isobaths; (C) Embayment deposition (E-deposition), erosion and deposition sectors within embayments were delimited by plotting parallel along-slope bathymetric profiles and defining a line connecting their inflection points.
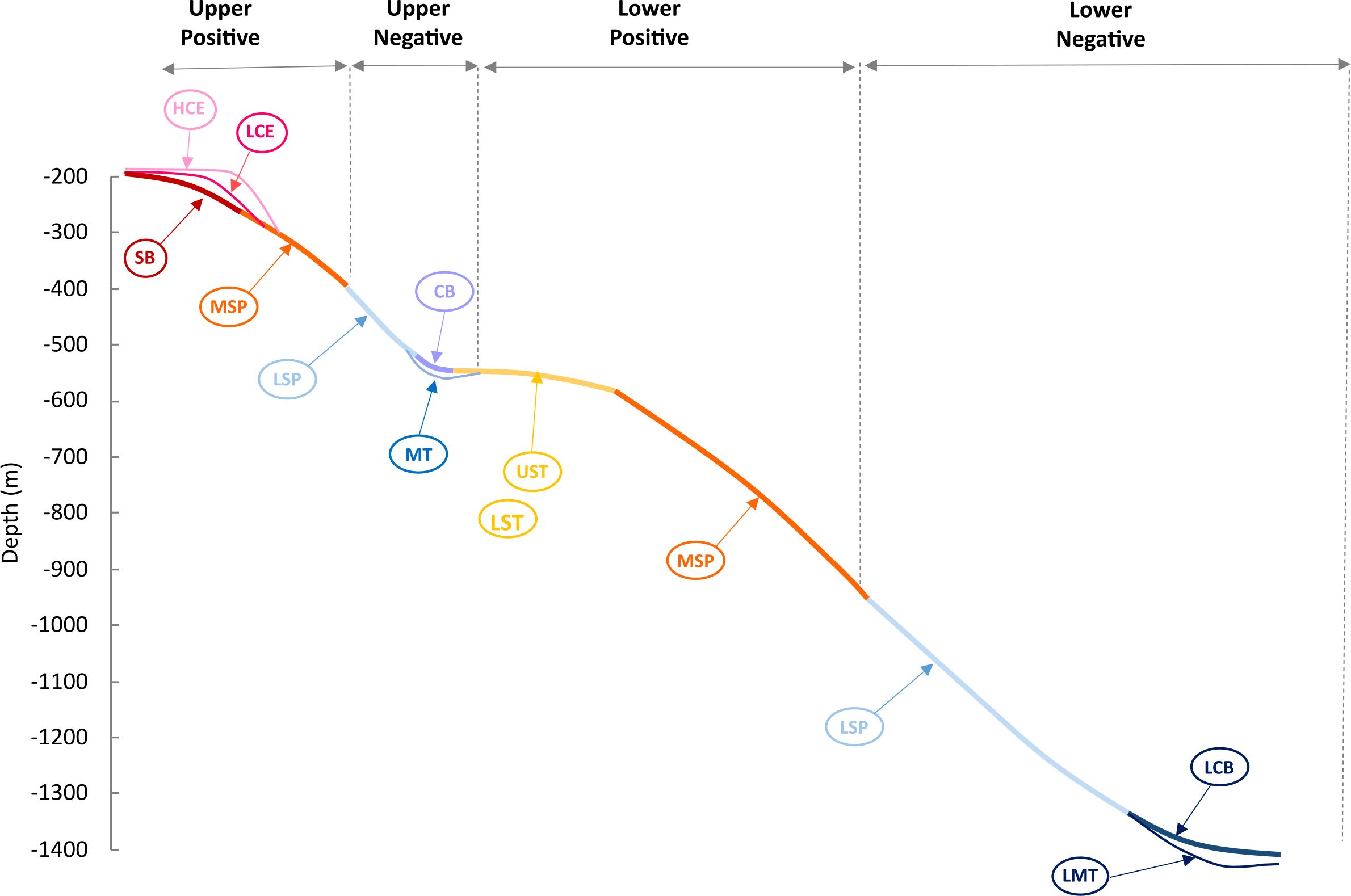
Figure 3. Across-slope distribution of bathymetry-derived seabed classes (geoforms) in a typical slope profile of the Santos and Pelotas Basins, Brazilian Meridional Margin. Full descriptions of these classes are presented in Supplementary Table 1. SB, Shelf Break; LCE, Low Cliff Edge; HCE, High Cliff Edge; MSP, Moderate Slope; UST, Upper Slope Terrace; LST, Lower Slope Terrace; LSP, Lower Slope; CB, Cliff Base; MT, Moat; LCB, Lower Cliff Base; LMT, Lower Moat. Also, in the figure is presented the four levels (categories) of the explanatory variable ‘slope profile’ included in the Generalized Linear Models (see Table 2). These levels comprised groups of classes along the depth profile: upper positive (HC, LC, SB, MSP), upper negative (LSCP, MT, CB), lower positive (UST, LST, MSP) and lower negative (LSP, LCB, LMT).
Model selection was based on a series of GLMs fitted using all possible combinations of the categorical explanatory variables evaluated in this analysis. The choice of best fit was based on the Akaike’s Information Criterion (AIC) method and in the analysis of deviance (Maunder and Punt, 2004; Sant’Ana and Perez, 2016). Covariates that were not statistically significant (deviance test, α= 0.05) were dropped from the final best fit model. Multiple comparisons of means z-tests (or asymptotic t-tests), using a Tukey contrasts matrix, were implemented for the lognormal and binomial components of the final delta-lognormal model, in order to assess the distinct effects of explanatory variable categories (levels) (Hothorn et al., 2008).
3 Results
3.1 Shelf break and slope geoforms
Terrain variables extracted from the Digital Bathymmetric Model described the general geomorphology of the shelf break and slope region of the BMM (Figure 4). The main geoforms derive from slope sedimentation processes, in particular, the development of contourite bodies formed by seafloor abrasion of currents following the bathymetric contours. Along the Santos and Pelotas basins, these bodies extend in a sequence of east-southeast-oriented embayments which contain terraces of variable widths formed by sediment drifts and erosional processes (terraces) (Figure 4). Elevated terrain (demonstrated by positive BPI-broad and BPI-fine) and increased sloping surfaces delineated the oceanward edges of these terraces (Figures 4A-D). Slope seafloor was generally smooth in the entire BMM, except for localized rugged areas at steep slopes and regions that border existing canyons (Figure 4F).
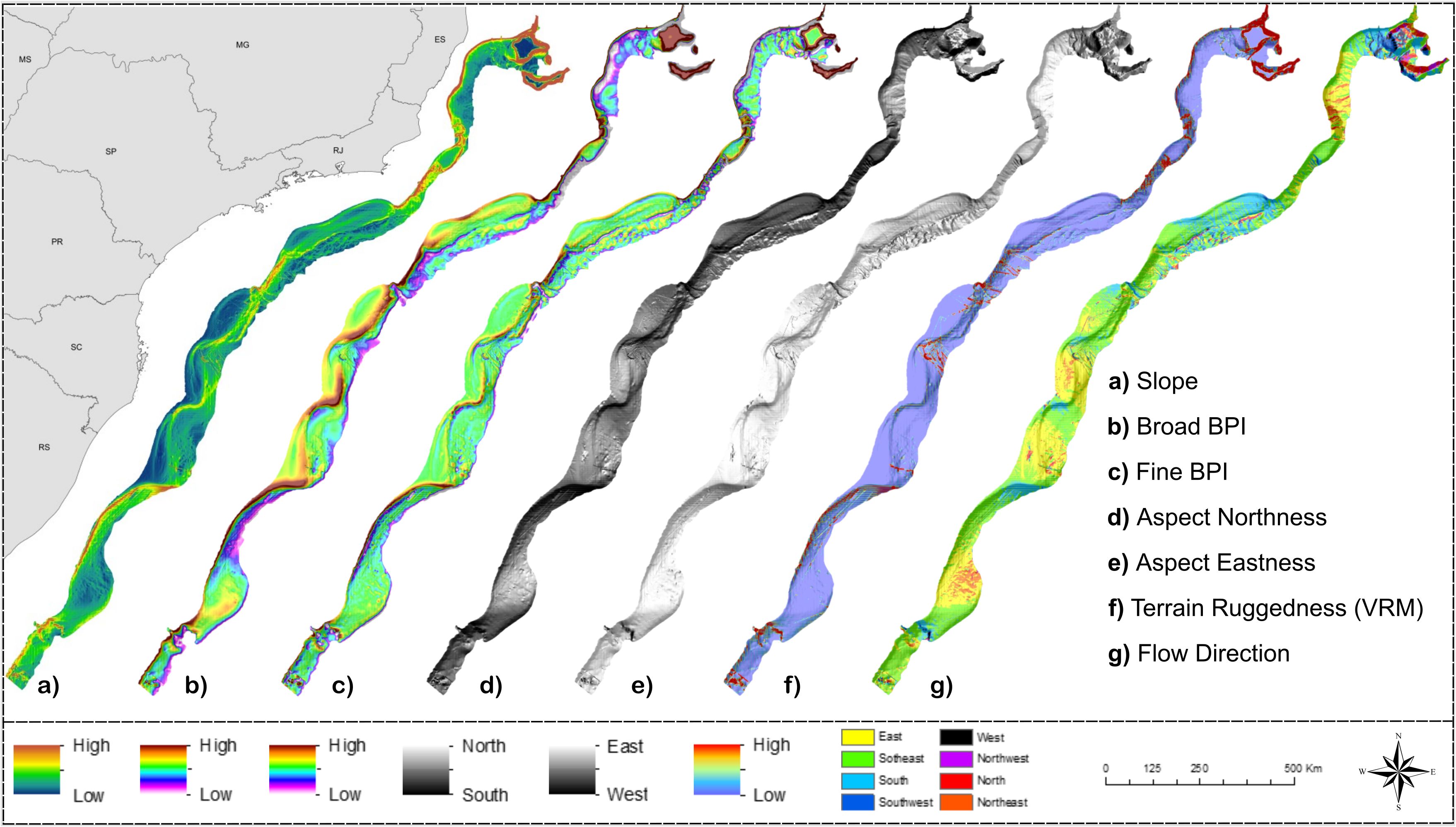
Figure 4. Terrain variables derived from the Digital Bathymetry Model of the Brazilian Meridional Margin. Projection World Mercator/WGS 84. Datum UTM 25 South.
The benthic terrain modelling procedure classified the BMM seafloor into 12 classes (Figure 5; Supplementary Table 1) which tended to describe the general topography across the shelf-slope depth gradient; six of them elevated from the surrounding seafloor (i.e. positive) and six of them were depressions (i.e. negative) (Supplementary Table 1). The ‘Shelf Break’ (SB), ‘High Cliff Edge’ (HCE) and ‘Low Cliff Edges’ (LCE) classes delineated the transition between flat and sloping seafloor areas. They usually occurred between the shelf edge and the upper slope, but they were also observed at the edge of terraces. ‘High Cliff Edge’ (HCE) and ‘Low Cliff Edges’ (LCE) classes described more abrupt transitions than the ‘Shelf Break’ (SB) (Figure 3). The sloping seafloor (1.5 – 2.0°) was classified into two classes: ‘Moderate Slope’ (MSP), a slightly positive feature, and the ‘Lower Slope’ (LSP), a slightly negative feature. These are the most extensive classes in the BMM, occupying 19.2 and 24.5% of the seafloor area, respectively (Supplementary Figure 2; Supplementary Table 1). In a large portion of the BMM area, the sloping seafloor is interrupted by relatively flat terraces classified into ‘Upper Slope Terrace’ (UST) and ‘Lower Slope Terrace’ (LST). The transition between sloping seafloor (classes ‘Moderate Slope’ and Lower Slope’) and these terraces (‘Upper Slope Terrace’ and ‘Lower Slope Terrace’) was usually characterized by the moderately negative ‘Cliff Base’ (CB) or a deeper, channel-like feature, here named ‘Moat” (MT) (Figure 3). At greater depths (~1700 m on average), similar transitions were classified into distinct classes: the ‘Lower Cliff Base’ (LCB) and ‘Lower Moat’ (LMT). Terrace areas (‘Upper Slope Terrace’ and ‘Lower Slope Terrace’) and slope-terrace transition areas (‘Cliff Base’ and ‘Moat’) occupied significant proportions of the BMM area; 14.4% and 18.0%, respectively (Supplementary Figure 2, Supplementary Table 1). The general cross-slope seabed classification presented in Figure 3 characterized most embayments of Santos and Pelotas Basins (Figure 5). Contrastingly, 30% and 24% of the area of the narrow and steep margins of Espírito Santo and Campos basins, were dominated by seafloor depressions, namely the Moat (MT) class (Figure 5).
