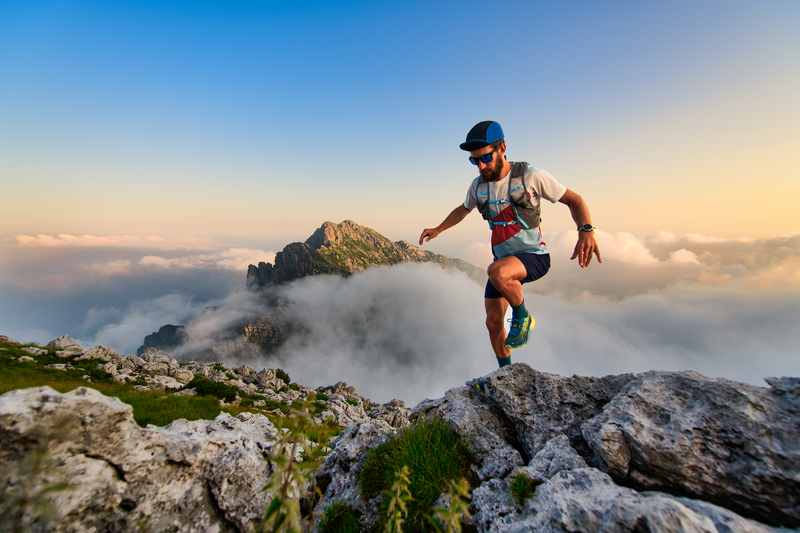
95% of researchers rate our articles as excellent or good
Learn more about the work of our research integrity team to safeguard the quality of each article we publish.
Find out more
ORIGINAL RESEARCH article
Front. Mar. Sci. , 14 January 2025
Sec. Marine Ecosystem Ecology
Volume 11 - 2024 | https://doi.org/10.3389/fmars.2024.1477142
The influence of climate change on marine organism abundance has rarely been assessed (1) at the functional-group level; (2) simultaneously in major functional groups within the same ecosystem; (3) for >10 years; and (4) at metapopulation/community scales. A study simultaneously addressing these gaps would greatly enhance our understanding of the influence of climate change on marine ecosystems. Here, we analyzed 21 years of abundance data at the functional-group and species levels on a regional scale for four major functional groups (benthic algae, sessile animals, herbivorous benthos, and carnivorous benthos) in a rocky intertidal habitat along the northeastern Pacific coast of Japan. We aimed to examine the 21-year trends in regional abundance at both functional-group and species levels, plus their driving mechanisms and their dependence on species properties (thermal niche, calcification status, and vertical niche). Significant temporal trends in abundance were detected at functional-group levels for benthic algae (increasing) and herbivores and carnivores (both decreasing); they followed the temporal population trends of the dominant species. At species level, the metapopulation size of 12 of 31 species were increasing and 4 of those were decreasing, depending on the thermal niche and species calcification status. At both functional-group and species levels, temporal trends in abundance are caused by the direct or indirect influence of warming and ocean acidification. Comparing these results with community responses to marine heat waves in the same study area offered two implications: (1) long-term ecosystem changes associated with global warming will be unpredictable from the community response to marine heat waves, possibly owing to a lack of knowledge of the influence of calcifying status on species’ responses to climate change; and (2) thermal niches contribute greatly to predictions of the influence of warming on population size, regardless of the time scale.
Global climate change is now accepted as a scientific fact, and its impacts are being discussed. In marine habitats, the increase in sea-surface temperatures associated with global warming (IPCC, 2001, 2007) and the acidification due to an increase in atmospheric CO2 concentration and the consequent increase in dissolved CO2 levels in the ocean (Feely et al., 2004; Caldeira and Wickett, 2005; Kleypas and Yates, 2009) are projected to have substantial influences on both pelagic and benthic ecosystems. In addition, marine heat waves, which are increasing in intensity and frequency because of climate change (Hobday et al., 2016), are also projected to have a substantial biological influence on marine ecosystems.
The influences of climate change, such as warming, ocean acidification, and marine heat waves, on the abundance of marine organisms are diverse. For example, the influences of warming and marine heat waves (negative, positive, or neutral) on species abundance vary by trophic position and taxon (Hobday et al., 2016; Ruthrof et al., 2018; Giddens et al., 2022; Hu et al., 2022; Ishida et al., 2023; Ziegler et al., 2023). The influences of ocean acidification on the abundance of marine organisms may also vary by trophic level, with herbivores reported to be substantially negatively influenced, whereas primary producers are partially negatively influenced (Hu et al., 2022). These interspecific variations in the response of abundance to climate change may be related to differences in species properties, or they may be caused by indirect influences (i.e. the influences of climate change on one species spilling over to another), or both. For example, differences in the responses of abundance to warming and marine heat waves have been reported to be related to differences in thermal niches among species (Harvey et al., 2013; Smale et al., 2017; Ishida et al., 2023). In addition, the responses of abundance to ocean acidification may vary depending on the calcifying status of body tissues (Harvey et al., 2013; Kunze et al., 2021). The differences among trophic levels in the responses of abundance to marine heat waves and ocean acidification may be caused by trophic cascades, namely the indirect spillover of abundance changes occurring in one trophic level to other, interacting, trophic levels (Smale and Wernberg, 2013; Wernberg et al., 2013; Smale et al., 2017; Suryan et al., 2021; Ishida et al., 2023).
Recently, many studies have evaluated the influence of climate change on the abundance of marine organisms, thus gradually elucidating the influence of warming and acidification at the species level (Wernberg et al., 2013). However, because research in this field is still heavily narrowly focused on terms of subjects and objectives, the influence of changes on marine ecosystems remains elusive (Table 1). First, studies assessing the influence of climate change on the abundance of marine organisms simultaneously in major functional groups within the same ecosystem are scarce. There is also a paucity of studies on the influence of climate change on abundance at the functional-group level. Therefore, studying in functional group-level abundance and species-level abundance of the major functional groups composing the ecosystem provide deeper insights that contributes to a better understanding of the influences of climate change on ecosystems, such as the indirect influences of climate change on some ecosystems and its spillover effects on other organisms, and provides deeper insight into the influences of climate change on ecosystem structure and function. Secondly, although long-term study ensure large number of observations (i.e., sample size), which allows for sensitive detection of smaller changes/influences of climate change, few long-term studies of more than 10 years have been conducted. Therefore, long-term studies of more than 10 years provide deeper insight into long-term changes/influences that cannot be captured by shorter-term studies. Thirdly, virtually only few studies have assessed the influences of climate change on the abundance of organisms at a broad spatial scale, including metapopulations (but see Mieszkowska et al., 2021). Therefore, a broad spatial scale studies, encompassing metapopulations provide deeper insight into the influences of climate change on the persistence of populations. Fourthly, although field-base study on both warming and acidification allows their influences can be quantitatively and directly compared, there are more studies examining the influences of warming than of acidification (Hu et al., 2022), and these studies are mostly in experimental systems (Hu et al., 2022). Therefore, field-base study focusing on both warming and acidification is providing insight into real-world impacts in the field that cannot be obtained from experimental systems alone. In this manner, addressing these issues simultaneously would substantially improve our understanding of the influences of climate change on marine ecosystems. To this end, it is important to clarify the long-term impact of climate change on the abundance of major functional groups at both the functional-group and species levels. These are major hierarchical levels of ecosystem organization that can be accurately quantified in model ecosystems. On the other hand, at the population (species) level, it would be very useful to assess the links between species properties that are thought to be relevant to responses to climate change, such as thermal niche and calcifying/non-calcifying status.
