- 1Department of Environmental Systems Science, Institute of Biogeochemistry and Pollutant Dynamics, Eidgenössische Technische Hochschule (ETH) Zürich, Zurich, Switzerland
- 2Institute of Earth Sciences, University of Lausanne, Lausanne, Switzerland
- 3Bedford Institute of Oceanography, Fisheries and Oceans Canada, Dartmouth, NS, Canada
- 4Laboratory of Ion Beam Physics, Department of Physics, ETH Zurich, Zurich, Switzerland
- 5Instituto de Investigacións Mariñas, IIM-CSIC, Vigo, Spain
- 6Laboratoire d’Océanographie Physique et Spatiale (LOPS), University of Brest, CNRS, Ifremer, IRD, IUEM, Plouzané, France
The subpolar North Atlantic (SPNA) is crucial in the global ocean circulation system and one of the few regions where deep convection occurs. The intermediate and deep waters formed in the SPNA have long been investigated, yet their sources and pathways are not fully understood. In this study, we employ a combination of two radionuclide tracers, namely, 129I and 236U, to understand water mass provenance and mixing in the SPNA. The concentrations measured between Portugal and Greenland and across the Labrador Sea in 2020/2021 agreed with previously observed tracer distributions. The highest tracer concentrations were measured in the East Greenland Current (EGC), Denmark Strait Overflow Water (DSOW), and, to a lesser extent, in the eastward-flowing Labrador Sea Water (LSW). In contrast, waters of southern origin such as the North East Antarctic Bottom Water and North East Atlantic Central Water (ENACW) carried comparably smaller amounts of 129I. By using a binary mixing model, we estimated that the EGC contains about 29%–32% of the Polar Surface Water outflowing the Fram Strait. DSOW was mainly derived from 20% to 35% Return Atlantic Water and mixed with LSW. The Iceland Scotland Overflow Water (ISOW) evolved into North East Atlantic Deep Water in the Irminger and Labrador seas primarily by mixing with LSW and, to a lesser extent, with DSOW. The 129I and 236U binary mixing approach was less conclusive for LSW, reaching the current limitation of the model. This study suggests potential benefits and limitations of using 129I and 236U to investigate the mixing and provenance of water masses in the SPNA.
1 Introduction
1.1 The subpolar North Atlantic
The subpolar North Atlantic (SPNA) has been recognized as key region for intermediate and deep-water formation since the last century e.g., Clarke and Gascard (1983); Pickart (1992). As such, the SPNA largely contributes to the formation of the southward flowing lower limb of the Atlantic Meridional Overturning Circulation (AMOC) by conversion of shallow northward flowing warm subtropical waters into colder, fresher, and denser waters (Frajka-Williams et al., 2019; Weijer et al., 2022; Lozier, 2023). The AMOC plays a major role in the climate system due to its great capacity to distribute heat and carbon. Thus, understanding its dynamics is of paramount importance (Zou et al., 2023; Lozier et al., 2019; Rousi et al., 2021). Mooring arrays, floats, and repeated hydrographic expeditions, such as the AR7W line in the Labrador Sea and the OVIDE Line in the central SPNA (Figure 1, red squares), closely monitor the AMOC variability and strength (Li et al., 2021; García-Ibáñez et al., 2018; Lavender et al., 2005; Frajka-Williams et al., 2019) as it may weaken or even collapse within this century (Ditlevsen and Ditlevsen, 2023). The main contributions to the lower limb of AMOC are the Labrador Sea Water (LSW), the Denmark Strait Overflow Water (DSOW), and the Iceland Scotland Overflow Water (ISOW) (Figure 1). In the Labrador Sea, the annual winter convection produces new LSW to a maximum depth of about 2000 m (Yashayaev, 2024). Nordic overflows entering through the Denmark Strait near Greenland (i.e., DSOW) and the Iceland–Scotland sills (i.e., ISOW) fill bottom or deeper depths, respectively (e.g., Bower et al., 2019, 2009).
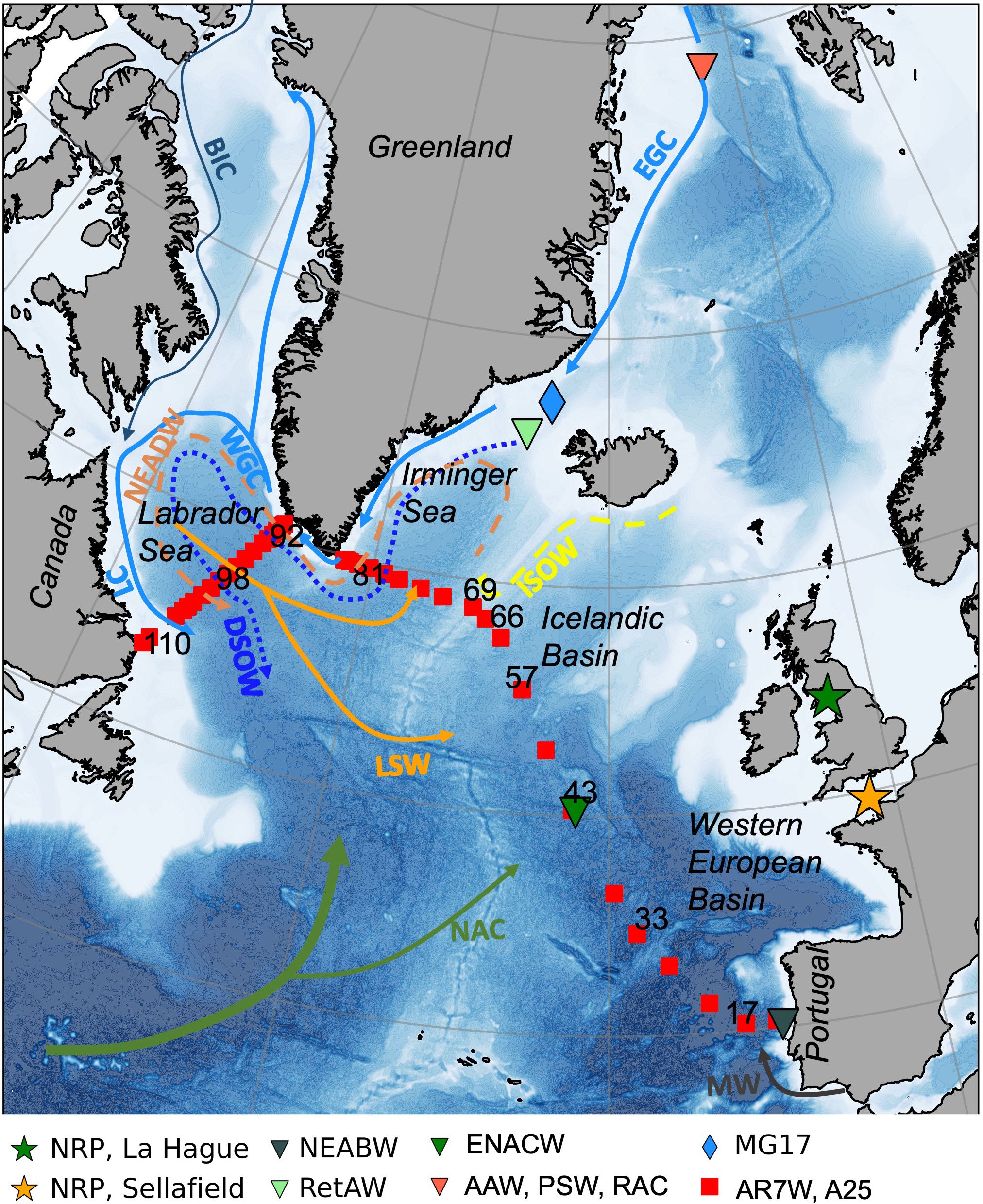
Figure 1. Study area, including the oceanographic transects AR7W and A25 sampled in 2020/2021. Red squares represent the location of the sampled stations, and their corresponding station numbers (not all were included to avoid too many labels). Arrows represent the schematic water mass circulation adapted from Daniault et al. (2016). The location of the nuclear fuel reprocessing plants of La Hague and Sellafield is represented by green and orange stars, respectively. The sampling locations of the water mass endmembers are indicated by triangles, and the endmember tracer concentration can be found in Supplementary Table S1. The blue diamond MG17 is a single EGC sample, published by Dale et al. (2024). AAW, Arctic Atlantic Water; BIC, Baffin Island Current; ENACW, Eastern North Atlantic Central Waters; DSOW, Denmark Strait Overflow Water; EGC, East Greenland Current; ISOW, Iceland Scotland Overflow Water; LC, Labrador Current; LSW, Labrador Sea Water; MW, Mediterranean Water; NAC, North Atlantic Current; NCC, Norwegian Coastal Current; NEABW, North East Atlantic Bottom Water; NRP, nuclear reprocessing plant; PSW, Polar Surface Water; WGC, West Greenland Current.
Ongoing AMOC research is trying to better understand the water mass structure, pathways, mixing, and origin (Zou et al., 2023)—for example, the Deep Western Boundary Current is broadly seen as the main mechanism to transport recently ventilated LSW and Nordic overflows to lower latitudes. However, tracer and float observations indicate intense re-circulation of waters and branching off the boundary current, resulting in extensive eastward intrusion of North Atlantic Deep Water (Susan Lozier et al., 2022). Furthermore, LSW has been shown to recirculate within the subpolar gyre and cross the Reykjanes Ridge into the eastern SPNA (Lavender et al., 2005; Lozier et al., 2013; Susan Lozier et al., 2017). The composition and origin of the above-mentioned waters are still a matter of investigation as it is their mixing with water masses formed south of the subpolar region.
1.2 Anthropogenic radionuclides as tracers of ocean circulation
Since the 1990s, anthropogenic radionuclides such as 137Cs, 90Sr, and 3H have been used to track the Atlantic waters flowing within the Arctic and North Atlantic regions (Dahlgaard et al., 1995; Livingston and Anderson, 1983; Smith et al., 1998). Other than the global fallout input following the atmospheric nuclear bomb tests, the main source of these radionuclides are the liquid releases from the two European nuclear reprocessing plants in Sellafield, UK, and La Hague, F (green and orange stars in Figure 1). The point-like radionuclide source which starts at the North Sea has been labeling the Atlantic waters entering the Arctic region and flowing back to the SPNA, allowing the pathways of Atlantic-sourced waters to be traced and the transport times and mixing to be estimated (Raisbeck and Yiou, 1999; Kershaw and Baxter, 1995; Alfimov, 2004; Smith et al., 1998; Smith, 2005; Smith et al., 2011). Among these radionuclides, the long-lived 129I emerged as a powerful tracer in the 1990s owing to advances in accelerator mass spectrometry (AMS), allowing 129I determinations in less than 1 L of sample (Christl et al., 2023; Vockenhuber et al., 2015; Christl et al., 2013). The fact that the discharged amounts (129I: >5,000 kg, 236U: >100 kg) (Aldahan et al., 2007; Christl et al., 2015b, a; He et al., 2013) were documented and that they increased considerably after the 1990s (Figure 2A) provided a unique opportunity to resolve transport timescales of tracer-labeled waters beyond the North Sea. Further developments in AMS permitted the measurement of another long-lived radionuclide, 236U, which when combined with 129I resulted in a valuable tool to trace the water exchange within and between the Atlantic and Arctic Oceans (Casacuberta et al., 2016, 2018; Castrillejo et al., 2018). The combination of 129I and 236U serves as a unique dye for water masses thanks to their long half-lives (129I: T1/2 = 15.7 Myr, 236U: T1/2 = 23.5 Myr), their negligible natural abundance, and their conservative behavior in open ocean waters (Aldahan et al., 2007; Sakaguchi et al., 2012).