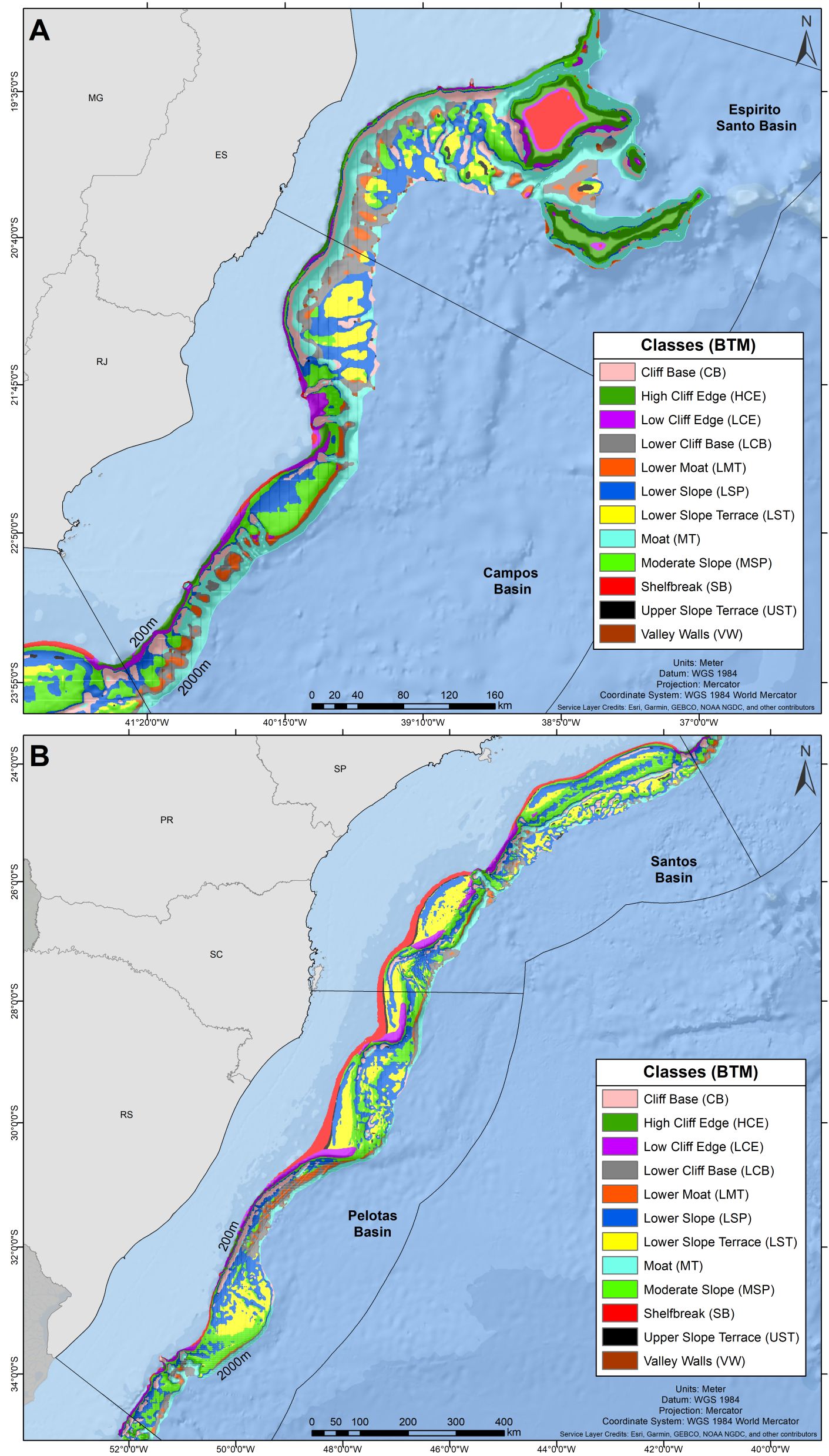
Figure 5. Classification of the shelf break/slope seabed of the Brazilian Meridional Margin (classes) based on bathymetry-derived terrain variables. (A) Espírito Santo and Campos Basins; (B) Santos and Pelotas Basins.
3.2 Slope morphology and catches
All fishing hauls included in this analysis produced, jointly, 15153 t of landed biomass during the studied period. Gillnet, trawl and pot operations accounted for 39.6%, 35.9% and 24.5% of the total landed biomass, respectively. The monkfish, the red crab and the argentine hake were the most abundant species in the catches, accounting for 42.8%, 33.8% and 15.7% of the total landed biomass, respectively (Table 1). Catches of demersal fishes (argentine hake, codling, monkfish) were originated from gillnet settings (67%) and bottom trawls conducted in the shelf break and upper slope (33%). Deep-sea crab catches originated from pot fishing (98%) and bottom trawls (2%). All catches of squid (argentine shortfin squid) and deep-sea shrimps (red giant shrimp, scarlet shrimp and alistado shrimp) originated from bottom trawls.
Over 97% of the overall catch originated from fishing operations in Santos (42.7%) and Pelotas (54.8%) sedimentary basins (Table 1). Within these basins, demersal catches were recorded on 11 of the 12 seabed classes described, but in some of them catches were particularly abundant. In Santos basin, the ‘Moderate Slope’ class (MSP) (Figure 3), covering 22% of the available fishing area, concentrated 55.7% of all fishing hauls and 38.6% of the catch (Figure 6). In Pelotas basin, the ‘Low Cliff Edges’ (LCE), at the transition between the shelf and slope regions (Figure 3), represented only 6% of the fishing area available but concentrated 29.5% of the fishing hauls and 30.2% of the total catch (Figure 6). The spatial distribution of fishes, deep-sea shrimps, deep-sea crabs and squid abundance (kg/haul) is presented in Figure 7, in relation to the spatial distribution of ‘Moderate Slope’ and ‘Low Cliff Edges’ classes. In most cases, higher abundances can be observed in the incurvate sectors of the embayments, over the upper (fishes and squid) and lower (deep-sea shrimps, deep-sea crabs) slope, mostly covered by ‘Moderate Slope’ and ‘Low Cliff Edges’ classes. These classes, however, extended to deeper areas of the slope, where catches were not abundant (Figure 7).
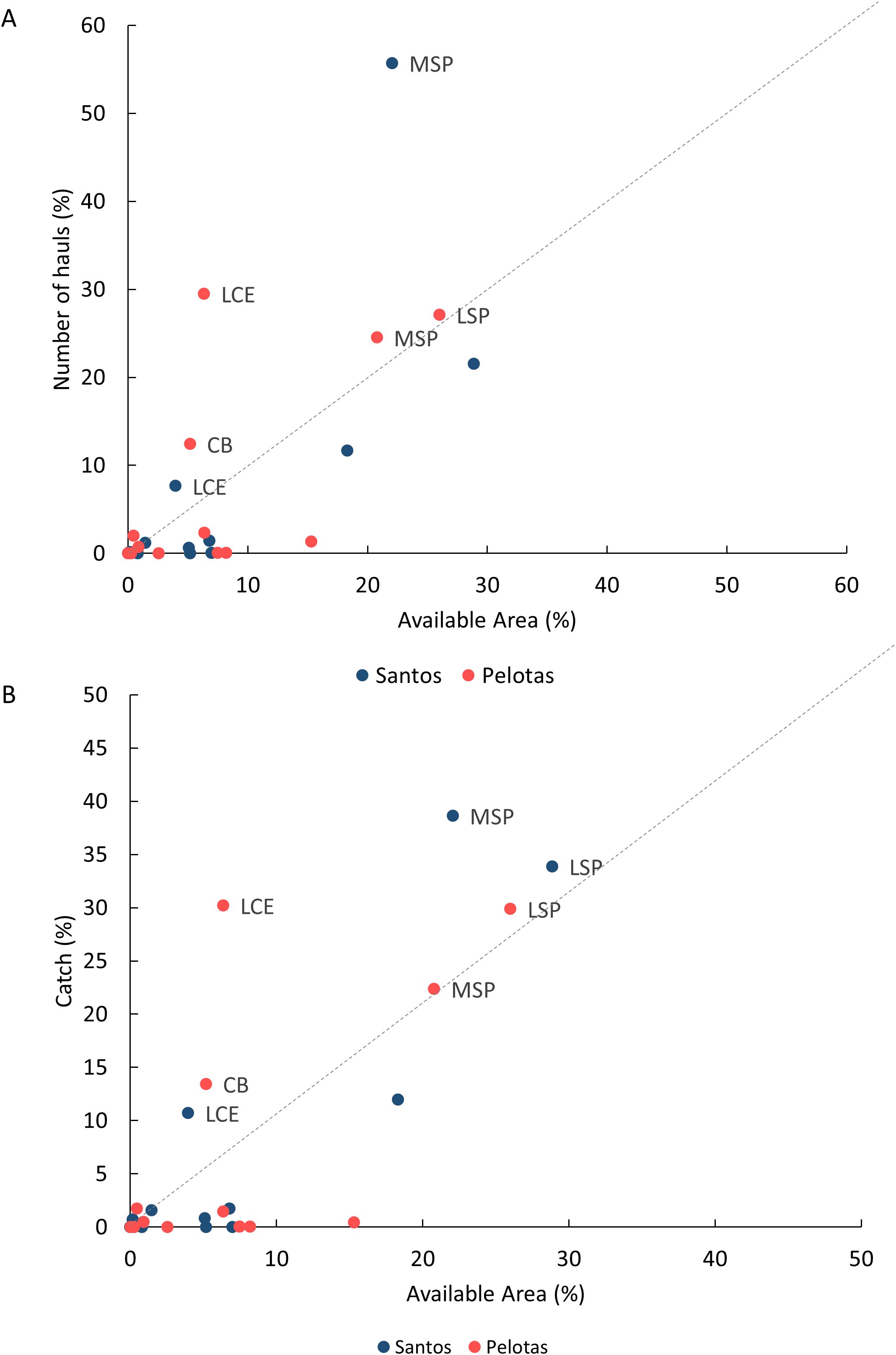
Figure 6. Relative importance of geoforms for slope catches in the Brazilian Meridional Margin. The plot compares total number of hauls (A) and the total reported catch (B) of the demersal fisheries (in %) with the area made available by each geoform (class) for fishing (in % of total slope area). Dot colors represent the sedimentary basins. Geoforms plotted above the dotted line were those that contained a relatively small area but included a relatively large proportion of the fishing hauls/catches. Only these features were labelled for identification: CB, Cliff Base; LCE, Low Cliff Edge; MSP, Moderate Slope; LSP, Lower Slope.
