- 1Marine Institute, University of Plymouth, Plymouth, United Kingdom
- 2Plymouth Marine Laboratory, Prospect Place, Plymouth, United Kingdom
- 3School of Biosciences, University of Birmingham, Birmingham, United Kingdom
This review provides a critical assessment of knowledge regarding the occurrence and behaviour of volatile, low molecular weight amines, particularly methylamines and quaternary amines, in marine aquatic systems. It provides an up-to-date evaluation of their presence within marine ecosystems, the processes likely to control their flux across the sea-air interface, and analytical techniques associated with their measurement. Interest in the occurrence and cycling of these groups of compounds in seawater has increased within the last 10–15 years, due to their potential role in climate regulation. As such, the need for wider measurements and mechanistic studies to elucidate their role within biological communities and, more widely, the nitrogen cycle and marine ecosystem models, is apparent. Finally, we make recommendations on what research questions are most suitable for future studies in this area.
Introduction
Nitrogen (N) is ubiquitous in marine systems and an essential macro-nutrient for primary production. It exists in both inorganic and organic form, with oxidation states ranging from +5 (e.g. nitrate; NO3-) to -3 (e.g. ammonia; NH3, glycine; CO2HCH2NH2). Molecules in the dissolved organic N (DON) pool can be ionised or neutral (gaseous), which influences both their volatility, aqueous residence time and atmospheric flux.
Trace gases play critical roles in marine biogeochemical cycles, atmospheric chemistry and climate (Carpenter et al., 2012; Almeida et al., 2013; Riccobono et al., 2014). Phytoplankton synthesise organic compounds as osmolytes and cryoprotectants, including dimethylsulfoniopropionate and quaternary amines (QAs). The former has received attention as a precursor of the cloud-promoting gas, dimethylsulfide (DMS); however, amines may be equally important (Almeida et al., 2013; Riccobono et al., 2014; Tilgner et al., 2021; Hoffmann et al., 2024). For example, glycine betaine, a QA commonly termed “betaine” in early literature, is among the most widely-used compatible solutes in nature (Yancey et al., 1982). Nitrogen-containing osmolytes (N-osmolytes), such as glycine betaine, are produced by marine phytoplankton to maintain osmotic pressure (Yancey, 2005; Burg and Ferraris, 2008). These molecules can degrade to produce methylamines (Jameson et al., 2016a; King, 1984; Oremland et al., 1982; King, 1988; Oren, 1990), chemical analogues of ammonia with the molecular formula (CH3)nNH3-n. Marine bacteria use MAs as a source of energy and remineralise the nitrogen to ammonium (Lidbury et al., 2015). The MAs are also required by bacteria for conversion of DMS to dimethylsulfoxide (Lidbury et al., 2016), firmly establishing them as important components in marine biogeochemical cycles.
In dissolved, gaseous form, MAs can diffuse across the sea-air interface to participate in climate-regulation processes (Figure 1). Indeed, atmospheric MA concentrations above 65 nmol m-3 could account for observed atmospheric particle-formation rates (Almeida et al., 2013).
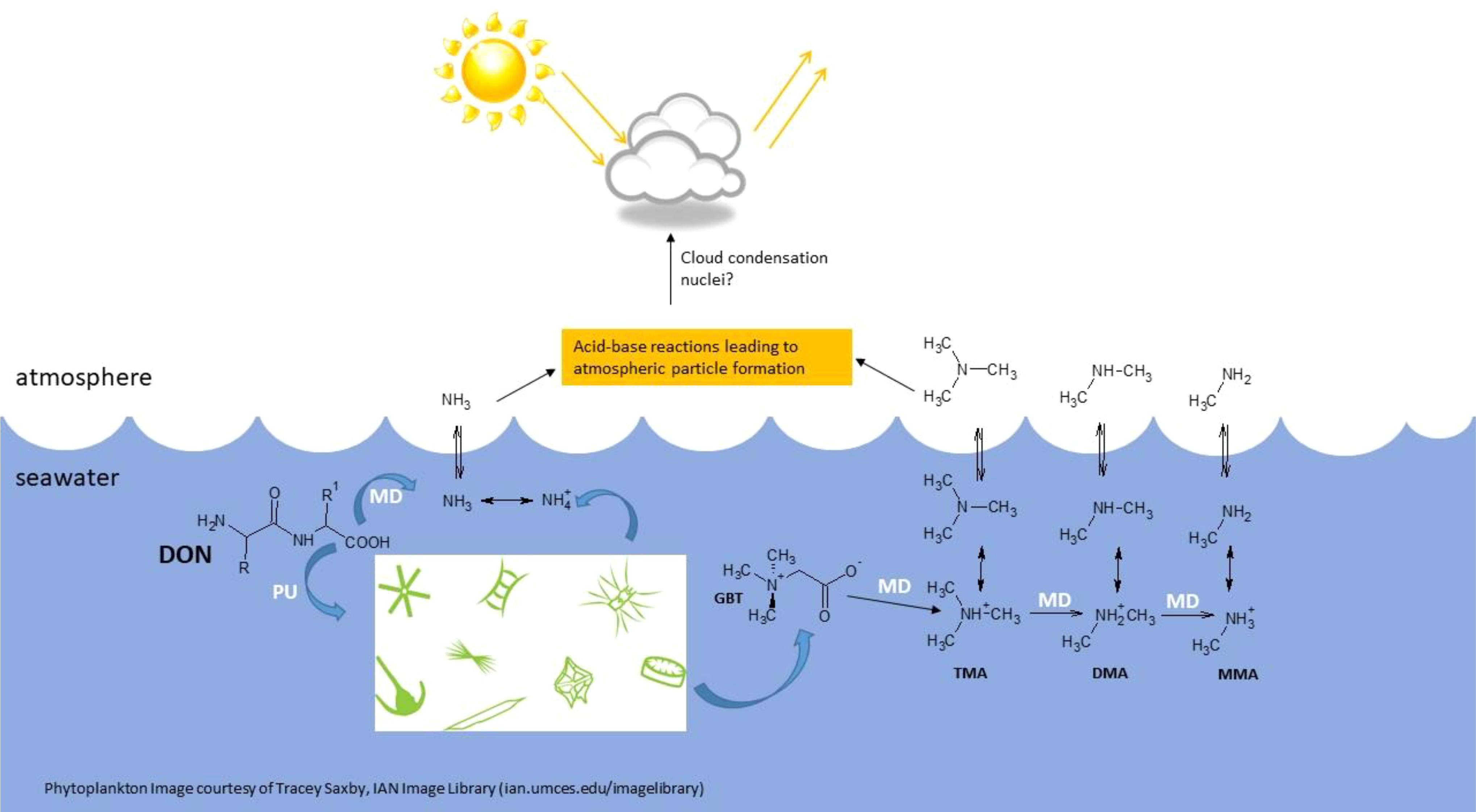
Figure 1. Proposed formation and fluxes of methylamines (MAs) and their precursors, the quaternary amines (QAs), in seawater. Glycine betaine is used as an example of a QA. DON, dissolved organic nitrogen (dipeptide molecule included; R and R1 refer to side chains on the amino acid residues); GBT, glycine betaine; TMA, trimethylamine; DMA, dimethylamine; MMA, monomethylamine; PU, phytoplankton uptake; MD, microbial degradation.
In this article, we review current knowledge on the occurrence, cycling and measurement of quaternary and ternary amines used as osmolytes, and volatile, low molecular weight amines (MAs and other amines whose presence has been reported). Their behaviour is evaluated with reference to molecular structure, physico-chemical properties, and identified microbial interventions and pathways. Finally, we propose how our understanding of the roles and significance of these compounds within the marine environment might be progressed.
Quaternary and ternary amines
Occurrence and role
Quaternary and ternary amines are used extensively by both phytoplankton and bacteria as osmoprotective compounds (osmolytes). Cells alter the cytoplasmic concentration of these compounds in response to changing salinity via synthesis and/or uptake (Sleator and Hill, 2002). Most quaternary amines are compatible solutes, meaning that they are highly soluble molecules which carry no net charge in vivo and do not interact with cellular proteins. As well as maintaining osmotic balance, compatible solutes have a stabilising effect on proteins, protecting against salinity, temperature changes and desiccation (Sleator and Hill, 2002). Glycine betaine (GBT) is a commonly-used osmolyte among both phytoplankton and bacteria; others include carnitine, trigonelline, homarine and the ternary amines proline and ectoine (Table 1).
Of the N-osmolytes listed in Table 1 and reported in the literature, GBT has received the most attention (Mäkelä et al., 2019). While the role of GBT as a compatible solute has been extensively reported, it may also be used for the biosynthesis of other N-osmolytes, including chloine, sarcosine and carnitine (Boysen et al., 2022). As such, most of the studies reported in this section focus on GBT but we note that information is needed on a wider range of N-osmolytes to fully appreciate the significance of N-osmolytes in marine ecosystems.