Table 1. Differences between our study and previous studies and the academic novelty (importance) of our study.
In rocky intertidal habitats, desiccation stress varies greatly over a narrow vertical range (Noda, 2009). The rocky intertidal community is composed of organisms at different functional groups; they can be classified as algae, sessile animals (most of which are suspension feeders), herbivores, and carnivores (Menge, 1976; Menge et al., 1986; Okuda et al., 2010; Iwasaki et al., 2016; Ishida et al., 2021; Weitzman et al., 2021; Scrosati et al., 2022; Ishida et al., 2023). Functional group means a grouping of species that share combinations of biological traits that affect ecosystem function in similar ways (Cummins, 1974; Blondel, 2003). In this habitat, each species occupies a specific elevational range depending on its desiccation tolerance, with the more desiccation-tolerant species generally occupying the upper intertidal zone. The rocky intertidal community is the most appropriate study system for assessing the response of marine benthic communities to climate change, for three reasons: first, the component species are at different trophic levels (i.e., they can be categorized as algae, sessile animals, herbivores, or carnivores). Secondly, the abundances of most species can be accurately quantified in a non-destructive way. Therefore, it is relatively easy to obtain continuous, highly accurate time-series data on the abundance of community component species. Thirdly, because of these species’ location at the boundary between sea and land, they are influenced by climate change via both seawater temperature and air temperature. For rocky intertidal organisms, species characteristics other than thermal niche and species calcifying status may also be relevant to differences in the species’ responses to climate change. For example, in rocky intertidal sessile organisms, the vertical niche represents the range of habitable elevations. The higher the vertical niche, the more vulnerable it may be to sea surface temperature increases associated with climate change. This is because the upper intertidal zone has a shorter submergence period than the lower intertidal zone. Also, thermal extremes and fluctuations are greater in intertidal habitats at lower to higher elevations (Benedetti-Cecchi et al., 2006; Watt and Scrosati, 2013).
Here, we aimed to clarify the temporal patterns and underlying processes of the 21-year dynamics of rocky intertidal communities on the eastern Pacific coast of Hokkaido, Japan. Over the past 21 years, the Pacific coast of eastern Hokkaido has experienced both warming and acidification. The daily mean air temperature from 2002 to 2022 at the Japan Meteorological Agency’s Chipomanai station, and the stationary sea-surface temperature data from 2003 to 2022 measured at the Japan Oceanographic Data Centre’s Akkeshi station showed significant increasing trend (see Supplementary Figure). Decreases in pH have been reported throughout the Japanese coast, including the Pacific coast of eastern Hokkaido (Japan Meteorological Agency, 2023). Specific objectives were to answer the following three questions about the abundance of four functional groups (benthic algae, sessile animals, herbivorous benthos, and carnivorous benthos at the functional-group and species levels on a regional scale:
1. For each functional group, what was the temporal trend of the community abundance (abundance of each functional group)? Furthermore, how were the community abundance dynamics of each functional group driven by temporal changes in the environmental variables, including air temperature, sea-surface water temperature, and pH of sea-surface water (ocean acidification) associated with climate change?
2. For each species, what was the temporal trend in population size? Furthermore, how were population dynamics driven by temporal changes in the environmental variables, including air temperature, sea-surface water temperature, and year, associated with climate change?
3. How did species properties, including thermal niche, species calcifying status (calcifying or non-calcifying), and vertical niche, affect the interspecific differences in the temporal trends in population size?
The study area was situated along the coast of southeast Hokkaido, Japan within the Oyashio Current in the Cold Temperate Northwest Pacific in the Temperate Northern Pacific (Figure 1) (Spalding et al., 2007; Payne et al., 2012; Ishida et al., 2021). The study area is inhabited by numerous benthic algae, including dominant species: the crustose coralline alga Corallina pilulifera, the red algae Gloiopeltis furcata and Chondrus yendoi, and the brown alga Analipus japonicus. The study area is inhabited by several sessile animals, including dominant species: the native barnacle Chthamalus dalli (Kanamori et al., 2017; Ishida et al., 2021). The study area is inhabited by several herbivorous benthos, including dominant species: the limpets Lottia radiata and Lottia cassis and the periwinkle Littorina sitkana (Ishida et al., 2023). The carnivorous benthos in the study area includes the whelk Nucella lima (Noda and Ohira, 2020).
Figure 1. Map of the study area. Five sites on shores located along the Pacific coast of Hokkaido were selected for the census of rocky intertidal communities.
During the study period (2003-2023), the area suffered from several meteorological and biological events that may have influenced the rocky intertidal communities. In the winters of 2003, 2008, and 2017, drift ice had arrived along the coastline (Japan Meteorological Agency). Furthermore, on 21 November 2006, a severe storm struck the area, reducing the abundances of sessile animals and some benthic algae because of the storm surge caused by strong winds (Iwasaki and Noda, 2018). In August 2016, an unusual occurrence of typhoons in northern Japan (Nayak and Takemi, 2020a, b). From September to November 2021, harmful algal blooms occurred in the area (Kuroda et al., 2021; Iwataki et al., 2022; Kuroda et al., 2022), causing declines in the abundances of several species of benthic algae and herbivorous benthic invertebrates (Yao and Noda, 2024). The non-native barnacle species Balanus glandula was introduced to the study area in 2004, and it is possible that its population dynamics have not yet reached equilibrium (Noda and Ohira, 2020).
Hierarchical nested sampling (Noda, 2004) was used to lay out each site. Four rocky shores were chosen for the census of intertidal organisms (Figure 1). Within each shore, five permanent census plots (50 cm wide and 100 cm high, with the vertical midpoint corresponding to mean tidal level) were established in semi-exposed locations, which are exposed to wind-generated waves from ~180°, while wind from the opposite direction generates minimal wave action. The horizontal extent of our study sites can be judged to cover the spatial extent of most Benthos metapopulations. This is because our sample range exceeds the specific distance of genetic dispersal scale for macroalgae (<4.2 km) and littorina (23 km) (Kinlan and Gaines, 2003). Within each census plot, the abundances of four functional groups (benthic algae, sessile animals, and herbivorous and carnivorous benthos) were censused at species level in summer (July or August) from 2003 to 2023. Observed organisms were categorized functional groups based on Ishida et al., 2023. Within each census plot, the abundances of benthic algae and sessile animals were measured by placing 200 grid points at 5-cm intervals both vertically and horizontally on the rock surface and identifying and recording the species occupying each grid point. The abundances of herbivorous and carnivorous benthos were recorded as the number of individuals of each species occurring within each census plot. However, we combined the numbers of individuals of the two limpet species, L. radiata and L. cassis, in the 2003 survey, because their identification has been possible in surveys since 2004. From the abundance data obtained for all census plots for each species in each year, yearly sum values were obtained for each of the four functional groups as the abundance at the functional-group level, and for each species in each functional group as the abundance at the species level, on a regional scale, means the spatial extent includes the meta-population encompassing the specific distance of the genetic dispersal scale, for each year.