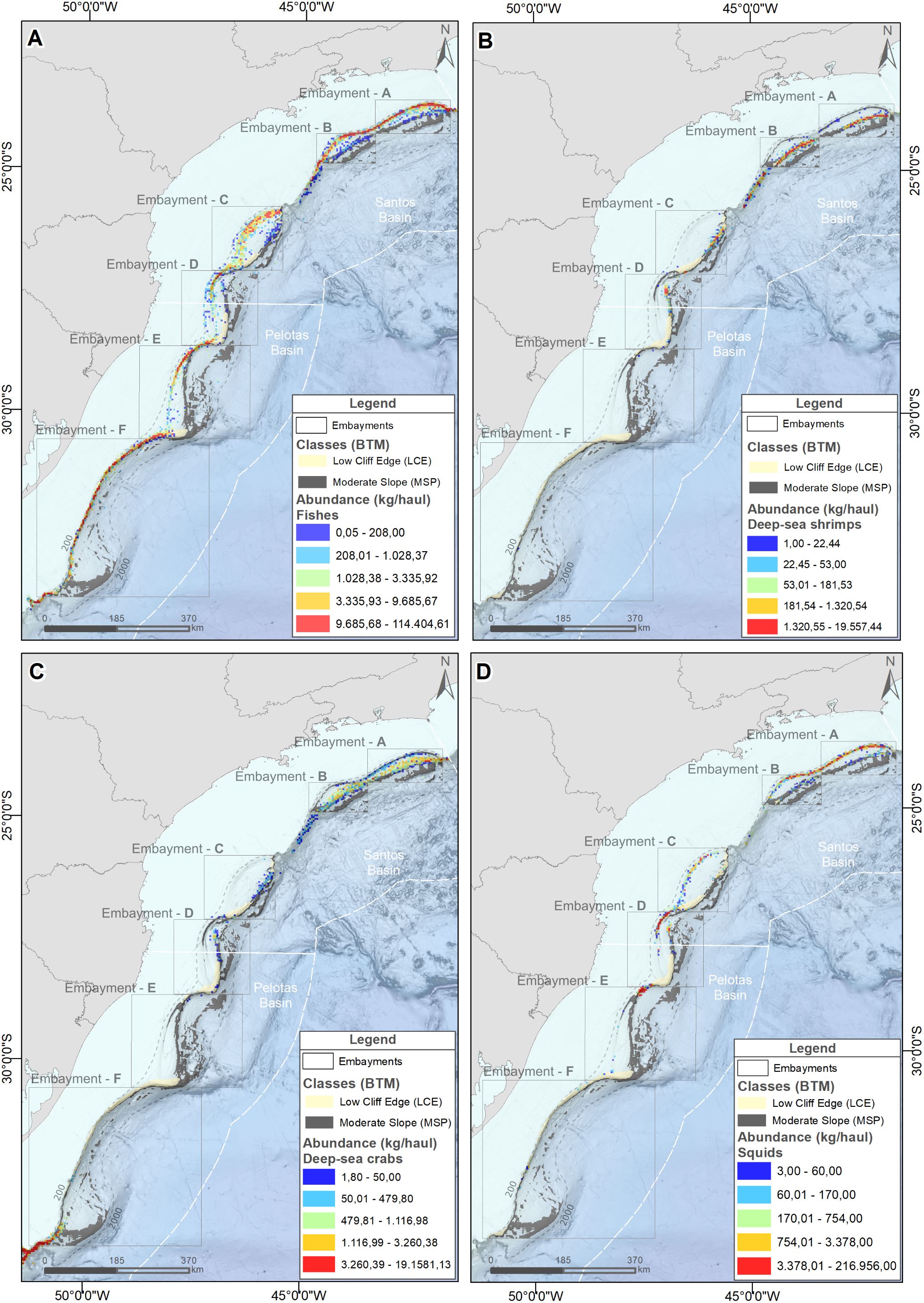
Figure 7. Spatial distribution of the abundance (kg/haul) of fishes (A), deep-sea shrimps (B), deep-sea crabs (C) and squids (D) on the Brazilian Meridional Margin. The spatial distribution of two geoforms (cross-slope classes) are also indicated: ‘moderate slope’ (MSP) and ‘Low Cliff Edge’ (LCE).
The delta-lognormal GLM measured the effect of the shelf break/slope geoforms (Table 2) in both the probability of obtaining positive catches (occurrences) and on the abundance of all catches combined and of the different taxonomic groups. Table 3 presents the effect of the geoforms (explanatory variables) in the best fit models, as defined by highest AICs (see Supplementary Tables 2–11). Residuals of lognormal models fitted to non-zero CPUEs tended to be uniformly distributed (Supplementary Figures 3–7). Best fit binomial models explained only 7.7% of all catches combined, but as much as 67.3% and 49.5% of the variance of deep-sea shrimps and crabs, respectively (Table 3). Best fit lognormal models selected to fishes, all catches combined, and squids explained 77.3%, 65.6% and 36.7% of the variance, respectively. These models explained less of the variance in deep-sea shrimps (13.6%) and deep-sea crabs (9.0%) (Table 3).
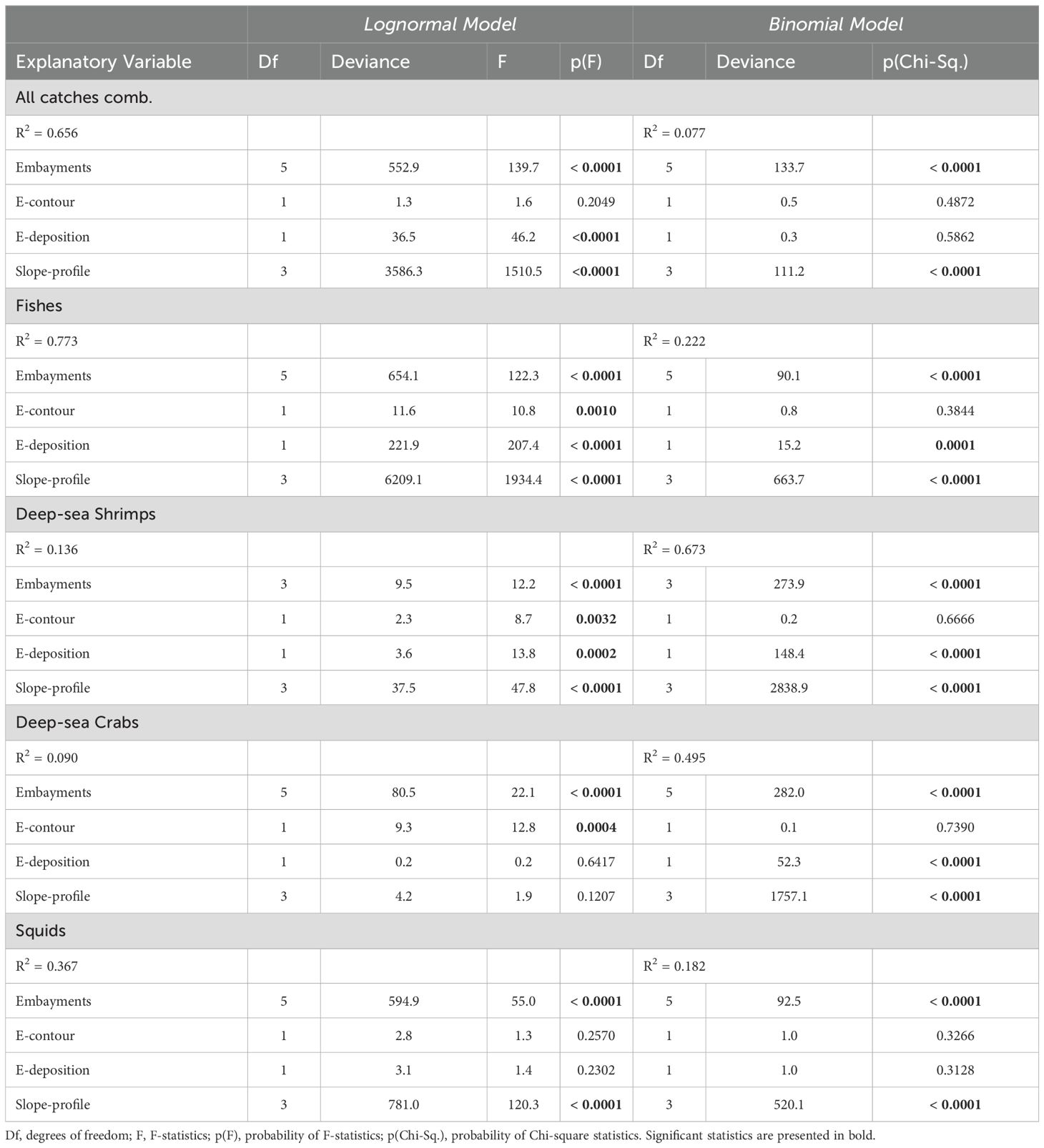
Table 3. Effect of slope geoforms (explanatory variables) on the abundance (CPUE, Lognormal Model) and the occurrence of positive catches (Binomial Model) of all catches combined, fishes, deep-sea shrimps, deep-sea crabs and squid captured by demersal fisheries in the Brazilian Meridional Margin between 2000 and 2007.
Across-slope features
The slope-profile explanatory variable significantly affected the probability of a positive occurrence of all catches combined and all taxonomic groups (binomial models, Table 3). It also had a significant positive effect in the abundance, except for the deep-sea crabs (lognormal model, Table 3). The probability of positive occurrence of all catches combined was significantly higher on lower slope sectors (Figure 8). This pattern was also observed in fishes and, particularly, deep-sea shrimps and crabs, but not squid, which was more likely to be caught in the upper slope sectors (Figure 9). The abundance of all catches combined was higher in upper slope sectors (Figure 8), which essentially reflected the pattern exhibited by fishes and, to a lesser extent squid, whereas higher abundances of deep-sea shrimps were observed on lower slope sectors (Figure 10).
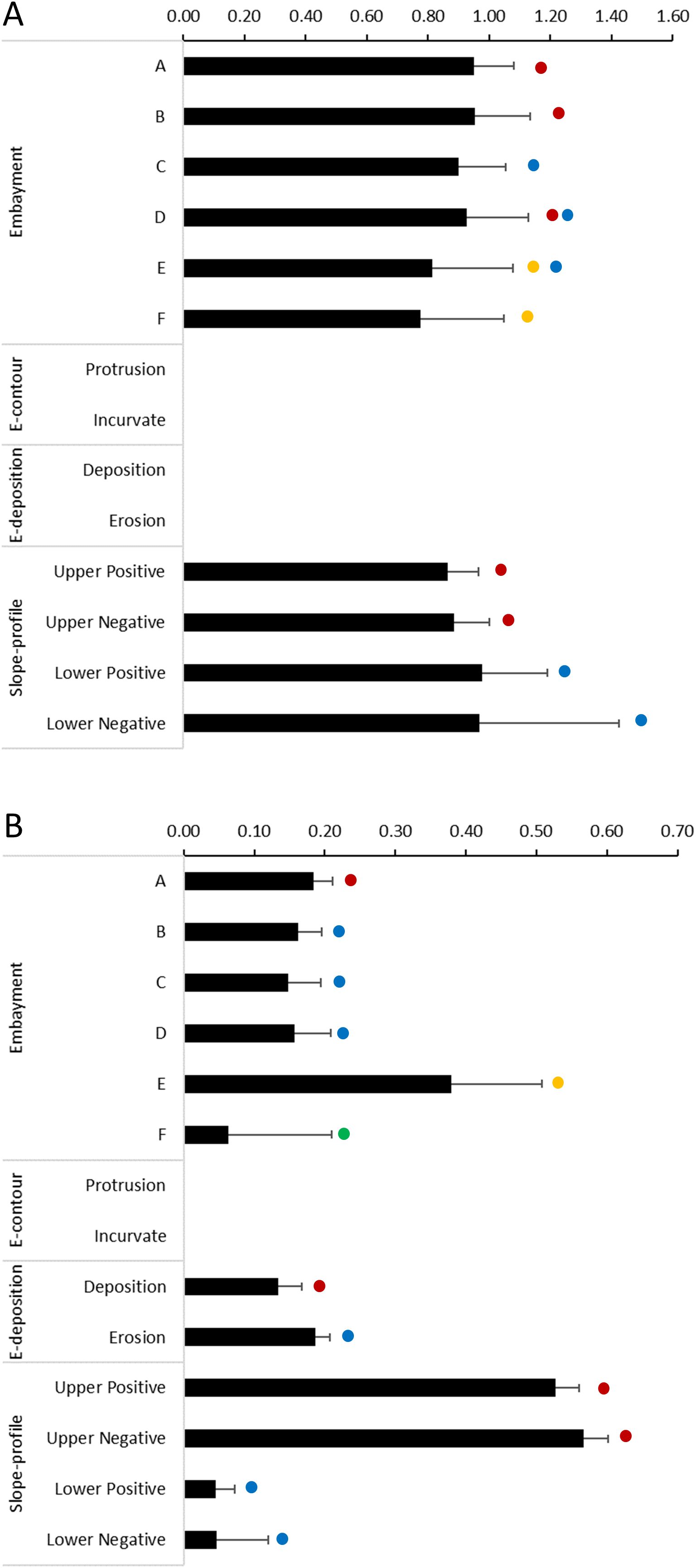
Figure 8. Effect of geoforms on catches of demersal megafauna (all catches combined) on slope regions of the Brazilian Meridional Margin. These effects (bars + Standard Error) result from the application of a Delta-Generalized Linear Model (GLM) where (A) depicts results of the binomial GLM using presence-absence data and (B) depicts results of the lognormal GLM using catch-per-unit-effort of positive fishing hauls. Dots with the same colours indicate the categories within each explanatory variable (embayments, E-contour, E-deposition, Slope-profile) that are not significantly different (multiple comparisons of means z-tests using a Tukey contrasts matrix, p>0.05).