The accumulation of specific osmolytes by bacteria depends on the level of osmotic stress, and the availability of substrates and osmolytes in the environment (Burg and Ferraris, 2008). Over diel cycles in the North Pacific Ocean, osmolytes exhibited the largest diel oscillations of all the metabolites detected, implying rapid turnover and metabolic roles beyond cell turgor maintenance (Boysen et al., 2021). This underlines the importance of GBT and other N-osmolytes as currencies for microbes in terms of the exchange of carbon (C) and N. In fact, labelling studies demonstrated that N derived from GBT made its way through the marine microbial community (Boysen et al., 2022). Although some groups of bacteria can access GBT as a source of N, C or energy, most bacteria target this compound for osmotic functions – many bacteria can transport it but not synthesise or break it down (McParland et al., 2021). In natural bacterial communities, 88% of GBT transported into cells remained unmetabolised (Boysen et al., 2022). The ability to break down GBT is a highly specialised metabolic activity (McParland et al., 2021). For example, SAR11 has a high affinity transporter for GBT (Noell and Giovannoni, 2019) and uses GBT as a source of methyl groups to fuel the methionine cycle (Boysen et al., 2022). Also, GBT transporters are found frequently in marine bacterial genomes and its transcripts can be detected in metatranscriptomic datasets (McParland et al., 2021). This further supports the importance of the compound in osmotic function. There was, however, substantial remineralisation of GBT as a C substrate in the global ocean transcriptome (McParland et al., 2021). In a seasonal study, prokaryotic GBT uptake was influenced mainly by sea surface temperature, salinity and macronutrient concentrations (Mausz et al., 2022). The uptake of this compound provides an effective alternative nutrient source for prokaryotes in marine systems and might be more widespread than previously thought (Mausz et al., 2022). Members of the Rhodobacteraceae have been found to metabolise MAs and GBT, providing an ammonium source to diatoms in co-culture (Zecher et al., 2020). This is proposed to occur in the phycosphere in the natural environment, demonstrating a nutritional benefit for diatoms to exude organic nitrogen compounds into the phycosphere (Zecher et al., 2020).
In a study of sinking particles across the South Atlantic Ocean, the metabolite profile was found to be dominated by GBT (Johnson et al., 2020). GBT also contributed a larger mole fraction in sinking material compared to surface material across several oceanic regions, indicating a widespread feature not linked to oceanographic location (Johnson et al., 2020). The high proportion of GBT was attributed to particle-associated microbial communities on the sinking particulates. Proline was also more dominant in sinking particles, suggesting both GBT and proline are important osmolytes at depth (Johnson et al., 2020).
GBT is also abundant in coral tissue, comprising 16% of coral tissue N (Hill, 2022). Corals have pathways for de novo synthesis as well as transport of GBT. It is proposed that GBT may be protective in warm water for reef-building corals, providing photoprotection from photon stress in these organisms, similar to descriptions for terrestrial plants (Hill, 2022).
Within phytoplankton, culture studies reveal that GBT production is relatively common, with GBT detected in 75% of the cultures analysed (Spielmeyer et al., 2011), though at lower concentrations than DMSP. GBT concentrations in phytoplankton have been found to depend strongly on growth conditions, including growth phase and nutrient limitation (Keller et al., 1999a; Keller et al., 1999b; Keller et al., 2004). In diatoms and a strain of the coccolithophore, Emiliania huxleyi, GBT production increased with both temperature and carbon dioxide levels (Spielmeyer and Pohnert, 2012). During a seasonal study in coastal temperate waters, GBT correlated with specific members of the dinoflagellate community in summer (Airs et al., 2023). Moreover a modelling study indicated GBT positively affects dinoflagellate fitness (Airs et al., 2023). The high cellular content of QA derivatives, such as GBT, has been shown to positively affect the buoyancy of marine phytoplankton (Boyd and Gradmann, 2002), indicating important roles beyond osmolarity. GBT is important for protection of drought, temperature and salt stress in higher plants (Sakamoto and Murata, 2002; Wang et al., 2010; Chen and Murata, 2011; Fan et al., 2012; Rivero et al., 2014). GBT has also been reported to stabilise antioxidant enzymes that can scavenge reactive oxygen species in plants (Liang et al., 2009), stabilise photosystem II (Papageorgiou and Murata, 1995; Huang et al., 2020) and accelerate the recovery of photosystem II in higher plants from heat stress (Li et al., 2014) or high light exposure (Kondo et al., 1999; Prasad and Saradhi, 2004) providing evidence of a photoprotective role for GBT (Wang et al., 2014; Li et al., 2021).
Analytical methods
Methods applied to the analysis of N-osmolytes have moved from stand-alone chromatography techniques, including thin layer chromatography (Storey and Wyn Jones, 1977; Dickson and Kirst, 1986) and high performance liquid chromatography, HPLC (Gorham, 1984), to hyphenated techniques utilising mass spectrometry, MS (Koc et al., 2002; Holm et al., 2003; Oufir et al., 2009; Airs and Archer, 2010; Spielmeyer et al., 2011; Beale and Airs, 2016; Ares et al., 2020). HPLC analysis typically comprised a cation exchange column and UV detection at a wavelength of 195 nm. The method required filtration of relatively large volumes of water (1-4 L) and was applied to measurements of phytoplankton cultures (Keller et al., 1999a; Keller et al., 1999b) and natural samples off the Gulf of Maine (Keller et al., 2004). The application of hyphenated LC-MS approaches yielded improvements in sensitivity (Airs and Archer, 2010; Beale and Airs, 2016), enabling smaller sample volumes. The LC-MS technique has been applied to QA analysis in coastal seawater (Airs and Archer, 2010; Beale and Airs, 2016), Atlantic Ocean (Airs and Archer, 2010) and Southern Ocean samples (Dall’Osto et al., 2017). Within the limited environmental sample sets analysed to date, osmolyte concentrations were highest in coastal systems (Keller et al., 2004; Airs and Archer, 2010; Airs et al., 2023), and Antarctic water influenced by sea ice (Dall’Osto et al., 2017). Osmolytes are particularly amenable to metabolomics methods, being among the most ubiquitous and abundant metabolites detected (Boysen et al., 2021; Johnson et al., 2020). This typically involves the filtration of large sample volumes, which is likely to be detrimental to accurate concentration measurements of osmolytes (Kiene and Slezak, 2006), but is very valuable for comparative studies of a wide range of metabolites (Boysen et al., 2021; Johnson et al., 2020). Absolute quantification is possible but requires addition of quantification standards for each compound to be quantified (Boysen et al., 2021). Normalisation techniques have been developed to optimise the use of internal standards for rectifying obscuring variation (the combined effect on peak areas of sample-matrix-induced ion suppression, injection volume, chromatographic quality and analytical drift) during analysis (Boysen et al., 2018).
While analysis of particulate-associated N-osmolytes has been possible for some time, methodology to determine dissolved concentrations of N-osmolytes in marine systems, together with other dissolved metabolites, has only recently been achieved via a cation exchange solid phase extraction method (Sacks et al., 2022). Sacks et al. (2022) achieved a limit of detection for dissolved GBT of 0.38 nM and reported concentrations of 2.5-5.2 nM in 3 marine samples. Mausz et al. (2022) applied an uptake kinetics approach of diluted and undiluted samples (Wright and Hobbie, 1966) to estimate the dissolved concentrations of GBT and choline in coastal seawater and determined them to be in the low nanomolar range (Mausz et al., 2022).
Volatile amines
Occurrence and role
Small volatile amines are nitrogen-containing molecules with the general formula RnNH(3-n); they are alkylated analogies of ammonia and N is in the -3 oxidation state. In seawater, the most reported low molecular weight amines are the methylamines (MAs), which exist as primary (monomethylamine; MMA), secondary (dimethylamine; DMA) and tertiary (trimethylamine; TMA) species (Abdul-Rashid et al., 1991; Gibb et al., 1999a; Gibb et al., 1999b; Gibb and Hatton, 2004; Dall’Osto et al., 2017; Cree et al., 2018; Dall’Osto et al., 2019). The MAs are highly soluble in water. At seawater pH they are predominantly protonated (cations), though in equilibrium with their neutral, gaseous forms. Table 2 shows physico-chemical characteristics of the MAs.
The MAs have been detected at micromolar levels in estuarine environments. In cation form they can sorb to particles and fractionate between sediment porewaters and particles (Wang and Lee, 1990; Wang and Lee, 1993; Fitzsimons et al., 2005; Fitzsimons et al., 2006). The sorptive interactions between the MA and a particle surface are identical to those reported for NH3. An ionic bond can form between the cation and a negatively-charged surface group on the particle, while the neutral species can react with a carbonyl functional group (carbonyl addition) to form a covalent bond - this is not possible for TMA or QAs as the molecule must contain at least one N-H bond. Ionic bonding should be the major sorption pathway for MAs, given the predominance of the protonated form at seawater pH. However, carbonyl addition may occur, and has been proposed for amino acids (which contain a primary amino function group), analogous to melaniodin-type reactions (Henrichs and Sugai, 1993).
The sorption of MAs to particulates is reversible. For example, Wang and Lee (1993) compared adsorption and desorption coefficients for the MAs using 14C-MAs on an inter-tidal sediment, and found that 63-90% of the label was desorbed, with the extent of desorption corresponding to the number of methyl substituents (MMA < DMA < TMA). A study on the influence of sediment resuspension on estuarine water column concentrations of MAs revealed that particulate release accounted for > 90% of the dissolved MA increase measured over a tidal cycle (Fitzsimons et al., 2006).