Thermal niche (i.e., the preferred temperature range) and calcifying status of all species, and vertical niche of sessile species (benthic algae, sessile animals) were determined as follows.
Ishida et al. (2023) had previously measured the thermal niches of species in this study area. They used the average annual sea-surface temperatures of the northern and southern ecoregions of the habitat as thermal niche. Information on habitat ecoregion was obtained from the work of Spalding et al. (2007). We used those data for our analysis.
Ishida et al. (2023) had previously measured the vertical niches of sessile species in this study area. To measure these properties, they estimated the vertical profile of the distribution of each species over the entire vertical extent of the intertidal zone. We used these data for our analysis.
Among the environmental changes caused by climate change, the influences of increased atmospheric CO2 concentrations and ocean acidification on organisms are particularly pronounced in species with calcareous body tissues (Fitzer et al., 2014). Here, molluscs with shells (Nishimura, 1995), barnacles (Nishimura, 1995), calcareous algae (Yoshida, 1998) and Serpulidae (Nishimura, 1995), were defined as calcifying species, whereas other species were defined as non-calcifying species.
To investigate the potential drivers of community dynamics for the four functional groups under climate change, we obtained annual climatic variables for air temperature and sea-surface water temperature, as well as for the pH of sea-surface water.
For the air and sea-surface water temperature variables we chose the following six variables: the annual mean air temperature; the monthly mean coldest-month air temperature; the monthly mean hottest-month air temperature; the annual mean sea temperature; the monthly mean coldest-month sea temperature, and the monthly mean hottest-month sea temperature.
The six temperature variables in each census year were calculated from the daily mean air temperature data from 2002 to 2022 at the Japan Meteorological Agency’s Chipomanai station, which is the air-temperature station closest to the study site, and from the stationary sea-surface temperature data from 2003 to 2022 measured at the Japan Oceanographic Data Centre’s Akkeshi station, which is the sea-water temperature station closest to our study site. Annual mean air and sea-surface temperatures were obtained from August of the previous year to July of the current year. As the data for the six variables (annual mean, monthly mean in the coldest month, and monthly mean in the hottest month for both air and sea-surface temperatures in each census year) were collinear to each other, the variables were subjected to principal component analysis and the first and second principal components were then treated as measures of the temperature conditions in each year. This is because 50.5% of the annual variation in these six variables could be explained by the first principal component and 31.5% could be explained by the second. The first principal component (hereafter referred to as PC1) was positively influenced by all temperature parameters and was particularly strongly influenced by the mean air and sea temperatures (Figure 2A); it can therefore be interpreted as representing a summary of the overall temperature trend. The second principal component (hereafter referred to as PC2) was more negatively influenced by air temperature than by sea temperature. It was strongly influenced by the monthly mean coldest and hottest air and sea-surface temperatures and was particularly positively influenced by the monthly mean coldest air temperature (Figure 2B); it can be interpreted as representing the annual range of temperature. PC1 was correlated with the number of years (0 to 21) since 2003, whereas PC2 was not (Figures 2C, D).
Figure 2. Temperature variables (PC1 and PC2): their relationships with six temperature variables (annual mean, monthly mean of the coldest month, and monthly mean of the hottest month) for both air temperature and sea-surface temperature in each census year, and their temporal trends. (A) Principal component (PC) loadings of the six temperature variables for the first principal component (PC1) from the principal component analysis (PCA). (B) PC loadings of the six temperature variables for PC2 from the PCA. (C) Yearly changes and temporal trends of PC1. (D) Yearly changes and temporal trends of PC2.
The year mean pH value of sea-surface water in each census year were calculated from the monthly mean pH of sea-surface water data from 2002 to 2022 (Japan Meteorological Agency, 2023).
For each functional group, to assess the temporal trend of community abundance (i.e., the total abundance (coverage of sessile species, individual numbers of mobile species) of all species comprising a functional group), we calculated the correlation coefficients between the log-transformed abundance values and the year. We used Pearson’s correlation coefficient to identify linear temporal trends and Spearman’s rank correlation coefficient to confirm non-linear temporal trends.
To evaluate how the dynamics of the community abundance of each functional group were driven by climate-change-associated temporal changes in environmental variables, we performed multiple regression analyses. We assumed that the dynamics of community abundance followed a Gompertz-type autoregressive model (Royama, 1992; Turchin, 2003; Dennis et al., 2006). We used the annual change in community abundance as the response variable, and the log of abundance in year t, the temperature conditions (PC 1 and PC2) in year t, and pH of sea-surface water were treated as explanatory variables. To ensure the accuracy of the analyses, we eliminated the influence of extreme events on the dynamics of the community abundance of each functional group, as follows. For each functional group, we compared the absolute values of y residuals of the multiple regression analysis between the periods without and with extreme events (drift ice in the winters of 2003, 2008, and 2017; a severe storm on 21 November 2006; an unusual occurrence of typhoons in August 2016; and a harmful algal bloom from September to November 2021). Then, if the values from the period with each extreme event deviated from the 95% confidence interval of the values from the period without an extreme event, we judged that the extreme event had had a significant influence on the dynamics of the community abundance of the functional group. Finally, we reran the multi-regression analyses and excluded both the response and the explanatory variables from the periods with significant extreme events; these periods were detected as those with a severe storm (2006, influenced 2007 data) that affected the community abundance of benthic algae and herbivorous benthos; Typhoons (2016, influenced 2017 data) or drift ice (2017, influenced 2017 data) that affected sessile animals and carnivorous benthos; and harmful algal blooms (2021, influenced 2022 data) that affected herbivorous benthos and carnivorous benthos. Therefore, we excluded the years in which we judged that the extreme event had had a significant influence on the dynamics of the community abundance of the functional group from the analysis. Before the regression analyses, we checked the variance inflation factors (VIFs) of PC1 and PC2, as well as pH of sea-surface water, and we found no collinearity because PC1’s VIF was 1.26, PC2’s was 1.05, and pH’s was 1.25, all less than 1.5. Model assumptions were verified by plotting residuals versus fitted values, versus each covariate in the model. We assessed the residuals for temporal dependency.
For each species, to assess the temporal trend of the population size (i.e., the total abundance of each species), we calculated the correlation coefficients between the log-transformed abundance values and the year. We used Pearson’s correlation coefficient to identify linear temporal trends and Spearman’s rank correlation coefficient to confirm non-linear temporal trends.