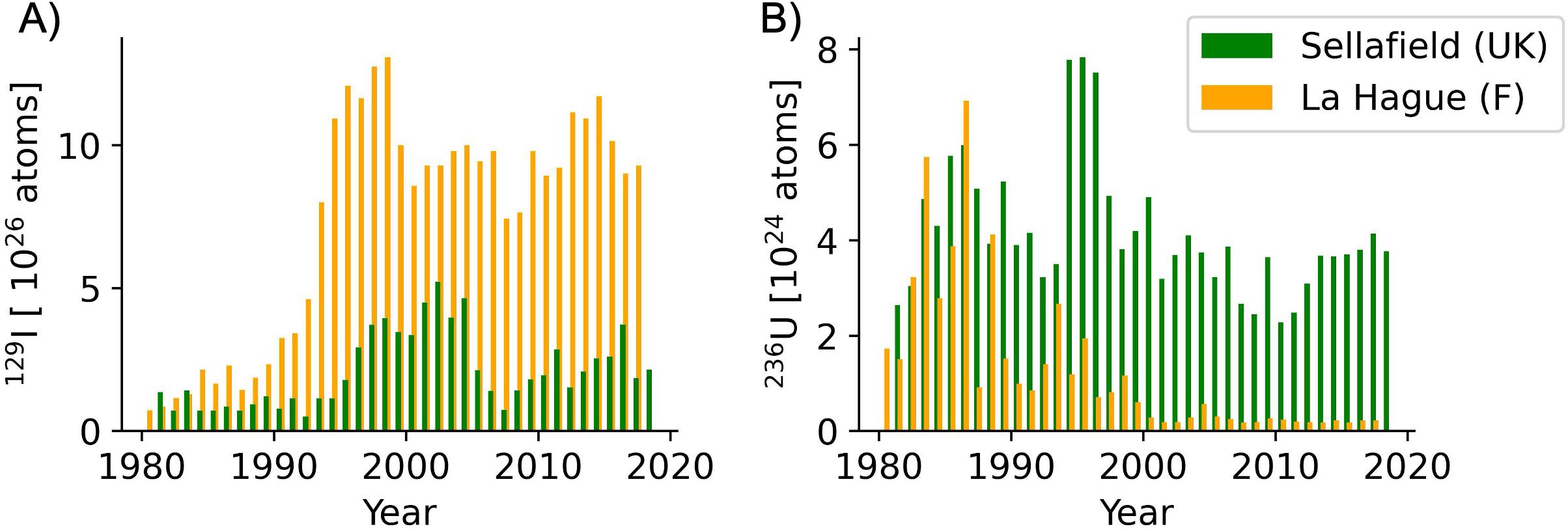
Figure 2. Annual discharge of (A) 129I and (B) 236U from the nuclear fuel reprocessing plants in Sellafield, United Kingdom (UK) and La Hague, France (F).
The combination of 129I and 236U highlights the strength of using tracers with different input histories, as the tracer signature leaving the North Sea is unique for a given year (Casacuberta and Smith, 2023). Although 129I is mostly released from La Hague (Figure 2A) and 236U comes predominantly from Sellafield (Figure 2B), both releases mix at the North Sea before being transported northward to the Barents Sea Opening and the Fram Strait (Smith et al., 2011; Casacuberta et al., 2018; Wefing et al., 2019; Castrillejo et al., 2018). In the Arctic Ocean, these two tracers have recently proven their suitability to estimate pathways, transit times, and mixing of Atlantic-sourced waters (Wefing et al., 2021; Casacuberta and Smith, 2023; Payne et al., 2024).
1.3 129I and 236U in the subpolar North Atlantic
In the SPNA, 129I proved to be a powerful tracer, especially in understanding the pathways and transport timescales of overflow waters such as DSOW, where it was observed since 1981 (Edmonds et al., 1998; Smith, 2005). Recent works by Castrillejo et al. (2018) and Dale et al. (2024) show that elevated concentrations of 129I and 236U are now highlighting the pathways of ISOW and LSW in the SPNA. The first dataset including both tracers took place along section A25 (or OVIDE line) in 2014 (Castrillejo et al., 2018) and two years later at Fram Strait (Wefing et al., 2019). These studies showed that Arctic Atlantic waters exiting the Fram Strait contained high tracer concentrations (up to 129I: 645 ± 16 × 107 at/L, 236U: 21.2 ± 0.5 × 106 at/L) (Wefing et al., 2019) within surface waters carried by the East Greenland Current (EGC) along the Greenland shelf. These waters are transported downstream to Irminger Sea (Havik et al., 2017; Holliday et al., 2007b), where concentrations of 129I: 256 ± 4 × 107 at/kg, 236U: 16 ± 2×106 at/kg were reported by Castrillejo et al. (2018) and Casacuberta and Smith (2023). In contrast to the high-latitude tracer-labeled water masses is the NAC which mostly brings the signal of global fallout from low latitudes (e.g., 129I: 0.2–8×107 at/kg) (Castrillejo et al., 2018). Finally, the North East Atlantic Bottom Waters (NEABW) present in the Western European Basin carry almost no tracers. NEABW originates from the Southern Ocean and has not been exposed to anthropogenic sources of 129I and 236U. These high ranges of concentrations observed in the different water masses at SPNA are key to provide insights into ocean circulation between the Arctic Ocean and SPNA—for example, a very recent work by Dale et al. (2024) proved that the combination of 129I and 236U, in a binary mixing model, can be used to understand ocean circulation and mixing in the vicinity of Iceland. One of the key points of their work is that ISOW and DSOW have different tracer signatures, and thus 129I and 236U can be used to distinguish these two water masses downstream of their source regions.
This study aims to explore water mass provenance and mixing in the SPNA using 129I and 236U. To this end, new 129I and 236U data are presented in seawater collected in the Labrador Sea (2020) and on A25 section (2021). The new tracer data are related to water mass structure and circulation patterns. Provenance and mixing of water masses are then investigated using a 129I–236U binary mixing model. The ultimate goal of this work is to evaluate the strengths and limitations of the binary mixing model at this region of the SPNA.
2 Methods
2.1 Study area
This study focuses on two hydrographic transects represented by red squares in Figure 1. The transect A25 from Portugal to Cape Farewell in southern Greenland covered the OVIDE line. AR7W crosses the Labrador Sea from Hamilton Bank on the Labrador Shelf to Cape Desolation on the Greenland Shelf. A25 and AR7W thus include four major basins, from east to west: the West European Basin (WEB), the Icelandic Basin, the Irminger Sea, and the Labrador Sea. The sections are suitably located to track water exchanges between the low-latitude Atlantic Ocean, Nordic, and Arctic seas. The major water masses and mean circulation of the SPNA are thoroughly reviewed in Daniault et al. (2016) and Liu and Tanhua (2021). Here the most important circulation features are briefly introduced in Figure 1 to provide the basis for the discussion of radionuclide distributions in Section 4.1. The WEB is mainly occupied by northward-flowing shallow waters originating from the subtropical gyre such as the East North Atlantic Central Water (ENACW), underlying Mediterranean Outflow Water (MOW), and a mixture of deep waters with a dominating influence from the Southern Ocean. In this work, deep waters bathing the WEB are named North East Atlantic Bottom Water (NEABW) following Liu and Tanhua (2021). To the west of the sub-Arctic front (≈22.5° W), low-latitude waters diminish to give way to higher-latitude waters. These include shallow waters with lower salinity such as the Subpolar Mode Water (SPMW) and intermediate waters such as LSW that may occupy the water column down to a maximum depth of 2000 m. Below that depth in the Labrador Sea and Iceland basin, dense overflows (ISOW, DSOW) are present after they overspill through sills between Iceland and either Greenland or Scotland, following primarily boundary currents at the Irminger and Labrador seas. Adopting the naming in Yashayaev (2007), waters in the Irminger Sea and the Labrador Sea derived from largely modified ISOW are named hereinafter Northeast Atlantic Deep Water (NEADW). Finally, surface and intermediate polar waters following the Irminger Sea and Labrador Sea boundaries are transported by boundary currents such as the East and the West Greenland Current (EGC and WGC). The latter waters mix with the inflow from the Canadian Arctic Archipelago and are transported by the Labrador Current (Pacini and Pickart, 2022).
2.2 Sampling
Sampling on AR7W took place in May 2020 onboard R/V Amundsen. A25 was visited in June 2021 onboard R/V Sarmiento de Gamboa during the BOCATS2 expedition. The fieldwork aimed at re-visiting stations that were previously sampled for 129I and 236U and to improve the spatial sampling resolution of 129I from previous years. At A25, a total of 280 seawater samples (250 for 129I and 30 for 236U) from 18 depth profiles were collected, covering the WEB (Figure 1, stations 1 to 43), the Iceland basin (stations 44 to 66), the Reykjanes Ridge (stations 67 to 69), and the Irminger Sea (stations 70 to 91). At AR7W in the Labrador Sea, a total of five depth profiles (stations 92 to 110) and 98 seawater samples were collected (79 for 129I and 19 for 236U). The waters were sampled using a rosette equipped with 24 Niskin bottles and conductivity–temperature–depth sensors. The seawater samples for 129I (250 mL) and 236U (2–5 L) were stored in opaque bottles and plastic cubitainers, respectively. The sampling resolution of 236U was lower than for 129I due to water volume constraints. The samples collected on AR7W were shipped to the Laboratory of Ion Beam Physics (LIP) at ETH-Zurich for chemical processing and analysis. On A25, the chemistry for iodine and partly for uranium was done onboard and finalized at LIP.
2.3 Sample processing and measurement
The sample processing for 129I followed the method described elsewhere (e.g., Wefing et al., 2019) which was adapted originally from Michel et al. (2012). Briefly, sample aliquots of about 250 mL were spiked with about 1.5 mg of Woodward iodine (127I) dissolved in solution. After thorough mixing, the aliquots were oxidized to iodate using saturated Ca(ClO)2 solution and then reduced to iodide using Na2S2O5 and NH2OH*HCl. The reaction mixture was raised to pH 5 to pH 6 before passing through a preconditioned DOWEX 1 × 8 ion exchange resin to purify and retain the iodine. Then, 2.25 M KNO3 was used to elute the iodine into an acidified AgNO3 solution, and it was precipitated as AgI. The precipitate was dried, mixed with Ag powder, and pressed into cathodes for AMS measurements.
The compact 0.5-MV Tandy AMS facility at LIP was used to measure the atomic 129I/127I ratios which were normalized to two ETH-Zurich in-house standards with nominal ratios of 129I/127I = 38.995 ± 0.467 × 10−12 (“C2conc”) and 129I/127I = 5.055 ± 0.068 × 10−12 (“C2dil”). The 129I concentration in seawater was calculated based on the measured 129I/127I ratio and the known amount of added Woodward Iodine carrier (Vockenhuber et al., 2015; Christl et al., 2013).
To check for the analytical background and internal reproducibility, one blank and one internal seawater standard were included in every second batch of samples. The blank was prepared with deionized water (n = 27, 129I = 3.8 ± 3 × 104 at/kg). The internal seawater standard was taken from station 15 on A25 (n = 13, 129I = 11.88 ± 0.03 × 107 at/kg).
The seawater samples for 236U were processed following the method described in Wefing et al. (2019) which consists of a pre-concentration and purification step. Briefly, the seawater samples of 2–5 L were acidified using concentrated HNO3 (25%) and spiked with 1 pg of 233U (acidic solution, PTB 2014-1126). Then, Fe(NO3)2 solution was added to the sample and precipitated as Fe2O3 using a concentrated NH3 solution. The Fe2O3 precipitate was redissolved in 8 M HNO3 and passed through UTEVA columns to purify U. The purified U was eluted, co-precipitated with Fe2O3, and thermally oxidized to UO. Finally, the precipitate was mixed with Nb powder and pressed into AMS cathodes.
The uranium isotopic ratios (236U/238U and 236U/233U) were analyzed using the compact AMS MILEA facility at LIP; details on the measurements are described by Christl et al. (2023). The measured ratios were normalized to the in-house ZUTRI ETH standard with a nominal isotopic ratio of 4055 ± 203 × 10−12 for 236U/238U and 3170 ± 830 × 10−12 for 233U/238U (Christl et al., 2013). The concentration of 236U in the seawater samples was calculated using the isotopic ratios and the known amount of 233U added to the sample. To correct for 236U, the 236U/233U ratio was measured in chemistry blanks prepared with deionized water that was treated following the same procedure as that for the samples. The blanks resulted in atom ratios of 5.75 ± 1.3 × 105 (n = 2) and 1.04 ± 0.08 × 104 (n = 3) for A25 and AR7W, respectively.
