- 1Australian Antarctic Program Partnership, University of Tasmania, Hobart, TAS, Australia
- 2Institute for Marine and Antarctic Studies, University of Tasmania, Hobart, TAS, Australia
- 3Australian Antarctic Division, Kingston, TAS, Australia
- 4Central Science Laboratory, University of Tasmania, Hobart, TAS, Australia
In large areas of the Southern Ocean, iron limits phytoplankton production. Although biologically mediated iron recycling has been studied for the higher trophic-level whales and the lower trophic-level krill, less is known of the numerically abundant seabirds foraging in Antarctic waters. In this study, we estimate the magnitude of iron recycled by two Antarctic breeding seabirds, the Adélie and emperor penguins, across the austral spring and summer in the Prydz Bay region, East Antarctica. Their contribution to iron recycling and associated pathways differs in line with their contrasting life history strategies (summer and winter breeding) and their breeding habitat (land and fast ice). We consider their breeding cycle in relation to their terrestrial activities compared to foraging periods at sea. High iron concentration (~419 mg kg−1) in guano of both penguin species suggests that they are a source of regenerated iron. Breeding emperor penguins supplied an average of 237 μmol iron m−2 day−1 on the fast ice that they breed on that eventually ends in the ocean when the ice melts completely in summer (November–February). During their foraging trips, the adult emperor penguins contribute between 7 × 10−5 and 4 × 10−4 μmol iron m−2 day−1, as their foraging ranges increase over the breeding season. In contrast, breeding Adélie penguins supplied between 254 and 1,243 μmol iron m−2 day−1 whilst at their colony, with a fraction of guano entering the ocean via meltwater flowing into the ocean. The flux decreases to 2 × 10−3 to 6 × 10−2 μmol iron m−2 d−1, whilst they are foraging. Our study finds that penguins redistribute a large flux of iron onto their colonies, which may enter the adjacent water through sea ice melt and facilitated through katabatic winds. Despite their high abundance in Prydz Bay, the contribution of penguins to iron flux during their foraging periods is minor, due to the enormous foraging range being covered. Further research into the bioavailability of iron by marine organisms coupled with parallel measurements of seawater iron concentration and phytoplankton uptake experiments will be invaluable in refining iron budgets in both this region and other hotspots along the Antarctic coast where higher trophic-level animals are abundant.
Introduction
Phytoplankton growth in one-third of the global ocean is limited by the availability of iron, an essential trace nutrient (Moore et al., 2004). This is important for ecosystem functioning and productivity as phytoplankton form the base of ecosystem food webs. The Southern Ocean is the largest and most climatically important iron-limited region as it is responsible for ~40% of anthropogenic carbon dioxide (CO2) uptake by the ocean (Takahashi et al., 2009; Landschutzer et al., 2015). At the basin scale, deep winter mixing coupled with year-round diapycnal diffusion represents the main physical processes that supply iron-rich subsurface waters to the surface ocean (Tagliabue et al., 2014). In addition to the subsurface reservoir, localised sources such as hydrothermal vents, especially around mid-ocean ridges (Tagliabue et al., 2010; Holmes et al., 2017), dust (Bowie et al., 2015), weathering of shelf sediments (Sedwick et al., 2008; Bowie et al., 2009), and the melting of glaciers (Lin et al., 2011), icebergs (Smith et al., 2007; Lin et al., 2011) and ice shelves (Herraiz-Borreguero et al., 2016) around the Antarctic coast all contribute to the dissolved iron inventory.
The seasonal formation and melting of sea ice acts as an important vector for iron storage and recycling that significantly influences phytoplankton growth in Antarctic waters (Lannuzel et al., 2011; van der Merwe et al., 2011). Bacterial remineralisation and viral lysis are two biologically mediated processes that recycle iron within the water column (Poorvin et al., 2004; Dalbec and Twining, 2009; Bowie et al., 2015). However, less is known about the biologically mediated recycling of iron by higher trophic levels, with previous studies focussing on lower trophic zooplankton such as salps (Salpa thompsoni) and Antarctic krill (Euphausia superba), and higher trophic baleen whales, which migrate to the productive Southern Ocean waters to feed during summer (Tovar-Sanchez et al., 2007, 2009; Nicol et al., 2010; Schmidt et al., 2011; Ratnarajah et al., 2016; Schmidt et al., 2016; Cabanes et al., 2017).
One component of the ecosystem that has potential to redistribute nutrients over large areas are seabirds (Zhu et al., 2014; Wing et al., 2014, 2017; Shatova et al., 2017; Otero et al., 2018; Belyaev et al., 2023). In contrast to whales, seabirds are central-place foragers during the breeding season, with foraging trips emanating from their breeding sites. High densities of birds can deposit large amounts of guano in coastal and terrestrial ecosystems (Otero et al., 2018; Belyaev et al., 2023). Numerically abundant, seabirds foraging in Antarctic waters include those that breed along the Antarctic coastline, offshore islands and ice shelves, and those that forage in the Southern Ocean but breed outside of Antarctica (e.g., Arctic terns and shearwaters; Perron et al., 2010; Raymond et al., 2010).
In this study, we examine the contribution of iron from two seabird species that breed exclusively in Antarctica—the Adélie (Pygoscelis adeliae) and emperor (Aptenodytes forsteri) penguins with their contrasting breeding seasons over summer and winter respectively. Adélie penguins and most other Antarctic seabird species breed on ice-free land along the continental coastline and offshore islands (Southwell et al., 2017), with obvious layers of guano accumulated over generations at their nesting sites (Gao et al., 2018). When fast ice surrounds their colonies, the penguins defecate on the ice during their traverse to reach the open water for foraging (Emmerson and Southwell, 2008) and in the water within their several hundred-kilometer foraging ranges (Ballance et al., 2009; Clarke et al., 2006). In contrast, emperor penguins breed almost exclusively on fast ice (sea ice attached to the continent) with guano stains so prominent that they can be seen from space (Fretwell and Trathan, 2009). At most sites, the sea ice on which emperor penguins breed melts in the peak of summer, releasing the deposited guano into the ocean.
For Antarctic land- and ice-breeding seabirds, there are several pathways through which iron can enter the oceanic system from the deposition of their guano. These pathways depend on the bird’s life cycle, the cycle of sea ice growth and retreat, and the timing of these in relation to when iron is needed for phytoplankton growth. When considering the iron cycle, iron from penguin guano can be supplied directly into the seawater when they are foraging, or as run-off from the colonies via snow or sea ice meltwater. Specifically, iron recycling pathways include (1) defecation at land-based breeding colonies (e.g., Adélie penguins) of which a fraction can be transported to the ocean via meltwater from melting snow forming substantial runoff through the nesting areas into the ocean immediately surrounding the colonies, (2) strong katabatic winds may supply a fraction of the guano to nearby seawater through atmospheric transport, (3) deposition on the ice or in the water during foraging trips which either enters the water immediately or is released when the ice melts, and (4) in the case of emperor penguins, guano deposited on the fast ice at their breeding site over winter enters the water when the sea ice on which they breed melts during the following summer months. Understanding seabird colony attendance patterns and activity as well as the area over which they are foraging and breeding is therefore critical in determining their contribution of nutrients into the ecosystem.