To evaluate how the population dynamics of each species were driven by climate-change-associated temporal changes in environmental variables, we performed multiple regression analyses in which the annual population growth rate (difference in log abundance from year t to year t + 1) of each species was the response variable (the same as in section 2.5.1 above). To ensure the accuracy of the analyses, we eliminated the influence of extreme events on the population dynamics of each species, as follows. For each species, we compared the absolute values of y residuals of the multiple regression analysis between the periods without and with extreme events (drift ice in the winters of 2003, 2008, and 2017; a severe storm on 21 November 2006; an unusual occurrence of typhoons in August 2016; and a harmful algal bloom from September to November 2021). Then, if the values from the period with each extreme event deviated from the 95% confidence interval of the values from the period without extreme events, we judged that the extreme event had had a significant influence on the population dynamics. Finally, we excluded the years in which we judged that the extreme event had had a significant influence on the population dynamics from the analysis for each species (Supplementary Table). The abundances of limpets L. radiata and L. cassis were analyzed for the period 2004 to 2023. Species that were present for less than one-third of the study period (less than seven occurrences) were excluded from the analysis, as the accuracy of the population dynamics estimate was subjectively considered low. Model assumptions were verified by plotting residuals versus fitted values, versus each covariate in the model. We assessed the residuals for temporal dependency.
To evaluate the relationship between species’ thermal niche and the temporal trend in population size for each species, we obtained the Pearson’s correlation coefficient between species’ thermal niche and the Pearson’s and Spearman’s correlation coefficients between year and the log-transformed abundance values for all species.
To evaluate the relationship between species’ calcifying status and the temporal trend in population size, we performed a Welch test to determine whether differences in the calcifying status of all species led to a difference in the Pearson’s and Spearman’s correlation coefficients between the year and log-transformed abundance values. The reason for not categorizing the species into four functional groups was that the numbers of species in the functional groups other than algae were small. This was particularly the case for carnivorous benthos, which consisted of only two species, indicating that sufficient data could not be secured.
To evaluate the relationship between species’ vertical niche and the temporal trend in population size for each species, we obtained the Pearson’s correlation coefficient between species’ vertical niche and the Pearson’s and Spearman’s correlation coefficients between year and the log-transformed abundance values for sessile species. The two functional groups of sessile species—benthic algae and sessile animals—were not analyzed separately because of the small number of species in the sessile animals group. The VIF values were used to examine the collinearity of the various properties; Thermal niche’s was 1.04, vertical niche’s was 1.08, and calcifying status’s was 1.04, they were all less than 1.5 and therefore not collinear).
Temporal trends in community abundance varied among the functional groups (Figure 3). Significant positive correlations between community abundance and year were detected by both Pearson’s and Spearman’s coefficients for benthic algae. In contrast, significant negative correlations were detected between community abundance and year by Pearson’s and Spearman’s coefficients for herbivorous benthos (Figure 3). Between community abundance and year, Pearson’s and Spearman’s correlation coefficients for sessile animals and carnivorous benthos were not significant (Figure 3).
Figure 3. Temporal trends in the regional-scale community abundances of four functional groups (benthic algae, sessile animals, herbivorous benthos, and carnivorous benthos). Temporal trends were evaluated on the basis of Pearson’s and Spearman’s correlation coefficients between log-transformed abundance and year. * P < 0.05.
For all functional groups, the standard partial regression coefficients of the log of abundance in year t (explanatory variable) were significantly negative (Table 2). This indicated that the dynamics of community abundance were under negative feedback control in all functional groups. In contrast, the environmental variables that drove the dynamics of community abundance differed among the functional groups. For benthic algae, PC1 and PC2 had a significant positive influence on annual changes in community abundance. For herbivorous benthos, only PC1 had a significant negative influence on annual changes in community abundance. For carnivorous benthos, only pH of sea-surface water had a significant positive influence on annual changes in community abundance. For sessile animals, none of the environmental variables had a significant influence on annual changes in community abundance.
Table 2. Community dynamics of each functional group, assuming that the community dynamics followed a Gompertz-type autoregressive model.
The temporal trends in population size varied by species (Figures 4–7). Among the 18 species of benthic algae, five (about 27.8%) showed a significant increasing trend by Pearson’s correlation coefficient and two (about 11.1%) showed a significant decreasing trend, six species (about 33.3%) showed a significant increasing trend by Spearman’s correlation coefficient, and one (about 5.6%) showed a significant decreasing trend (Figure 4). Among the seven species of sessile animals, two species (about 28.6% by Pearson’s correlation coefficient) and four species (57.1% by Spearman’s correlation coefficient) showed significant increasing ltrends (Figure 5). Among the herbivorous benthos, one species (20%) showed a significant increasing trend by only Spearman’s correlation coefficients, whereas the two species (40%) showed a significant decreasing trend (Figure 6). The only one species of carnivorous benthos, N. lima showed no significant trend (Figure 7).
Figure 4. Temporal trends in the regional-scale population sizes of benthic algae. These trends were based on Pearson’s and Spearman’s correlation coefficients between log-transformed abundance and year. * P < 0.05, ** P < 0.01, *** P < 0.001.
Figure 5. Temporal trends in the regional-scale population sizes of sessile animals. These trends were based on Pearson’s and Spearman’s correlation coefficients between log-transformed abundance and year. * P < 0.05, ** P < 0.01, *** P < 0.001.
Figure 6. Temporal trends in the regional-scale population sizes of herbivorous benthos. These trends were based on Pearson’s and Spearman’s correlation coefficients between log-transformed abundance and year. * P < 0.05, *** P < 0.001.
Figure 7. Temporal trends in the regional-scale population sizes of carnivorous benthos. These trends were based on Pearson’s and Spearman’s correlation coefficients between log-transformed abundance and year.
For most of the species, the standard partial regression coefficients of one of the explanatory variables, namely the log of abundance in year t, were significantly negative (Tables 3, 4), indicating that the population dynamics were under negative feedback control for these species.
Table 3. Population dynamics of sessile species (benthic algae, sessile animals), assuming that the population dynamics followed a Gompertz-type autoregressive model.
Table 4. Population dynamics of mobile species (herbivorous benthos, carnivorous benthos), assuming that the population dynamics followed a Gompertz-type autoregressive model.
The influence of environmental variables on the population dynamics of sessile species (benthic algae and sessile animals) varied by species (Table 3). For benthic algae, the population dynamics of Pterosiphonia bipinnata was significantly positively influenced by PC1. The population dynamics of Pterosiphonia bipinnata was significantly positively influenced by pH of sea-surface water. The population dynamics of Hildenbrandia spp. and Ptilota pectinata were significantly negatively influenced by pH of sea-surface water. For sessile animals, the population dynamics of Dexiospira spirillum and Halichondria panicea were significantly negatively influenced by pH of sea-surface water.
The influence of each environmental variable on the population dynamics of mobile benthos (herbivorous and carnivorous benthos) varied among species (Table 4). Littorina sitkana was significantly negatively influenced by PC1. pH of sea-surface water had a significant positive influence on L. cassis, L. uchidai, L. radiata, and Nucella lima.
There were significant positive correlations between species’ thermal niche and both Pearson’s and Spearman’s correlation coefficients between year and the log-transformed abundance values (Figures 8A, B).