2.4 Data interpretation
Prior to the tracer analysis, water masses were defined using potential temperature and salinity following the work of García-Ibáñez et al. (2018) and Liu and Tanhua (2021).
The mixing of different water masses was studied using a binary mixing model based on two radionuclide tracers, namely, 129I and 236U. The key components of this 2D model are the endmembers, which represent the tracer signatures of specific water masses. These signatures are measured either at the water mass’ source region or at a representative location where data is available. The endmembers create the boundary conditions that span between water masses with high tracer concentrations to others with low concentrations. All samples should fall within these boundary conditions, and the samples’ tracer concentration is the result of the mixing between two different endmembers. This model has been previously described by Dale et al. (2024). In this work, we have extended the tracer space to other water masses found in the study region. The endmember locations are indicated by the triangles in Figure 1, and their location in the tracer space of the binary mixing model is shown in Figure 3. The error bars of each endmember represent the variability in the tracer concentrations within one water mass. An overview of the mean, minimum, and maximum tracer concentrations as well as the corresponding hydrographic properties is given in Supplementary Table S1. The endmember tracer values adopted here were mostly assigned in earlier works (Castrillejo et al., 2022, 2018; Wefing et al., 2019; Dale et al., 2024).
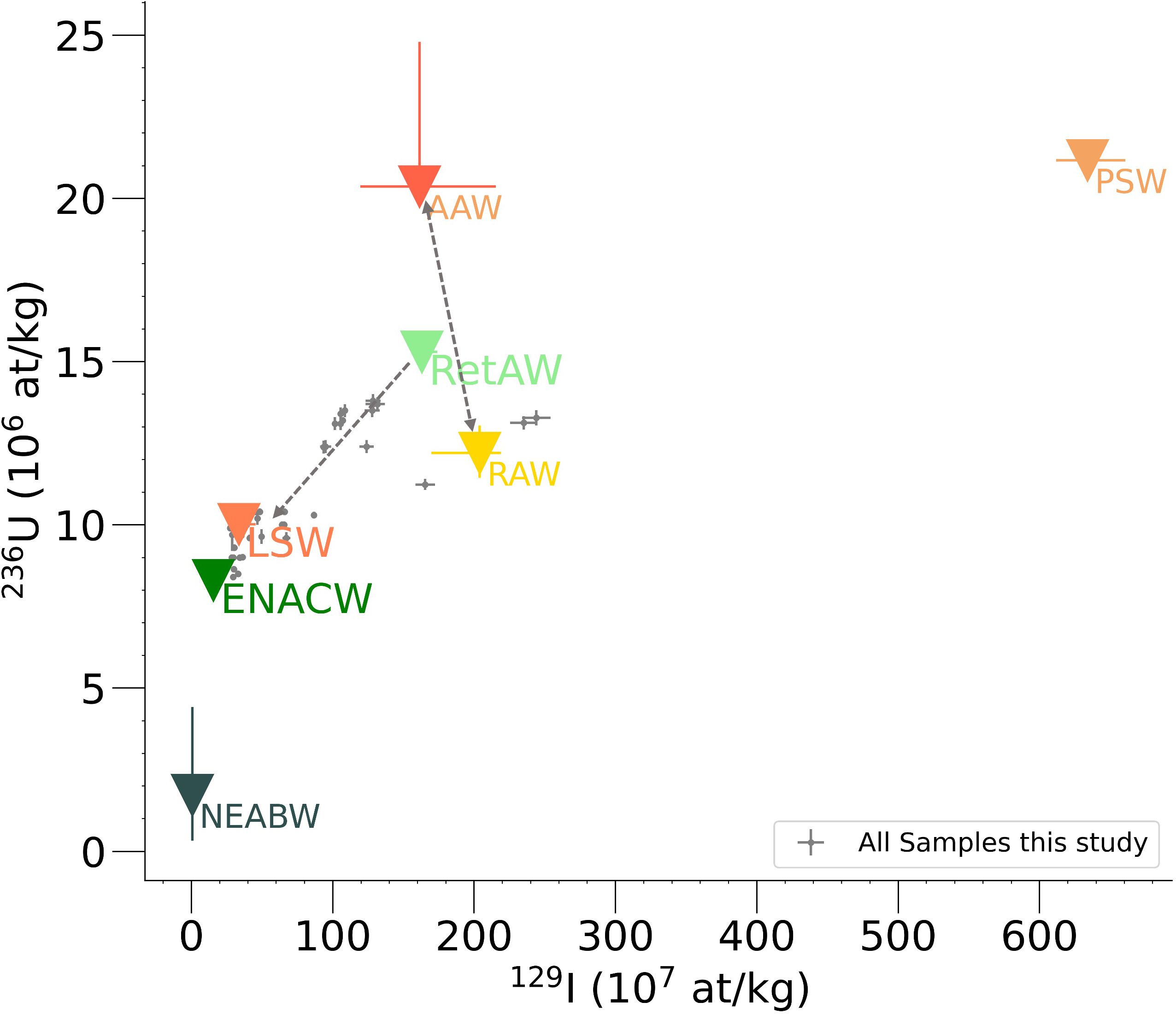
Figure 3. Overview of tracer data and water mass endmembers in a binary mixing model of 129I–236U. This study’s samples are represented by gray symbols, while endmembers are shown in color with error bars indicating their maximum spread. Gray arrows illustrate the formation of RetAW by mixing AAW with RAW and a further dilution of IW (Dale et al., 2024), not shown here, which would be located at the ENACW endmember. The sampling year and references for the endmembers are provided in Supplementary Table S1, and their sampling location can be found in Figure 1. The LSW endmember is derived from samples in this study; its sampling location is indicated in Figure 4. AAW, Arctic Atlantic Water; ENACW, Eastern North Atlantic Central Water; LSW, Labrador Sea Water; NEABW, North East Atlantic Bottom Water; PSW, Polar Surface Water; RAW, Recirculating Atlantic Water; RetAW, Return Atlantic Water.
In the SPNA, the endmembers (Figure 3) with the highest tracer concentrations are the water masses with the direct influence of European nuclear reprocessing plants (Casacuberta and Smith, 2023). These waters, namely, Arctic Atlantic Water (AAW, red triangle, Figure 3) and polar surface water (PSW, orange triangle), recirculated in the Arctic Ocean and entered the SPNA as the East Greenland Current (EGC) was exiting the Arctic Ocean via Fram Strait and passing the Nordic Seas (Holliday et al., 2007b; Vage et al., 2013). A third water mass, the Return Atlantic Water (RetAW, light green triangles), is formed by the mixing of Arctic Atlantic Water (AAW) with Recirculating Atlantic Water (RAW) (Dale et al., 2024) and RAC (Wefing et al., 2022, 2019) which did not enter into the Arctic Ocean (Dale et al., 2024; Wefing et al., 2019, 2022; De Steur et al., 2014; Olsson et al., 2005; Rudels, 2002). In contrast, ENACW (teal triangle) provides the best estimate for waters coming from upstream locations relative to nuclear reprocessing plants and thus carries relatively low concentrations of both 129I and 236U mostly derived from nuclear weapon tests (Castrillejo et al., 2018).
The endmembers of AAW and PSW correspond to values observed in Fram Strait in 2016 (Wefing et al., 2019). Although Fram Strait was sampled in 2018 and 2019, we decided to choose the 2016 data because of its stronger influence on the Arctic Atlantic outflowing water (Wefing et al., 2022), and it already proved to be a suitable endmember for the upstream water mass formation (Dale et al., 2024). The RetAW is represented by values obtained from the GEOTRACES Metal Gate cruise and monitoring program around Iceland in 2021 and represents the best estimate of the high tracer concentration in water masses originating in the Nordic Seas (Dale et al., 2024). The ENACW and NEABW endmembers were classified after Castrillejo et al. (2018). An average of all tracer measurements was calculated for NEABW. On the other hand, measurements at their GEOVIDE Station 26 (50°16.67′ N, 22°36.28′ W) were found to best represent the reprocessing-free waters carried by the NAC.
The water mass fraction is calculated by dividing the distance between the sample and one endmember by the total distance between the two endmembers. For each water mass, this model considers the mixing between two endmembers only, which is one of its main limitations. However, the tracer signature in each water mass is only affected by mixing with tracer-labeled water masses and is not subject to hydrographic changes. This allows one to quantify mixing in a 2D model between tracer-rich Arctic-origin water and tracer-poor water of Atlantic origin. Currently, the model reaches its limitations, where the overall tracer concentration is low (129I: 20–50 × 107 at/kg; 236U: 8.5–10.5×106 at/kg) and the tracer input happens via multiple vertical and lateral mixing processes and sources. This might be addressed by including the tracers in an optimum multiparameter analysis (Poole and Tomczak, 1999), which is not the scope of this manuscript but could be achieved in future studies as more data become available.
3 Results
All radionuclide data are reported in atoms per kilogram (at/kg) along with metadata and hydrographic variables (T, S) in Supplementary Table S2, while the acronyms are listed in Appendix A. The concentrations of 129I with overlaid salinity and 236U concentrations are presented in Figure 4. The distributions of 129I and 236U are related to the water masses of the region following the classifications and naming from García-Ibáñez et al. (2018) and Liu and Tanhua (2021) and earlier work on these tracers in the SPNA (Castrillejo et al., 2022, 2018).
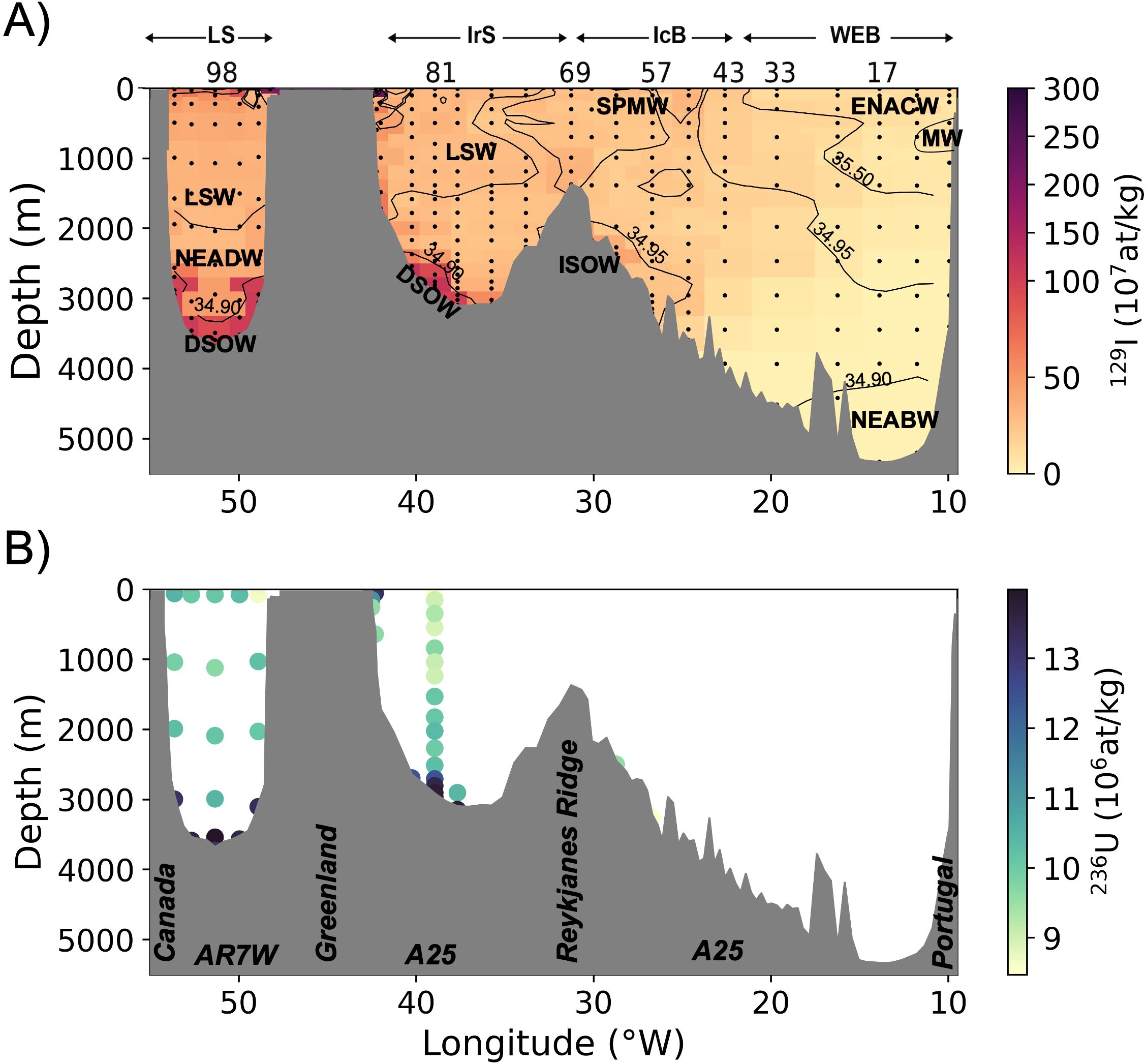
Figure 4. Zonal distribution of (A) 129I and (B) 236U concentrations along A25 and AR7W, respectively, in 2021 and 2020. Isohalines (black lines) overlay the interpolated 129I concentrations to represent the water mass distribution following Daniault et al. (2016); Liu and Tanhua (2021), and Yashayaev (2007). The 236U concentrations, fewer in number, are represented as colored dots.