Here, we examine whether Adélie and emperor penguins are an important source of recycled iron, which could influence phytoplankton productivity across Prydz Bay. We chose this area because it has an abundant Antarctic krill population (Nicol et al., 2000), is home to the largest Adélie penguin metapopulation in East Antarctica (Southwell et al., 2017), and contains a large emperor penguin colony (Fretwell et al., 2012). This area includes multiple important bird areas based on the large and species-diverse seabird populations (Harris et al., 2015). We measure the concentration of iron in fresh guano and use these values in conjunction with published estimates of population size, foraging area, and the bird’s attendance patterns at their colonies to estimate their contribution to iron recycling across the austral spring and summer (mid-October to mid-February). Our calculations incorporate differences associated with life history stages and activities at their colonies and whilst foraging in the ocean. To quantify the flux of iron supplied over spring and summer, we used field-derived data and published literature values to take into account species-specific differences in daily activity and the well-known changes in their foraging distribution during the breeding season (Wienecke et al., 2004; Kirkwood and Robertson 1997; Kato et al., 2003; Clarke et al., 2006; Kokubun et al., 2021).
Methods
Study sites and species
Our focus here is on quantifying the contribution of recycled iron by Adélie and emperor penguins breeding in the Prydz Bay area in East Antarctica extending from 72°E to 81°E (Figure 1). Guano from emperor penguins from Auster Rookery was also collected but only used to determine if guano iron concentrations were similar between sites, as their foraging region is outside the Prydz Bay region (Figure 1) and hence not included in flux calculations. Flux estimation used data on penguin (1) population size, (2) activity patterns in terms of presence at the colony or at sea foraging, (3) foraging ranges, and (4) measurements of iron content in fresh guano. We sampled guano from emperor and Adélie penguins and used data from long-term monitoring in Prydz Bay or nearby East Antarctic sites to determine their population size, activity patterns, and foraging range (Table 1). The spring/summer period was selected because it coincides with the phytoplankton bloom period as seasonal changes in light limit phytoplankton production at other times of the year. In this study, fluxes are calculated from 15 October to 15 February (inclusive), although we acknowledge that penguins feed and defecate on fast ice, land, and at sea at other times of the year based on their breeding, foraging, and moulting patterns (Figure 2A). Our focal period covers the entire Adélie penguin breeding cycle (Emmerson et al., 2011) but is at the end of the emperor penguin breeding cycle (Wienecke and Robertson, 1997) and includes the annual injection of fledglings into the marine environment departing the colonies for the first time on their maiden journeys (Wienecke et al., 2010).
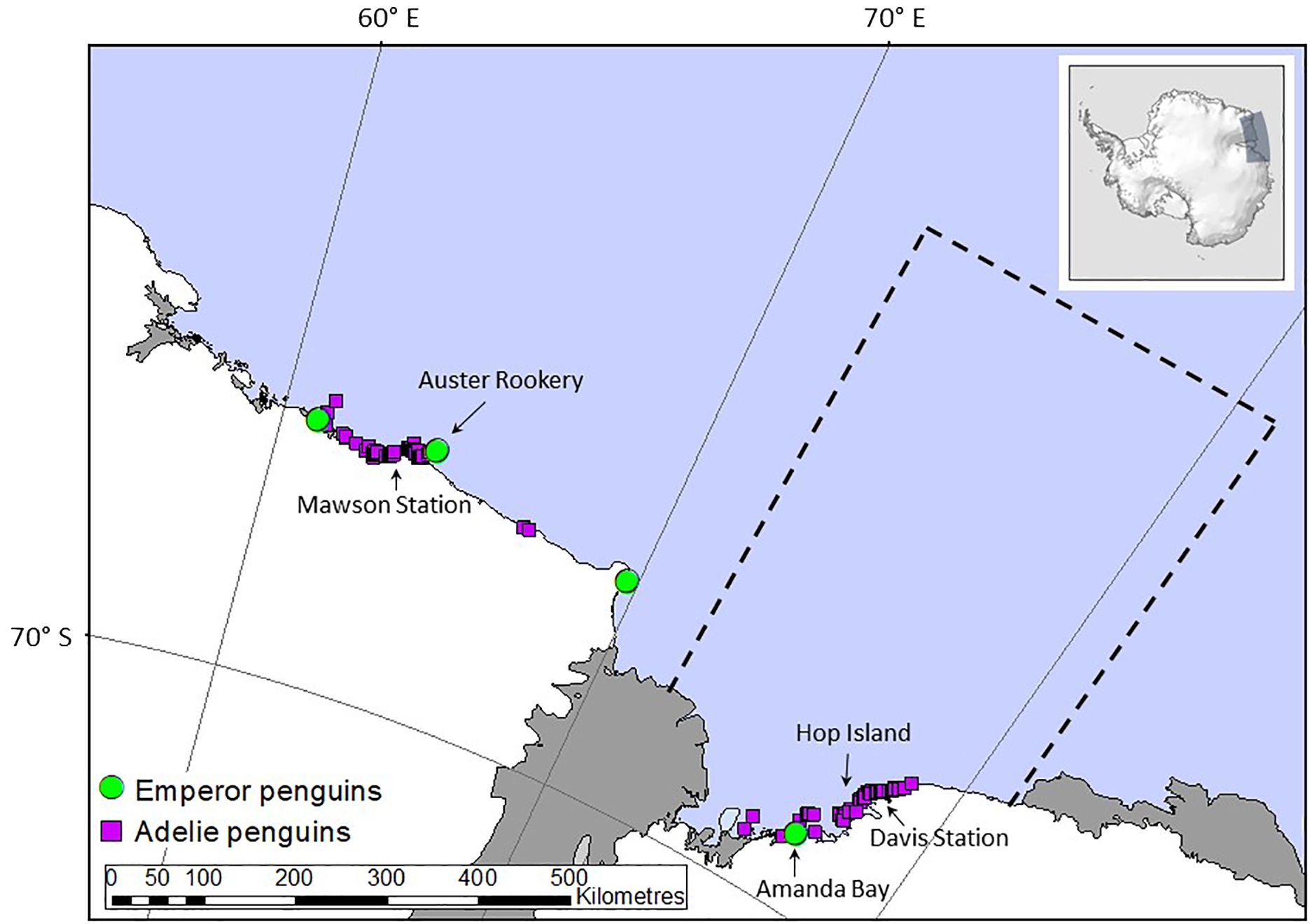
Figure 1. Location of Adélie (purple squares) and emperor (green circles) penguins breeding sites. The black arrows indicate where the guano samples were collected from Hop Island, Amanda Bay and Auster Rookery. The black dashed line marks the location of Prydz Bay where the flux of iron from penguins were calculated. This area is aligned with the breeding penguins foraging range during summer based on data from Hop Island (Adélie penguins). We only have quantitative information on emperor penguin fledgling foraging ranges within Prydz Bay from Amanda Bay, and not the adults. However, emperor penguin adult foraging ranges were available from Auster Rookery, hence we used the extent of their foraging ranges from emperor penguin adults from Auster Rookery and assumed a similar extent within Prydz Bay. Note that arrows also indicate Mawson and Davis stations but no guano samples were collected from these locations. Inset on top right indicated study location in Antarctica.