Figure 8. Relationships between species properties (thermal niche, species’ calcifying status, and vertical niche) and temporal trend of abundance for each species. (A) Pearson’s correlation coefficients between species’ thermal niche and Pearson’s correlation coefficients between year and log-transformed abundance values for all species. (B) Pearson’s correlation coefficients between species’ thermal niche and Spearman’s correlation coefficients between year and log-transformed abundance values for all species. (C) Difference in Pearson’s correlation coefficients between year and log-transformed abundance values for all species, depending on species’ calcifying status, for all species examined by Welch’s test. (D) Difference in Spearman’s correlation coefficients between year and log-transformed abundance values for all species, depending on species’ calcifying status, for all species examined by Welch’s test. (E) Pearson’s correlation coefficients between species’ vertical niche and Pearson’s correlation coefficients between year and log-transformed abundance values for sessile species (benthic algae, sessile animals). (F) Pearson’s correlation coefficients between species’ vertical niche and Pearson’s correlation coefficients between year and log-transformed abundance values for sessile species (benthic algae, sessile animals). * P < 0.05, ** P < 0.01.
Compared with non-calcifying species, calcifying species had significantly smaller Pearson’s correlation coefficients between year and the log-transformed abundance values (Figure 8C). On the other hands, they had no significantly differences Spearman’s rank correlation coefficients between year and the log-transformed abundance values (Figure 8D).
No significant correlations were found between species’ vertical niche and either Pearson’s or Spearman’s correlation coefficient between year and the log-transformed abundance values for sessile species (Figures 8E, F).
The 21-year temporal trends in community abundance detected in benthic algae, herbivorous benthos, and carnivorous benthos may have been caused by direct or indirect influences of climate change. The temporal trend in community abundance in benthic algae (increasing) and herbivorous benthos (decreasing) may have been driven by the direct influence of increasing temperatures during 2003 to 2023, because PC1 influenced community dynamics in benthic algae (positive) and herbivorous benthos (negative). The decreasing trend in community abundance of carnivorous benthos may have been caused by the direct influence of ocean acidification, because there was a significant positive influence of pH of sea-surface water on community dynamics. The increasing trend in the community abundance of benthic algae also may be attributable to a trophic cascade caused by a decrease in herbivorous benthos with increasing temperature during 2003 to 2023, because grazing pressure often limits the community abundance of benthic algae in rocky intertidal zones (Cubit, 1984; Guerry et al., 2009; Poore et al., 2012).
Some of the 21-year temporal trends in population size detected in the rocky intertidal community were likely to have been caused by the direct or indirect influences of climate change. Of the nine benthic algae that showed significant temporal trends in population size, P. bipinnata, which showed a decreasing trend, was negatively influenced by ocean acidification (positively influenced by pH of sea-surface water). However, it was also positively influenced by PC1, indicating warming. This suggests that decrease due to ocean acidification exceeded increase due to warming on P. bipinnata. In contrast, the increasing trends of Hildenbrandia spp. and Ptilota pectinata may have been caused by ocean acidification, because they were influenced negatively by pH of sea-surface water. Of the five species of sessile animals that showed significant temporal trends in population size, Dexiospira spirillum and Halichondria panicea showed an increasing trend due to ocean acidification. Of the three species of herbivorous benthos that showed significant temporal trends in population size, L. cassis and L. radiata showed a decreasing trend due to ocean acidification: pH of sea-surface water, which was negatively correlated with the number of years, had a significant positive influence on the population dynamics (see Table 4).
Ocean acidification may be more important than warming as a cause of temporal population trends in the rocky intertidal community, because more species had their population dynamics influenced by the ocean acidification (pH of sea-surface water, showed decreasing trend) than had their population dynamics influenced by the warming (PC1, showed increasing trend). Of the seventeen species that displayed temporal population trends, one was influenced by PC1, whereas seven were influenced by the pH of sea-surface water.
For benthic algae, community abundance showed an increasing trend, whereas the population size of half of the algal species showed an increasing trend. For sessile animals, community abundance showed no temporal trend, whereas population size of five of the seven species of sessile animals showed a temporal trend. Such differences in temporal trends between community abundance and population size may be due to the fact that temporal trends in community abundance are highly influenced by the temporal population trends of the dominant species. For example, of the dominant benthic algae species, only C. pilulifera showed a temporal population trend. In contrast, Chthamalus dalli, the most dominant species among the sessile animals, showed no temporal trend. Similarly, the temporal trends of community abundance of both herbivorous benthos and carnivorous benthos were highly dependent on the temporal population trends of the dominant species; the decreasing trends in community abundance for both functional groups were dominated by the temporal trends of the dominant species (L. cassis and L. radiata in herbivorous benthos and N. lima in carnivorous benthos).
Identifying the species niche properties susceptible to climate changes will improve the predictability the responses of marine organisms to climate changes. The results of our study show that over the 21-year period from 2003 to 2023, temporal trends in species abundance in rocky intertidal communities differed among species, with an increase in abundance of species with higher temperature niches and a decrease in species with lower thermal niches (Figures 8A, B). A relationship between thermal niche and species response to marine heat waves has also been reported, with marine heat waves causing a decrease in cold-water species and an increase in warm-water species (Smale et al., 2017; Ishida et al., 2023). These facts suggest that focusing on the temperature niche among various species niche characteristics may help to understand and predict the ecological processes behind the response of marine organisms to warming.
Decreasing population trends were more prominent among calcifying species than among non-calcifying species, possibly because calcifying species are highly susceptible to the acidification of surface seawater (Harvey et al., 2013), because there was a significant difference in Pearson’s and correlation coefficients between calcifying and non-calcifying species (Figure 8C).
Although D. spirillum is a calcifying species, it showed increasing population trend and their population growth rates increased by ocean acidification. Serpulidae, including D. spirillum, have been reported to have fragile calcareous tubes upon acidification (Díaz-Castañeda et al., 2019), contradicting the expected results. Future research will be needed to clarify this discrepancy between population response and morpho-physical vulnerability to ocean acidification.
We hypothesized that rocky intertidal organisms with higher vertical distributions (i.e., species with higher vertical niche positions) would be less vulnerable to warming, because they are regularly exposed to broader, or even more extreme, thermal conditions. However, this was not the case: we found only non-significant, weak negative correlations between species’ vertical niche and both Pearson’s and Spearman’s correlation coefficients between year and log-transformed abundance values for sessile species. It is unclear whether this result was simply due to the low importance of vertical niche in vulnerability to warming or to the low statistical power resulting from the small sample size (small number of species analyzed) in our analysis.