Wang and Lee (1990) reported seasonal control on the concentrations of MAs in inter-tidal sediments, where an increase corresponded with annual senescence of salt marsh grasses. While increasing the land-air flux of MAs, this process also provides non-competitive substrates for methanogenic bacteria (King, 1984) and allows them to co-exist with sulphate-reducing bacteria that outcompete them for more energetically favourable molecules, such as acetate. As concentrations of the MAs are thought to represent under 1% of total sedimentary N (Burdige et al., 1995; Lee and Olsen, 1984), it was suggested that this N-fraction has limited environmental significance (Burdige et al., 1995). However, as interest grows in their potential influence on climate (Almeida et al., 2013), quantification of the MA flux from sediments, particularly inter-tidal sediments, will be an important part of elucidating their role in atmospheric processes.
Concentrations of volatile amines have been measured in both coastal and oceanic waters (e.g. Gibb et al., 1999a; Gibb and Hatton, 2004; Cree et al., 2018). In addition, concurrent water and air concentrations have been reported from a number of studies (Gibb et al., 1999b; Dall’Osto et al., 2017; Dall’Osto et al., 2019; Van Pinxteren et al., 2019); these are summarised in Table 3. Concentrations of MAs of up to 38 nM were measured in seawater by Gibb et al. (1995), though the most abundant MAs measured varied between studies. For example, MMA was the most abundant MA measured in the Mediterranean (Gibb et al., 1995) and Arabian Seas (Gibb et al., 1999a), while TMA was most abundant in samples measured in the English Channel and the Southern Ocean (Cree et al., 2018), where MMA was not detected. Higher MA concentrations were measured in samples from the Sea Surface Microlayer (up to 50 nM) but this study did not include analysis of TMA (Van Pinxteren et al., 2019).
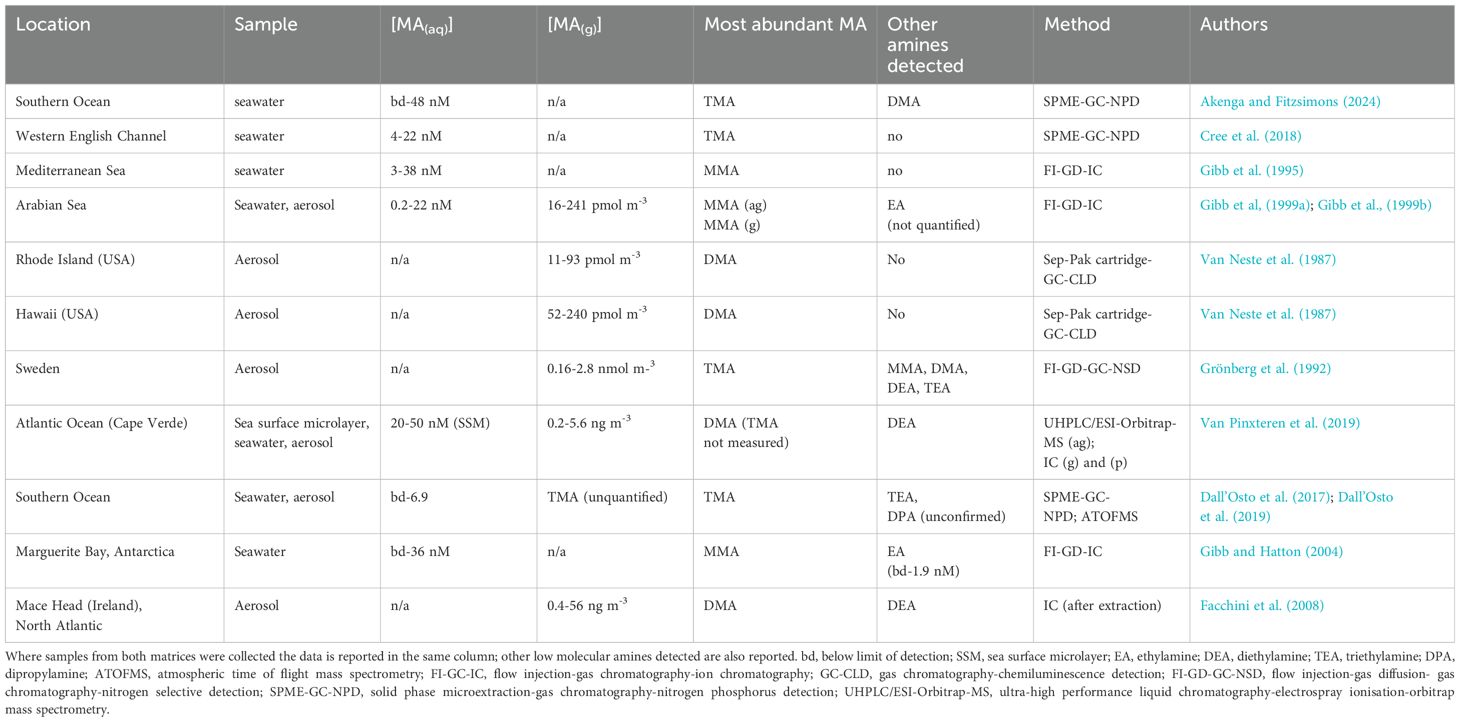
Table 3. Examples of methylamines’ concentrations measured in seawater and marine atmospheric samples, and those reported for other volatile amines.
The concurrent detection of MAs in seawater and atmospheric samples has suggested an oceanic source for these analytes (Gibb et al., 1999a; Gibb et al., 1999b; Dall’Osto et al., 2017; Dall’Osto et al., 2019). Although Facchini et al. (2008) did not measure amine concentrations in waters of the North Atlantic, concentrations of DMA and diethylamine (DEA) in marine aerosol were highest during periods of increased biological activity. Interestingly, while Van Pinxteren et al. (2019) associated highest MA concentrations with biological activity through correlation with chlorophyll-a and fucoxanthin, a diatom pigment, flux calculations suggested that seawater may be a sink for DMA. While the production of MAs in the water column can be linked to the degradation of quaternary amines, such as GBT, trimethylamine oxide and choline (Lidbury et al., 2015), precursors for other detected amines, namely mono-, di- and triethylamine do not have obvious sources, via C-N bond cleavage, from within the marine organic nitrogen pool.
Figure 2 shows a range of MA concentrations reported for porewaters and seawater, and the atmospheric concentrations estimated, assuming sea-air exchange based on Henry’s Law (Leng et al., 2015; Dall’Osto et al., 2019). TMA was used as the representative MA for these calculations with its physical constants applied. Although the maximum values calculated are higher than reported atmospheric concentrations (Table 3) there is substantial overlap.
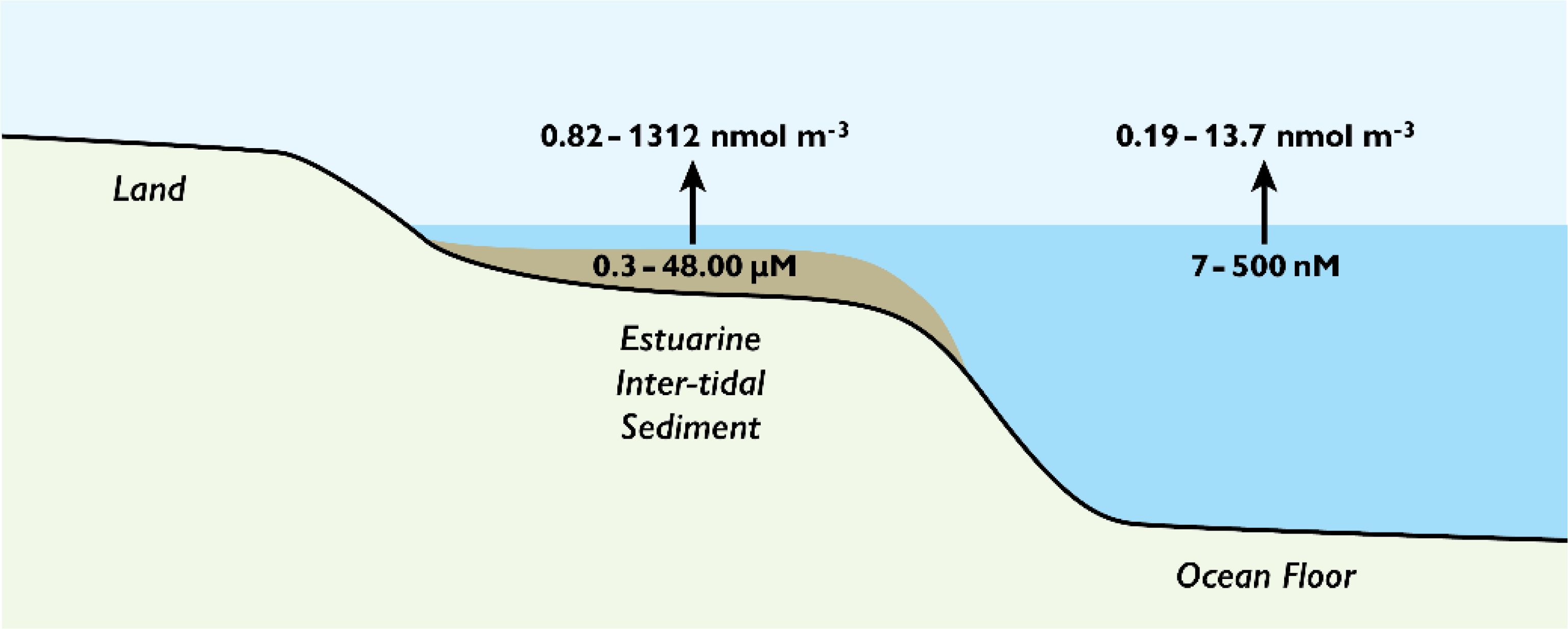
Figure 2. Estimates of the atmospheric concentrations of methylamines that could arise from sea-air exchange based on reported porewater and seawater concentrations. The porewater and seawater concentration ranges were obtained from Fitzsimons et al. (2023) and data from studies shown in Table 3, respectively. Trimethylamine (TMA) was used as a proxy as each compound has specific constants, and the concentrations calculated according to Leng et al. (2015). The seawater pH used was 8.2, and the pKa for TMA at 298 K.