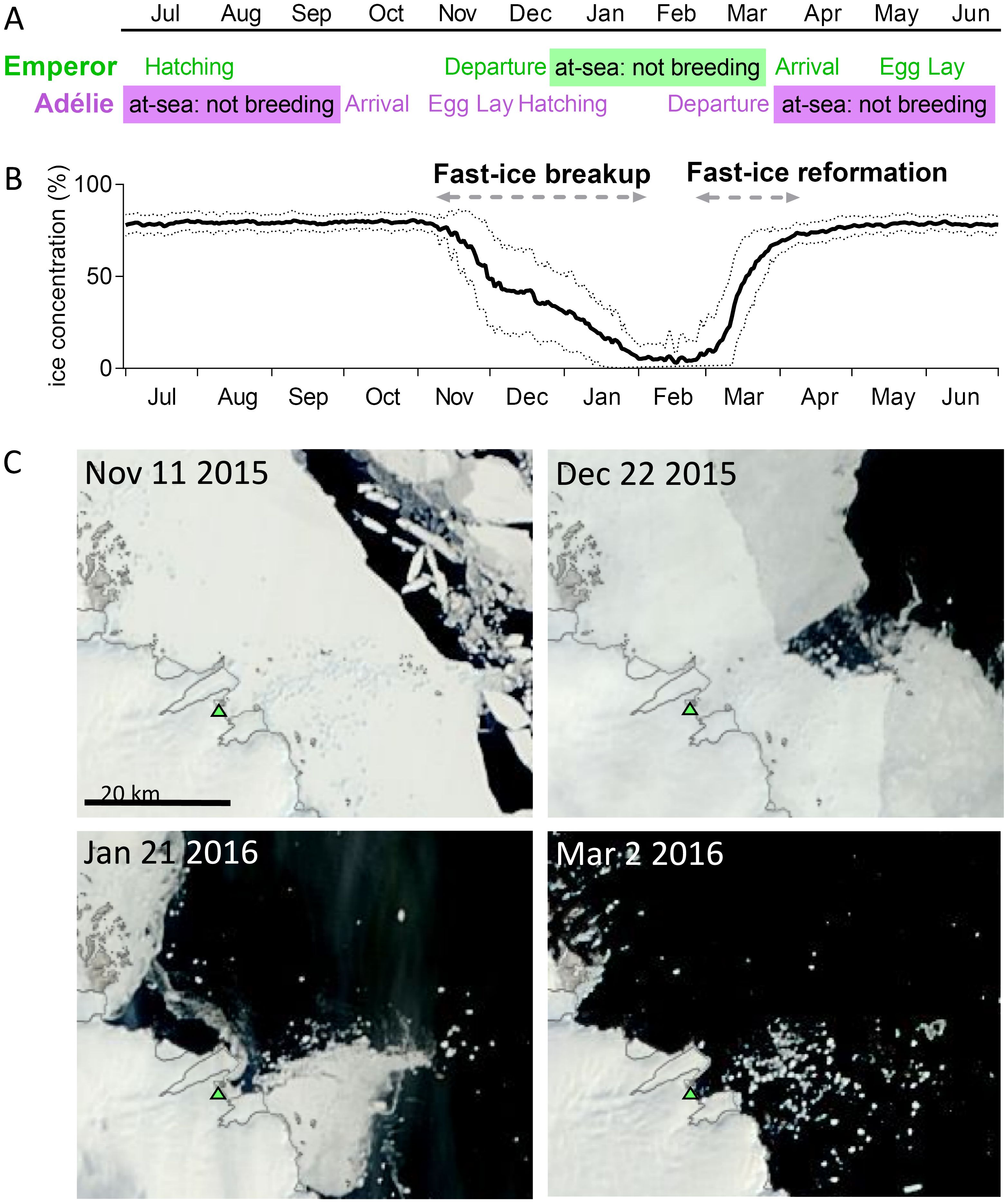
Figure 2. Seasonal breeding cycle for East Antarctic breeding Adélie and emperor penguin and sea-ice phenology in the Amanda Bay area: (A) breeding cycle of winter breeding Emperor and summer breeding Adélie penguins across the year (B) annual cycle of sea-ice phenology including melting and re-formation of sea ice around the Amanda Bay emperor penguin colony with mean across years 1979–2017 indicated by solid line and standard deviation in dashed lines, and (C) satellite images between November and March showing the breakup of the sea ice at Amanda Bay (green triangle) and the surrounding area. Satellite images obtained from https://earthdata.nasa.gov/labs/worldview/.
Across the Prydz Bay region, there were an estimated 551,058 occupied Adélie penguin nests spread across 62 breeding sites (Table 1 and Figure 1) in 2010, which translates to over a million breeding birds nesting over an area covering approximately 500,000 m2 on land (Southwell et al., 2017). Approximately 81,400 pairs of these Adélie penguins were breeding on Hop Island (Southwell et al., 2017) in the Rauer Group (68° 49’S, 77° 42’E) where fresh guano was collected from adult Adélie penguins (n = 10) and chicks (n = 9) in January 2016. Amanda Bay (69°15’S, 76°49’E) is the only emperor penguin rookery in Prydz Bay and is located approximately 100 km to the southwest of Davis Station between Hovde Glacier and Flatnes Ice Tongue (68°35′S, 77°58′E, Figure 1). The Amanda Bay emperor penguin colony numbered 6,831 breeding pairs in 2009 and is located on the fast ice in the southwestern part of the bay, covering an area of 7,315 m2 of fast ice (Fretwell et al., 2012). Guano from emperor penguins were collected at Amanda Bay (n = 6) within Prydz Bay in December 2015 and from Auster Rookery (n = 6), Mac. Roberston Land in November 2015 located approximately 50 km to the east of Mawson Station (63°58’S, 67°23’E), thus providing a comparison between guano iron concentration collected from different breeding populations. The Auster emperor penguin rookery numbered 7,855 breeding pairs in 2009 (Fretwell et al., 2012).
Penguin colony attendance
Understanding the life cycle of seabirds, and particularly their attendance at colonies, on the fast ice and when foraging in the water, is crucial for calculating the magnitude of iron that could be supplied by penguins into nearby waters (Otero et al., 2018). Seabirds breeding in Antarctica have a regular breeding cycle that is primarily dictated by the necessity to reproduce whilst conditions are suitable (Figure 2).
Although Adélie and emperor penguins breed during summer and winter, respectively, both species have predictable annual cycles of arrival, egg lay, incubation, chick guard, chick crèche, the departure of adults from the colonies to forage prior to moult, and the departure of fledglings from the colonies to commence their winter migration (Figure 2A and Table 1). Emperor penguins typically breed on fast ice between April and December, whereas Adélie penguins breed on ice-free breeding sites around the continent from mid-October to mid-February. The emperor penguin breeding cycle therefore has to be complete by the time the fast ice that the birds breed on melts, which is between November and March each year at Amanda Bay (Figures 2B, C).
Adélie penguins arrive at their colonies in mid-October and remain until ~21 November when the females depart the colonies to forage at sea, with one parent remaining with the chick at the colony during the guard period (i.e., 50% of population at colony between ~22 November and ~15 January, Table 1). Once the chick’s crèche, on average 80% of the breeding population is then at sea at any given point in time from ~16 January to ~15 February (Emmerson et al., 2011; Southwell et al., 2015). Adélie penguin chicks fledge from mid-February onwards (Emmerson et al., 2011), and the adults depart the colonies from early February to forage in preparation for their moult (Emmerson et al., 2019). We used an average value of 0.9 chicks per occupied nest based on data from Magnetic Island in Prydz Bay (Whitehead et al., 1990).