The geographical distribution of 129I (Figure 4A) shows increasing concentrations from east to west. The lowest 129I concentrations, ≈ 0.2 × 107 at/kg, were found below 3000 m in the WEB. The highest 129I concentrations, up to 235 × 107 at/kg, were observed in surface waters in the Labrador and Irminger seas. Intermediate concentrations, between 30 and 40 × 107 at/kg, were found at intermediate depths between the Iceland Basin and the Labrador Sea. The fewer 236U data (Figure 4B) generally followed the distribution of 129I. As for 129I, 236U was highest at shallow depths in the vicinity of Greenland and near-bottom depths of the Labrador Sea and Irminger Sea (>13 × 106 at/kg, >2700 m). The average concentrations of 236U (9 to 10 × 106 at/kg) corresponded to intermediate depths in these basins, while lower concentrations (<9 × 106 at/kg) filled the bottom depths east of Reykjanes Ridge.
In the WEB, depths greater than 3000 m were occupied by cold and relatively fresh (Tpot< 2.6°C, S < 34.90,129I: <1 × 107 at/kg) northward-flowing NEABW. At about 1000 m depth was the core of the saline and warm MOW (Tpot≈ 11.7°C, S > 36.00, 129I: 5–7 × 107 at/kg), more concentrated near Portugal. The shallower depth layer was filled by the warmer ENACW with elevated 129I (Tpot> 12°C, S: 35.4–35.7, 129I: 5-14 × 107 at/kg).
The upper 700 m in the Iceland Basin was occupied by comparable fresher and colder Subpolar Mode Water (SPMW, Tpot: 7.2°C, S: 35.07) and SAIW (Tpot: 6.0°C, S: 34.7) that contained 129I concentrations between 20 × 107 and 35 × 107 at/kg. Below SPMWs was LSW (Tpot: ≈3°C, 129I: 30 × 107 at/kg) with comparable salinity but colder temperature. Confined to the eastern flank of the Reykjanes Ridge was ISOW (Tpot ≈ 3.5°C, S ≈ 34.95, 129I: 41 × 107 at/kg), while the NEADW (Tpot ≈ 2.9°C, S ≈ 34.92, 129I: 27–48 × 107 at/kg) was occupying a similar depth in the Irminger and Labrador seas.
In the Labrador and Irminger seas, near-bottom depths were filled by the very dense, cold, and fresher DSOW (Tpot<1.5°C, S < 34.92) that contained high 129I concentrations between 108.6 ± 1.3 × 107 at/kg and 131.6 ± 5.4 × 107 at/kg. The remaining water column structure in the Labrador Sea largely resembled that of the Irminger Sea. The cold and freshest water mass in both basins, the Polar Surface Water (S < 34.92), was carried along the Greenland and Canada shelves by the Western Boundary Current as part of the EGC, the WGC, and the Labrador Current. The highest 129I concentration up to 235 × 107 at/kg, measured in this study, characterized the EGC and WGC at the Greenlandic shelf. The Labrador Current also showed elevated 129I (95 × 106 at/kg), although not as high as in the EGC. The surface waters in the middle of the Labrador Sea showed a heterogeneous distribution with 129I in the range of 30–60 × 107 at/kg.
The 236U distribution was detailed for the Labrador Sea but limited to one full-depth station in the central Irminger Sea and a few other samples within the Irminger Sea and east of Reykjanes Ridge. The 236U distribution in the Labrador and Irminger seas (Figure 4B) was similar to 129I but displayed a narrower range of concentrations: 8.4 to 14 × 106 at/kg. The highest 236U concentrations were located in the EGC (13.1 ± 0.2 × 106 at/kg) and DSOW (13.8 ± 0.2 × 106 at/kg). Then declined to 10.4 × 106 at/kg in the NEADW and LSW and to 8.6 ± 0.2 × 106 at/kg in the SPMW.
4 Discussion
The distribution of 129I and 236U in the SPNA varies over time and space due to two primary factors: discharges from nuclear reprocessing plants and changes in ocean circulation. Nuclear reprocessing plants have been releasing 129I and 236U since the 1960s, but their contributions to the North Sea have fluctuated. 236U discharges peaked in the 1980s, while 129I discharges increased exponentially between 1990 and 2000 (Figure 2) (Casacuberta and Smith, 2023). The circulation and mixing of water masses transport distinct tracer signals based on their origin and mixing processes. If ocean circulation is unchanging, the circulation should reflect the dynamics of nuclear reprocessing plant discharges. However, this relationship may be disrupted if circulation patterns and, consequently, mixing change.
To disentangle the two drivers of 129I and 236U concentrations in the SPNA, firstly, we relate the distribution of both tracers to the circulation patterns in 2020/2021. Secondly, we examine the potential of using 129I and 236U in a binary mixing model that allows us to understand water mass provenance and mixing in the SPNA.
4.1 Tracer distribution in relation to circulation patterns
To relate the tracer distribution to the circulation patterns, both 129I and 236U are plotted in iso-surface maps at the surface (upper 50 m), intermediate (1,500 m/1,000 m), and bottom depths (deepest sample). Due to scarcity of 236U, the discussion mainly focuses on the 129I distribution. At the surface (Figures 5A, B), the highest concentrations of both tracers are observed in the East/West Greenland currents both in the Irminger Sea (129I: 235.2 ± 9.8 × 107 at/kg) and Labrador Sea (129I: 232.0 ± 2.8 × 107 at/kg). These high concentrations relate to the ones observed at East Greenland Current (EGC) both in Fram Strait (129I: 645 ± 2.1 × 107 at/kg) and downstream at Denmark Strait (129I: 432 ± 21.7 ×107 at/kg) (Wefing et al., 2019; Dale et al., 2024), indicating the advectiveness of this current from its source region in Fram Strait down to the tip of Greenland. The 129I content of the Labrador Current (95 × 107 at/kg) is about half of the concentrations observed in the EGC (235 × 107 at/kg), most probably being related to a mixing of the West Greenland Current (WGC) with 129I-poor Arctic waters carried by the Baffin Iceland Current. These waters enter the Labrador Sea through the Arctic–Canadian Archipelago and mix at the Davis Strait (Wefing et al., 2022; Pacini and Pickart, 2022; Chamizo et al., 2022). The heterogeneous tracer distribution in the central Labrador Sea (Figure 5A) might reflect the eddy transport of tracer-rich polar surface water (PSW) from the boundary current to the open ocean (Holliday et al., 2020; Lilly et al., 2003; Gou et al., 2022; Holliday et al., 2007b). Moving to the east of the A25 line, surface tracer concentrations progressively decrease to 10 × 107 at/kg.
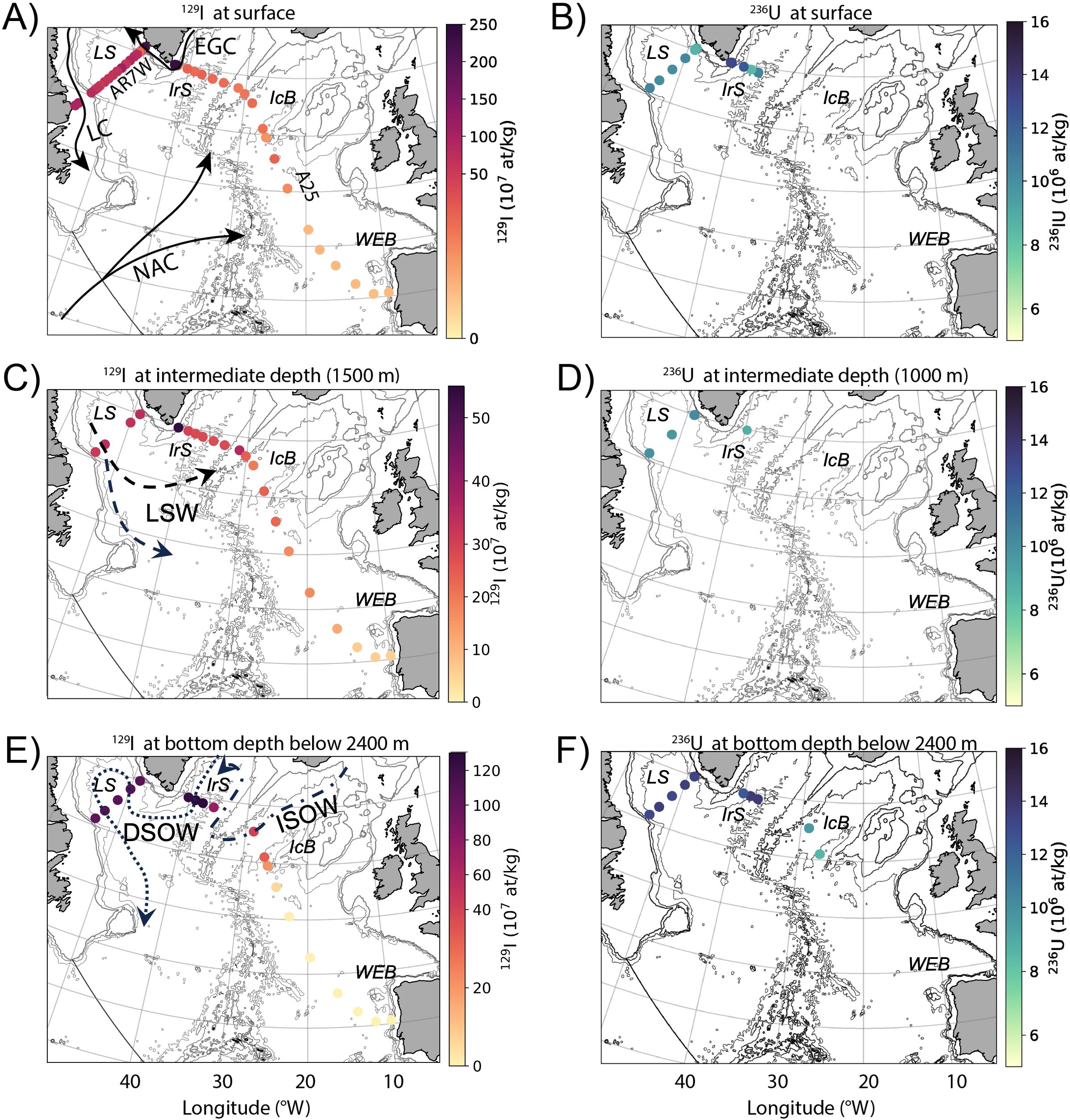
Figure 5. Isosurface distributions of 129I and 236U concentrations at (A, B) the surface, (C) 1,500 m for 129I, (D) 1,000 m for 236U, and (E, F) bottom depth below 2,400 m.