Analytical methods
Given their low concentrations in marine samples, analytical methods employed for volatile amine analysis have typically employed a pre-concentration step. This step aims to both bring the compounds within the sensitivity of the instrument used for separation and detection and remove them from a complex sample matrix in advance of instrumental measurement. An early method employed microdiffusion of 50 mL seawater samples in an adapted Quikfit flask, adjusted to pH > 12, where the amines diffused into the flask headspace under heating over 24 hours, and were then captured in a small volume of dilute hydrochloric acid (Abdul-Rashid et al., 1991). While this method was applied to estuarine sediments and inshore waters, the small sample volume precluded measurements of oceanic samples with the lowest amine concentrations. Flow injection has been coupled with chromatography to achieve in-line pre-concentration of samples in several oceanic studies (Gibb et al., 1999a; Gibb et al., 1999b; Gibb and Hatton, 2004), where both seawater and atmospheric measurements were reported. Circulation diffusion was coupled with gas chromatography to measure amines in porewater and seawater (Yang et al., 1993). This method could detect amines in seawater down to 3 nM but required a sample volume of 1 L to measure the lowest concentrations, while a 0.5 L sample was sufficient for porewater samples.
Solid phase microextraction (SPME) coupled with gas chromatography (optimised for nitrogen molecules) has been recently used for analysis of seawater samples and has achieved limits of detection as low as any previously reported (Cree et al., 2018), enabling measurement of amines in water samples from the Southern Ocean (Dall’Osto et al., 2017; Dall’Osto et al., 2019). Limitations of this technique are the large sample volume required, and the time needed to extract each sample (1 L and 2.5 h, respectively). While the water samples are preserved after filtration (Cree et al., 2018), sample analysis and storage are limited with such large seawater volumes so installation of the gas chromatograph on board ship has been necessary for oceanic studies. Akenga and Fitzsimons (2024) developed an automated, in-line, preconcentration and sample injection procedure. The analytical method also employed SPME but with separate sample equilibration and extraction steps, which enabled them to achieve limits of detection for seawater samples that were close to those of Cree et al. (2018) while sample volumes (10 mL) were 100 x lower. This advance offers a systematic and standardized method for MA analysis in seawater and can significantly advance understanding of their abundance and role in marine systems.
The studies included in Table 3 show which MAs (and other volatile amines) were measured, the methodology employed, and which was most abundant. The number of analytes potentially detected was dependent on the method used (e.g. derivatization of analytes excludes TMA). Although each dataset of measurements is of considerable value, the low number of studies and the application of different analytical methods makes it difficult to compare data (Fitzsimons et al., 2023). Nonetheless, it is encouraging to see that the methods reported have been suitable for the measurement of MAs at the low concentrations expected for oceanic waters, and that the concentration ranges for water column measurements are broadly similar.
Synthesis and degradation of MAs and QAs
It is not completely understood how MAs can be formed in oxygenated marine waters. The fact that their concentration in marine sediments is at least one order of magnitude higher than in marine water columns suggests that MAs likely originate from anoxic marine sediment through microbial transformation of DOM. It is likely that TMA is the key molecule bridging the QA degradation cycle to the formation of MAs in oceanic waters through microbe-dependent and -independent pathways (see below).
There are at least four independent microbial pathways bridging the marine QA cycle to TMA formation: 1) bacterial degradation of choline to TMA via a glycyl radical containing choline-TMA lyase (Craciun and Balskus, 2012; Jameson et al., 2016b; Jameson et al., 2018); 2) degradation of GBT to TMA using a GBT reductase (Meyer et al., 1995); 3) reduction of TMAO to TMA through TMAO reductase (Barrett and Kwan, 1985) and 4) TMA formation from carnitine using a Rieske-containing carnitine oxygenase aerobically (Zhu et al., 2014) or through a coenzyme A (Co-A) dependent anaerobic pathway (Rajakovich et al., 2021). The first three pathways and Co-A dependent carnitine degradation are found in strict or facultative anaerobes. The carnitine oxygenase pathway was first characterized in aerobic human gut microbiota, and it remains to be established whether marine bacteria can indeed transform carnitine to TMA using this enzyme.
It is important to note that TMA may also be formed in the oxygenated marine water column independent of microbial transformation. A previous study has noted that betaine-containing lipids (e.g. diacylglyceryl trimethyl-β-alanine, DGTA) can spontaneously degrade to TMA. These non-phosphorus containing lipids are abundant in marine phytoplankton and may therefore represent an important source of oceanic TMA that has been overlooked (Vogel et al., 1990; Dembitsky, 1996).
The transformation of TMA to other MAs in the marine environment has also been studied over the past decade. TMA can be oxidised to DMA via either TMA dehydrogenase (Burgess et al., 2008) or through TMAO as the intermediate (Chen et al., 2011; Lidbury et al., 2014). Similarly, DMA oxidation to MMA can be catalysed by either a DMA dehydrogenase (Yang et al., 1995) or a DMA monooxygenase (Lidbury et al., 2017). MMA is further degraded to ammonium and CO2 through MMA dehydrogenase or a multi-step enzyme system involving two methylated amino acids as key intermediates (reviewed in Mausz and Chen, 2019). In the marine water column, MAs appear to serve as important C, N and energy sources for cosmopolitan marine microbes, particularly Alphaproteobacteria of the marine Roseobacter clade and the SAR11 clade (Chen et al., 2011; Sun et al., 2011; Lidbury et al., 2014; Lidbury et al., 2015; Lidbury et al., 2017).
The way forward
Interest in the occurrence and cycling of low molecular weight volatile amines and their precursor N-osmolytes in marine systems has increased considerably, with the former linked to processes influencing climate regulation. However, relatively little is known about the absolute concentrations of QAs and MAs in the global ocean compared to other biogeochemically relevant compounds, and this data is a key requirement for their future inclusion within marine models. While several analytical methods have been successfully applied to their determination, standardisation of methodology or inter-laboratory comparison studies would optimise confidence in datasets produced, through increased understanding of analytical variation and biases that can be incorporated into data interpretation. Small sample volume methods (50 mL) have been developed for measurement of QAs (Beale and Airs, 2016) and volatile amines (Akenga and Fitzsimons, 2024) in seawater, and these analytical advances can facilitate uncomplicated collection and preservation of samples throughout the world ocean for measurement of standing stocks and through dynamic periods of biological productivity. This will make a major contribution to the development of a substantial global database on their seawater concentrations, and can form the basis of models to quantify their significance within marine ecosystems.
Knowledge of metabolic pathways around the production and catabolism of these nitrogenous compounds is more advanced, but there remain significant gaps. For example, a convincing source of TMA in surface waters independent of anaerobic pathways has not been put forward. Given their widespread occurrence and utilisation by microbes, these low molecular weight compounds are emerging as a crucial underpinning currency in microbial food webs. As such, combined studies addressing biogeochemistry, analytical chemistry, transcriptomics and labelling studies are required to fully address research questions around these molecules.
The importance of QAs and MAs to marine biogeochemistry is further emphasised by the coupling of the nitrogen and sulphur cycles via their reliance on MAs for the oxidation of DMS to DMSO via the enzyme Tmm, and the use of the same enzyme for the oxidation of TMA to trimethylamine oxide (TMAO). The full ramifications of these intriguing links are yet to be established. Like DMSP and MAs, GBT can be a substrate for methanogens, providing important links to biological methane production.
Finally, metabolomics studies are really valuable for providing context across a wide range of marine metabolites (Durham et al., 2022). They can also provide focus for more targeted studies to better understand important processes, such as diel fluctuations of osmolytes and osmolyte use with depth.
Author contributions
MF: Funding acquisition, Supervision, Writing – original draft, Writing – review & editing. RA: Funding acquisition, Supervision, Writing – original draft, Writing – review & editing. YC: Funding acquisition, Writing – original draft, Writing – review & editing.
Funding
The author(s) declare that no financial support was received for the research, authorship, and/or publication of this article.
Acknowledgments
We are grateful to the Natural Environmental Research Council for a number of awards to support our understanding of methylamines and their analysis in marine systems ((NE/1528542/1; NE/R010382/1; NE/P008526/1). We thank Jamie Quinn (UoP) for the preparation of Figure 2 and Drs Manuel Dall’Osto and Marco Paglione for advice on sea-air exchange calculations. Finally, we are grateful to the reviewers for their constructive feedback and recommendations for improving the article.
Conflict of interest
The authors declare that the research was conducted in the absence of any commercial or financial relationships that could be construed as a potential conflict of interest.