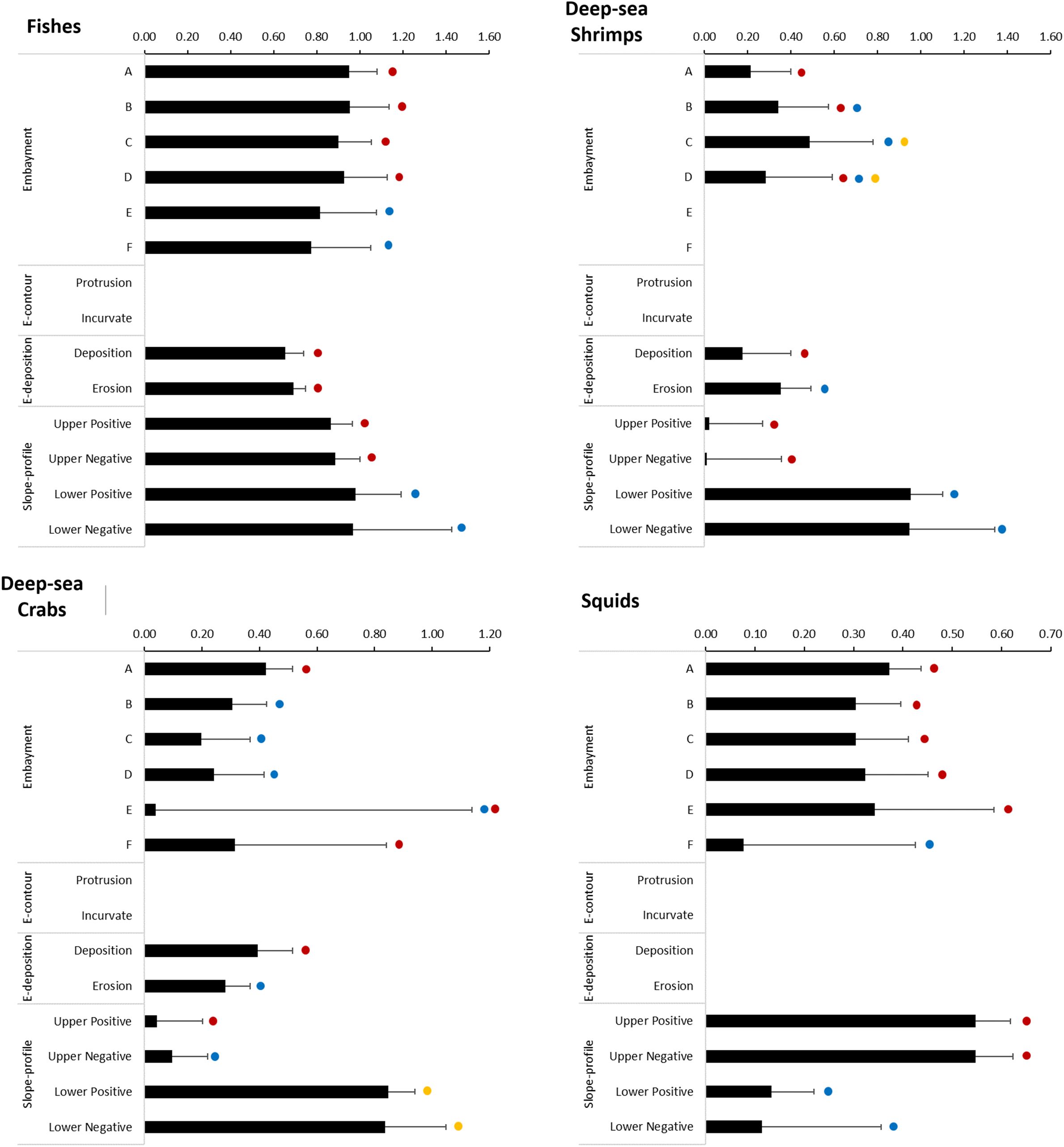
Figure 9. Effect of geoforms on the occurrence of positive catches of fishes deep-sea shrimps, deep-sea crabs and squids, on slope regions of the Brazilian Meridional Margin. These effects (bars + Standard Error) result from the application of a binomial Generalized Linear Model using presence-absence data. Dots with the same colours indicate the categories within each explanatory variable (embayments, E-contour, E-deposition, Slope-profile) that are not significantly different (multiple comparisons of means z-tests using a Tukey contrasts matrix, p>0.05).
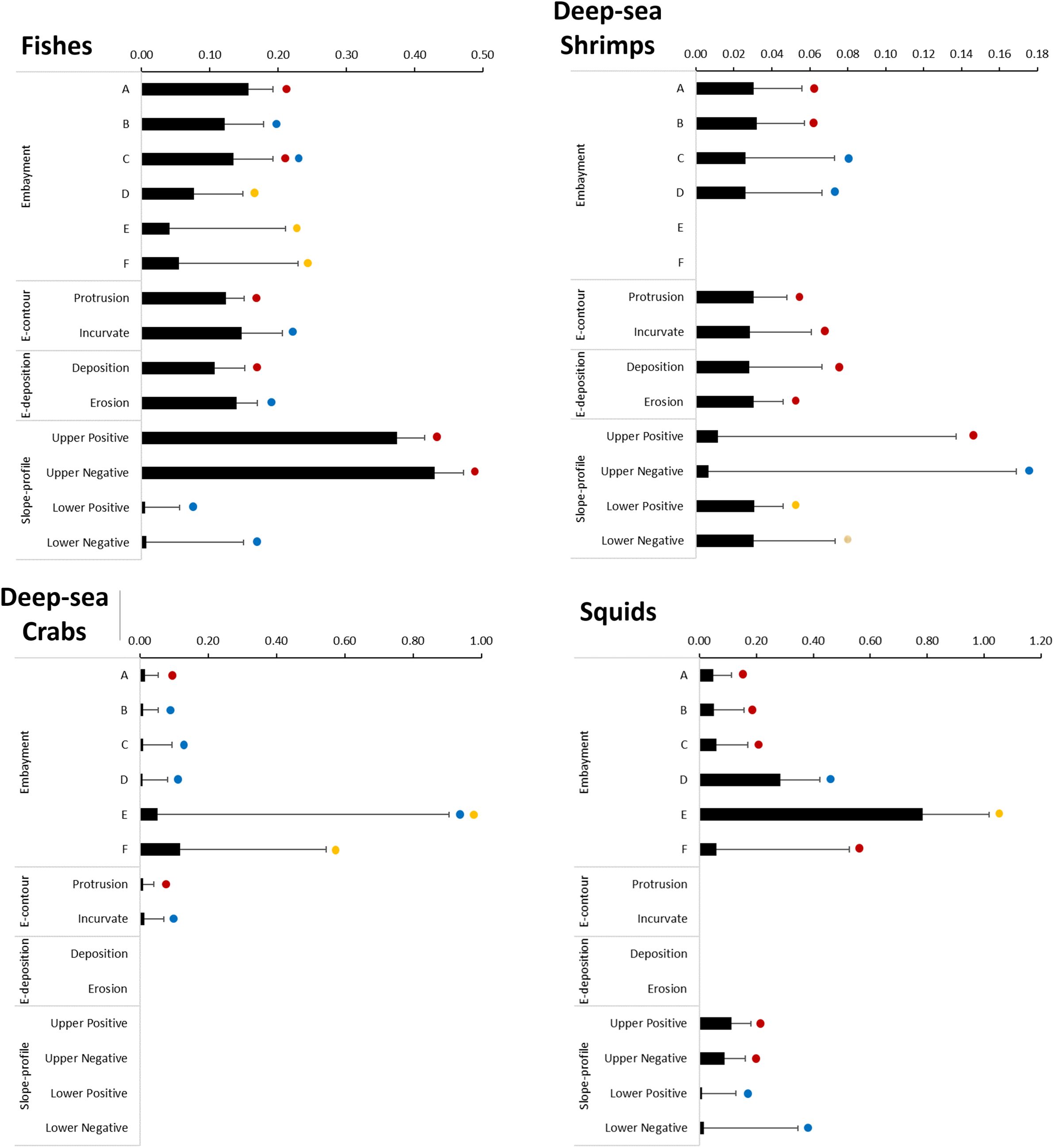
Figure 10. Effect of geoforms on the abundances of fishes, deep-sea shrimps, deep-sea crabs and squids on slope regions of the Brazilian Meridional Margin. These effects (bars + Standard Error) result from the application of a lognormal Generalized Linear Model using catch-per-unit-effort of positive fishing hauls only. Dots with the same colours indicate the categories within each explanatory variable (embayments, E-contour, E-deposition, Slope-profile) that are not significantly different (multiple comparisons of means z-tests using a Tukey contrasts matrix, p>0.05).
Along-slope features
The series of embayments distributed along the edge of the continental margin significantly affected the probability of positive occurrence (binomial model) and the abundance (lognormal model) of all catches combined and all taxonomic groups (Table 3). The probability of positive occurrence of all catches combined was significantly lower in the southern embayments (E and F, Figure 8). The same pattern was observed in fishes, but tended to vary in other taxonomic groups: deep-sea shrimps were more likely to be caught in embayment C, deep-sea crabs in embayments A and F, and squids in all embayments except F (Figure 9). The abundance of all catches combined was particularly high in embayment E (Figure 8) which reflects the pattern exhibited by squid abundance (Figure 10D). The abundance of fishes and deep-sea shrimps tended to be higher in the northern embayments (Figures 10A–C).
The embayment contour (E-contour) did not affect significantly the probability of positive catches (binomial model), but it did affect the abundance (lognormal model) of fishes, deep-sea shrimps and crabs (Table 3). The abundances of deep-sea shrimps were similar in both protrusion and incurvate sectors, but higher abundances of fishes and deep-sea crabs were observed in the incurvate sector of the embayments (Figure 10).
The embayment deposition (E-deposition) affected significantly the probability of positive catches of fishes, deep-sea shrimps and crabs (binomial model) (Table 3). It also affected the abundance (lognormal model) of fishes, deep-sea shrimps and all catches combined (Table 3). All catches and deep-sea shrimps were more likely to be caught in the erosion sector (Figures 8, 9). The probability of catching fishes was similar in both deposition and erosion sectors, whereas deep-sea crabs were most likely to be caught in the deposition sector (Figures 8, 9). The abundance of all catches combined was higher in the erosion sector (Figure 8), a pattern also observed in fishes, but not deep-sea shrimps in which abundance was similar in both sectors (Figure 10).
4 Discussion
During one decade (2000 – 2009) fully monitored demersal fishing operations captured fish, crustaceans and cephalopods on the largely unexplored deep seafloor of the Brazilian Margin, covering a wide range of latitudes, depths and geoforms (Perez et al., 2009a; Sant’Ana and Perez, 2016). Because these were commercial operations seeking profit, they identified aggregations of targeted species, the spatial distribution of which were shown to be affected by geoforms extending along- and across the shelf break and slope. Fishes, deep-sea shrimps, crabs and squid exhibited variable responses to these features. Notwithstanding, there were general patterns emerging particularly in models that explained higher fractions (>50%) of the total variance, namely those fit to the abundance of all catches combined (65.6%), to the abundance of fishes (77.3%), and to the probability of positive catches of deep-sea shrimps (67.3%) (Table 3). These models highlighted the importance of shelf break embayments of Santos Basin and, within them, the sectors incurvated and excavated by erosive action of the Brazil Current (Figure 2). The upper ‘moderate slopes’ (300 – 500 m depths) were particularly favorable for fishes and squid concentrations, whereas the lower ‘moderate slopes’ (600 – 800 m depths) increased the probability of deep-sea shrimp positive catches. Because these patterns resulted from the analyzes of catches of different fishing fleets, their interpretation require an initial consideration as to what extent they may reflect the effect of fishing gears and the spatial dynamics of fishing operations rather than the species abundance patterns.