This highlights the subarctic front at 22.5°W with a sudden drop (station 50, 129I: 34.3 ± 1.4 × 107 at/kg to station 43, 129I: 23.1 ± 1 × 107 at/kg) in the tracer concentration. The lowest values (<2.5 × 107 at/kg) at the surface never reach values as low as the ones expected from global fallout (Edmonds et al., 1998; Castrillejo et al., 2018). This is in agreement with previous studies that suggest the formation of Eastern North Atlantic Central Water (ENACW) as a result of mixing between Subpolar Mode Water (SPWM, tracer-labeled) and tracer-poor, nuclear reprocessing plant-free tropical waters transported by the North Atlantic Current (NAC) (Liu and Tanhua, 2021; Castrillejo et al., 2022; Daniault et al., 2016). This interpretation differs from He et al. (2013) and Castrillejo et al. (2017) who suggested a direct influence of the nuclear reprocessing plants by waters approaching from France and the UK toward the coast of Portugal and from the MW which still carries the imprint from the closed nuclear reprocessing plant of Marcoule in Southern France. However, the gradual decrease in surface concentration from west to east might favor the influence of SPMW on ENACW.
At intermediate depths, the highest concentrations of both tracers were observed at the Labrador and Irminger seas (Figures 5C, D). One of the hypotheses is that the tracers remain at this depth due to winter convection and/or re-circulation within the subpolar gyre. This finding is consistent with previous studies tracking the re-circulation and pathways of Labrador Sea Water (LSW) using hydrographic data, ARGO floats, CFCs, and 129I (Holliday et al., 2007a, 2009; Rhein et al., 2011; Castrillejo et al., 2022). Another explanation is that tracer concentrations at intermediate depths are coming from upstream locations, e.g., at mid-depths at Denmark Strait (Rudels, 2002), something that will be further discussed with the binary-mixing model in Section 4.3. Toward the east, the concentrations gradually decrease, showing the influence of LSW at intermediate waters and at least as far as 32.5°W. LSW are mostly formed in the middle of the Labrador and Irminger seas, thus having 129I and 236U concentrations that are further diluted from the ones observed at the surface of the Labrador Sea (129I < 50 × 107 at/kg).
Below 2400 m (Figures 5E, F), a clear separation is observed between tracer-rich waters west and along the flanks of the Reykjanes Ridge and tracer-poor waters at the eastern part of the transect. In agreement with previous studies (Smith, 2005; Castrillejo et al., 2022), the high tracer concentrations are carried by the two overflow waters: Denmark Strait Overflow Water (DSOW) and Iceland Scotland Overflow Water (ISOW). These two water masses overflowing through the Greenland–Iceland and Iceland–Scotland passages pick the tracer signal from shallower depths and bring it to the abyssal layers (Dale et al., 2024). On the contrary, the deep North East Atlantic Bottom Water (NEABW) from the south carries the natural 129I signal, thus representing very old waters that have not yet seen the anthropogenic influence of neither weapon test nor reprocessing plant discharges.
4.2 Pseudo-steady-state of the tracers in the study area
The discharges of both 129I and 236U into the marine environment have varied over time (Figure 2), allowing for their use as transient tracers, thereby requiring a non-steady state approach (Casacuberta and Smith, 2023; Payne et al., 2024). In this study, however, we follow the approach described in Dale et al. (2024) where the tracers are assumed to be not significantly changing during the period from 2016 till the present. The study of Dale et al. (2024) proves that while the tracer signature of the Atlantic water entering the Arctic Ocean is changing over time, the rate of change of the inputs is slow enough that one can compare the measurements of water masses relatively proximal in the ocean circulatory system. Small changes in the inputs and upstream mixing regimes are represented by error bars of the endmembers (Figure 3), showing the maximal spread of tracer concentration within one source water mass. In comparison, the error bars attached to the samples of this study represent the analytical error which is significantly smaller. Here the validity of this assumption is further addressed by looking at the time variability of tracer concentrations in water masses sampled in previous years. Figure 6 shows the 129I vs. 236U concentrations from 2014 (GEOVIDE, light gray) (Castrillejo et al., 2018), 2018 (OVIDE, dark gray) (Castrillejo et al., 2022), and 2020/2021 (this study, blue), where deep and intermediate water masses cluster at two different domains within the 129I –236U tracer space. The cluster that plots on the top right represents the core of DSOW, while the cluster plotting at the bottom left represents the intermediate waters, both in the Irminger and Labrador seas. When looking at the DSOW cluster of the Irminger Sea (blue diamonds), there are slight differences in 129I and236U concentrations between the years 2018 and 2021 (no data for 2014), with more recent data located at higher 129I. The observed increase in 129I corresponded well with further releases in 129I after 1999 (Figure 2), with transit times of 16–18 years from the North Sea to the deep Irminger and Labrador seas (Castrillejo et al., 2018) and about 1 year from Denmark Strait sill to Cape Farewell (Xu et al., 2015). The DSOW cluster sampled in the Labrador Sea (blue crosses) overlaps the DSOW sampled in the Irminger Sea in 2018 (gray diamonds) which showed the advective character and a transit time of about 1 year, which is in agreement with earlier studies (Xu et al., 2015; Smith, 2005). The concentrations of both tracers could indeed have been different for both years considering the discharge history. However, the DSOW samples from 2018 and 2021 in Figure 6 remain very clustered and detached from the rest considering the strong mixing in the Nordic Seas, which points toward a diffusive formation and thus a dilution of the tracer signal along the formation pathway. This proves the robustness of the binary mixing model (Figure 3) and validates the assumption of a steady state.
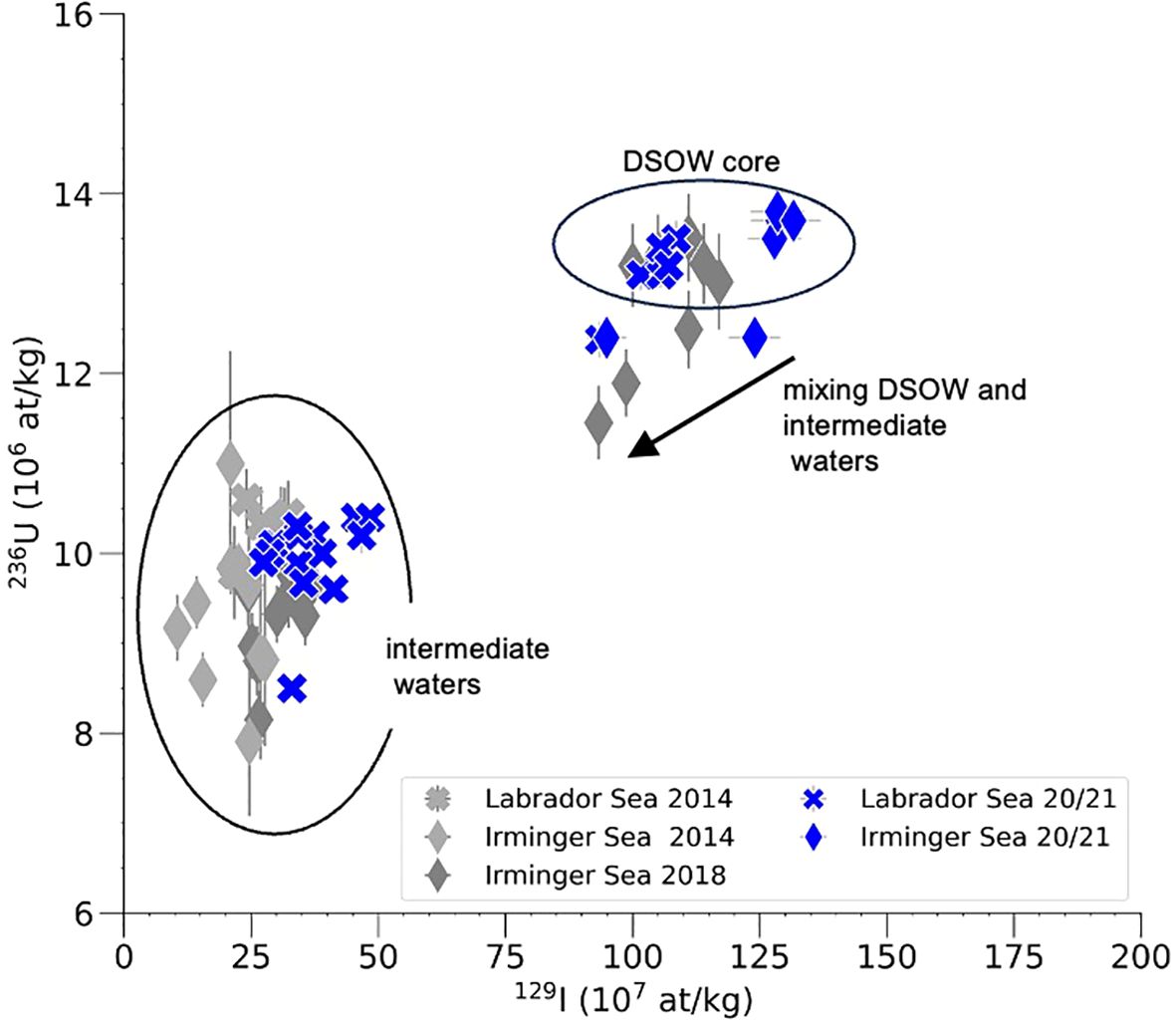
Figure 6. Historic evolution of the tracers in the 129I–236U binary mixing model between 2014 (light gray), 2018 (dark gray), and 2020/2021 (blue). The Labrador Sea was not sampled in 2018.
4.3 Water mass provenance and mixing
To study the provenance and mixing of water masses, we utilized the binary mixing model described in Section 2.4. Since this model relies on the concentration of both 129I and 236U, the discussion is limited to data that includes both tracers. Water masses were assigned using hydrographic data, as described in Section 3 . Results and the Supplementary Material.
The surface samples (Figure 7A, blue diamonds) with the highest concentrations of both 129I and 236U are located in the Irminger Sea and sampled within the EGC. They are a further dilution of station MG17 (Dale et al., 2024) sampled north of Denmark Strait and nicely show the continuation of the EGC along the Greenlandic Coast. The samples of this study were taken during the transit from the central Irminger Basin to the coast of Greenland. Thus, samples that plot closer to the PSW endmember correspond to the ones that best represent the core of the EGC. These waters preserve a significant fraction (i.e., 29%–32%) of the Polar Surface Water exiting the Arctic Ocean through the Fram Strait. The samples that plot down the mixing line indicate the mixing with the low-tracer northward-flowing ENACW. This work therefore corroborates previous studies about the connection and evolution of the waters exiting the Arctic Ocean and being transported to lower latitudes through the EGC (Holliday et al., 2007b; Sutherland et al., 2009). However, while other studies relied on the use of hydrographic parameters and models only (Holliday et al., 2007b; Sutherland et al., 2009), this is the first work that uses radionuclide tracers as a new tool to understand the contribution of PSW to the East and West Greenland currents and further to the formation of LSW.