Publisher’s note
All claims expressed in this article are solely those of the authors and do not necessarily represent those of their affiliated organizations, or those of the publisher, the editors and the reviewers. Any product that may be evaluated in this article, or claim that may be made by its manufacturer, is not guaranteed or endorsed by the publisher.
References
Abdul-Rashid M. K., Riley J. P., Fitzsimons M. F., Wolff G. A. (1991). Determination of volatile amines in sediment and water samples. Anal. Chim. Acta 252, 223–226. doi: 10.1016/0003-2670(91)87219-W
Airs R., Archer S. (2010). Analysis of glycine betaine and choline in seawater particulates by liquid chromatography/electrospray ionization/mass spectrometry. Limnol. Oceanogr. Meth. 8, 499–506. doi: 10.4319/lom.2010.8.499
Airs R. L., Beale R., Polimene L., Chen Y., Mausz M. A., Scanlan D. J., et al. (2023). Seasonal measurements of the nitrogenous osmolyte glycine betaine in marine temperate coastal waters. Biogeochemistry 162, 309–323. doi: 10.1007/s10533-022-01006-7
Akenga P. C., Fitzsimons M. F. (2024). Automated method for the sensitive analysis of volatile amines in seawater. ACS ES&T Water 4, 2504–2510. doi: 10.1021/acsestwater.4c00007
Almeida J., Schobesberger S., Kurten A., Ortega I. K., Kupiainen-Maatta O., Praplan A. P.. (2013). Molecular understanding of sulphuric acid-amine particle nucleation in the atmosphere. Nature 502, 359–363. doi: 10.1038/nature12663
Ares A. M., Toribio L., Nozal M. J., Martín M. T., Bernal J. (2020). Simultaneous determination of betaines and other quaternary ammonium related compounds in bee pollen by hydrophilic interaction liquid chromatography-mass spectrometry. Microchem. J. 157, 105000. doi: 10.1016/j.microc.2020.105000
Barrett E. L., Kwan H. S. (1985). Bacterial reduction of trimethylamine oxide. Annu. Rev. Microbiol. 39, 131–149. doi: 10.1146/annurev.mi.39.100185.001023
Beale R., Airs R. (2016). Quantification of glycine betaine, choline and trimethylamine N-oxide in seawater particulates: Minimisation of seawater associated ion suppression. Anal. Chim. Acta 938, 114–122. doi: 10.1016/j.aca.2016.07.016
Boyd C., Gradmann D. (2002). Impact of osmolytes on buoyancy of marine phytoplankton. Mar. Biol. 141, 605–618. doi: 10.1007/s00227-002-0872-z
Boysen A. K., Carlson L. T., Durham B. P., Groussman R. D., Aylward F. O., Ribalet F., et al. (2021). Particulate metabolites and transcripts reflect diel oscillations of microbial activity in the surface ocean. mSystems 6, e00896–e00820. doi: 10.1128/mSystems.00896-20
Boysen A. K., Durham B. P., Kumler W., Key R. S., Heal K. R., Carlson L. T., et al. (2022). Glycine Betaine uptake and metabolism in marine microbial communities. Environ. Microbiol. 24, 2380–2240. doi: 10.1111/1462-2920.16020
Boysen A. K., Heal K. R., Carlson L. T., Ingalls A. E. (2018). Best-matched internal standard normalization in liquid chromatography-mass spectrometry metabolomics applied to environmental samples. Anal. Chem. 90, 1363–1369. doi: 10.1021/acs.analchem.7b04400
Brill J., Hoffmann T., Bleisteiner M., Bremer E. (2011). Osmotically controlled synthesis of the compatible solute proline is critical for cellular defense of Bacillus subtilis against high osmolarity. J. Bacteriol. 193, 5335–5346. doi: 10.1128/JB.05490-11
Burdige D. J., Huang C. M., Krishna N., Scully F. E. (1995). Aliphatic amines in chesapeake bay sediments. Mar. Chem. 51, 45–54. doi: 10.1016/0304-4203(95)00042-P
Burg M. B., Ferraris J. D. (2008). Intracellular organic osmolytes: function and regulation. J. Biol. Chem. 283, 7309–7313. doi: 10.1074/jbc.R700042200
Burgess S. G., Messiha H. L., Katona G., Rigby S. E. J., Leys D., Scrutton N. S. (2008). Probing the dynamic interface between trimethylamine dehydrogenase (TMADH) and electron transferring flavoprotein (ETF) in the TMADH–2ETF complex: role of the Arg-α237 (ETF) and Tyr-442 (TMADH) residue pair. Biochemistry 47, 5168–5181. doi: 10.1021/bi800127d
Carpenter L. J., Archer S. D., Beale R. (2012). Ocean-atmosphere trace gas exchange. Chem. Soc Rev. 41, 6473–6506. doi: 10.1039/c2cs35121h
Chen T. H. H., Murata N. (2011). Glycinebetaine protects plants against abiotic stress: mechanisms and biotechnological applications. Plant Cell Environ. 34, 1–20. doi: 10.1111/j.1365-3040.2010.02232.x
Chen Y., Patel N. A., Crombie A., Scrivens J. H., Murrell J. C. (2011). Bacterial flavin-containing monooxygenase is trimethylamine monooxygenase. Proc. Natl. Acad. Sci. U.S.A. 108, 17791–17796. doi: 10.1073/pnas.1112928108
Craciun S., Balskus E. P. (2012). Microbial conversion of choline to trimethylamine requires a glycyl radical enzyme. Proc. Natl. Acad. Sci. U.S.A. 109, 21307–21312. doi: 10.1073/pnas.1215689109
Cree C., Airs R., Archer S. D., Fitzsimons M. F. (2018). Measurement of methylamines in seawater using solid phase microextraction and gas chromatography. Limnol. Oceanogr. Meth. 16, 411–420. doi: 10.1002/lom3.10255
Dall’Osto M., Airs R. L., Beale R., Cree C., Fitzsimons M. F., Beddows D., et al. (2019). Simultaneous detection of alkylamines in the surface ocean and atmosphere of the antarctic sympagic environment. ACS Earth Space Chem. 3, 854–862. doi: 10.1021/acsearthspacechem.9b00028
Dall’Osto M., Ovadnevaite J., Paglione M., Beddows D. C. S., Ceburnis D., Cree C.. (2017). Antarctic sea ice region as a source of biogenic organic nitrogen in aerosols. Sci. Rep. 7, 6047. doi: 10.1038/s41598-017-06188-x
Dawson H. M., Heal K. R., Torstensson A., Carlson L. T., Ingalls A. E., Young J. (2020). Large diversity in nitrogen- and sulfur-containing compatible solute profiles in polar and temperate diatoms. N. Integr. Comparat. Biol. 60, 1401–1413. doi: 10.1093/icb/icaa133
Debevere J., Devlieghere F., Van Sprundel P., De Meulenaer B. (2001). Influence of acetate and CO2 on the TMAO-reduction reaction by Shewanella baltica Int. J. Food Microbiol. 68, 115–123. doi: 10.1016/S0168-1605(01)00484-6
Dembitsky V. M. (1996). Betaine ether-linked glycerolipids: Chemistry and biology. Prog. Lipid Res. 35, 1–51. doi: 10.1016/0163-7827(95)00009-7
Dickson D. M. J., Kirst G. O. (1986). The role of ß-dimethylsulphoniopropionate, glycine betaine and homarine in the osmoacclimation of Platymonas subcordiformis. Planta 167, 536–543. doi: 10.1007/BF00391230
Dickson D. M. J., Kirst G. O. (1987a). Osmotic adjustment in marine eukaryotic algae—the role of inorganic-ions, quarternary ammonium, tertiary sulfonium and carbohydrate solutes. 1. Diatoms and a rhodophyte. New Phytol. 106, 645–655. doi: 10.1111/j.1469-8137.1987.tb00165.x
Dickson D. M. J., Kirst G. O. (1987b). Osmotic adjustment in marine eukaryotic algae-the role of inorganic-ions, quarternary ammonium, tertiary sulfonium and carbohydrate solutes. 2. Prasinophytes and haptophytes. New Phytol. 106, 657–666. doi: 10.1111/j.1469-8137.1987.tb00166.x
Dos Santos J., Iobbi-Nivol C., Couillault C., Giordano G., Méjean V. (1998). Molecular analysis of the trimethylamine N -oxide (TMAO) reductase respiratory system from a Shewanella species. J. Mol. Biol. 284, 421–433. doi: 10.1006/jmbi.1998.2155
Durham B. P., Boysen A. K., Carlson L. T., Groussman R. D., Heal K. R., Cain K. R., et al. (2019). Sulfonate-based networks between eukaryotic phytoplankton and heterotrophic bacteria in the surface ocean. Nat. Microbiol. 4, 1706–1715. doi: 10.1038/s41564-019-0507-5
Durham B. P., Boysen A. K., Heal K., Carlson L. T., Boccamazzo R., Deodato C. R., et al. (2022). Chemotaxonomic patterns in intracellular metabolites of marine microbial plankton. Front. Mar. Sci. 9. doi: 10.3389/fmars.2022.864796
Facchini M. C., Decesari S., Rinaldi M., Carbone C., Finessi E., Mircea M., et al. (2008). Important source of marine secondary organic aerosol from biogenic amines. D. Environ. Sci. Technol. 42, 9116–9121. doi: 10.1021/es8018385
Fan W., Zhang M., Zhang H., Zhang P. (2012). Improved tolerance to various abiotic stresses in transgenic sweet potato (Ipomoea batatas) expressing spinach betaine aldehyde dehydrogenase. PloS One 7, e37344. doi: 10.1371/journal.pone.0037344
Fenizia S., Thume K., Wirgenings M., Pohnert G. (2020). Ectoine from bacterial and algal origin is a compatible solute in microalgae. Mar. Drugs 18, 42. doi: 10.3390/md18010042
Fenizia S., Weissflog J., Pohnert G. (2021). Cysteinolic acid is a widely distributed compatible solute of marine microalgae. Mar. Drugs 19, 683. doi: 10.3390/md19120683
Fessenden R. J., Fessenden J. S. (1994). Organic chemistry. Fifth Edition (Pacific Grove. CA, USA: Brooks Cole), 1122.