Emperor penguins arrive at their rookeries in April. Egg laying occurs in mid-May after which the females go on a long foraging trip leaving the males to incubate the egg (~June to ~15 July) (Wienecke and Robertson, 1997). Males leave the colonies in July/August once the females return, and chick rearing occurs from mid-July through December (Wienecke and Robertson, 1997). Chicks fledge by ~7 December, after which the adults are at sea to prepare for moulting, which starts mid-January (Wienecke and Robertson, 1997). During the spring/summer period, 20% of the emperor penguin population remains at the colony and 80% forage at sea from mid-October until approximately the 20 December. Approximately 100% of the population is then at sea from 20 December to 22 January (Kooyman et al., 2000). From ~22 January until mid-February, the Emperors are at their moulting sites generally along the coastline (Kooyman et al., 2000, Wienecke, Kirkwood, and Robertson 2004). We used an average breeding success of 69% based on studies at Taylor Glacier in the Mac. Robertson Land area (Robertson et al., 2013).
We use published dates for key breeding phenology events such as arrival, egg lay (incubation), chick hatch, chick crèche, and departure for their pre-moult hyperphagia to determine the ratio and number of breeding penguins (i.e., breeding pairs × 2) present in the colony or foraging at sea for each species (Table 1). The number of chicks at a breeding sites was estimated from published breeding success values applied to colony population size to allow their inclusion in the production of guano. Dates and literature sources are summarised in Table 1.
Foraging area
We calculated the total area of the at sea foraging range of Adélie and emperor penguins breeding across Prydz Bay. For the Adélie penguins which have multiple breeding sites across this area, we used the method developed by Critchley et al. (2018) and Handley et al. (2021) for central-place foragers as a pragmatic means of projecting at-sea foraging distribution when tracking data are not available from every colony. The approach delineates the foraging range for central-place foragers by a sector of a circle extending from the breeding site location and with the extent determined by a maximum foraging distance, a central foraging direction, and a span of foraging directions to either side of the central direction.
Adélie penguins foraging ranges were estimated for all local breeding populations in Prydz Bay for the incubation (end November to end December when the chicks hatched), guard (end December to mid-January) and crèche (mid-January to mid-February) breeding stages based on the average maximum distances for each foraging trip and direction data from a tracking study at Hop Island (Kokubun et al., 2021). We assumed the same average maximum distance and foraging direction applied to all local populations based on results presented in Kokubun et al. (2021) from a single breeding site (Hop Island) located centrally within the study region. Because the projected foraging sectors of neighbouring local populations can overlap, our estimate of the total foraging area for the entire Prydz Bay population was calculated such that overlapping foraging areas of neighbouring local populations were only included once in the calculation. Hence, our estimate of foraging area is the overall area expected for foraging penguins across the entire study region.
Because Amanda Bay is the only emperor penguin colony within Prydz Bay and fledgling penguins are not central-place foragers, we estimated foraging area based on 75% minimum convex polygons (MCP) of tracking data instead of applying the Critchley et al. (2018) method. The 75% MCP is a simple measure of the foraging range. Foraging location data for fledgling emperor penguins were available from Amanda Bay, in Prydz Bay during December, January, and February (Wienecke and Robertson, 2016). As we did not have foraging location data for breeding emperor penguins specifically from within Prydz Bay, at-sea foraging areas of breeding emperor penguins were based on tracking data for adults breeding at Auster Rookery, near Mawson Station during October, December, and January (Wienecke et al., 2004; Wienecke and Robertson, 1997).
Faecal sample collection and laboratory analysis
Five millilitre low-density polyethylene (LDPE) nutrient tubes were used for collecting and drying penguin guano specimens, sample dilution, and analysis. New LDPE nutrient tubes were soaked in 2% Decon 90 (Decon Laboratories) cleaning solution for at least 7 days and soaked in 10% (v/v) hydrochloric acid (HCl, Merck, Analytical grade 32%, Germany) for 4 weeks prior to use to avoid contamination. Teflon perfluoroalkoxy (PFA) screw cap vials (15 mL; Savillex Corp., USA) for use in sample digestion were soaked in 50% (v/v) HCl for 1 week. Following all acid leaches, materials were rinsed thoroughly (5 times) with ultra-high purity water and left to dry in a high-efficiency particulate air system (HEPA)–filtered Class 100 laminar flow bench.
Sample collection
Fresh penguin guano was carefully collected using new food grade straws as tweezers to lift the sample into acid-cleaned 5-ml LDPE tubes. Individual straws for each sample were used to ensure there was no contamination between samples. The upper most portion (excluding the outer membrane that had formed) of fresh faecal samples were collected to minimise contamination with sea ice or rock sediments. Likewise, any guano samples that were deeply embedded in the ice were avoided as the guano sample was likely impacted by iron from melting ice or snow. Antarctica is predominantly ice covered, which greatly reduces access to sources of dust, and circumpolar winds and currents isolate much of the Southern Ocean from the other continents further north (Wagener et al., 2008). Therefore, dust impact on penguin guano is considered negligible. For the emperor penguins, we were unable to determine whether the sample had come from an adult or a fledgling. As the Adélie penguin guano samples were typically accessible on land rather than ice at the time of collection, only the upper part of the sample was collected to prevent contamination of the sample from terrestrial material. It was possible to collect samples for both adults and chicks for Adélie penguins. Guano samples were frozen at −20°C until analysis. To avoid sample disturbance and contamination, no underlying soil or bedrock samples were collected from the rookeries.
Analysis of iron
Guano samples were dried at 60°C overnight to attain constant weight. Digestion of 5–960 mg subsamples were performed in acid-cleaned 15 ml Teflon (PFA) vials (Savillex, Minnetonka, MN, USA) by adding 1 ml of concentrated nitric acid (HNO3) and 0.125 ml of hydrogen peroxide (H2O2) (all Ultrapure, Seastar Baseline, Choice Analytical). Samples were then heated at 125°C for 8 h on a Teflon-coated digestion hotplate, housed in a bench-top fume hood coupled with HEPA filters to ensure supply of clean air (Digiprep, France). Use of hydrofluoric acid (HF) was unnecessary as this method has been successfully used with prior faecal samples to obtain the biogenic iron content in faecal material (Ratnarajah et al., 2016). We note that penguins may occasionally swallow stones, which are stored in the pyloric region of the gizzard to aid in the mechanical breakdown of food (Baune et al., 2008), but the effects of these stones on iron recycling are not considered in this study since the combination of HNO3 and H2O2 is not expected to digest the stones without an addition of HF.
Identical procedures were applied to procedural blanks (n = 3) and to one certified reference material (CRM, BCR-414 plankton, n = 3) to assess detection limit and digestion efficiency, respectively. Prior to analysis, all samples, CRMs, and digest blanks were diluted 100-fold in 2% v:v HNO3 (Ultrapure, Seastar Baseline). Indium (In) (High-Purity Standards, USA) was added to all samples at a final concentration of 10 μg L−1 and used as an internal standard. Representative subsamples (n = 4) from each analytical sequence were also spiked with a multi-element solution (QCD Analysts, MISA suite of solutions, final concentration of 10 μg L−1, Spring Lake, USA) to monitor instrumental elemental recoveries in the final sample matrices considered.