Firstly, it is important to note that the international fleets covered a previously unexplored region of the BMM, to identify profitable grounds of mostly virginal resources (see review in Perez et al., 2009a). The spatial patterns of their operations throughout this period, therefore, is the outcome of a process of finding high concentrations of the targeted species, which, in essence, optimized the delimitation of potential productivity hotspots. Vessels operating different gear had almost exclusive targets (i.e., the taxonomic groups considered) and their operations exhibited spatial patterns that tended to maximize the catch of these targets (Sant’Ana and Perez, 2016). As a consequence, it was possible to observe that catches of taxomonic groups tended to occur in most geoforms explored by the fleets (binomial model, Figure 8), but their abundance was higher only in specific ones (lognormal model, Figure 9), possibly due to natural conditions that favored their concentration. Demersal fishes could be an exception, since they were targeted by both upper slope trawlers and gillnet vessels. The latter, however, was responsible for nearly 70% of the catches and the effect of the spatial patterns of their operations predominated in the analysis. Therefore, it is plausible to conclude that the spatial patterns of abundance of the different taxonomic groups were not significantly affected by variable efficiencies of different fishing gear or spatial operation patterns of different fleets, but, on the other hand, these elements possibly affected results obtained in the analysis of all catches combined. Nevertheless, when these results were analyzed in the context of those obtained in the analyzes of the taxonomic groups separately, the spatial patterns of the areas regarded as productivity hotspots remained robust.
Demersal fishing was widely recorded in most cross-shelf geoforms (classes). However, fishing hauls and catches concentrated preferentially over few slope geoforms distributed along the entire BMM following topography breaks, i.e., the shelf break (300 – 500 m depths) and terraces break (600 – 800 m) (Figure 3). Higher abundances of the taxonomic groups were observed over these preferential geoforms, but only in certain portions of their spatial distribution (Figure 7). Therefore, it is uncertain whether the species aggregating factor was directly linked to the preferential geoforms or these were components of more complex biophysical processes operating on the slope of the BMM. Previous studies using the same catch records revealed that suitable habitats for deep-sea shrimps and monkfish were mostly driven by variables related to water masses impinging on the seafloor, surface productivity and deep-sea circulation (e.g. mixed layer depth, seafloor salinity and temperature, current speed and direction) (Sant’Ana, 2023). In the deep-sea, these variables interact with geomorphology, and particularly with abrupt topography, contributing to localized biophysical phenomena that may promote aggregation of predatory megafauna by enhancing prey supply in an otherwise generally impoverished deep seafloor (Genin, 2004; Maier et al., 2023). In that sense, the effect of geoforms on the occurrence and abundance of demersal megafauna in the BMM may be associated with biophysical processes resulting from their interaction with along-slope and across-slope circulation processes.
Deep-sea abrupt topographies (e.g. shelf break-slopes, seamounts, canyons) are known to aggregate zooplankton and fish through biophysical coupling mechanisms involving enhanced population growth and/or accumulation processes (reviewed by Genin, 2004). Growth of predatory fish populations has been attributed to (a) localized upwelling events that promote enhanced biological productivity propagating through the food web or enhancement of organic matter sink of dead organisms and fecal pellets during productivity peaks, or (b) other mechanisms that increase the supply of prey through zooplankton and fish responses to deep-water flow dynamics, referred to as ‘trophic subsidy’ (Gooday and Turley, 1990; Genin, 2004). Aggregations often reported on shelf break and slope regions have been associated with vertical water flow (upwelling or uplifting) that can either enrich waters in the photic zone long enough to enhance primary productivity and enable energy to be transferred through local food web, or provoking active responses of zooplankton that tends to become spatially aggregated and promptly available for predators.
In the Brazilian Meridional Margin, the offshore geostrophic flow of the BC transports generally warm and oligotrophic water masses. Yet topographically-induced mesoscale (100s of km) and sub-mesoscale (10s of km) processes of the BC result in cross-shelf transport and upwelling/uplift of nutrient-enriched SACWs that drive the formation of patches of oceanic biological productivity over mostly oligotrophic shelf break and slope regions (Pereira et al., 2024). Along these regions the uplift of SACWs has been described (Mesquita et al., 1983; Brandini, 1990; Campos et al., 2000) and attributed to the effect of surface wind stress (Ekman transport), pressure gradients derived from the interaction between the BC flow and the shelf break topography, and eddies and meanders of the BC (Campos et al., 2000; Palma and Matano, 2009; Combes et al., 2021a, 2023; Pereira et al., 2024). All these processes tend to describe an enhanced onshelf transport of bottom waters across the slope and shelf break benthic boundary layer in the Santos Basin region, between 25° – 21°S, overlying sectors where the abundance of catches was highest (embayments A, B, C, Figure 10). In this region, onshelf transport of bottom waters seems to be favored by an important change in coastline orientation that modifies the along-shore pressure gradient and, through geostrophy, promotes onshore bottom flow and uplift (Palma and Matano, 2009). In addition, the shelf break sinusoidal morphology produces a series of abrupt changes in orientation that may also locally enhance cross-shelf transport (Combes et al., 2021a). Cyclonic eddies originate from the strengthening of the BC flow, and propagate downstream of regions where the coastline changes orientation (~23°S), particularly within shelf break embayments (‘shelf break bights’, Combes et al., 2023). These eddies promote cross-shelf water exchange and vertical mixing, entrapping for several weeks cold, fresh, nutrient-rich shelf waters over the shelf break embayments (Campos et al., 2000; Combes et al., 2023; Amorim et al., 2024). The spatial connection between these shelf break vertical water flow processes and concentrations of predatory megafauna (Figure 10) suggests the incidence of a biophysical aggregating mechanism (see below) and support the spatial delimitation of mesoscale productivity hotspots at the Santos Basin shelf break embayments. At a sub-mesoscale, within the embayments, it is plausible that the Brazil Current flow, past the protrusion sector, detaches from the shelf break, accelerating the uplift flow over the incurvate and steeper sector of the embayment (Figures 7, 10) where demersal fishes seem to concentrate (Combes et al., 2023). This uplift flow may be decelerated over the sediment terraces (sediment deposition area) that extends downstream within the embayments, a process described by models that compare deep-water upwelling in narrow vs. wide continental shelves (Jiang et al., 2011). Interestingly, in Figure 4G, the ‘flow direction’ terrain variable demonstrates that water flow over terraces in fact differs from the continental slope general flow.
The uplift of nutrient-rich SACW up to the photic zone (at surface or subsurface depths) can enhance phytoplankton growth leading to the formation of patches of herbivorous zooplankton that support growth of predatory fish populations (Genin, 2004). In the BMM, maxima of chlorophyll-a concentrations have been reported year-round in association with BC eddies over shelf break embayments at surface (visible in satellite imaging, Combes et al., 2023; Amorim et al., 2024) and subsurface layers (Brandini, 1990; Pereira et al., 2024). The propagation of energy down to predatory fishes dwelling on or near the slope seafloor, however, would require pelagic-benthic coupling mechanisms, such as a ‘biological active transport’ (Trueman et al., 2014). This transport is provided by diel vertical migrators including zooplankton, fishes and squids, that during daytime may reach slope depths above 500 m becoming available as prey to both benthic and benthopelagic feeders (Trueman et al., 2014). The most abundant species caught on the shelf break and slope within the embayments are benthic (monkfish) and benthopelagic feeders (argentine hake, codling and the argentine squid). All of them include pelagic (euphasid shrimps, engraulids, myctophids, rough scad Trachurus lathami) and demersal prey (Merluccius hubbsi, Cynoscion guatucupa, Synagrops bellus, Illex argentinus and others) in their diets, which indicate they have access to vertically migrating prey or migrate themselves following them (Figure 11) (Haimovici et al., 1993; Santos and Haimovici, 1997; Nascimento, 2006; Valentim et al., 2008; Muto and Soares, 2011; Costa et al., 2019). The productivity hotspots of Santos Basin embayments could, therefore, be sustained by biophysical shelf break uplift processes coupled with biological active transport of pelagic and demersal fish vertical migrators, some of them targeted by bottom fisheries (Perez et al., 2009a).
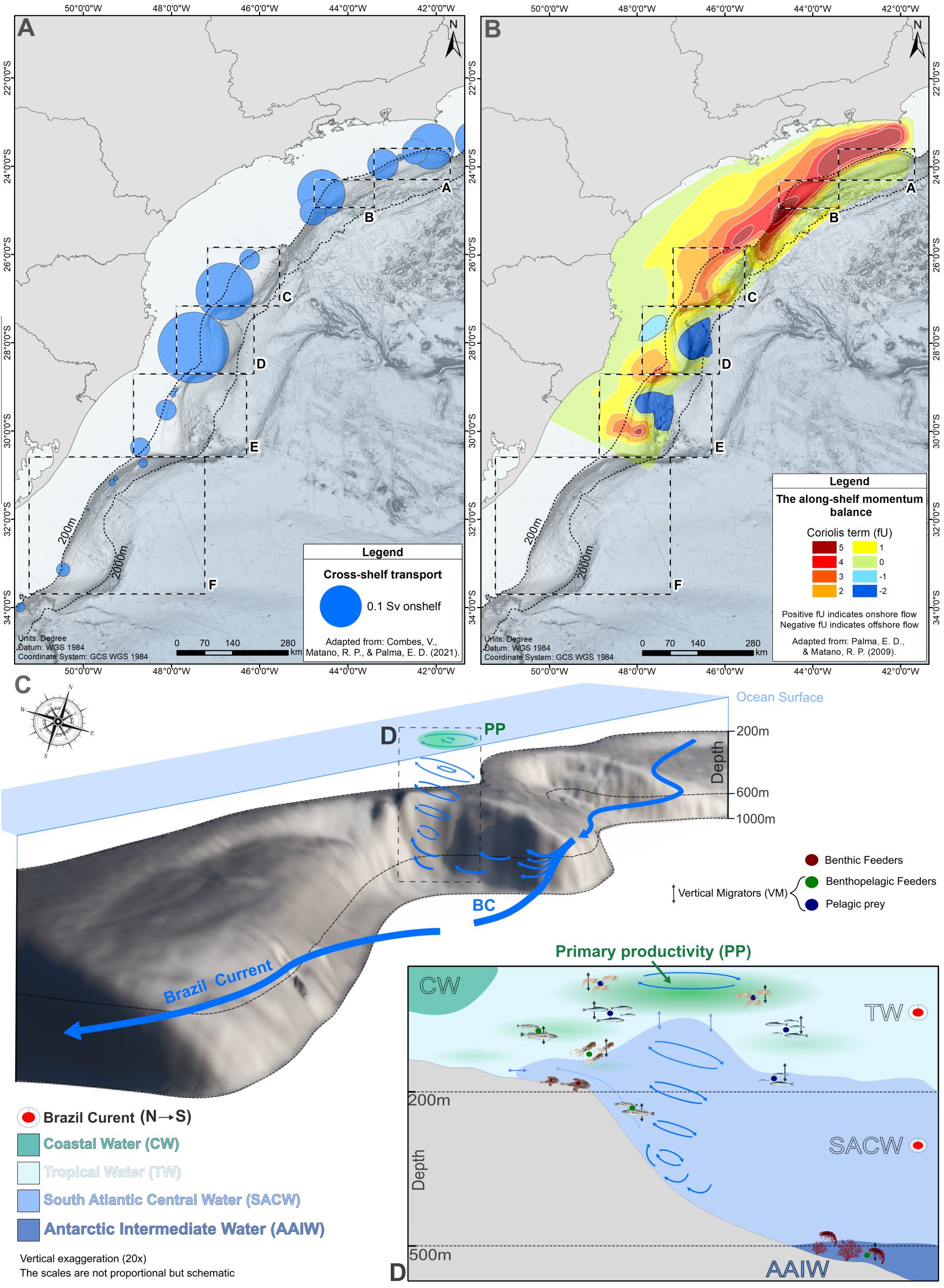
Figure 11. A conceptual view of shelf break/slope productivity hotspots in the Brazilian Meridional Margin. The geographic position of shelf break embayments (A-F) are shown in relation with the spatial distribution of onshelf transport areas, as represented by (A) onshelf flow velocities (blue circles, from Combes et al., 2021a) and (B) the Coriolis term (fU) of the along-shelf momentum balance (1-5, from Palma and Matano, 2009). In (C) a schematic representation of South Atlantic Central Water (SACW) uplift process in shelf break embayments, as produced by cyclonic eddies resulting from the interaction between slope topography and the Brazil Current (BC) flow. In the insert (D), the uplift of nutrient-rich SACWs reach the photic zone enhancing primary productivity (PP) benefiting pelagic prey that vertically migrate, becoming accessible to benthic and benthopelagic feeders living on the seafloor in 200 – 500 m depths (biological active transport).