Firth E., Carpenter S. D., Sørensen H. L., Collins R. E., Deming J. W. (2016). Bacterial use of choline to tolerate salinity shifts in sea-ice brines. Elementa: Sci. Anthropocene 4, 120. doi: 10.12952/journal.elementa.000120
Fitzsimons M. F., Dawit M., Revitt D. M., Rocha C. (2005). Effects of early tidal inundation on the cycling of methylamines in inter-tidal sediments. Mar. Ecol. Prog. Ser. 294, 51–61. doi: 10.3354/meps294051
Fitzsimons M. F., Millward G. E., Revitt D. M., Dawit M. D. (2006). Desorption kinetics of ammonium and methylamines from estuarine sediments: Consequences for the cycling of nitrogen. Mar. Chem. 101, 12–26. doi: 10.1016/j.marchem.2005.12.006
Fitzsimons M. F., Tilley M., Cree C. H. L. (2023). The determination of volatile amines in aquatic marine systems : A review. Anal. Chim. Acta 1241, 340707. doi: 10.1016/j.aca.2022.340707
Fulda S., Huckauf J., Schoor A., Hagemann M. (1999). Analysis of stress responses in the cyanobacterial strains Synechococcus sp. PCC 7942, Synechocystis sp. PCC 6803, and Synechococcus sp. PCC 7418: osmolyte accumulation and stress protein synthesis. J. Plant Physiol. 154, 240–249. doi: 10.1016/S0176-1617(99)80215-6
Galinski E. A., Oren A. (1991). Isolation and structure determination of a novel compatible solute from the moderately halophilic purple sulfur bacterium Ectothiorhodospira marismortui. Eur. J. Biochem. 198, 593–598. doi: 10.1111/j.1432-1033.1991.tb16055.x
Gebser B., Pohnert. G. (2013). Synchronized regulation of different zwitterionic metabolites in the osmoadaption of phytoplankton. Mar. Drugs 11, 2168–2182. doi: 10.3390/md11062168
Gibb S. W., Hatton A. D. (2004). The occurrence and distribution of trimethylamine-N oxide in Antarctic coastal waters. Mar. Chem. 91, 65–75. doi: 10.1016/j.marchem.2004.04.005
Gibb S. W., Mantoura R. F. C., Liss P. S. (1995). Analysis of ammonia and methylamines in natural waters by flow injection gas diffusion coupled to ion chromatography, anal. Chim. Acta 316, 291–304.
Gibb S. W., Mantoura R. F. C., Liss P. S., Barlow R. G. (1999a). Distributions and biogeochemistries of methylamines and ammonium in the Arabian Sea. Deep Sea Res. Part II Top. Stud. Oceanogr. 46, 593–615. doi: 10.1016/S0967-0645(98)00119-2
Gibb S. W., Mantoura R. F. C., Liss P. S., Barlow R. G. (1999b). Ocean-atmosphere exchange and atmospheric speciation of ammonia and methylamines in the region of the NW Arabian Sea. Global Biogeochem. Cy. 13, 161–177. doi: 10.1029/98GB00743
Gorham J. (1984). Separation of plant betaines and their sulphur analogues by cation exchange high performance liquid chromatography. J. Chromatogr. 287, 345–351. doi: 10.1016/S0021-9673(01)87710-4
Götz F., Longnecker K., Soule M. C. K., Becker K. W., McNichol J., Kujawinski E. B., et al. (2018). Targeted metabolomics reveals proline as a major osmolyte in the chemolithoautotroph Sulfurimonas denitrificans. Microbiol. Open 7, e00586. doi: 10.1002/mbo3.586
Grönberg' L., Lövkvist P., Jönsson J. (1992). Measurement of aliphatic amines in ambient air and rainwater. Chemosphere 24, 1533–1540.
Henrichs S. M., Sugai S. F. (1993). Adsorption of amino-acids and glucose by sediments of Resurrection Bay, Alaska, USA – functional-group effects. Geochim. Cosmochim. Acta 57, 823–835. doi: 10.1016/0016-7037(93)90171-R
Hill R. W. (2022). Quarternary ammonium compounds as candidate photoprotective compounds in reef-building compounds. Front. Mar. Sci. 9. doi: 10.3389/fmars.2022.869739
Hoffmann E. H., Tilgner A., Herrmann H. (2024). An improved multiphase chemistry mechanism for methylamines: significant dimethylamine cloud production. NPJ Clim. Atmos. Sci. 7, 119. doi: 10.1038/s41612-024-00665-7
Hoffmann T., Wensing A., Brosius M., Steil L., Völker U., Bremer E. (2013). Osmotic control of opuA expression in Bacillus subtilis and its modulation in response to intracellular glycine betaine and proline pools. J. Bacteriol. 195, 510–522. doi: 10.1128/JB.01505-12
Holm P. I., Ueland P. M., Kvalheim G., Lien E. A. (2003). Determination of choline, betaine and dimethylglycine in plasma by a high-throughput method based on normal phase chromatography-tandem mass spectrometry. Clin. Chem. 49, 286–294. doi: 10.1373/49.2.286
Huang S., Zuo T., Ni W. (2020). Important roles of glycinebetaine in stabilizing the structure and function of the photosystem II complex under abiotic stresses. Planta 251, 36. doi: 10.1007/s00425-019-0330-z
Hung K., Kleber H. P. (1985). Occurrence and regulation of carnitine dehydrogenase of Pseudomonas species. Wiss Z KarlMarx-Univ Leipzig Math-Nat R 34, 293–296.
Imhoff J. F., Rodriguez-Valera F. (1984). Betaine is the main compatible solute of halophilic eubacteria. J. Bacteriol. 160, 478–479. doi: 10.1128/jb.160.1.478-479.1984
Ivanova E. P., Gorshkova N. M., Bowman J. P., Lysenko A. M., Zhukova N. V., Sergeev A. F., et al. (2004). Shewanella pacifica sp. nov., a polyunsaturated fatty acid-producing bacterium isolated from sea water. Int. J. Syst. Evol. Microbiol. 54, 1083–1087. doi: 10.1099/ijs.0.02993-0
Jameson E., Doxey A. C., Airs R., Purdy K. J., Murrell J. C., Chen. Y. (2016a). Metagenomic data-mining reveals contrasting microbial populations responsible for trimethylamine formation in human gut and marine ecosystems. Microb. Genom. 2, e000080. doi: 10.1099/mgen.0.000080
Jameson E., Fu T., Brown I. R., Paszkiewicz K., Purdy K. J., Frank S., et al. (2016b). Anaerobic choline metabolism in microcompartments promotes growth and swarming of Proteus mirabilis. Environ. Microbiol. 18, 2886–2898. doi: 10.1111/1462-2920.13059
Jameson E., Stephenson J., Jones H., Millard A., Kaster A. K., Purdy K. J., et al. (2018). Deltaproteobacteria (Pelobacter) and Methanococcides are responsible for choline-dependent methanogenesis in a coastal saltmarsh sediment. ISME J. 13, 277–289. doi: 10.1038/s41396-018-0269-8
Johnson W. M., Longnecker K., Kido Soule M. C., Arnold W. A., Bhatia M. P., Hallam S. J., et al. (2020). Metabolite composition of sinking particles differs from surface suspended particles across a latitudinal transect in the South Atlantic. Limnol. Oceanogr. 65, 111–127. doi: 10.1002/lno.11255
Keller M., Matrai P., Kiene R., Bellows W. (2004). Responses of coastal phytoplankton populations to nitrogen additions: dynamics of cell-associated dimethylsulfoniopropionate (DMSP), glycine betaine (GBT), and homarine. Can. J. Fish. Aquat. Sci. 61, 685–699. doi: 10.1139/f04-058
Keller M. D., Kiene R. P., Matrai P. A., Bellows W. K. (1999a). Production of glycine betaine and dimethylsulfoniopropionate in marine phytoplankton. I. Batch cultures. Mar. Biol. 135, 237–248. doi: 10.1007/s002270050621
Keller M. D., Kiene R. P., Matrai P. A., Bellows W. K. (1999b). Production of glycine betaine and dimethylsulfoniopropionate in marine phytoplankton. II. N-limited chemostat cultures. Mar. Biol. 135, 249–257. doi: 10.1007/s002270050622
Kiene R., Slezak D. (2006). Low dissolved DMSP concentrations in seawater revealed by small volume gravity filtration and dialysis sampling. Limnol. Oceanogr. Meth. 4, 80–95. doi: 10.4319/lom.2006.4.80
King G. M. (1984). Metabolism of trimethylamine, choline and glycine betaine by sulfate-reducing and methanogenic bacteria in marine sediments. Appl. Environ. Microbiol. 48, 719–725. doi: 10.1128/aem.48.4.719-725.1984
King G. M. (1988). Methanogenesis from methylated amines in a hypersaline algal mat. Appl. Environ. Microbiol. 54, 130–136. doi: 10.1128/aem.54.1.130-136.1988
Koc H., Mar M. H., Ranasinghe A., Swenberg J. A., Zeisel S. H. (2002). Quantitation of choline and its metabolites in tissues and foods by liquid chromatography/electrospray ionization-isotope dilution mass spectrometry. Anal. Chem. 74, 4734–4740. doi: 10.1021/ac025624x
Kondo Y., Sakamoto A., Nonaka H., Hayashi H., Saradhi P. P., Chen T. H., et al. (1999). Enhanced tolerance to light stress of transgenic Arabidopsis plants that express the codA gene for a bacterial choline oxidase. Plant Mol. Biol. 40, 279–288. doi: 10.1023/A:1006121821883
Leblanc L., Gouffi K., Leroi F., Hartke A., Blanco C., Auffray Y., et al. (2001). Uptake of choline from salmon flesh and its conversion to glycine betaine in response to salt stress in Shewanella putrefaciens. Int. J. Food Microbiol. 65, 93–103. doi: 10.1016/S0168-1605(00)00516-X
Lee C., Olson B. L. (1984). Dissolved, exchangeable and bound aliphatic amines in marine sediments: initial results, org. Geochem 6, 259–263.