Four calibration standards with concentrations 0, 1, 5, and 10 μg L−1 were prepared by serial dilution from multi-element stock solutions (QCD Analysts, MISA suite of solutions, Spring Lake, USA). All analyses were conducted using sector field inductively coupled plasma mass spectrometry (ICP-MS) (Thermo Fisher ELEMENT 2, Bremen, Germany), following methods described in Bowie et al. (2010) and Townsend (2000). This ICP-MS has three pre-defined spectral resolutions available enabling isotopes to be quantified with minimal spectral interferences. Iron was measured using “medium” resolution mode (m/dm ~4,500). Average procedural blanks were 0.4 ± 0.1 μg L−1 and limits of detection (defined as 3 times the standard deviation of the blank) was 0.3 μg L−1 for iron. Certified reference material (BCR-414) recovery was 86% (BCR-414 Referenced value for iron were 1,850 ± 190 mg kg−1 and measured average (n = 3) were 1,588 ± 46 mg kg−1). Multi-element spike recoveries of iron in guano digests ranged between 94% and 105% (n = 4).
Statistical analysis
Type II analysis of variance (ANOVA) was used for unbalanced data to determine if there were significant differences in iron concentration between adult and chick Adélie penguins, and between sites for sample collection and species. The iron concentrations were log-transformed to meet the assumptions of ANOVA. Statistical analysis was performed using R.
Flux calculation
The flux of iron was calculated as:
where NA is the number of adult breeding individuals (i.e., breeding pairs × 2) and the number of chicks is calculated as the number of breeding pairs multiplied by the breeding success (BS) (Table 1), M is mass of guano released per day (84.5 g individual−1 d−1 dry weight for adults, Qin et al., 2014, and we assume the value is halved for chicks due to their smaller size), [Fe] is the species and life stage-specific mean concentration of iron in guano (mg kg−1), and A is the area occupied (m2) whilst on land, ice or whilst foraging at sea as described in the Study sites section (Table 2). We converted values of mean specific concentration of iron in guano to μmol kg−1 to facilitate comparison with other published flux estimates.
Results
Within-breeding season changes in foraging area
The estimated areas for foraging ranges for the Adélie penguin metapopulation across Prydz Bay were 86,061 km2 during incubation, 5,793 km2 during guard, and 38,605 km2 during the crèche periods. We assume that Amanda Bay adult emperor penguins foraged over a similar area to Auster Rookery emperor penguins due to lack of site-specific foraging data for adults during summer at Amanda Bay. On this basis, we estimate their 75% minimum convex polygon foraging areas to be 31,980 km2 during October, 98,733 km2 during December, and 24,436 km2 during January (Wienecke and Robertson, 2016; Wienecke et al., 2004, and Wienecke and Robertson, 1997). We assumed the area covered for November where we lacked data to be the same as October. For fledglings from Amanda Bay where observational data is available, we estimated that they forage over 25,938 km2 during December, 499,005 km2 during January, and 276,920 km2 during the start of February (Wienecke and Robertson, 2016).
Iron concentration in penguin faeces
Penguins release guano on land (Adélie), fast ice (Emperor), and in the ocean (both). Iron concentrations in Adélie penguin guano were 418 ± 269 mg kg−1 dry weight for adults (n = 10) and 592 ± 538 mg kg−1 dry weight for chicks (n = 9) (Figure 3). The concentration of iron in emperor penguin guano were 228 ± 187 mg kg−1 dry weight for guano samples collected from Auster Rookery (n = 6) and 351 ± 236 mg kg−1 dry weight for guano samples collected at Amanda Bay (n = 6) (Figure 3). We found no significant difference between iron concentrations measured in the guano of Adélie penguin adults and chicks (p = 0.8), between guano samples collected from emperor penguins for the two locations (p = 0.3), or between Adélie and emperor penguins (p = 0.1). As there was no significant difference between species, life stage and location, pooling all the data results in an iron concentrations of 419 ± 362 mg kg−1 dry weight.
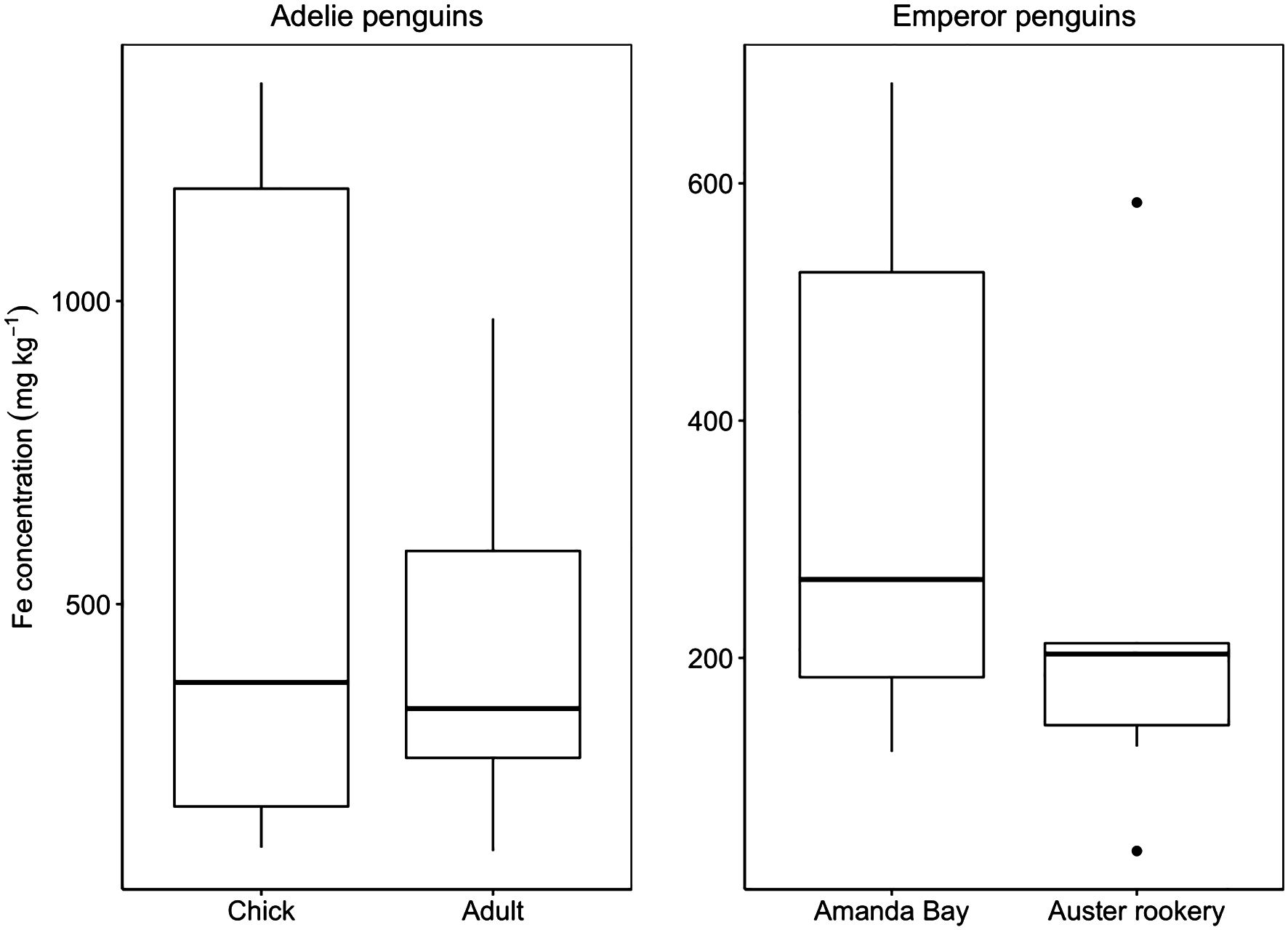
Figure 3. Guano iron (Fe) concentrations for Adélie penguin chick (n = 9) and adult (n = 10) and emperor penguin from Amanda Bay (n = 6) and Auster Rookery (n = 6) (mg kg–1). Thick black horizontal line indicates median value. All points outside of the box and whiskers are outliers (1.5× below the 1st quartile or 1.5× above the third quartile).