The scarlet shrimp (Aristaeopsis edwardsiana) is the dominant deep-sea shrimp species in Santos Basin shelf break embayments (Dallagnolo et al., 2009). The species is an active swimmer that preys on both infauna invertebrates and pelagic fishes and cephalopods (Rezende et al., 2014). It forms year-round reproductive aggregations concentrated in a narrow depth zone (600 – 700 m depth), under the influence of the AAIWs that extends underneath the SACWs (> 500 m depth, Dallagnolo et al., 2009; Pezzuto and Dias, 2009; Sant’Ana, 2023). This zone is also characterized by concentrations of cold-water corals at Campos and Santos Basins, that thrive on zooplankton and particulate organic matter on 500 – 800 m depths (Kitahara et al., 2020; Bernardino et al., 2023). In association with cold-water corals, there is a diverse fish, crustacean and cephalopod fauna, all susceptible to deep-sea shrimp trawling (Perez et al., 2013). It is uncertain whether these deep fauna aggregations benefit from the biophysical shelf break uplift processes, or may be under the influence of other topography-related trophic subsidy mechanisms that may affect the transition zone between SACW and AAIW. Below intermediate slope depths (>500 m), trophic subsidy is often related to enhanced horizontal flux due to currents impinging on sloping topographies, tides and internal waves that can accelerate the near bottom flow of food particles and zooplankton, and laterally advect vertical migrating micronekton. Cold-water corals grow faster under the enhanced prey flow regime (Maier et al., 2023), aggregating demersal fauna including deep-sea shrimp prey. Moreover, such prey flow can directly benefit demersal predators that hunt pelagic prey advected by currents in-between periods of rest in less dynamic regions near the seafloor (the ‘feed-rest’ strategy Genin, 2004). The sepiolid Heteroteuthis dispar, and the squid Pyroteuthis margargaritifera are water column vertical migrators that advect during the day over abrupt topographies, such as seamounts (Clarke, 2007). These pelagic cephalopods have been reported in the diet of the scarlet shrimp, suggesting they can prey on pelagic micronekton advected by currents onto the slope benthic boundary layer, while also feeding on small invertebrates that live in the sediment (Rezende et al., 2014).
Squid and deep-sea crabs showed a less clear association with the productivity hotspots. The argentine squid (Illex argentinus) has a complex population structure on the BMM, formed by a resident spawning group as well as large north Patagonian spawning migrators that reach the region during the austral winter-spring months (Perez et al., 2009b). The latter sustained elevated catches between 28° - 30°S and 400 – 700 m depths, as well as the abundance peaks observed in the upper slope of the shelf break embayments D and E (Figure 8D). Winter-spring reproductive migrators of the southern population travel within the cold SACWs (Haimovici et al., 2008) and are possibly transient components of southern shelf break embayments productive areas. A similar link between life history patterns and the Gulf Stream shelf break exchange process was demonstrated for the co-generic species I. illecebrosus in the NW Atlantic (Salois et al., 2023). Deep-sea crab catches in embayments A-D were exclusively sustained by the royal crab (Chaceon ramosae), whereas in embayment F only the red crab (Chaceon notialis) was caught (Perez et al., 2009a). Both species exhibit complex reproductive bathymetric and latitudinal migrations, when mature and immature males and females tend to segregate in space and time (Pezzuto and Sant’Ana, 2009). Modelling the effect of geoforms on these population strata distribution could inform better if and how deep-sea crab species relate do shelf break embayments productive areas.
The analysis of historical demersal fishing operations delimited potential mesoscale and sub-mesoscale productivity hotspots in the BMM. In these hotspots, topography-induced biophysical processes may be the source of biological productivity sustaining patches of predatory demersal fishes, crustaceans and squid of commercial interest. Integrated geological, physical and biological field studies are required, however, to confirm and properly describe general mechanisms. Recent efforts have been conducted to explore habitats in the slope areas of Santos Basin, mostly driven by the need to characterize cold seeps and cold-water coral zones in association with pockmarks and other geological features (e.g. Bendia and Carrerette, 2022; Perez et al., 2023). The present study contributes to these efforts adding spatial patterns of predatory megafauna that can be integrated with the understanding of habitat structure and biophysical processes of the BMM. More importantly, these patterns strengthen the concept of productivity hotspot in the conservation debate of marine areas off the Brazilian margin (Briscoe et al., 2016).
The occurrence of vulnerable marine ecosystems (e.g., cold-water coral banks) along the slope regions of Santos, Campos and Espírito Santo basins have long justified calls for conservation action, including de implementation of marine protected area networks that could protect these ecosystems from the expanding oil and gas exploration activities (Bernardino and Sumida, 2017; Bernardino et al., 2023). The region has also been prioritized for conservation in models that combined biodiversity representation, population connectivity and threatened species, with human cumulative impacts, including fishing (Magris et al., 2020; Costa, 2024). This study demonstrates that exploiting deep-sea fishing resources and protecting biodiversity in the region may be related to the existence of mesoscale and sub-mesoscale biophysical processes that fuel productive deep ecosystems in the generally oligotrophic western boundary limb of the South Atlantic subtropical gyre (Pereira et al., 2024). We advocate that these unique productivity hotspots couple pelagic and benthic processes and should be taken into consideration in systematic conservation planning actions (Levin et al., 2017). Strategies to elaborate these actions, however, often penalize areas where there could be economic losses, excluding them from priorities areas for conservation (Combes et al., 2021b). Because biological productivity and biodiversity uniqueness are often indissociable in the deep-sea (e.g., Rosa et al., 2008), area-based initiatives towards conservation of oceanic regions and the high seas may need to conciliate both properties (Briscoe et al., 2016; Karim and Cheung, 2024).
Data availability statement
The raw data supporting the conclusions of this article will be made available by the authors, without undue reservation.
Ethics statement
Ethical approval was not required for the studies on animals in accordance with the local legislation and institutional requirements because the data was collected from industrial fishing practices.
Author contributions
JP: Conceptualization, Funding acquisition, Investigation, Project administration, Writing – original draft, Writing – review & editing. LG: Formal Analysis, Investigation, Methodology, Visualization, Writing – original draft, Writing – review & editing. RS: Formal Analysis, Investigation, Methodology, Writing – original draft, Writing – review & editing.
Funding
The author(s) declare financial support was received for the research, authorship, and/or publication of this article. The funding for this study was provided by the EU H2020-BG-2018-2020 project iAtlantic ‘Integrated Assessment of Atlantic Marine Ecosystems in Space and Time’ (Grant Agreement 818123). JP is supported by the National Council for Scientific and Technologic Development-CNPq, through the National Institute of Science and Technology -INCT Mar-COI (Process 400551/2014-4).
Acknowledgments
Authors acknowledge all observers on board the international deep-sea fishing fleet, whose hard work during many days at sea provided the invaluable data analysed in this study. Murray Roberts and the EU consortium iAtlantic ‘Integrated Assessment of Atlantic Marine Ecosystems in Space and Time’ provided critical support to this study and all the project’s scientific activities in Brazil.
Conflict of interest
The authors declare that the research was conducted in the absence of any commercial or financial relationships that could be construed as a potential conflict of interest.
The author(s) declared that they were an editorial board member of Frontiers, at the time of submission. This had no impact on the peer review process and the final decision.
Publisher’s note
All claims expressed in this article are solely those of the authors and do not necessarily represent those of their affiliated organizations, or those of the publisher, the editors and the reviewers. Any product that may be evaluated in this article, or claim that may be made by its manufacturer, is not guaranteed or endorsed by the publisher.
Supplementary material
The Supplementary Material for this article can be found online at: https://www.frontiersin.org/articles/10.3389/fmars.2024.1477960/full#supplementary-material
References
Alberoni A. A. L., Jeck I. K., Silva C. G., Torres L. C. (2019). The new Digital Terrain Model (DTM) of the Brazilian Continental Margin: detailed morphology and revised undersea feature names. Geo-Marine Lett. 40, 949–964. doi: 10.1007/s00367-019-00606-x
Alves T. M. (2010). A 3-D morphometric analysis of erosional features in a contourite drift from offshore SE Brazil. Geophys. J. Int. 183, 1151–1164. doi: 10.1111/j.1365-246X.2010.04827.x
Amorim J. P., Silveira I. C. A., Borges-Silva M., Souza-Neto P. W. M., Bernardo P. S., Dottori M., et al. (2024). The Brazil current cyclonic meandering and shelf-slope water exchanges at 27°S–31°S. Deep–Sea Res. I 206, 104276. doi: 10.1016/j.dsr.2024.104276
Anderson T., Nichol S. L., Syms C., Przeslawski R., Harris P. (2011). Deep-sea bio-physical variables as surrogates for biological assemblages, an example from the Lord Howe Rise. Deep-Sea Res. II 58, 979–991. doi: 10.1016/j.dsr2.2010.10.053
Artana C., Provost C., Lellouche J.-M., Rio M.-H., Ferrari R., Sennéchael N. (2019). The Malvinas Current at the Confluence with the Brazil Current: Inferences from 25 years of Mercator. Ocean reanalysis. J. Geophysical Research: Oceans 124, 7178–71200. doi: 10.1029/2019JC015289
Barbier E. B., Moreno-Mateos D., Rogers A. D., Aronson J., Pendleton L., Danovaro R., et al. (2014). Ecology: Protect the deep sea. Nature 505, 475–477. doi: 10.1038/505475a
Bendia A. G., Carrerette O. (2022). A multidisciplinar approach for studying deep-sea habitats in Santos Basin. Ocean Coast. Res. 70 (suppl 2), 1–5. doi: 10.1590/2675-2824070.22161agb
Bernardino A. F., Gaurisas D. Y., Sumida P. Y. G. (2023). “Biology, ecology, and threats to cold-water corals on Brazil’s deep-sea margin,” in Cold-Water Coral Reefs of the World, Coral Reefs of the World, vol. 19 . Eds. Cordes E., Mienis F. (Switzerland: Springer Cham) 19, 31–50. doi: 10.1007/978-3-031-40897-7_2
Bernardino A. F., Sumida P. Y. G. (2017). Deep risks from offshore development. Science 358, 6361. doi: 10.1126/science.aaq0779
Blondel P. (2001). “Seabed classification at ocean margins,” in Ocean Margin Systems. Ed. Wefer G., Billet D., Hebbeln D., Jorgensen B.B., Schluter M., Weering T. C. E., et al (Berlin: Springer Verlag), 1–20.
Boschen R. E., Rowden A. A., Clark M. R., Pallentin A., Gardner J. P. A. (2016). Seafloor massive sulfide deposits support unique megafaunal assemblages: Implications for seabed mining and conservation. Mar. Environ. Res. 115, 78–88. doi: 10.1016/j.marenvres.2016.02.005
Brandini F. P. (1990). Hydrography and characteristics of the phytoplankton in shelf and oceanic waters off southeastern Brazil during winter (July/August 1982) and summer (February/March 1984). Hydrobiologia 196, 111–148. doi: 10.1007/BF00006105
Brandini F. P., Boltovskoy D., Piola A., Kocmur S., Rottgers R., Abreu P. C., et al. (2000). Multiannual trends in fronts and distribution of nutrients and chlorophyll in the southwestern Atlantic (30-62°S). Deep-Sea Res. I 47, 1015–1033. doi: 10.1016/S0967-0637(99)00075-8
Briscoe D. K., Maxwell S. M., Kudela R., Crowder L. B., Croll D. (2016). Are we missing important areas in pelagic marine conservation? Redefining conservation hotspots in the ocean. Endangered Species Res. 29, 229–237. doi: 10.3354/esr00710
Brown C. J., Smith S. J., Lawton P., Anderson J. T. (2011). Benthic habitat mapping: A review of progress towards improved understanding of the spatial ecology of the seafloor using acoustic techniques. Estuarine Coast. Shelf Sci. 92, 502–520. doi: 10.1016/j.ecss.2011.02.007
Camerlenghi A. (2018). “Drivers of seafloor geomorphic change,” in Submarine Geomorphology. Eds. Micallef A., Krastel S., Savini A. (Swittzerland: Springer Geology), 135–160.