Leng C., Kish J. D., Roberts J. E., Dwebi I., Chon N., Liu Y. (2015). J. Phys. Chem. A 119, 8884–8891. doi: 10.1021/acs.jpca.5b05174
Li M., Li Z., Li S., Guo S., Meng Q., Li G., et al. (2014). Genetic engineering of glycine betaine biosynthesis reduces heat-enhanced photoinhibition by enhancing antioxidative defense and alleviating lipid peroxidation in tomato. Plant Mol. Biol. Rep. 32, 42–51. doi: 10.1007/s11105-013-0594-z
Li D., Wang M., Zhang T., Chen X., Li C., Liu Y., et al. (2021). Glycinebetaine mitigated the photoinhibition of photosystem II at high temperature in transgenic tomato plants. Photosyn. Res. 147, 301–315. doi: 10.1007/s11120-020-00810-2
Liang C., Zhang X. Y., Luo Y., Wang G. P., Zou Q., Wang W. (2009). Overaccumulation of glycine betaine alleviates the negative effects of salt stress in wheat. Russ. J. Plant Physiol. 56, 370–376. doi: 10.1134/S1021443709030108
Lichty K. E. B., Loughran R. M., Ushijima B., Richards G. P., Boyd E. F. (2024). Osmotic stress response of the coral and oyster pathogen Vibrio coralliilyticus: acquisition of catabolism gene clusters for the compatible solute and signaling molecule myo-inositol. Appl. Environ. Microbiol. 90, e00920–e00924. doi: 10.1128/aem.00920-24
Lidbury I., Kröber E., Zhang Z., Zhu Y., Murrell J. C., Chen Y., et al. (2016). A mechanism for bacterial transformation of dimethylsulfide to dimethylsulfoxide: a missing link in the marine organic sulfur cycle. Environ. Microbiol. 18, 2754–2766. doi: 10.1111/1462-2920.13354
Lidbury I., Mausz M., Scanlan D. J., Chen Y. (2017). Identification of dimethylamine monooxygenase in marine bacteria reveals a metabolic bottleneck in the marine methylated amine degradation pathway. ISME J. 11, 1592–1601. doi: 10.1038/ismej.2017.31
Lidbury I., Murrell J. C., Chen Y. (2014). Trimethylamine N-oxide metabolism by abundant marine heterotrophic bacteria. P. Natl. Acad. Sci. U.S.A. 111-, 2715. doi: 10.1073/pnas.1317834111
Lidbury I., Murrell J. C., Chen Y. (2015). Trimethylamine and trimethylamine N-oxide are supplementary energy sources for a marine heterotrophic bacterium: Implications for marine carbon and nitrogen cycling. ISME J. 9, 760–769. doi: 10.1038/ismej.2014.149
Mackay M. A., Norton R. S., Borowitzka L. J. (1984). Organic osmoregulatory solutes in cyanobacteria. J. Gen. Microbiol. 130, 2177–2191. doi: 10.1099/00221287-130-9-2177
Mausz M. A., Airs R. L., Dixon J. L., Widdicombe C. E., Tarran G. A., Polimene L., et al. (2022). Microbial uptake dynamics of choline and glycine betaine in coastal seawater. Limnol. Oceanogr. 67, 1052–1064. doi: 10.1002/lno.12056
Mausz M. A., Chen Y. (2019). Microbiology and ecology of methylated amine metabolism in the marine environment. Curr. Issues Mol. Biol. 33, 133–148. doi: 10.21775/cimb.033.133
McParland E. L., Alexander H., Johnson W. M. (2021). The osmolyte ties that bind: genomic insights into synthesis and breakdown of organic osmolytes in marine microbes. Front. Mar. Sci. 8. doi: 10.3389/fmars.2021.689306
Meng X. L., Gao X., Si Y. M., Xu L. L., Guo L. Z., Lu W. D. (2022). Role of carnitine in adaptation of Chromohalobacter salexigens DSM 3043 and its mutants to osmotic and temperature stress in defined medium. Extremophiles 26, 28. doi: 10.1007/s00792-022-01276-x
Meyer M., Granderath K., Andreesen J. R. (1995). Purification and characterization of protein PB of betaine reductase and its relationship to the corresponding proteins glycine reductase and sarcosine reductase from Eubacterium acidaminophilum. Eur. J. Biochem. 234, 184–191. doi: 10.1111/j.1432-1033.1995.184_c.x
Monnich K., Hanschmann H., Kleber H. P. (1995). Utilization of D-carnitine by pseudomonas sp. AK 1. FEMS Microbiol. Lett. 132, 51–55. doi: 10.1111/j.1574-6968.1995.tb07809.x
Mäkelä P. S. A., Jokinen K., Himanen K. (2019). “Roles of endogenous glycinebetaine in plant abiotic stress responses”. In: Hossain M., Kumar V., Burritt D., Fujita M., Mäkelä P. (eds) Osmoprotectant-mediated abiotic stress tolerance in plants. Springer, Cham. doi: 10.1007/978-3-030-27423-8_7
Noell S., Giovannoni S. (2019). SAR11 bacteria have a high affinity and multifunctional glycine betaine transporter. Environ. Microbiol. 21, 2559–2575. doi: 10.1111/1462-2920.14649
Ongagna-Yhombi S. Y., Boyd E. F. (2013). Biosynthesis of the osmoprotectant ectoine, but not glycine betaine, is critical for survival of osmotically stressed Vibrio parahaemolyticus cells. Appl. Environ. Microbiol. 79, 5038–3049. doi: 10.1128/AEM.01008-13
Oremland R. S., Marsh L. M., Polcin S. (1982). Methane production and simultaneous sulphate reduction in anoxic, salt marsh sediments. Nature 296, 143–145. doi: 10.1038/296143a0
Oren A. (1990). Formation and breakdown of glycine betaine and trimethylamine in hypersaline environments. Antonie van Leeuwenhoek 58, 291–298. doi: 10.1007/BF00399342
Oufir M., Schulz N., Vallikhan P. S. S., Wilhelm E., Burg K., Hausman J. F., et al. (2009). Simultaneous measurement of proline and related compounds in oak leaves by high-performance ligand-exchange chromatography and electrospray ionization mass spectrometry for environmental stress studies. J. Chromatogr. A 1216, 1094–1099. doi: 10.1016/j.chroma.2008.12.030
Papageorgiou G. C., Murata N. (1995). The unusually strong stabilizing effects of glycine betaine on the structure and function of the oxygen-evolving photosystem-II complex. Photosynth. Res. 44, 243–252. doi: 10.1007/BF00048597
Pflughoeft K. J., Kierek K., Watnick P. I. (2003). Role of Ectoine in Vibrio cholerae osmoadaptation. Appl. Environ. Microbiol. 69, 5919–5927. doi: 10.1128/AEM.69.10.5919-5927.2003
Prasad K. V. S. K., Saradhi P. P. (2004). Enhanced tolerance to photoinhibition in transgenic plants through targeting of glycine betaine biosynthesis into the chloroplasts. Plant Sci. 166, 1197–1212. doi: 10.1016/j.plantsci.2003.12.031
Rajakovich L. J., Fu B., Bollenbach M., Balskus E. P. (2021). Elucidation of an aerobic pathway for metabolism of L-carnitine-derived butyrobetaine to trimethylamine in human gut bacteria. P. Natl. Acad. Sci. U.S.A. 118, e2101498118. doi: 10.1073/pnas.2101498118
Raymond J. A., Plopper G. E. (2002). A bacterial TMAO transporter. Comp. Biochem. Physiol. B Biochem. Mol. Biol. 133, 29–34. doi: 10.1016/S1096-4959(02)00097-0
Reed R. H., Borowitzka L. J., Mackay M., Chudek J. A., Foster R., Warr S. R. C., et al. (1986). Organic solute accumulation in osmotically stressed cyanobacteria. FEMS Microbiol. Lett. 39, 51–56. doi: 10.1111/j.1574-6968.1986.tb01842.x
Riccobono F., Schobesberger S., Scott C. E., Dommen J., Ortega I. K., Rondo L., et al. (2014). Oxidation products of biogenic emissions contribute to nucleation of atmospheric particles. Science 344, 717–721. doi: 10.1126/science.1243527
Rivero R. M., Mestre T. C., Mittler R., Rubio F., Garcia-Sanchez F., Martinez V. (2014). Stress combination in tomato plants. Plant Cell Environ. 37, 1059–1073. doi: 10.1111/pce.12199
Roberts M. F. (2005). Organic compatible solutes of halotolerant and halophilic microorganisms. Saline Syst. 1, 5. doi: 10.1186/1746-1448-1-5