Iron flux for terrestrial and marine activity
Because there was no significant difference in iron concentration in the guano, we extrapolated the results to the entire Adélie penguin population in Prydz Bay. Whilst neighbouring populations are likely to have overlapping foraging ranges, the Critchley et al. (2018) method accounts for this, and by calculating the common area used by the entire population, not the sum of individual penguins’ ranges which if overlapping would over-estimate the populations foraging range due to repeat counting of range overlaps. The flux of iron supplied via penguin recycling varies based on the breeding/foraging patterns (Tables 1, 2 and Supplementary Material). The flux is greater at their colonies compared to the ocean (Table 2), which is consistent with their dense aggregations at their breeding colonies as opposed to their widespread foraging range. Across the regional Adélie penguin population of Prydz Bay, breeding Adélie penguins supplied between 254 and 1,243 μmol iron m−2 day−1 at their colony and 0.002 and 0.06 μmol iron m−2 d−1 whilst they are foraging. This is supplemented by the guano from their chicks, which adds an additional 285 μmol iron m−2 day−1 at their colony. Note that our flux calculations are from 15 October until the 15 February, but chicks only release guano after they have hatched, which occurs towards the end of December. Emperor penguins have a different life cycle and, over the same period, the breeding population from Amanda Bay supplies between 237 and 1,184 μmol iron m−2 day−1 when at their fast ice colonies compared to 7 × 10−5 to 4 × 10−4 μmol iron m−2 day−1 when foraging at sea. Emperor penguin chicks contribute towards the guano iron pool over the entire period as well and therefore supplement an additional 204 μmol iron m−2 day−1 when at their fast ice colonies and 3 × 10−6 to 6 × 10−5 μmol iron m−2 day−1 when foraging at sea.
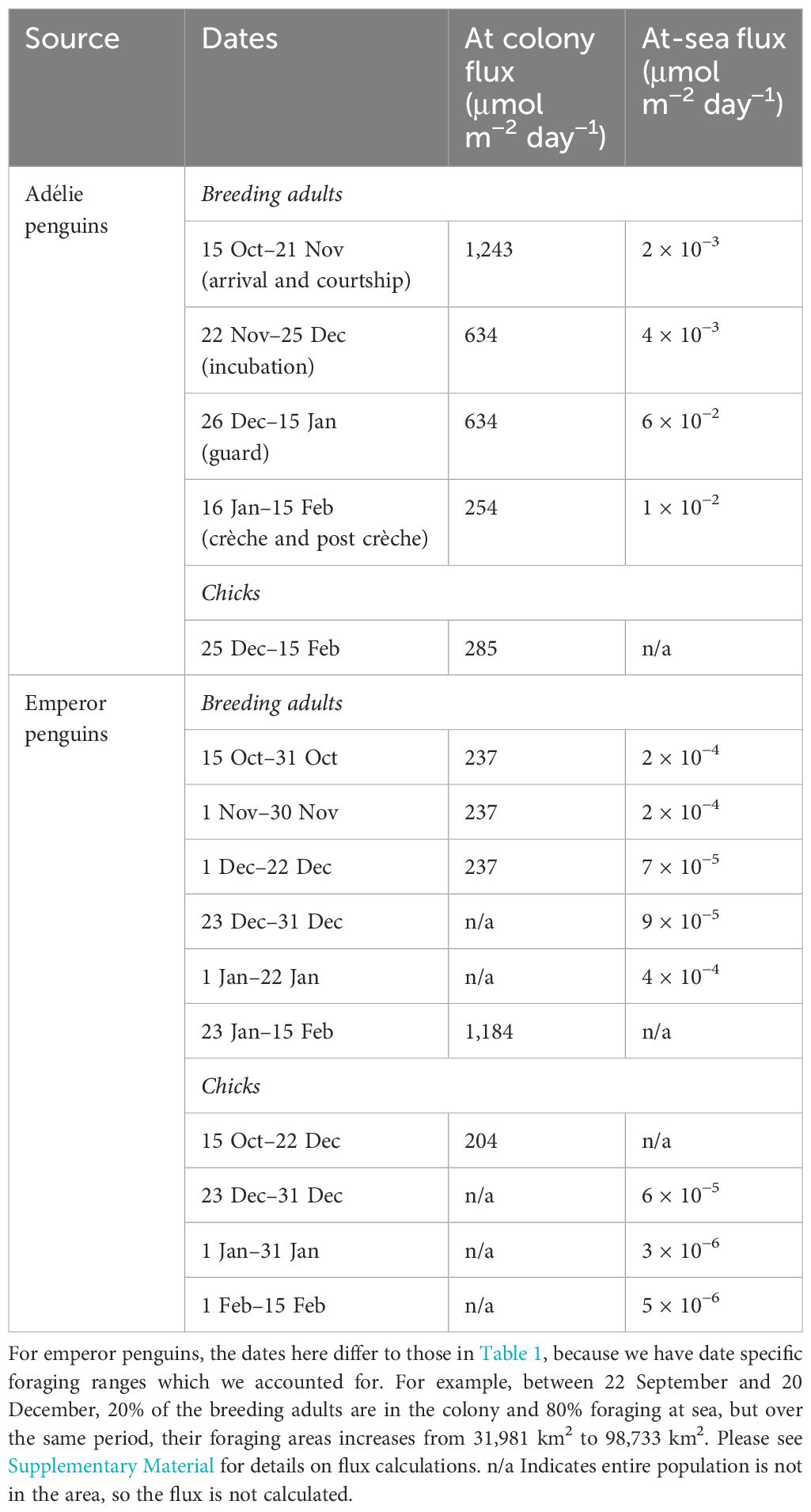
Table 2. Flux of iron from Adélie and emperor penguins breeding across the Prydz Bay region based on their life stage and time spend at the colony vs foraging at sea.
Discussion
How does penguin guano compare to other recycled sources of iron in the region?
When compared to other biological iron sources, Adélie and emperor penguin guano from this study (419 ± 362 mg kg−1, n = 31) are higher that what is observed for Yellow eyed penguin (140 ± 28 mg kg−1, Wing et al., 2017), Southern royal albatross (158 ± 42 mg kg−1, Wing et al., 2017) and whale faecal material (ranging from 64 to 237 mg kg−1 depending on species, Nicol et al., 2010). Interestingly, Chinstrap penguin guano from Deception Island had iron concentrations 6 times higher (3,020 ± 1,455 mg kg−1, Belyaev et al., 2023) compared to what was observed in this study. Whilst Chinstrap penguins also feed on Antarctic krill, there is an active submarine volcano in Deception Island. Antarctic krill can feed at the seabed (Clarke and Tyler, 2008; Schmidt et al., 2011), and iron concentration in the fifth abdominal segment (krill muscle) alone of Antarctic krill from Deception Island were significantly higher (140 ± 20 mg kg−1, Deheyn et al., 2005) compared to whole krill (19 ± 7 mg kg−1, 10 ± 3 mg kg−1, 18 ± 12 mg kg−1 depending on year, Ratnarajah et al., 2016) or krill muscle (5 ± 1 mg kg−1, Ratnarajah et al., 2016) from Prydz Bay. This suggest that geothermally sourced iron is transferred up the food chain and can be recycled through the biology (i.e., both Antarctic krill and penguins), leading to greater recycling of iron in iron-rich hotspots.