Campos E. J. D., Velhote D., Silveira I. C. A. (2000). Shelf break upwelling driven by Brazil Current Cyclonic meanders. Geophysical Res. Lett. 27, 751–754. doi: 10.1029/1999GL010502
Carreiro-Silva M., Martins I., Riou V., Raimundo J., Caetano M., Bettencourt R., et al. (2022). Mechanical and toxicological effects of deep-sea mining sediment plumes on a habitat-forming cold water octocoral. Front. Mar. Sci. 9. doi: 10.3389/fmars.2022.915650
Cheung W. L., Palacios-Abrantes J., Frolicher T. L., Palomares M. L., Clarke M. L., Lam V. W. Y., et al. (2022). Rebuilding fish biomass for the world's marine ecoregions under climate change. Global Change Biol. 28, 6254–6267. doi: 10.1111/gcb.16368
Clark M. R. (2009). Deep-sea seamount fisheries: a review of global status and future prospects. Lat. Am. J. Aquat. Res. 37, 501–505. doi: 10.3856/vol37-issue3-fulltext-17
Clark M. R., Bowden D. A., Rowden A. A., Stewart R. (2019). Little evidence of benthic community resilience to bottom trawling on seamounts after 15 years. Front. Mar. Sci. 6. doi: 10.3389/fmars.2019.00063
Clarke M. (2007). “Seamounts and cephalopods. Chapter 11,” in Seamounts: Ecology, Conservation and Management. Eds. Pitcher T. J., Morato T., Hart P. J. B., Clark M. R., Haggan N., Santos R. S. (Fish and Aquatic Resources Series, Blackwell, Oxford, UK), 207–229.
Combes V., Matano R. P., Palma E. D. (2021b). Circulation and cross-shelf exchanges in the northern shelf region of the Southwestern Atlantic: kinematics. J. Geophysical Research: Oceans 126, e2020JC016959. doi: 10.1029/2020JC016959
Combes V., Matano R. P., Palma E. D. (2023). Circulation and cross-shelf exchanges in the northern shelf of the Southwestern Atlantic: dynamics. J. Geophysical Research: Oceans 128, e2023JC019887. doi: 10.1029/2023JC019887
Combes M., Vaz S., Grehan A., Morato T., Arnaud-Haond S., Dominguez-Carrió C., et al. (2021a). Systematic conservation planning at an ocean basin scale: identifying a viable network of deep-sea protected areas in the north Atlantic and the Mediterranean. Front. Mar. Sci. 8. doi: 10.3389/fmars.2021.611358
Company J. B., Ramirez-Llodra E., Sardà F., Puig P., Canals M., Calafat A., et al. (2012). Submarine canyons in the Catalan Sea (NW Mediterranean): megafaunal biodiversity patterns and anthropogenic threats Mediterranean Submarine Canyons: Ecology and Governance. Ed. Gland W. M. (Gland and Málaga: IUCN), 133–144.
Costa J. A. (2024). Pressões e impactos ecossistêmicos da pesca demersal no sudeste e sul do Brasil (Itajaí, Santa Catarina, Brazil: Universidade do Vale do Itajaí).
Costa P. A. S., Braga A. C., Malavolti G. S., Franco M. A. L., Gatts A. B., Rezende C. E. (2019). Feeding habits and trophic status of Merluccius hubbsi along the northernmost limit of its distribution in the South-western Atlantic. J. Mar. Biol. Assoc. United Kingdom 99, 1399–1408. doi: 10.1017/S0025315419000237
Costa J. A., Schwarz R., Perez J. A. A. (2024). Cumulative ecosystem pressures exerted by demersal fisheries in the Brazilian Meridional Margin: Hotspots and refuges. Ocean Coast. Manage. 247, 106935. doi: 10.1016/j.ocecoaman.2023.106935
Costello M. J., Vale M. M., Kiessling W., Maharaj S., Price J., Talukdar G. H. (2022). “Cross-chapter paper 1: biodiversity hotspots, in climate change 2022: impacts, adaptation and vulnerability,” in Contribution of Working Group II to the Sixth Assessment Report of the Intergovernmental Panel on Climate Change. Eds. Pörtner H.-O., Roberts D. C., Tignor M., Poloczanska E. S., Mintenbeck K., Alegría A., Craig M., Langsdorf S., Löschke S., Möller V., Okem A., Rama B. (Cambridge University Press, Cambridge, UK and New York, NY, USA), 2123–2161. doi: 10.1017/9781009325844.018
Cragg J. G. (1971). Some statistical models for limited dependent variables with applications to the demand for durable goods. Econometrica 39, 829–844. doi: 10.2307/1909582
Dallagnolo R., Perez J. A. A., Pezzuto P. R., Wahrlich R. (2009). The deep-sea shrimp fishery off Brazil (Decapoda: Aristeidae): development and present status. Latin Am. J. Aquat. Res. 37, 327–346. doi: 10.3856/vol37-issue3-fulltext-5
Danovaro R., Corinaldesi C., Dell’Anno A., Snelgrove V. R. (2017). The deep-sea under global change. Curr. Biol. 27, R431–R510. doi: 10.1016/j.cub.2017.02.046
DeLeo F., Bernardino A. F., Sumida P. Y. G. (2020). “Continental Slope and Submarine Canyons: benthic diversity and human impacts,” in Brazilian Marine Biodiversity. Eds. Sumida P. Y. G., Bernardino A. F., DeLeo F. C. (Cham: Springer Nature), 37–72.
de Mahiques M. M., Fukumoto M. M., Silveira I. C. A., Figueira R. C. L., Bícego M. C., Lourenço R. A., et al. (2007). Sedimentary changes on the Southeastern Brazilian upper slope during the last 35,000 years. Anais da Academia Bras. Ciências 79, 171–181. doi: 10.1590/S0001-37652007000100018
Duarte C. S. L., Viana A. R. (2016). “Santos Drift System: stratigraphic organization and implications for late Cenozoic palaeocirculation in the Santos Basin, SW Atlantic Ocean,” in Economic and Palaeoceanographic Significance of Contourite Deposits. Viana A. R., Rebesco M. Eds. (Geological Society, London, Special Publications) vol. 276, 171–198.
Dunn D., Van Dover C. L., Etter R. J., Smith C. R., Levin L. A., Morato T., et al. (2018). A strategy for the conservation of biodiversity on mid-ocean ridges from deep-sea mining. Sci. Adv. 4, eaar4313. doi: 10.1126/sciadv.aar4313
Erdey-Heydorn M. D. (2008). An ArcGIS seabed characterization toolbox developed for investigating benthic habitats. Mar. Geod. 31, 318–358. doi: 10.1080/01490410802466819
Fernandez-Arkaya U., Ramirez-Llodra E., Aguzzi J., Allcock L. A., Davies J. S., Dissanayke A., et al. (2017). Ecological role of submarine canyons and need for canyon conservation: A review. Front. Mar. Sci. 4. doi: 10.3389/fmars.2017.0000
Franco B. C., Defeo O., Piola A. R., Barreiro M., Yang H., Ortega L., et al. (2020). Climate change impacts on the atmospheric circulation, ocean, and fisheries in the southwest South Atlantic Ocean: a review. Climatic Change 162, 2359–2377. doi: 10.1007/s10584-020-02783-6
Genin A. (2004). Bio-physical coupling in the formation of zooplankton and fish aggregations over abrupt topographies. J. Mar. Syst. 50, 3–20. doi: 10.1016/j.jmarsys.2003.10.008
Gerhardinger L. C., Quesada-Silva M., Gonçalves L. R., Turra A. (2019). Unveiling the genesis of a marine spatial planning arena in Brazil. Ocean Coast. Manage. 179, 104825. doi: 10.1016/j.ocecoaman.2019.104825
Gooday A. J., Turley C. M. (1990). Responses by benthic organisms to inputs of organic material to the ocean floor: a review. Philos. Trans. R. Soc. London. Ser. A Math. Phys. Sci. 331, 119–138. doi: 10.1098/rsta.1990.0060
Haimovici M., Martins A. S., Teixeira E. R. L. (1993). Distribución, alimentación y observaciones sobre la reproducción de la merluza (Merluccius hubbsi) en el sur de Brasil. Frente Marítimo 14, 33–40.
Haimovici M., Rossi-Wongstchowski C. L. D. B., Bernardes R. A., Fisher L. G., Vooren C. M., dos Santos R. A., et al. (2008). Prospecção pesqueira de espécies com rede de arrasto-de fundo na região sudeste-sul do Brasil (São Paulo: Série Documentos REVIZEE, Score Sul. Instituto Oceanográfico, Universidade de São Paulo).
Halpern B. S., Frazier M., Potapenko J., Casey K. S., Koening K., Longo C., et al. (2015). Spatial and temporal changes in cumulative human impacts on the world’s ocean. Nat. Commun. 6, 7615. doi: 10.1038/ncomms8615
Harris P. T., Macmillan-Lawler M., Rupp J., Baker E. K. (2014). Geomorphology of the oceans. Mar. Geology 352, 4–24. doi: 10.1016/j.margeo.2014.01.011
Healey M. C., Thomson R. E., Morris J. F. T. (1990). Distribution of commercial troll fishing vessels off Southwest Vancouver Island in relation to fishing success and oceanic water properties and circulation. Can. J. Fish. Aquat. Sci. 47, 1846–1864. doi: 10.1139/f90-210
Hothorn T., Bretz F., Westfall P. (2008). Simultaneous inference in general parametric models. Biometrical J. 50, 346–363. doi: 10.1002/bimj.200810425
Jiang L., Yan X.-H., Tseng Y.-H., Breaker L. C. (2011). A numerical study on the role of wind forcing, bottom topography, and nonhydrostacy in coastal upwelling. Estuarine Coast. Shelf Sci. 95, 99e109. doi: 10.1016/j.ecss.2011.08.019
Karim M. S., Cheung W. W. L. (2024). The new UN high seas marine biodiversity Agreement may also facilitate climate action: a cautiously optimistic view. NPJ Clim. Action 3, 8. doi: 10.1038/s44168-023-00088-9
Kenchington E. L., Cogswell A. T., MacIsaac K. G., Beazley L., Law B. A., Kenchington T. J. (2014). Limited depth zonation among bathyal epibenthic megafauna of the Gully submarine canyon, northwest Atlantic. Deep-Sea Res. II 104, 67–82. doi: 10.1016/j.dsr2.2013.08.016
Kitahara M. V., Cordeiro R. T. S., Barbosa R. V., Pires D. O., Sumida P. Y. G. (2020). “Brazilian deep-sea corals,” in Brazilian Marine Biodiversity. Eds. Sumida P. Y. G., Bernardino A. F., DeLeo F. C. (Cham: Springer Nature), 73–108.