Unlike long-term ecosystem changes associated with warming, ecosystem responses to marine heat waves are easily evaluated without the need for long-term census data spanning decades. Consequently, our knowledge of ecosystem responses to marine heat waves has been rapidly increasing in recent years (Hobday et al., 2016). The ability to use ecosystem responses to marine heat waves to estimate the long-term ecosystem changes associated with warming would give us a great advantage in establishing plans for the sustainable use and conservation of ecosystems by predicting marine ecosystems under ongoing climate change. However, we still do not know whether ecosystem responses to marine heat waves a good indicator of the long-term ecosystem changes are associated with warming, because these responses have rarely been compared with the long-term changes associated with progressive warming in the same ecosystem in the same region. Fortunately, however, this comparison can be performed for the rocky intertidal ecosystem on the eastern Pacific coast of Hokkaido, Japan. Comparison of our current results with the responses of community abundance to marine heat waves in our study area (Ishida et al., 2023) revealed agreement in terms of decreases in community size for herbivorous benthos, as well as an increase in community size for benthic algae. However, the causes of the temporal trends in community abundance were different. For example, the long-term decreasing trend in the community abundance of herbivorous benthos was caused by temporal trends in pH of sea-surface water. Ishida et al., 2023 confirmed a decrease due to marine heat waves (i.e., higher temperatures), which is inconsistent with the trend in this study. These findings suggest that long-term ecosystem changes associated with the progression of global warming are not likely to be predicted from the responses of ecosystems to marine heat waves. An important reason for this may be those studies of the influences of marine heat waves have not adequately considered the calcifying status of species, which is a crucial property that determines their response to ocean acidification. Therefore, our understanding of these factors is still incomplete, but it may be particularly important for predicting long-term ecosystem changes associated with warming. In contrast, the importance of thermal niche in predicting the influence of temperature-related climate change on ecosystems, regardless of the time scale, is confirmed by the fact that, for species differences in population-size changes over time, both changes before and after marine heat waves (Ishida et al., 2023) and long-term temporal trends (this study) were explained well by thermal niche. This confirms the importance of thermal niche in predicting the influence of temperature-related climate change on ecosystems, regardless of the time scale. The strength and frequency of marine heat waves are projected to further increase because of global warming (Frölicher et al., 2018). Predicting long-term ecosystem changes requires an understanding of not only the influences of long-term climate change (as in this study), but also the influences of short-term phenomena such as marine heat waves.
It is important to understand the influence of climate change on marine ecosystems at large scales, such as in the global scale. If the response of marine ecosystems to climate change at the global scale could be predicted from the responses of specific model systems in which ecosystem properties are easily quantified, then this would be of great benefit in planning for the sustainable use and conservation of ecosystems. Rocky intertidal communities have long been model systems for marine ecosystem studies, but it is still unclear whether the response of these systems to climate change will be consistent with the responses of marine ecosystems to climate change at the global scale.
Hu et al. (2022) performed a meta-analysis of the responses of marine ecosystems to climate change by examining numerous fragmented studies of varying scale and precision. The results suggested that primary producers will be highly tolerant of both warming and ocean acidification, whereas herbivores will be strongly negatively influenced by both warming and acidification. The meta-analysis also suggested that, in the global scale, the influence of climate change on marine ecosystems will be more pronounced from ocean acidification than from warming, and that this influence will be more pronounced in calcifying species. These results (Hu et al., 2022) are generally consistent with ours, in which the negative influence of climate change was more pronounced in herbivorous benthos than in sessile algae and the negative influence of the pH of sea-surface water on population dynamics (which is considered to reflect the progression of ocean acidification) was more pronounced in calcifying species (Figure 8C). These findings may indicate that rocky intertidal communities are well suited as model systems for examining the influence of climate change on marine ecosystems. They also once again highlight the usefulness of rocky intertidal zones as model systems in ecosystem studies.
Here, we clarified the temporal patterns and underlying processes of the 21-year dynamics of four functional groups (benthic algae, sessile animals, herbivorous benthos, and carnivorous benthos) in rocky intertidal communities at both the functional-group level and the species level on a regional scale. At the functional-group level, the community abundance of benthic algae was increasing, whereas those of both herbivorous benthos and carnivorous benthos were decreasing. The population sizes of 12 of the 31 species examined were increasing, whereas 4 of those were decreasing. The temporal trends in community abundance were highly influenced by the temporal population trends of the dominant species. At both the functional-group level and the species level, the temporal trends in abundance were likely to have been caused by the direct or indirect influences of warming, acidification of surface seawater. Temporal trends in population size, and their driving mechanisms, were to some extent related to species properties: temporal trends in population size increased for species with high thermal niches and decreased for species with low thermal niches and for calcifying species. Comparison of our results with those of community abundance in response to marine heat waves in our study area (Ishida et al., 2023) had two implications. First, long-term ecosystem changes associated with the progression of global warming are not likely to be predicted from the responses of ecosystems to marine heat waves, possibly because of our lack of knowledge of the influence of species’ calcifying status on species’ response to climate change. Secondly, thermal niche contributes greatly to our prediction of the influence of temperature-related climate change on population size, regardless of the time scale. Our results are generally consistent with those of a preliminary meta-analysis of general trends in the responses of marine ecosystems to climate change (Hu et al., 2022). This may demonstrate that rocky intertidal communities have characteristics that make them suitable model systems for examining the influences of climate change on marine ecosystems.
The raw data supporting the conclusions of this article will be made available by the authors, without undue reservation.
The manuscript presents research on animals that do not require ethical approval for their study.
HS: Data curation, Formal Analysis, Investigation, Methodology, Project administration, Writing – original draft. KI: Data curation, Investigation, Methodology, Supervision, Writing – review & editing. TN: Conceptualization, Data curation, Funding acquisition, Investigation, Methodology, Supervision, Writing – review & editing.
The author(s) declare that financial support was received for the research, authorship, and/or publication of this article. This research was funded by grants-in-aid from the Japan Society for the Promotion of Science to TN (nos. 20570012, 24570012, 15K07208, 18H02503, 23H02546 and 23K27237).
We also thank Drs. I. Koizumi, M. Nakaoka, and M. Senzaki for reviewing this paper. We thank Dr. A. Iwasaki, A. Shikine, and R. Motomatsu of Tohoku University, and Dr. Y. Kanamori of the Japan Fisheries Research and Education Agency, for the field census. We additionally thank Mr.s M. Tachibana, R. Fujii, Y. Yao, A. Lu, S. Arai, R. Inatomi, S. Nara, H. Imatake, Ms.s H. Kubota, A. Imamura, H. Ogawa, and Dr. Y. Wadafor their helpful discussions, as well as for the field census and data analysis. This work was made possible by the generous support and encouragement of Ms. M. Tanaka; the local fishers and fishery officers of the Hokkaido Federation of Fisheries Cooperative Associations; and our families.
The authors declare that the research was conducted in the absence of any commercial or financial relationships that could be construed as a potential conflict of interest.
All claims expressed in this article are solely those of the authors and do not necessarily represent those of their affiliated organizations, or those of the publisher, the editors and the reviewers. Any product that may be evaluated in this article, or claim that may be made by its manufacturer, is not guaranteed or endorsed by the publisher.