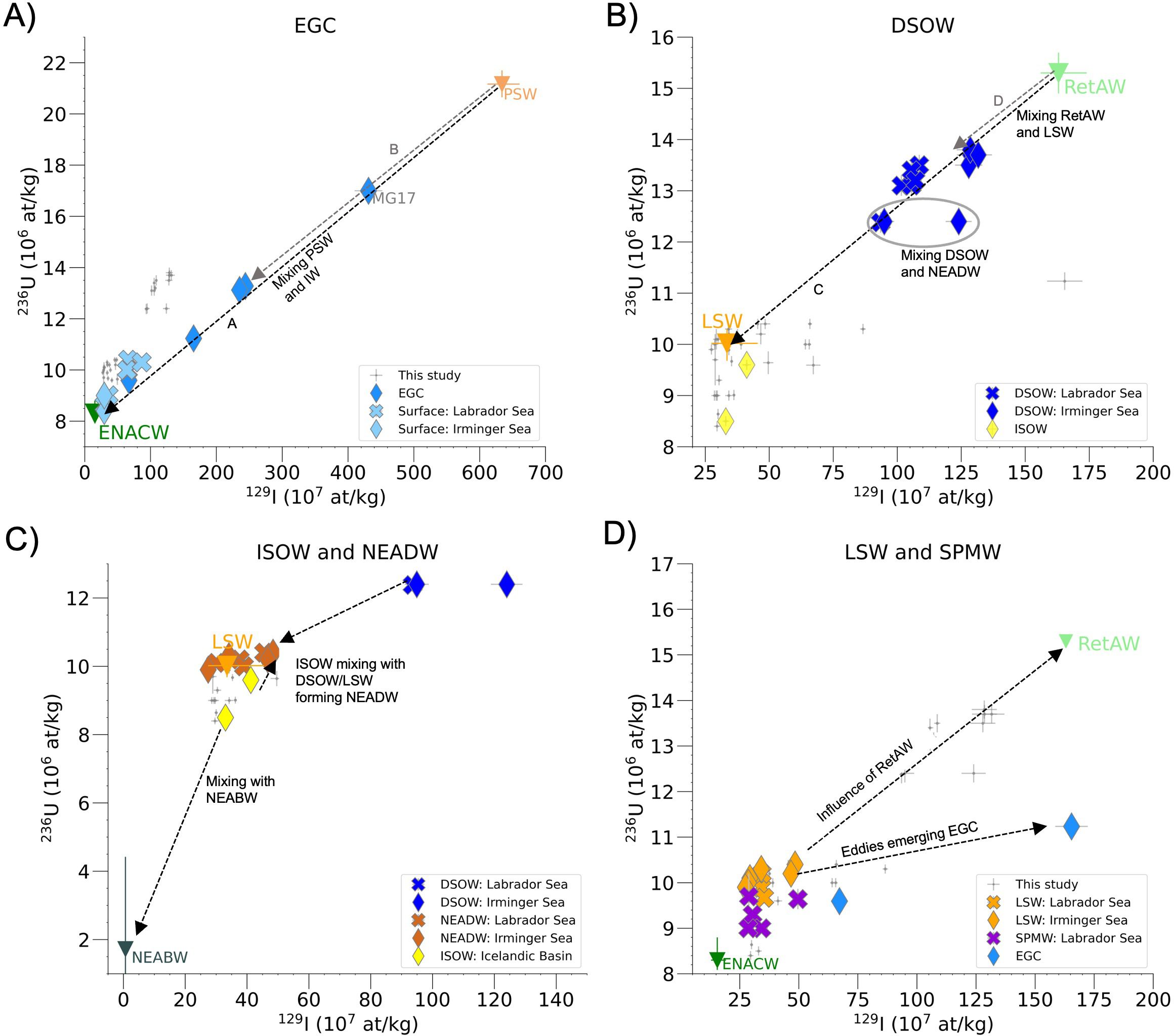
Figure 7. Binary mixing model of 129I–236U with endmembers as previously described in Figure 3. Gray symbols represent all study samples, while different colors highlight different water masses. (A) EGC and surface waters, with mixing calculated as: PSW (fraction) = length A/B, (B) DSOW and its formation by dilution of RetAW with LSW as calculated by fraction RetAW = length C/D, (C) ISOW and NEADW, and (D) LSW and SPMW. Triangles represent endmembers as described in Supplementary Table S1 and Figure 3, while the dotted lines indicate mixing between different water masses. All acronyms are detailed in Appendix A.
The composition of the DSOW (Figure 7B, dark blue diamonds and crosses) in the Irminger and Labrador seas is mainly a mixture of Return Atlantic Water (RetAW) with LSW and North East Atlantic Deep Water (NEADW). Small fractions of strongly tracer-labeled waters such as PSW (orange triangle Figure 3, Supplementary Table S1) and AAW (red triangle Figure 3) may contribute to the formation of DSOW (Rudels, 2002; Dale et al., 2024; Tanhua et al., 2005; Tanhua, 2005; Lin et al., 2020), which could lead to the two observed DSOW clusters. The cluster plotting slightly left of the two endmembers’ mixing line and with lower 129I concentrations represents samples in the Labrador Sea with a fraction of RetAW of 20%–24%. The second cluster composed of samples in the Irminger Sea plots on top of the RetAW-LSW/NEADW mixing line with a fraction of RetAW of 27%–35%, which is in agreement with the 33%–43% reported by Dale et al. (2024). A stronger contribution of AAW to the formation of RetAW might already lead to an increase of 129I with respect to the 236U concentrations. A dilution of DSOW between the Irminger Sea and Labrador Sea is less likely (see Figure 6B) since the signal of DSOW found in 2018 in Irminger Sea is well conserved in the Labrador Sea signal in 2020, which agrees with a transit time of about 1 year (Xu et al., 2015; Tanhua, 2005). Another feature observed here is that once we move away from the DSOW core, the tracers show a strong influence of intermediate water masses such as NEADW/ISOW, which is in agreement with the observations of Dale et al. (2024) (Figure 7B, gray-shadowed circle).
At the southern part of the Reykjanes Ridge, ISOW (Figure 7C, yellow diamonds) is already entrained by 60% of LSW (Dale et al., 2024; Fogelqvist et al., 2003) and located at lower tracer concentrations. Johns et al. (2021) described two branches of ISOW on the eastern flank of Reykjanes Ridge that are visible in the tracer space: the main branch has higher 236U and plots close to the NEADW cluster, representing the main influence of LSW and SPMW (Furey et al., 2024). The second branch might have a stronger influence of NEABW and therefore lower 236U concentration, which is in agreement with the water mass analyzed by García-Ibáñez et al. (2015). However, since the data of the dual tracer pair in this region is limited, a further interpretation of the spatial distribution along the Reykjanes Ridge is not yet possible.
The evolution of ISOW to NEADW (brown crosses) from the Irminger Sea to the Labrador Sea can be followed by increasing 236U and a slight increase in 129I, which could be attributed to a further entrainment of LSW and mixing with DSOW (Dale et al., 2024; García-Ibáñez et al., 2015; Furey et al., 2024; Smethie et al., 2000; Stramma et al., 2004). As the mixing with DSOW seems to push the ISOW to a slightly higher 236U, the NEADW, in turn, leads to a spread of DSOW to lower 236U at the mixing interfaces.
Finally, LSW is generally very diluted with regard to both tracers and thus located at the lower end of the tracer space (Figure 7D). The LSW (orange diamonds and crosses) might have multiple tracer sources, as it is formed via vertical winter time convection, lateral mixing between intermediate waters in Labrador and Irminger seas, and advection of DSOW into bottom waters (Yashayaev, 2024; Xu et al., 2015; Dale et al., 2024). A tracer source to the surface are eddies emerging from the boundary currents (EGC/WGC, LC) to the central Labrador Sea (Zunino et al., 2017), carrying especially high 129I concentration. While eddies may contribute to the observed tracer concentrations during winter convection, they cannot be the sole source, as the tracer signature within the EGC is characterized by high levels of 129I but relatively low levels of 236U. The RetAW introduced to the LSW via passing over the Eastern Greenland continental shelf (Rudels et al., 1999; Pickart, 1992; Zou et al., 2024) and mixing across density surfaces seems to be another tracer source, considering the higher 236U concentration in LSW located at Irminger Basin in comparison to the LSW located at Labrador Sea. This further supports the hypothesis of Dale et al. (2024) of a “contamination” of the intermediate waters at the subpolar gyre with elevated 236U due to entrainment of RetAW. Furthermore, the recirculation of LSW within the subpolar gyre might cause a smoothing of the tracer signal and preserve higher 236U concentrations in older waters (Lavender et al., 2005; Lozier et al., 2013). To further evaluate the tracer sources to the LSW, tracer pair data of the WGC, LC, and from the Davis Strait would be necessary (Wefing et al., 2021; Chamizo et al., 2022; Colombo et al., 2019; Zhang et al., 2021a, b).
Finally, the SPMW (purple crosses) plots at comparable low 236U concentrations. This suggests the strong influence of eddies emerging from the EGC (Holliday et al., 2009).
5 Conclusion
This study aimed to constrain the origin and mixing of water masses in the subpolar North Atlantic by combining two artificial radionuclides, 129I and 236U. To that end, we used new data collected in the A25 (OVIDE) and AR7W (Labrador Sea) lines in 2020/2021 and used a new dual tracer approach to infer water mass provenance and mixing. The results for both radionuclides tracers, 129I and 236U, were consistent with tracer distributions reported in earlier studies but highlighted the contrast between high-tracer waters influenced by nuclear reprocessing plants and low-tracer waters originating from southern latitudes. Using the tracer pair in a binary mixing model, the PSW contributes 29–32% to the EGC at Cape Farewell. With 29%–35%, RetAW was found to be a main contributor to DSOW in the Irminger and Labrador seas.
The binary mixing model reached its limitation in the identification of the water mass composition of LSW, SPMW, and ISOW because of the availability of suitable endmembers. A more detailed sampling of possible contributors was conducted in the Nordic Seas and Baffin Bay (2022). This will help constrain better endmembers to investigate the origin and mixing of these water masses. Overall, the study shows that 129I and 236U are suitable to address the provenance and mixing of key components of the SPNA circulation, which contributes to a better understanding of circulation patterns, pathways and mixing of water masses, and their effect on the evolution of the AMOC. While this study also points to the limitations of this method, it underscores the potential of 129I and 236U as valuable tracers to validate and improve ocean circulation models, particularly in the complex mixing zones of the North Atlantic.
Data availability statement
The original datasets for this study can be found in the article/Supplementary Material and radioisotope data are available at MARIS (Marine Radioactivity Information System): https://maris.iaea.org/datasets. The CTD-Oxygen data from the OVIDE cruise are reported in Le Bihan et al. (2023).
Author contributions
LL: Writing – original draft, Visualization, Validation, Methodology, Formal analysis, Data curation. MCa: Writing – review & editing, Visualization, Validation, Supervision, Data curation. JS: Writing – review & editing. MCh: Writing – review & editing, Resources, Data curation. CV: Writing – review & editing, Data curation. AV: Data curation, Validation, Investigation, Funding acquisition, Resources, Writing – review & editing. PL: Data curation, Validation, Investigation, Funding acquisition, Resources, Writing – review & editing. NC: Writing – review & editing, Supervision, Resources, Project administration, Funding acquisition, Conceptualization.
Funding
The author(s) declare that financial support was received for the research, authorship, and/or publication of this article. This work was mainly funded by the European Research Council grant TITANICA awarded to NC (Grant agreement 101001451). Additional funds came from the Swiss National Science Foundation (Grant number PR00P2_193091) awarded to NC and the ETH Career SEED Grant (SEED-06 19-2) awarded to MCa as well as the consortium partners of the ETH Zurich Laboratory of Ion Beam Physics (EAWAG, EMPA, and PSI). Statement: Open access funding is provided by Swiss National Science Foundation (SNSF). This work has been supported by BOCATS2 (PID2019-104279GB-C21) project funded by MICIU/AEI/10.13039/501100011033.
Acknowledgments
Special acknowledgments are extended to the chief scientist of the AR7W Cruise, Mark Ringuette. Further acknowledged are the captains and the crew of the RV Amand and RV Sarmiento de Gamboa. The scientists involved in the sampling are deeply acknowledged. Kayley Kündig is thanked for her contributions to ETH-based laboratories. We thank Xiaobiao Xu and Xabier Davila for their comments that contributed to a substantial improvement of this paper.
Conflict of interest
The authors declare that the research was conducted in the absence of any commercial or financial relationships that could be construed as a potential conflict of interest.
Publisher’s note
All claims expressed in this article are solely those of the authors and do not necessarily represent those of their affiliated organizations, or those of the publisher, the editors and the reviewers. Any product that may be evaluated in this article, or claim that may be made by its manufacturer, is not guaranteed or endorsed by the publisher.
Supplementary material
The Supplementary Material for this article can be found online at: https://www.frontiersin.org/articles/10.3389/fmars.2024.1470675/full#supplementary-material
Supplementary Table 1 | Hydrographic and tracer data for GEOVIDE.
Supplementary Table 2 | Endmember concentration and hydrographic water mass properties.