Iron in guano considered in this study was also comparatively lower than that measured for brown skua guano (6,577 ± 1,309 mg kg−1, Wing et al., 2017), Southern giant petrel (2,343 ± 501 mg kg−1, Wing et al., 2017), Auckland Island shag (2,254 ± 1,301 mg kg−1, Wing et al., 2017) and Antarctic krill faecal pellets (25,245 ± 36,577 mg kg−1, Schmidt et al., 2016; Ratnarajah et al., 2016) (Figure 4). The higher iron concentration noted for brown skua guano may arise due to their feeding on burrowing petrels and Antarctic fur seal (Anderson et al., 2008), whilst the higher, and highly variable, iron content in Antarctic krill faecal pellets may result from their feeding at the seabed and/or the surface (Schmidt et al., 2011, 2016; Ratnarajah et al., 2016).
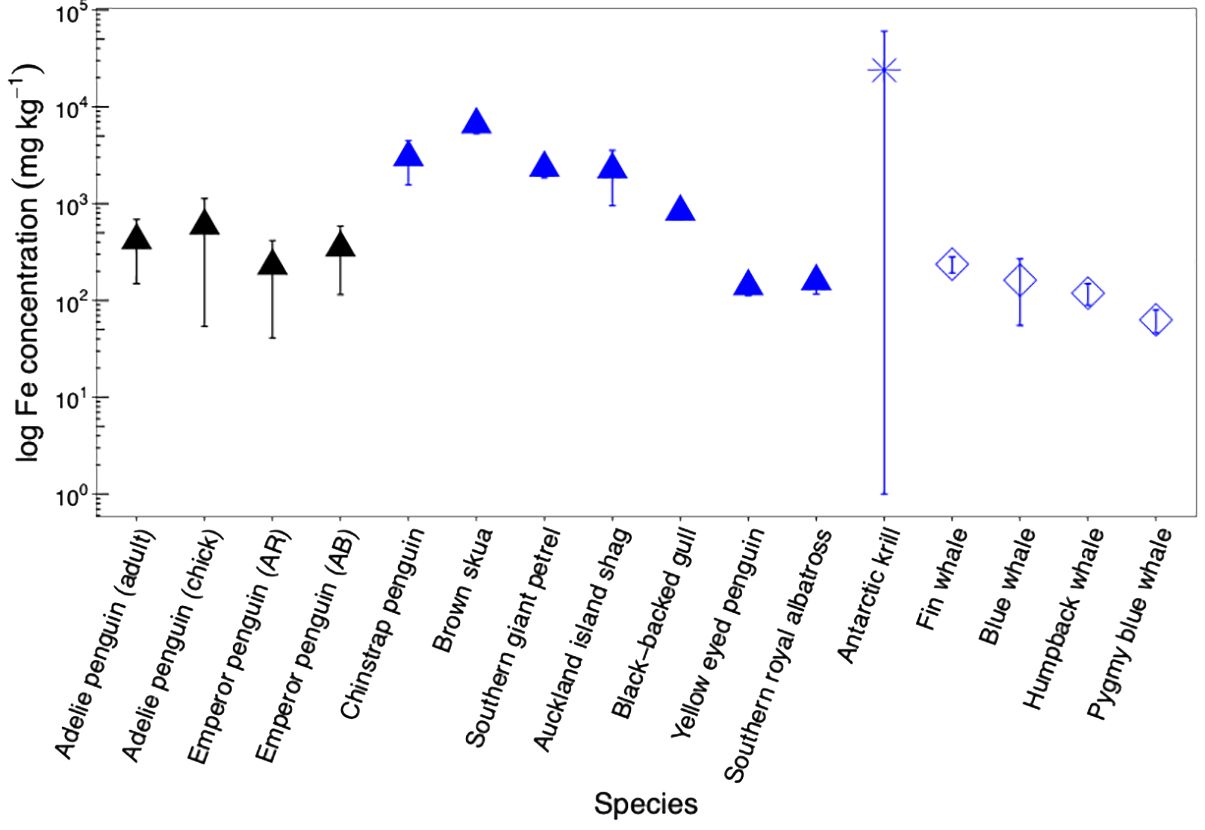
Figure 4. Average iron (Fe) concentrations (as log values) and standard deviation in Adélie (adult and chick) and emperor [from Auster Rookery (AR) and Amanda Bay (AB)] penguin guano from this study (black triangle) compared to faecal material from other biological sources (blue icons) in the Southern Ocean. Blue triangles denote other Southern Ocean seabirds such as Brown skua, Southern giant petrel, Auckland Island shag, black-backed gull, yellow eyed penguin, and Southern royal albatross (Wing et al., 2017; Belyaev et al., 2023). Blue asterisk represents iron concentration for Antarctic krill (Ratnarajah et al., 2016. Schmidt et al., 2016). Blue diamonds denote Pygmy blue whale, blue whale, fin whale, and humpback whale from the Southern Ocean (Nicol et al., 2010).
Are penguins a source of recycled iron to Southern Ocean surface seawaters?
In large areas of the Southern Ocean, dissolved iron concentration in surface seawater is typically less than 0.5 nmol L−1 which limits phytoplankton production (Tagliabue et al., 2012). The high iron concentration in penguin guano suggests that they are a source of recycled iron. Using the average iron concentration in penguin guano (419 mg kg−1), and excretion of guano per day (84.5 g individual−1 day−1 dry weight, Qin et al., 2014), the entire breeding population of emperor penguins at Amanda Bay and Adélie penguins within Prydz Bay (1,115,778 individuals) can mobilise the redistribution of up to 39 kg of iron per day. However, penguins reside on land or ice and forage in the open ocean, thus the flux of iron released is dependent on their location (e.g., on fast ice versus land), timing within a breeding season (e.g., penguin breeding or feeding season and timing of ice melt), seabird abundance, and the extent of the inhabited/foraging area. Consequently, to determine the relative contribution of penguins to the cycling of iron, we calculated the daily flux of iron from penguins accounting for their different breeding patterns (Table 1).
For breeding Adélie penguins, at the start of spring/summer, 98% of penguins are in their colonies whilst 2% are foraging at sea (Table 1). Over this period, Adélie penguins contribute 1,243 μmol iron m−2 day−1 in their colonies (Table 2). Over the incubation and guard period, 50% of the population are at the colonies and 50% are at sea, but during the guard period, their foraging extent decreases. Consequently, whilst their flux at the colonies remains the same for both periods (634 μmol iron m−2 day−1, Table 2), their flux during foraging increases ~13 times (from 0.005 to 0.06 μmol iron m−2 day−1, Table 2). During the crèche and post crèche periods, 20% of the population are at the colonies and 80% are at sea. Despite most of Adélie penguins foraging at sea over this period, they contribute only 0.01 μmol iron m−2 day−1 (Table 2) because the 881,693 individuals cover a large foraging range of 38,605 km2.