Roman D., Meisinger P., Guillonneau R., Peng C. C., Peltner L. K., Jordan P. M., et al. (2024). Structure revision of a widespread marine sulfonolipid class based on isolation and total synthesis. Angew Chem. Int. Ed Engl. 63, e202401195. doi: 10.1002/anie.202401195
Sacks J. S., Heal K. R., Boysen A. K., Carlson L. T., Ingalls A. E. (2022). Quantification of dissolved metabolites in environmental samples through cation-exchange solid-phase extraction paired with liquid chromatography–mass spectrometry. Limnol. Oceanogr. Meth. 20, 683–700. doi: 10.1002/lom3.10513
Sakamoto A., Murata N. (2002). The role of glycine betaine in the protection of plants from stress: clues from transgenic plants. Plant Cell Environ. 25, 163–171. doi: 10.1046/j.0016-8025.2001.00790.x
Sleator R. D., Hill C. (2002). Bacterial osmoadaptation: the role of osmolytes in bacterial stress and virulence. FEMS Microbiol. Rev. 26, 49–71. doi: 10.1016/S0168-6445(01)00071-7
Smith A. F., Silvano E., Päuker O., Guillonneau R., Quareshy M., Murphy A., et al. (2021). A novel class of sulfur-containing aminolipids widespread in marine roseobacters. ISME J. 15, 2440–2453. doi: 10.1038/s41396-021-00933-x
Spielmeyer A., Gebser B., Pohnert G. (2011). Dimethylsulfide sources from microalgae: Improvement and application of a derivitization-based method for the detemination of dimethylsulfoniopropionate and other zwitterionic osmolytes in phytoplankton. Mar. Chem. 124, 48–56. doi: 10.1016/j.marchem.2010.12.001
Spielmeyer A., Pohnert G. (2012). Influence of temperature and elevated carbon dioxide on the production of dimethylsulfoniopropionate and glycine betaine by marine phytoplankton. Mar. Environ. Res. 73, 62–69. doi: 10.1016/j.marenvres.2011.11.002
Storey R., Wyn Jones R. G. (1977). Quaternary ammonium compounds in plants in relation to salt resistance. Phytochemistry 16, 447–453. doi: 10.1016/S0031-9422(00)94326-7
Sun J., Steindler L., Thrash J. C., Halsey K. H., Smith D. P., Carter A. E., et al. (2011). One carbon metabolism in SAR11 pelagic marine bacteria. PloS One 6, e23973. doi: 10.1371/journal.pone.0023973
Tilgner A., Schaefer T., Alexander B., Barth M., Collett J. L. Jr., Fahey K. M., et al. (2021). Acidity and the multiphase chemistry of atmospheric aqueous particles and clouds. Atmos. Chem. Phys. 21, 13483–13536. doi: 10.5194/acp-21-13483-2021
Van Neste A., Duce R. A., Lee C. (1987). Methylamines in the marine atmosphere. Geophysical Res. Lett. 7, 711–714. doi: 10.1029/GL014i007p00711
Van Pinxteren M., Fomba K., van Pinxteren D., Triesch N., Hoffmann E., Cree C., et al. (2019). Aliphatic amines at the Cape Verde Atmospheric Observatory: abundance, origins and sea-air fluxes. Atmos. Environ. 203, 183–195. doi: 10.1016/j.atmosenv.2019.02.011
Vogel G., Woznica M., Gfeller H., Müller C., Stämpfli A. A., Jenny T. A., et al. (1990). 1(3),2-Diacylglyceryl-3(1)-O-2′-(hydroxymethyl)(N,N,N,-trimethyl)-β-alanine (DGTA): A novel betaine lipid from Ochoromonas danica (Chrysophyceae). Chem. Phys. Lipids 52, 99–109. doi: 10.1016/0009-3084(90)90154-J
Wang G. P., Hui Z., Li F., Zhao M.-R., Zhank J., Wang W. (2010). Improvement of heat and drought photosynthetic tolerance in wheat by overaccumulation of glycinebetaine. Plant Biotechnol. Rep. 4, 213–222. doi: 10.1007/s11816-010-0139-y
Wang X. C., Lee C. (1990). The distribution and adsorption behavior of aliphatic-amines in marine and lacustrine sediments. Geochim. Cosmochim. Acta 54, 2759–2774. doi: 10.1016/0016-7037(90)90010-I
Wang X. C., Lee C. (1993). Adsorption and desorption of aliphatic amines, amino acids and acetate by clay minerals and marine sediments. Mar. Chem. 44, 1–23. doi: 10.1016/0304-4203(93)90002-6
Wang Y., Liu S., Zhang H., Zhao Y., Zhao H., Liu H. (2014). Glycine betaine application in grain filling wheat plants alleviates heat and high light-induced photoinhibition by enhancing the psbA transcription and stomatal conductance. Acta Physiol. Plant 36, 2195–2202. doi: 10.1007/s11738-014-1596-7
Wright R. R., Hobbie J. E. (1966). Use of glucose and acetate by bacteria and algae in aquatic ecosystems. Ecology 47, 447–464. doi: 10.2307/1932984
Yancey P. H. (2005). Organic osmolytes as compatible, metabolic and counteracting cytoprotectants in high osmolarity and other stresses. J. Exp. Biol. 208, 2819–2830. doi: 10.1242/jeb.01730
Yancey P. H., Clark M. E., Hand S. C., Bowlus R. D., Somero G. N. (1982). Living with water stress: evolution of osmolyte systems. Science 217, 1214–1222. doi: 10.1126/science.7112124
Yang X. H., Lee C., Scranton M. I. (1993). Determination of nanomolar concentrations of individual dissolved low molecular weight amines and organic acids in seawater. Anal. Chem. 65, 572–576. doi: 10.1021/ac00053a014
Yang C. C., Packman L. C., Scrutton N. S. (1995). The primary structure of Hyphomicrobium X dimethylamine dehydrogenase. Relationship to Trimethylamine Dehydrogenase and Implications for Substrate Recognition. Eur. J. Biochem. 232, 264–271. doi: 10.1111/j.1432-1033.1995.tb20808.x
Yin Q., Zhang W., Li X., Zhou L., Qi X., Zhang C., et al. (2019). Contribution of trimethylamine N-oxide on the growth and pressure tolerance of deep-sea bacteria. J. Ocean. Limnol. 37, 210–222. doi: 10.1007/s00343-019-7377-9
Zecher K., Hayes K. R., Philipp B. (2020). Evidence of interdomain ammonium cross-feeding from methylamine- and glycine betaine-degrading Rhodobacteraceae to diatoms as a widespread interaction in the marine phycosphere. Front. Microbiol. 11. doi: 10.3389/fmicb.2020.533894
Zhang W. J., Zhang C., Zhou S., Li X. G., Mangenot S., Fouteau S., et al. (2021). Comparative genomic analysis of obligately piezophilic Moritella yayanosii DB21MT-5 reveals bacterial adaptation to the challenger deep, Mariana Trench. Microb. Genom. 7, 7. doi: 10.1099/mgen.0.000591
Keywords: amines, marine, biogeochemistry, microbial pathways, analytical techniques, sea-air exchange
Citation: Fitzsimons MF, Airs R and Chen Y (2024) The occurrence and biogeochemical cycling of quaternary, ternary and volatile amines in marine systems. Front. Mar. Sci. 11:1466221. doi: 10.3389/fmars.2024.1466221
Received: 17 July 2024; Accepted: 06 November 2024;
Published: 20 December 2024.
Edited by:
Nicholas David Ward, Pacific Northwest National Laboratory (DOE), United StatesReviewed by:
Angie Boysen, Pacific Lutheran University, United StatesRichard Hill, Michigan State University, United States
Copyright © 2024 Fitzsimons, Airs and Chen. This is an open-access article distributed under the terms of the Creative Commons Attribution License (CC BY). The use, distribution or reproduction in other forums is permitted, provided the original author(s) and the copyright owner(s) are credited and that the original publication in this journal is cited, in accordance with accepted academic practice. No use, distribution or reproduction is permitted which does not comply with these terms.
*Correspondence: Mark F. Fitzsimons, bWZpdHpzaW1vbnNAcGx5bW91dGguYWMudWs=
†ORCID: Mark F. Fitzsimons, orcid.org/0000-0002-6443-6087
Ruth Airs,
orcid.org/0000-0003-0861-742X
Yin Chen, orcid.org/0000-0002-0367-4276