Supplementary Table 3 | Hydrographic and tracer data for A25 and AR7W.
References
Aldahan A., Alfimov V., Possnert G. (2007). 129 I anthropogenic budget: Major sources and sinks. Appl. Geochemistry 22, 606–618. doi: 10.1016/j.apgeochem.2006.12.006
Alfimov V. (2004). Tracing water masses with 129 I in the western Nordic Seas in early spring 2002. Geophysical Res. Lett. 31, L19305. doi: 10.1029/2004GL020863
Bower A., Lozier S., Biastoch A., Drouin K., Foukal N., Furey H., et al. (2019). Lagrangian views of the pathways of the atlantic meridional overturning circulation. J. Geophysical Research: Oceans 124, 5313–5335. doi: 10.1029/2019JC015014
Bower A. S., Lozier M. S., Gary S. F., Böning C. W. (2009). Interior pathways of the North Atlantic meridional overturning circulation. Nature 459, 243–247. doi: 10.1038/nature07979
Casacuberta N., Christl M., Vockenhuber C., Wefing A.-M., Wacker L., Masqué P., et al. (2018). Tracing the three atlantic branches entering the arctic ocean with 129 I and 236 U. J. Geophysical Research: Oceans 123, 6909–6921. doi: 10.1029/2018JC014168
Casacuberta N., Masqué P., Henderson G., Rutgers van-der Loeff M., Bauch D., Vockenhuber C., et al. (2016). First 236 U data from the Arctic Ocean and use of 236 U/238 U and 129 I/236 U as a new dual tracer. Earth Planetary Sci. Lett. 440, 127–134. doi: 10.1016/j.epsl.2016.02.020
Casacuberta N., Smith J. N. (2023). Nuclear reprocessing tracers illuminate flow features and connectivity between the arctic and subpolar north atlantic oceans. Annu. Rev. Mar. Sci. 15, 203–221. doi: 10.1146/annurev-marine-032122-112413
Castrillejo M., Casacuberta N., Christl M., Garcia-Orellana J., Vockenhuber C., Synal H.-A., et al. (2017). Anthropogenic 236 U and 129 I in the Mediterranean Sea: First comprehensive distribution and constrain of their sources. Sci. Total Environ. 593-594, 745–759. doi: 10.1016/j.scitotenv.2017.03.201
Castrillejo M., Casacuberta N., Christl M., Vockenhuber C., Synal H.-A., García-Ibáñez M. I., et al. (2018). Tracing water masses with \textbackslashchemˆ129I and \textbackslashchemˆ236U in the subpolar North Atlantic along the GEOTRACES GA01 section. Biogeosciences 15, 5545–5564. doi: 10.5194/bg-15-5545-2018
Castrillejo M., Casacuberta N., Vockenhuber C., Lherminier P. (2022). Rapidly increasing artificial iodine highlights pathways of Iceland-scotland overflow water and labrador sea water. Front. Mar. Sci. 9. doi: 10.3389/fmars.2022.897729
Chamizo E., Christl M., López-Lora M., Casacuberta N., Wefing A., Kenna T. (2022). The potential of 233 U/ 236 U as a water mass tracer in the arctic ocean. C. J. Geophysical Research: Oceans 127, e2021JC017790. doi: 10.1029/2021JC017790
Christl M., Casacuberta N., Lachner J., Maxeiner S., Vockenhuber C., Synal H.-A., et al. (2015a). Status of 236 U analyses at ETH Zurich and the distribution of 236 U and 129 I in the North Sea in 2009. Nucl. Instruments Methods Phys. Res. Section B: Beam Interact. Materials Atoms 361, 510–516. doi: 10.1016/j.nimb.2015.01.005
Christl M., Casacuberta N., Vockenhuber C., Elsässer C., Bailly du Bois P., Herrmann J., et al. (2015b). Reconstruction of the 236 U input function for the northeast atlantic ocean: Implications for 129 I/ 236 U and 236 U/ 238 U-based tracer ages. J. Geophysical Research: Oceans 120, 7282–7299. doi: 10.1002/2015JC011116
Christl M., Gautschi P., Maxeiner S., Müller A. M., Vockenhuber C., Synal H.-A. (2023). 236 U analyses with the ETH Zurich MILEA prototype system. Nucl. Instruments Methods Phys. Res. Section B: Beam Interact. Materials Atoms 534, 61–71. doi: 10.1016/j.nimb.2022.11.009
Christl M., Vockenhuber C., Kubik P., Wacker L., Lachner J., Alfimov V., et al. (2013). The ETH Zurich AMS facilities: Performance parameters and reference materials. Nucl. Instruments Methods Phys. Res. Section B: Beam Interact. Materials Atoms 294, 29–38. doi: 10.1016/j.nimb.2012.03.004
Clarke R. A., Gascard J.-C. (1983). The formation of labrador sea water. part i: Large-scale processes. J. Phys. Oceanography 131764–1778. doi: 10.1175/1520-0485(1983)013⟨1764:TFOLSW⟩2.0.CO;2
Colombo M., Rogalla B., Myers P. G., Allen S. E., Orians K. J. (2019). Tracing dissolved lead sources in the canadian arctic: insights from the canadian GEOTRACES program. ACS Earth Space Chem. 3, 1302–1314. doi: 10.1021/acsearthspacechem.9b00083
Dahlgaard H., Chen Q., Herrmann J., Nies H., Ibbett R., Kershaw P. (1995). On the background level of 99Tc, 90Sr and 137Cs in the North Atlantic. J. Mar. Syst. 6, 571–578. doi: 10.1016/0924-7963(95)00025-K
Dale D., Christl M., Vockenhuber C., Macrander A., Ólafsdóttir S., Middag R., et al. (2024). Tracing ocean circulation and mixing from the arctic to the subpolar north atlantic using the 129i–236u dual tracer. J. Geophysical Research: Oceans 129, e2024JC021211. doi: 10.1029/2024JC021211.E2024JC0212112024JC021211
Daniault N., Mercier H., Lherminier P., Sarafanov A., Falina A., Zunino P., et al. (2016). The northern North Atlantic Ocean mean circulation in the early 21st century. Prog. Oceanography 146, 142–158. doi: 10.1016/j.pocean.2016.06.007
De Steur L., Hansen E., Mauritzen C., Beszczynska-Möller A., Fahrbach E. (2014). Impact of recirculation on the east Greenland current in fram strait: Results from moored current meter measurements between 1997 and 2009. Deep Sea Res. Part I: Oceanographic Res. Papers 92, 26–40. doi: 10.1016/j.dsr.2014.05.018
Ditlevsen P., Ditlevsen S. (2023). Warning of a forthcoming collapse of the Atlantic meridional overturning circulation. Nat. Commun. 14, 4254. doi: 10.1038/s41467-023-39810-w
Edmonds H. N., Smith J. N., Livingston H. D., Kilius L. R., Edmond J. M. (1998). 129I in archived seawater samples. Deep Sea Res. Part I: Oceanographic Res. Papers 45, 1111–1125. doi: 10.1016/S0967-0637(98)00007-7
Fogelqvist E., Blindheim J., Tanhua T., Østerhus S., Buch E., Rey F. (2003). Greenland–scotland overflow studied by hydro-chemical multivariate analysis. Deep Sea Res. Part I: Oceanographic Res. Papers 50, 73–102. doi: 10.1016/S0967-0637(02)00131-0
Frajka-Williams E., Ansorge I. J., Baehr J., Bryden H. L., Chidichimo M. P., Cunningham S. A., et al. (2019). Atlantic meridional overturning circulation: observed transport and variability. Front. Mar. Sci. 6. doi: 10.3389/fmars.2019.00260
Furey H., Bower A., Ramsey A., Houk A., Meunier T. (2024). Variability of Iceland scotland overflow water across the reykjanes ridge: 2-years of moored observations in the bight fracture zone. JGR Oceans 129, e2023JC020463. doi: 10.1029/2023JC020463
García-Ibáñez M. I., Pardo P. C., Carracedo L. I., Mercier H., Lherminier P., Ríos A. F., et al. (2015). Structure, transports and transformations of the water masses in the Atlantic Subpolar Gyre. Prog. Oceanography 135, 18–36. doi: 10.1016/j.pocean.2015.03.009
García-Ibáñez M. I., Pérez F. F., Lherminier P., Zunino P., Mercier H., Tréguer P. (2018). Water mass distributions and transports for the 2014 GEOVIDE cruise in the North Atlantic. Biogeosciences 15, 2075–2090. doi: 10.5194/bg-15-2075-2018
Gou R., Pennelly C., Myers P. G. (2022). The changing behavior of the west Greenland current system in a very high-resolution model. J. Geophysical Research: Oceans 127. doi: 10.1029/2022JC018404
Håvik L., Pickart R. S., Våge K., Torres D., Thurnherr A. M., Beszczynska-Möller A., et al. (2017). Evolution of the east Greenland current from fram strait to Denmark strait: Synoptic measurements from summer 2012. JGR Oceans 122, 1974–1994. doi: 10.1002/2016JC012228
He P., Hou X., Aldahan A., Possnert G., Yi P. (2013). Iodine isotopes species fingerprinting environmental conditions in surface water along the northeastern atlantic ocean. Sci. Rep. 3, 2685. doi: 10.1038/srep02685
Holliday N. P., Bacon S., Allen J., McDonagh E. L. (2009). Circulation and transport in the western boundary currents at cape farewell, Greenland. J. Phys. Oceanogr. 39, 1854–1870. doi: 10.1175/2009JPO4160.1
Holliday N. P., Bersch M., Berx B., Chafik L., Cunningham S., Florindo-López C., et al. (2020). Ocean circulation causes the largest freshening event for 120 years in eastern subpolar north atlantic. Nat. Commun. 11, 585. doi: 10.1038/s41467-020-14474-y
Holliday N. P., Meyer A., Bacon S., Alderson S., de Cuevas B. A. (2007a). The retroflection of part of the east Greenland current at cape farewell. Geophys. Res. Lett. 34, L07609. doi: 10.1029/2006GL029085
Holliday N. P., Meyer A., Bacon S., Alderson S. G., de Cuevas B. (2007b). Retroflection of part of the east Greenland current at Cape Farewell. Geophysical Res. Lett. 34, L07609. doi: 10.1029/2006GL029085
Johns W. E., Devana M., Houk A., Zou S. (2021). Moored observations of the Iceland-scotland overflow plume along the eastern flank of the reykjanes ridge. J. Geophysical Research: Oceans 126. doi: 10.1029/2021JC017524
Kershaw P., Baxter A. (1995). The transfer of reprocessing wastes from north-west Europe to the Arctic. Deep Sea Res. Part II: Topical Stud. Oceanography 42, 1413–1448. doi: 10.1016/0967-0645(95)00048-8
Lavender K. L., Brechner Owens W., Davis R. E. (2005). The mid-depth circulation of the subpolar North Atlantic Ocean as measured by subsurface floats. Deep Sea Res. Part I: Oceanographic Res. Papers 52, 767–785. doi: 10.1016/j.dsr.2004.12.007
Le Bihan C., Lherminier P., Le Bot P., Hamon M. (2023). BOCATS 2021. Rapport de données CTD-O2. ifremer. doi: 10.13155/98984
Li F., Lozier M. S., Bacon S., Bower A. S., Cunningham S. A., De Jong M. F., et al. (20213002). Subpolar North Atlantic western boundary density anomalies and the Meridional Overturning Circulation. Nat. Commun. 12. doi: 10.1038/s41467-021-23350-2
Lilly J. M., Rhines P. B., Schott F., Lavender K., Lazier J., Send U., et al. (2003). Observations of the Labrador Sea eddy field. Prog. Oceanography 59, 75–176. doi: 10.1016/j.pocean.2003.08.013
Lin P., Pickart R. S., Jochumsen K., Moore G. W. K., Valdimarsson H., Fristedt T., et al. (2020). Kinematic structure and dynamics of the Denmark strait overflow from ship-based observations. J. Phys. Oceanography 503235–3251. doi: 10.1175/JPO-D-20-0095.1
Liu M., Tanhua T. (2021). Water masses in the Atlantic Ocean: characteristics and distributions. Ocean Sci. 17, 463–486. doi: 10.5194/os-17-463-2021