The Supplementary Material for this article can be found online at: https://www.frontiersin.org/articles/10.3389/fmars.2024.1477142/full#supplementary-material
Benedetti-Cecchi L., Bertocci I., Vaselli S., Maggi E. (2006). Temporal variance reverses the impact of high mean intensity of stress in climate change experiments. Ecology 87, 2489–2499. doi: 10.1890/0012-9658(2006)87[2489:TVRTIO]2.0.CO;2
Blondel J. (2003). Guilds or functional groups: does it matter? Oikos 100, 223–231. doi: 10.1034/j.1600-0706.2003.12152.x
Caldeira K., Wickett M. E. (2005). Ocean model predictions of chemistry changes from carbon dioxide emissions to the atmosphere and ocean. J. Geophys. Res. 110, C09S04. doi: 10.1029/2004JC002671
Cubit J. D. (1984). Herbivory and the seasonal abundance of algae on a high intertidal rocky shore. Ecology 65, 1904–1917. doi: 10.2307/1937788
Cummins K. W. (1974). Structure and function of stream ecosystems. BioScience 24, 631–641. doi: 10.2307/1296676
Dennis B., Ponciano J. M., Lele S. R., Taper M. L., Staples D. F. (2006). Estimating density dependence, process noise, and observation error. Ecol. Monogr. 76, 323–341. doi: 10.1890/0012-9615(2006)76[323:EDDPNA]2.0.CO;2
Díaz-Castañeda V., Cox T. E., Gazeau F., Fitzer S., Delille J., Alliouane S., et al. (2019). Ocean acidification affects calcareous tube growth in adults and reared offspring of serpulid polychaetes. J. Exp. Biol. 222, jeb196543. doi: 10.1242/jeb.196543
Feely R., Chris S., Lee K., Berelson W., Kleypas J., Fabry V., et al. (2004). Impact of anthropogenic CO2 on the caCO3 system in the oceans. Science 305, 362–366. doi: 10.1126/science.1097329
Fitzer S. C., Cusack M., Phoenix V. R., Kamenos N. A. (2014). Ocean acidification reduces the crystallographic control in juvenile mussel shells. J. Struct. Biol. 188, 39–45. doi: 10.1016/j.jsb.2014.08.007
Frölicher T. L., Fischer E. M., Gruber N. (2018). Marine heatwaves under global warming. Nature 560, 360–364. doi: 10.1038/s41586-018-0383-9
Giddens J., Kobayashi D. R., Mukai G. N., Asher J., Birkeland C., Fitchett M., et al. (2022). Assessing the vulnerability of marine life to climate change in the Pacific Islands region. PloS One 17, e0270930. doi: 10.1371/journal.pone.0270930
Guerry A. D., Menge B. A., Dunmore R. A. (2009). Effects of consumers and enrichment on abundance and diversity of benthic algae in a rocky intertidal community. J. Exp. Mar. Biol. Ecol. 369, 155–164. doi: 10.1016/j.jembe.2008.11.011
Harvey B. P., Gwynn-Jones D., Moore P. J. (2013). Meta-analysis reveals complex marine biological responses to the interactive effects of ocean acidification and warming. Ecol. Evol. 3, 1016–1030. doi: 10.1002/ece3.516
Hobday A. J., Alexander L. V., Perkins S. E., Smale D. A., Straub S. C., Oliver E. C., et al. (2016). A hierarchical approach to defining marine heatwaves. Prog. Oceanogr. 141, 227–238. doi: 10.1016/j.pocean.2015.12.014
Hu N., Bourdeau P. E., Harlos C., Liu Y., Hollander J. (2022). Meta-analysis reveals variance in tolerance to climate change across marine trophic levels. Sci. Total Environ. 827, 154244. doi: 10.1016/j.scitotenv.2022.154244
IPCC (Intergovernmental Panel on Climate Change) (2001).Climate Change 2001: The Scientific Basis. In: Contribution of Working Group I to the Third Assessment Report of the Intergovernmental Panel on Climate Change (New York: Cambridge Univ. Press). Available online at: https://www.ipcc.ch/report/ar3/wg1/ (Accessed February 3, 2024).
IPCC (Intergovernmental Panel on Climate Change) (2007).Climate Change 2007: The Scientific Basis. In: Contribution of Working Group I to the Fourth Assessment Report of the Intergovernmental Panel on Climate Change (New York: Cambridge Univ. Press). Available online at: https://www.ipcc.ch/report/ar4/syr/ (Accessed February 3, 2024).
Ishida K., Tachibana M., Hori M., Okuda T., Yamamoto T., Nakaoka M., et al. (2021). Quantifying the dynamics of rocky intertidal sessile communities along the pacific coast of Japan: implications for ecological resilience. Sci. Rep. 11, 16073. doi: 10.1038/s41598-021-95348-1
Ishida K., Tachibana M., Yao Y., Wada Y., Noda T. (2023). The impact of marine heatwaves on rocky intertidal communities: evidence of accumulative carryover effects of marine heatwaves. Front. Mar. Sci. 10. doi: 10.3389/fmars.2023.1146148
Iwasaki A., Fukaya K., Noda T. (2016). “Quantitative evaluation of the impact of the great East Japan earthquake and tsunami on the rocky intertidal community,” in Ecological impacts of tsunamis on coastal ecosystems: lessons from the great East Japan earthquake, ecological research monographs, vol. 35–46 . Eds. Urabe J., Nakashizuka T. (Springer, Tokyo).
Iwasaki A., Noda T. (2018). A framework for quantifying the relationship between intensity and severity of impact of disturbance across types of events and species. Sci. Rep. 8, 795. doi: 10.1038/s41598-017-19048-5
Iwataki M., Lum W. M., Kuwata K., Takahashi K., Arima D., Kuribayashi T., et al. (2022). Morphological variation and phylogeny of Karenia selliformis (Gymnodiniales, Dinophyceae) in an intensive cold-water algal bloom in eastern Hokkaido, Japan. Harmful Algae 114, 102204. doi: 10.1016/j.hal.2022.102204
Japan Meteorological Agency Statistical data on coastal sea ice observations in Hokkaido. Available online at: https://www.data.jma.go.jp/kaiyou/db/seaice/hokkaido/hokkaido_static.html (Accessed October 21, 2024).
Japan Meteorological Agency (2023). Long-term trend of pH change in surface seawater (near Japan). Available online at: https://www.data.jma.go.jp/kaiyou/shindan/a_3/pHtrend_jpn/pHtrend_jpn.html (Accessed October 21, 2024).
Kanamori Y., Fukaya K., Noda T. (2017). Seasonal changes in community structure along a vertical gradient: patterns and processes in rocky intertidal sessile assemblages. Popul. Ecol. 59, 301–313. doi: 10.1007/s10144-017-0596-z
Kinlan B. P., Gaines S. D. (2003). Propagulre dispersal in Marine and terrestrial environments: A community perspective. Ecology 84, 2007–2020. doi: 10.1890/01-0622
Kleypas J. A., Yates K. K. (2009). Coral reefs and ocean acidification. Oceanography 22, 108–117. Available at: http://www.jstor.org/stable/24861028 (Accessed January 7, 2025).