Koslow J. A., Boehlert G. W., Gordon J. D. M., Haedrich R. L., Lorance P., Parin N. (2000). Continental slope and deep-sea fisheries: implications for a fragile ecosystem. ICES J. Mar. Sci. 57, 548–557. doi: 10.1006/jmsc.2000.0722
Levin L. A., Dayton P. K. (2009). Ecological theory and continental margins: where shallow meets deep. Trends Ecol. Evol. 24, 606–617. doi: 10.1016/j.tree.2009.04.012
Levin N., Kark S., Danovaro R. (2017). Adding the third dimension to marine conservation. Conserv. Lett. 11, 1–14. doi: 10.1111/conl.12408
Levin L. A., LeBris N. L. (2015). The deep ocean under climate change. Science 350 (6262), 766–768. doi: 10.1126/science.aad0126
Lo N. C., Jacobson L. D., Squire J. L. (1992). Indices of relative abundance for fish spotter data based on delta-lognormal models. Can. J. Fish. Aquat. Sci. 49, 2515–2526. doi: 10.1139/f92-278
Lobo F. J., dos Passos Nascimento J. J., Durán R., Lopez-Quiróz A., Guillén J., Pereira F., et al. (2024). Geomorphological features along the shelf of the southern Brazilian margin: Implications for shallow-water sediment transport induced by ocean currents. Geomorphology 456, 109215. doi: 10.1016/j.geomorph.2024.109215
Magris R. A., Costa M. D. P., Ferreira C. E. L., Vilar C. C., Joyeux J. C., Creed J. C., et al. (2020). A blueprint for securing Brazil’s marine biodiversity and supporting the achievement of global conservation goals. Divers. Distrib. 27, 198–215. doi: 10.1111/ddi.13183
Maier S. R., Brooke S., De Clippele L. H., de Foe E., van der Kaaden A.-S., Kutti T., et al. (2023). On the paradox of thriving cold-water coral reefs in the food-limited deep sea. Biol. Rev. 98, 1768–1795. doi: 10.1111/brv.12976
Mann K. H., Lazier J. R. N. (2005). Dynamics of Marine Ecosystems: Biological-Physical Interactions in the Oceans (Boston: Blackwell Publishing Ltd). doi: 10.1002/9781118687901
Martins L. R., Coutinho P. N. (1981). The Brazilian continental margin. Earth-Sciences Rev. 17, 87107. doi: 10.1016/0012-8252(81)90007-6
Maunder M. N., Punt A. E. (2004). Standardizing catch and effort data. A Rev. Recent approaches. Fish. Res. 70, 141–159. doi: 10.1016/j.fishres.2004.08.002
Mesquita A. R., Leite J. B. A., Rizzo R. (1983). Note on a shelf break upwelling off the Southeast coast of Brazil (LAT. 26°30’ S). Bolm. Inst. oceanogr. 32, 193–198. doi: 10.1590/S0373-55241983000200010
Misiuk B., Brown C. J. (2024). Benthic habitat mapping: A review of three decades of mapping biological patterns on the seafloor. Estuarine Coast. Shelf Sci. 296, 108599. doi: 10.1016/j.ecss.2023.108599
Mohriak W. U. (2003). “Sedimentary basins of the Brazilian continental margin,” in Geologia, Tectônica e Recursos Minerais do Brasil. Eds. Bizzi L. A., Schobbenhaus C., Vidotti R. M., Gonçalves J. H.(Brasília, Brazil: Companhia de Pesquisa de Recursos Minerais), 87–94.
Muto E. Y., Soares L. S. H. (2011). Spatio-temporal variations in the diet and stable isotope composition of the Argentine hake Merluccius hubbsi Marini 1933 of the continental shelf of southeastern Brazil. Mar. Biol. 158, 1619–1630. doi: 10.1007/s00227-011-1674-y
Nascimento M. C. (2006). Alimentação de peixes na plataforma continental externa e talude superior na região Sudeste – Sul do Brasil (São Paulo, Brazil: Universidade Estadual Paulista “Julio de Mesquita Filho”).
Palma E. D., Matano R. (2009). Disentangling the upwelling mechanisms of the South Brazil Bight. Continental Shelf Res. 29, 1525–1534. doi: 10.1016/j.csr.2009.04.002
Pereira F., da Silveira I. C. A., Tandon A., Franks P. J. S., Luko C. D., Santos D. M. C., et al. (2024). Phytoplankton responses to mesoscale and submesoscale processes in a tropical meander. J. Geophysical Research: Oceans 129, e2023JC020685. doi: 10.1029/2023JC020685
Perez J. A. A., Abreu J. G. N., Lima A. O. S., Silva M. A. C., Souza L. H. P., Bernardino A. F. (2020). “Living and non-living resources in Brazilian deep waters,” in Brazilian Marine Biodiversity. Eds. Sumida P. Y. G., Bernardino A. F., DeLeo F. C. (Cham: Springer Nature), 217–254. doi: 10.3856/vol37-issue3-fulltext-18
Perez J. A. A., Barros Neto H. M. C., Arantes R. C. M., Gaurisas D. Y., Silva C. F., Alves F. M. M., et al. (2023). Deep sea ecosystem exploration and ‘health check’: sampling strategy and methods applied during the iAtlantic_BR10_Petrobras cruise in the Santos Basin, Southwest Atlantic. Ocean Coast. Res. 71, e23046. doi: 10.1590/2675-2824071.23069jaap
Perez J. A. A., Pereira B. N., Pereira D. A., Schroeder R. (2013). Composition and diversity patterns of megafauna discards in the deep-water shrimp trawl fishery off Brazil. J. Fish Biol. 83, 804–825. doi: 10.1111/jfb.12141
Perez J. A. A., Pezzuto P. R., Wahrlich R., Soares A. L. S. (2009a). Deep-water fisheries in Brazil: history, status and perspectives. Lat. Am. J. Aquat. Res. 37, 513–541.
Perez J. A. A., Silva T. N., Schroeder R., Schwarz R., Martins R. S. (2009b). Biological patterns of the Argentine shortfin squid Illex argentinus in the slope trawl fishery off Brazil. Lat. Am. J. Aquat. Res. 37, 409–428. doi: 10.3856/vol37-issue3-fulltex-10
PEW (2024). A Path to Creating the First Generation of High Seas Protected Areas. Science-based method highlights 10 sites that would help safeguard biodiversity beyond national waters. Pew Charitable Trusts Rep., 38 p. Available online at: https://www.pewtrusts.org/-/media/assets/2024/04/highseas_mpa_report_2024.pdf
Pezzuto P. R., Dias M. C. (2009). Reproductive cycle and population structure of the deepwater shrimp Aristeus antillensis A. Milne Edwards & Bouvier 1909 (Decapoda: Aristeidae) on southeast Brazilian continental slope. Lat. Am. J. Aquat. Res. 37, 443–454. doi: 10.3856/vol37-issue3-fulltext-13
Pezzuto P. R., Sant’Ana R. (2009). Sexual maturity of the deep-sea royal crab Chaceon ramosae Manning, Tavares & Albuquerque 1989 (Brachyura: Geryonidae) in southern Brazil. Lat. Am. J. Aquat. Res. 37, 297–311. doi: 10.3856/vol37-issue3-fulltext-3
Ramirez-Llodra E., Brandt A., Danovaro R., De Mol B., Escobar E., German C. R., et al. (2010). Deep, diverse and definitely different: unique attributes of the world’s largest ecosystem. Biogeosciences 7, 2851–2899. doi: 10.5194/bg-7-2851-2010
Rezende G. A., Pezzuto P. R., Dumont L. F., D’Incao F. (2014). Feeding habits of the deep-sea shrimp Aristaeopsis edwardsiana (Johnson 1867) (Decapoda: Aristeidae) on the Southeastern and Southern Brazilian coast. Pan-American J. Aquat. Sci. 9, 167–178.
Rosa R., Dierssen H. M., Gonzalez L., Seibel B. A. (2008). Large-scale diversity patterns of cephalopods in the Atlantic open ocean and deep sea. Ecology 89, 3449–3461. doi: 10.1890/08-0638.1
Salois S. L., Hyde K. J. W., Silver A., Lowman B. A., Gangopadhyay A., Gawarkiewicz G., et al. (2023). Shelf break exchange processes influence the availability of the northern shortfin squid, Illex illecebrosus, in the Northwest Atlantic. Fisheries Oceanography 32, 461–478. doi: 10.1111/fog.12640
Sant’Ana R. (2023). Mudanças Climáticas e Seus Efeitos Sobre os Habitats e a Distribuição Espacial da Megafauna Oceânica e Profunda no Atlântico Sudoeste (Itajaí, Santa Catarina, Brazil: Universidade do Vale do Itajaí).
Sant’Ana R., Perez J. A. A. (2016). Surveying while fishing in the slope areas off Brazil: direct assessment of fish stock abundance from data recorded during commercial trawl fishing operations. Lat. Am. J. Aquat. Res. 44, 1039–1054. doi: 10.3856/vol44-issue5-fulltext-15
Santos R. A., Haimovici M. (1997). Food and feeding of the short-finned squid Illex argentinus (Cephalopoda: Ommastrephidae) off southern Brazil. Fisheries Res. 33, 139–147. doi: 10.1016/S0165-7836(97)00071-4
Scales K. L., Miller P. I., Hawkes L. A., Ingram S. N., Sims D. W., Votier S. C. (2014). On the Front Line: frontal zones as priority at-sea conservation areas for mobile marine vertebrates. J. Appl. Ecol. 51, 1575–1583. doi: 10.1111/1365-2664.12330
Shimabukuro M., Alfaro-Lucas J. M., Bernardino A. F., Ramos R. B., Mahiques M. M., Sumida P. Y. G. (2020). “Chemosynthetic ecosystems on the Brazilian deep-sea margin,” in Brazilian Marine Biodiversity. Eds. Sumida P. Y. G., Bernardino A. F., DeLeo F. C. (Cham: Springer Nature), 109–132.
Silveira I. C. A., Napolitano D. C., Farias I. U. (2020). “Water masses and oceanic circulation of the Brazilian Continental Margin and adjacent abyssal plain,” in Brazilian Marine Biodiversity. Eds. Sumida P. Y. G., Bernardino A. F., DeLeo F. C. (Cham: Springer Nature), 7–36.
Sumida P. Y. G., De Leo F. C., Bernardino A. F. (2020). “Chapter 1. An introduction to the Brazilian deep-sea biodiversity,” in Brazilian Marine Biodiversity. Eds. Sumida P. Y. G., Bernardino A. F., DeLeo F. C. (Cham: Springer), 1–5.
Sweetman A. K., Thurber A. R., Smith C. R., Levin L. A., Mora C., Wei C.-L., et al. (2017). Major impacts of climate change on deep-sea benthic ecosystems. Elem. Sci. Anth. 5. doi: 10.1525/elementa.203
Thurber A. R., Sweetman A. K., Narayanaswamy B. E., Jones D. O., Ingels J., Hansman R. L. (2014). Ecosystem function and services provided by the deep sea. Biogeosciences 11, 3941–3963. doi: 10.5194/bg-11-3941-2014
Trueman C. N., Johnston G., O’Hea B., MacKenzie K. M. (2014). Trophic interactions of fish communities at midwater depths enhance long-term carbon storage and benthic production on continental slopes. Proc. R. Soc B 281, 20140669. doi: 10.1098/rspb.2014.0669
Valentim M. F. M., Caramaschi E. P., Vianna M. (2008). Feeding ecology of monkfish Lophius gastrophysus in the south-western Atlantic Ocean. J. Mar. Biol. Assoc. United Kingdom 88, 205–212. doi: 10.1017/S0025315408000301
Viana A. R. (2002). Seismic expression of shallow- to deep-water contourites along the south-eastern Brazilian margin. Mar. Geophysical Res. 22, 509–521. doi: 10.1023/A:1016307918182
Visali M. E., Best B. D., Cabral R. B., Cheung W. W. L., Clark N. A., Garilao C., et al. (2020). Data-driven approach for highlighting priority areas for protection in marine areas beyond national jurisdiction. Mar. Policy 122, 103927. doi: 10.1016/j.marpol.2020.103927
Walbridge S., Slocum N., Pobuda M., Wright D. J. (2018). Unified geomorphological analysis workflows with benthic terrain modeler. Geosciences 8, 94. doi: 10.3390/geosciences8030094
Wilson M. F. J., O’Connel B., Brown C., Guinan J. C., Grehan A. J. (2007). Multiscale terrain analysis of Multibeam bathymetry data for habitat mapping on the continental slope. Mar. Geodesy 30, 3–35. doi: 10.1080/01490410701295962
Keywords: productivity hotspots, Southwest Atlantic, deep-sea conservation, deep-sea fisheries, seabed modelling
Citation: Perez JAA, Gavazzoni L and Sant’Ana R (2024) Historical fishing regimes uncover deep-sea productivity hotspots in the SW Atlantic Ocean. Front. Mar. Sci. 11:1477960. doi: 10.3389/fmars.2024.1477960
Received: 08 August 2024; Accepted: 28 October 2024;
Published: 21 November 2024.
Edited by:
Les Watling, University of Hawaii at Manoa, United StatesReviewed by:
Fábio L. Matos, University of Aveiro, PortugalFrancis Neat, World Maritime University, Sweden
Copyright © 2024 Perez, Gavazzoni and Sant’Ana. This is an open-access article distributed under the terms of the Creative Commons Attribution License (CC BY). The use, distribution or reproduction in other forums is permitted, provided the original author(s) and the copyright owner(s) are credited and that the original publication in this journal is cited, in accordance with accepted academic practice. No use, distribution or reproduction is permitted which does not comply with these terms.
*Correspondence: Jose Angel A. Perez, YW5nZWwucGVyZXpAdW5pdmFsaS5icg==
†These authors have contributed equally to this work and share first authorship