Livingston H. D., Anderson R. F. (1983). Large particle transport of plutonium and other fallout radionuclides to the deep ocean. Nature 303, 228–231. doi: 10.1038/303228a0
Lozier M. S. (2023). Overturning in the subpolar north atlantic: a review. Phil. Trans. R. Soc A. 381, 20220191. doi: 10.1098/rsta.2022.0191
Lozier M. S., Gary S. F., Bower A. S. (2013). Simulated pathways of the overflow waters in the North Atlantic: Subpolar to subtropical export. Deep Sea Res. Part II: Topical Stud. Oceanography 85, 147–153. doi: 10.1016/j.dsr2.2012.07.037
Lozier M. S., Li F., Bacon S., Bahr F., Bower A. S., Cunningham S. A., et al. (2019). A sea change in our view of overturning in the subpolar North Atlantic. Science 363, 516–521. doi: 10.1126/science.aau6592
Michel R., Daraoui A., Gorny M., Jakob D., Sachse R., Tosch L., et al. (2012). Iodine-129 and iodine-127 in European seawaters and in precipitation from Northern Germany. Sci. Total Environ. 419, 151–169. doi: 10.1016/j.scitotenv.2012.01.009
Olsson K. A., Jeansson E., Tanhua T., Gascard J.-C. (2005). The East Greenland Current studied with CFCs and released sulphur hexafluoride. J. Mar. Syst. 55, 77–95. doi: 10.1016/j.jmarsys.2004.07.019
Pacini A., Pickart R. S. (2022). Meanders of the west Greenland current near cape farewell. Deep Sea Res. Part I: Oceanographic Res. Papers 179, 103664. doi: 10.1016/j.dsr.2021.103664
Payne A., Wefing A., Christl M., Vockenhuber C., Williams W., Smith J. N., et al. (2024). Circulation timescales and pathways of atlantic water in the Canada basin: Insights from transient tracers 129 i and 236 u. JGR Oceans 129, e2023JC020813. doi: 10.1029/2023JC020813
Pickart R. S. (1992). Water mass components of the north atlantic deep western boundary current. Deep Sea Res. Part A. Oceanographic Res. Papers 39, 1553–1572. doi: 10.1016/0198-0149(92)90047-W
Poole R., Tomczak M. (1999). Optimum multiparameter analysis of the water mass structure in the atlantic ocean thermocline. Deep Sea Res. Part I: Oceanographic Res. Papers 46, 1895–1921. doi: 10.1016/S0967-0637(99)00025-4
Raisbeck G., Yiou F. (1999). 129I in the oceans: origins and applications. Sci. Total Environ., 237–238. doi: 10.1016/S0048-9697(99)00122-9
Rhein M., Kieke D., Hüttl-Kabus S., Roessler A., Mertens C., Meissner R., et al. (2011). Deep water formation, the subpolar gyre, and the meridional overturning circulation in the subpolar North Atlantic. Deep Sea Res. Part II: Topical Stud. Oceanography 58, 1819–1832. doi: 10.1016/j.dsr2.2010.10.061
Rousi E., Selten F., Rahmstorf S., Coumou D. (2021). Changes in north atlantic atmospheric circulation in a warmer climate favor winter flooding and summer drought over europe. J. Climate 34, 2277–2295. doi: 10.1175/JCLI-D-20-0311.1
Rudels B. (2002). The east Greenland current and its contribution to the Denmark strait overflow. ICES J. Mar. Sci. 59, 1133–1154. doi: 10.1006/jmsc.2002.1284
Rudels B., Eriksson P., Grönvall H., Hietala R., Launiainen J. (1999). Hydrographic observations in Denmark strait in fall 1997, and their implications for the entrainment into the overflow plume. Geophysical Res. Lett. 26, 1325–1328. doi: 10.1029/1999GL900212
Sakaguchi A., Kadokura A., Steier P., Takahashi Y., Shizuma K., Hoshi M., et al. (2012). Uranium-236 as a new oceanic tracer: A first depth profile in the Japan Sea and comparison with caesium-137. Earth Planetary Sci. Lett. 333 (334), 165–170. doi: 10.1016/j.epsl.2012.04.004
Smethie W. M., Fine R. A., Putzka A., Jones E. P. (2000). Tracing the flow of North Atlantic Deep Water using chlorofluorocarbons. J. Geophys. Res. 105, 14297–14323. doi: 10.1029/1999JC900274
Smith J. N. (2005). Iodine 129/CFC 11 transit times for Denmark Strait Overflow Water in the Labrador and Irminger Seas. J. Geophysical Res. 110, C05006. doi: 10.1029/2004JC002516
Smith J. N., Ellis K. M., Kilius L. R. (1998). 129I and 137Cs tracer measurements in the Arctic Ocean. Deep Sea Res. Part I: Oceanographic Res. Papers 45, 959–984. doi: 10.1016/S0967-0637(97)00107-6
Smith J. N., McLaughlin F. A., Smethie W. M., Moran S. B., Lepore K. (2011). Iodine-129, 137 Cs, and CFC-11 tracer transit time distributions in the Arctic Ocean. J. Geophysical Res. 116, C04024. doi: 10.1029/2010JC006471
Stramma L., Kieke D., Rhein M., Schott F., Yashayaev I., Koltermann K. P. (2004). Deep water changes at the western boundary of the subpolar north atlantic during 1996 to 2001. Deep Sea Res. Part I: Oceanographic Res. Papers 51, 1033–1056. doi: 10.1016/j.dsr.2004.04.001
Susan Lozier M., Bacon S., Bower A. S., Cunningham S. A., Femke De Jong M., De Steur L., et al. (2017). Overturning in the subpolar north atlantic program: A new international ocean observing system. Bull. Am. Meteorological Soc. 98, 737–752. doi: 10.1175/BAMS-D-16-0057.1
Susan Lozier M., Bower A. S., Furey H. H., Drouin K. L., Xu X., Zou S. (2022). Overflow water pathways in the North Atlantic. Prog. Oceanography 208, 102874. doi: 10.1016/j.pocean.2022.102874
Sutherland D. A., Pickart R. S., Peter Jones E., Azetsu-Scott K., Jane Eert A., d Olafsson J. (2009). Freshwater composition of the waters off southeast Greenland and their link to the Arctic Ocean. J. Geophysical Res. 114, C05020. doi: 10.1029/2008JC004808
Tanhua T. (2005). Spreading of overflow water from the Greenland to the Labrador Sea. Geophys. Res. Lett. 32, L10605. doi: 10.1029/2005GL022700
Tanhua T., Olsson K. A., Jeansson E. (2005). Formation of Denmark Strait overflow water and its hydro-chemical composition. J. Mar. Syst. 57, 264–288. doi: 10.1016/j.jmarsys.2005.05
Våge K., Pickart R. S., Spall M. A., Moore G., Valdimarsson H., Torres D. J., et al. (2013). Revised circulation scheme north of the Denmark strait. Deep Sea Res. Part I: Oceanographic Res. Papers 79, 20–39. doi: 10.1016/j.dsr.2013.05.007
Vockenhuber C., Casacuberta N., Christl M., Synal H.-A. (2015). Accelerator Mass Spectrometry of 129 I towards its lower limits. Nucl. Instruments Methods Phys. Res. Section B: Beam Interact. Materials Atoms 361, 445–449. doi: 10.1016/j.nimb.2015.01.061
Wefing A.-M., Casacuberta N., Christl M., Dodd P. A. (2022). Water mass composition in Fram Strait determined from the combination of 129 I and 236 U: Changes between 2016, 2018, and 2019. Front. Mar. Sci. 9. doi: 10.3389/fmars.2022.973507
Wefing A.-M., Casacuberta N., Christl M., Gruber N., Smith J. N. (2021). Circulation timescales of Atlantic Water in the Arctic Ocean determined from anthropogenic radionuclides. Ocean Sci. 17, 111–129. doi: 10.5194/os-17-111-2021
Wefing A., Christl M., Vockenhuber C., Rutgers van der Loeff M., Casacuberta N. (2019). Tracing atlantic waters using 129 I and 236 U in the fram strait in 2016. J. Geophysical Research: Oceans 124, 882–896. doi: 10.1029/2018JC014399
Weijer W., Haine T., Siddiqui A., Cheng W., Veneziani M., Kurtakoti P. (2022). Interactions between the arctic mediterranean and the atlantic meridional overturning circulation: A review. Oceanog. 35 (3–4), 118–127. doi: 10.5670/oceanog.2022.130
Xu X., Rhines P. B., Chassignet E. P., Schmitz W. J. (2015). Spreading of Denmark strait overflow water in the western subpolar north atlantic: insights from eddy-resolving simulations with a passive tracer. J. Phys. Oceanography 45, 2913–2932. doi: 10.1175/JPO-D-14-0179.1
Yashayaev I. (2007). Changing freshwater content: Insights from the subpolar North Atlantic and new oceanographic challenges. Prog. Oceanography 73, 203–209. doi: 10.1016/j.pocean.2007.04.014
Yashayaev I. (2024). Intensification and shutdown of deep convection in the labrador sea were caused by changes in atmospheric and freshwater dynamics. Commun. Earth Environ. 5, 156. doi: 10.1038/s43247-024-01296-9
Zhang Y., Chen C.-S., Shen X.-Y., Xu D.-Y., Shao W.-Z., Beardsley R. C., et al. (2021b). Role of sea level pressure in variations of the canadian arctic archipelago throughflow. Adv. Climate Change Res. 12, 539–552. doi: 10.1016/j.accre.2021.07.009
Zhang J., Weijer W., Steele M., Cheng W., Verma T., Veneziani M. (2021a). Labrador Sea freshening linked to Beaufort Gyre freshwater release. Nat. Commun. 12, 1229. doi: 10.1038/s41467-021-21470-3
Zou S., Bower A. S., Lozier M. S., Furey H. H. (2023). Deep ocean circulation in the subpolar north atlantic observed by acoustically-tracked floats. Prog. Oceanography 211, 102975. doi: 10.1016/j.pocean.2023.102975
Zou S., Petit T., Li F., Lozier M. S. (2024). Observation-based estimates of water mass transformation and formation in the labrador sea. J. Phys. Oceanography. 54, 1411–1429. doi: 10.1175/JPO-D-23-0235.1
Zunino P., Lherminier P., Mercier H., Daniault N., García-Ibáñez M. I., Pérez F. F. (2017). The GEOVIDE cruise in May–June 2014 reveals an intense Meridional Overturning Circulation over a cold and fresh subpolar North Atlantic. Biogeosciences 14, 5323–5342. doi: 10.5194/bg-14-5323-2017
Appendix A: Acronyms
Keywords: radionuclides, I-129, U-236, tracers, water masses provenance, subpolar North Atlantic
Citation: Leist LGT, Castrillejo M, Smith JN, Christl M, Vockenhuber C, Velo A, Lherminier P and Casacuberta N (2024) 129I and 236U distribution in the subpolar North Atlantic unravels water mass provenance in AR7W and A25 lines. Front. Mar. Sci. 11:1470675. doi: 10.3389/fmars.2024.1470675
Received: 25 July 2024; Accepted: 05 November 2024;
Published: 18 December 2024.
Edited by:
Toru Miyama, Japan Agency for Marine-Earth Science and Technology, JapanReviewed by:
Xiaobiao Xu, Florida State University, United StatesXabier Davila, Norwegian Research Institute (NORCE), Norway
Copyright © 2024 Leist, Castrillejo, Smith, Christl, Vockenhuber, Velo, Lherminier and Casacuberta. This is an open-access article distributed under the terms of the Creative Commons Attribution License (CC BY). The use, distribution or reproduction in other forums is permitted, provided the original author(s) and the copyright owner(s) are credited and that the original publication in this journal is cited, in accordance with accepted academic practice. No use, distribution or reproduction is permitted which does not comply with these terms.
*Correspondence: Lisa G. T. Leist, bGlzYS5sZWlzdEB1c3lzLmV0aHouY2g=