Kunze C., Wölfelschneider M., Rölfer L. (2021). Multiple driver impacts on rocky intertidal systems: The need for an integrated approach. Front. Mar. Sci. 8. doi: 10.3389/fmars.2021.667168
Kuroda H., Azumaya T., Setou T., Hasegawa N. (2021). Unprecedented outbreak of harmful algae in Pacific coastal waters off southeast Hokkaido, Japan, during late summer 2021 after record-breaking marine heatwaves. J. Mar. Sci. Eng. 9, 1335. doi: 10.3390/jmse9121335
Kuroda H., Taniuchi Y., Watanabe T., Azumaya T., Hasegawa N. (2022). Distribution of harmful algae (Karenia spp.) in October 2021 off southeast Hokkaido, Japan. Front. Mar. Sci. 9. doi: 10.3389/fmars.2022.84136
Menge B. A. (1976). Organization of the new England rocky intertidal community: role of predation, competition, and environmental heterogeneity. Ecol. Monogr. 46, 355–393. doi: 10.2307/1942563
Menge B. A., Lubchenco J., Gaines S. D., Ashkenas R. L. (1986). A test of the menge-Sutherland model of community organization in a tropical rocky intertidal food web. Oecologia 71, 75–89. doi: 10.1007/BF00377324
Mieszkowska N., Burrows M. T., Hawkins J. S., Sugden H. (2021). Impacts of pervasive climate change and extreme events on rocky intertidal communities: evidence from long-term data. Front. Mar. Sci. 8. doi: 10.3389/fmars.2021.642764
Nayak S., Takemi T. (2020a). Typhoon induced precipitation characterization over northern Japan: a case study for typhoons in 2016. Prog. Earth Planet. Sci. 7, 39. doi: 10.1186/s40645-020-00347-x
Nayak S., Takemi T. (2020b). Robust responses of typhoon hazards in northern Japan to global warming climate: cases of landfalling typhoons in 2016. Meteorol 27, e1954. doi: 10.1002/met.1954
Nishimura S. (1995). Guide to seashore animals of Japan with Color Pictures and Keys (Osaka, Japan: Hoikusha).
Noda T. (2004). Spatial hierarchical approach in community ecology: a way beyond high context-dependency and low predictability in local phenomena. Popul. Ecol. 46, 105–117. doi: 10.1007/s10144-004-0184-
Noda T. (2009). Metacommunity-level coexistence mechanisms in rocky intertidal sessile assemblages based on a new empirical synthesis. Popul. Ecol. 51, 41–55. doi: 10.1007/s10144-008-0117-1
Noda T., Ohira M. (2020). Transition in population dynamics of the intertidal barnacle Balanus glandula after invasion: causes and consequences of change in larval supply. J. Mar. Sci. Eng. 8, 915. doi: 10.3390/jmse8110915
Okuda T., Noda T., Yamamoto T., Hori M., Nakaoka M. (2010). Contribution of environmental and spatial processes to rocky intertidal metacommunity structure. Acta Oecol. 36, 413–422. doi: 10.1016/j.actao.2010.04.002
Payne M. C., Brown C. A., Reusser D. A., Lee H. II (2012). Ecoregional analysis of nearshore sea-surface temperature in the north pacific. PloS One 7, e30105. doi: 10.1371/journal.pone.0030105
Poore A. G. B., Campbell A. H., Coleman R. A., Edgar G. J., Jormalainen V., Reynolds P. L., et al. (2012). Global patterns in the impact of marine herbivores on benthic primary producers. Ecol. Lett. 15, 912–922. doi: 10.1111/j.1461-0248.2012.01804.x
Ruthrof K. X., Breshears D. D., Fontaine J. B., Froend R. H., Matusick G., Kala J., et al. (2018). Subcontinental heat wave triggers terrestrial and marine, multi-taxa responses. Sci. Rep. 8, 13094. doi: 10.1038/s41598-018-31236-5
Scrosati R. A., Freeman M. J., Ellrich J. A., Petzold W. (2022). Biogeography of algae and invertebrates from wave- exposed rocky intertidal habitats along the Atlantic coast of Nova Scotia (Canada): Latitudinal and interannual patterns and possible underlying drivers. Front. Mar. Sci. 9. doi: 10.3389/fmars.2022.987162
Smale D. A., Wernberg T. (2013). Extreme climatic event drives range contraction of a habitat-forming species. Proc. R. Soc B. Biol. Sci. 280, 1–9. doi: 10.1098/rspb.2012.2829
Smale D. A., Wernberg T., Vanderklift M. A. (2017). Regional-scale variability in the response of benthic macroinvertebrate assemblages to a marine heatwave. Mar. Ecol. Prog. Ser. 568, 17–30. doi: 10.3354/meps12080
Spalding M. D., Fox H. E., Allen G. R., Davidson N., Ferdaña Z. A., Finlayson M., et al. (2007). Marine ecoregions of the world: a bioregionalization of coastal and shelf areas. BioScience 57, 573–583. doi: 10.1641/B570707
Suryan R. M., Arimitsu M. L., Coletti H. A., Hopcroft R. R., Lindeberg M. R., Barbeaux S. J., et al. (2021). Ecosystem response persists after a prolonged marine heatwave. Sci. Rep. 11, 6235. doi: 10.1038/s41598-021-83818-5
Turchin P. (2003). Complex population dynamics: a theoretical/empirical synthesis (Princeton, United States: Princeton University Press).
Watt C. A., Scrosati R. A. (2013). Bioengineer effects on understory species richness, diversity, and composition change along an environmental stress gradient: experimental and mensurative evidence. Estuar. Coast. Shelf Sci. 123, 10–18. doi: 10.1016/j.ecss.2013.02.006
Weitzman B., Konar B., Iken K., Coletti H., Monson D., Suryan R., et al. (2021). Changes in rocky intertidal community structure during a marine heatwave in the northern gulf of Alaska. Front. Mar. Sci. 8. doi: 10.3389/fmars.2021.556820
Wernberg T., Smale D. A., Tuya F., Thomsen M. S., Langlois T. J., De Bettignies T., et al. (2013). An extreme climatic event alters marine ecosystem structure in a global biodiversity hotspot. Nat. Clim. Change 3, 78–82. doi: 10.1038/nclimate1627
Yao Y., Noda T. (2024). Immediate impact of the 2021 Harmful Algal Bloom in southeast Hokkaido on the rocky intertidal benthic community and its spatial variation. J. Mar. Sci. Eng. 12, 928. doi: 10.3390/jmse12060928
Keywords: climate change, community dynamics, abundance, rocky intertidal shore, temporal trend, functional group, thermal niche, species calcifying status
Citation: Sato H, Ishida K and Noda T (2025) Temporal trends of community and climate changes in the anthropocene: 21-year dynamics of four major functional groups in a rocky intertidal habitat along the Pacific coast of Japan. Front. Mar. Sci. 11:1477142. doi: 10.3389/fmars.2024.1477142
Received: 07 August 2024; Accepted: 23 December 2024;
Published: 14 January 2025.
Edited by:
Alberto Basset, University of Salento, ItalyReviewed by:
Lucia Fanini, University of Salento, ItalyCopyright © 2025 Sato, Ishida and Noda. This is an open-access article distributed under the terms of the Creative Commons Attribution License (CC BY). The use, distribution or reproduction in other forums is permitted, provided the original author(s) and the copyright owner(s) are credited and that the original publication in this journal is cited, in accordance with accepted academic practice. No use, distribution or reproduction is permitted which does not comply with these terms.
*Correspondence: Hiroki Sato, cG93amFtZmNkc0BlaXMuaG9rdWRhaS5hYy5qcA==
Disclaimer: All claims expressed in this article are solely those of the authors and do not necessarily represent those of their affiliated organizations, or those of the publisher, the editors and the reviewers. Any product that may be evaluated in this article or claim that may be made by its manufacturer is not guaranteed or endorsed by the publisher.
Research integrity at Frontiers
Learn more about the work of our research integrity team to safeguard the quality of each article we publish.