Emperor penguins have a different life cycle compared to Adélie penguins, therefore by the start of the flux calculation period (15 Oct), only 20% are at their fast ice colonies and 80% are foraging at sea as it is their post crèche period (Table 1). Over this period, the population remaining at the fast ice colonies contribute 237 μmol iron m−2 day−1 (Table 2) whilst those at sea contribute between 9 × 10−5 and 2 × 10−4 μmol iron m−2 day−1, as their foraging ranges increase over time (Table 2 and Supplementary Material). The entire chick population is at the colony, and this adds a contribution of 204 μmol iron m−2 day−1. During the pre-moult hyperphagia, the entire breeding population is at sea, and this coincides with the chicks fledgling, so 100% of the breeding adults and chicks are at sea. The breeding adults add between 9 × 10−5 and 4 × 10−4 μmol iron m−2 day−1 as their foraging ranges changes between these months. Similarly, the chicks contribute between 3 × 10−6 and 6 × 10−5 μmol iron m−2 day−1, also due to changing foraging ranges.
Penguins redistribute a large fraction of iron onto their colonies (237–1,243 μmol m−2 day−1). Because emperor penguins inhabit fast ice, ultimately the large flux of iron deposited within the colonies enters the seawater in spring/summer depending on the melt rate of sea ice (Figures 2B, C, 5). Fast ice melts from November to February and emperor penguins begin re-colonising the fast ice as it forms again (Figure 2B). In contrast, Adélie penguin guano on land accumulates at their nesting sites on the island and in the vicinity of their breeding ground and can only enter the seawater via meltwater (from above the colonies that wash the guano into the water), and/or strong katabatic winds that could blow the guano into the ocean. However, the accumulation of guano visible on Hop Island and at other Adélie penguin colonies suggests that the large amount of guano deposited whilst Adélie penguins are on land may largely remain there.
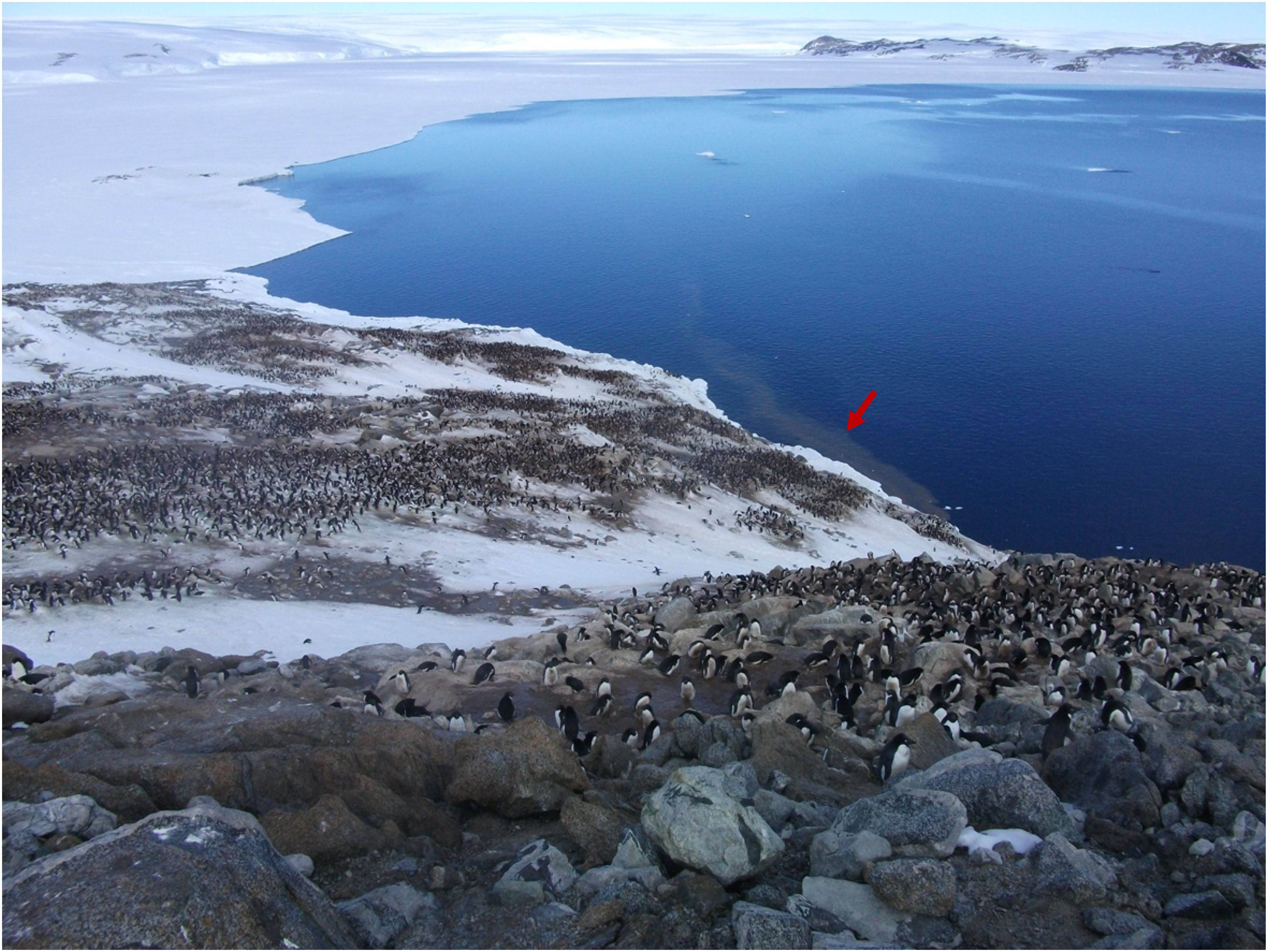
Figure 5. Image showing plume of guano and mud flowing into the Antarctic coastline from Adélie penguin colony [credit: L. Emmerson]. Red arrow indicates plume.
Whilst foraging at sea, the flux of iron from penguin guano is comparable to flux from dust deposition (0.00027–0.09 μmol m−2 day−1, Duce and Tindale, 1991; Lefèvre and Watson, 1999; Edwards and Sedwick, 2001; Bowie et al., 2001; Edwards et al., 2006; Lannuzel et al., 2007; Bowie et al., 2009, 2015) but is minor in comparison to other sources of iron in the region. Compared to another biological source, the flux of iron from Antarctic krill can be over an order of magnitude higher (0.006–0.076 μmol m−2 day−1, Schmidt et al., 2011). Various other physical sources dominate the supply of iron in different regions of the Southern Ocean. For example, in the Atlantic sector, the horizontal flux (advection and diffusion) is an important source of iron but decreases with increasing distance from the shelf break (1–1,375 μmol m−2 day−1, deJong et al., 2012). Other studies demonstrate that horizontal flux is lower, such as in the northeast of the tip of the Antarctic Peninsula (1.8 μmol m−2 day−1, Dulaiova et al., 2009) and at Crozet Islands (0.39 μmol m−2 day−1, Charette et al., 2007; Planquette et al., 2007). Lateral dissolved iron fluxes from sediments also represent an important supply with estimates as high as 136 μmol m−2 day−1 in the Kerguelen Island shelf (Blain et al., 2008) and lower estimates of 1.3–15.5 and 0.028–8.2 μmol m−2 day−1 in the Atlantic sector (deJong et al., 2012) and in the Ross Sea (Marsay et al., 2014), respectively. Other important sources of iron include vertical upward advective/diffusive supply (0.001–5.1 μmol m−2 day−1, deJong et al., 2012; Tagliabue et al., 2014; Schine et al., 2021), hydrothermal input (up to 1 μmol m−2 day−1, Tagliabue et al., 2010), melting icebergs (0.1–1 μmol m−2 day−1, Arrigo et al., 2008; Shaw et al., 2011), and melting sea ice (0.03–1.5 μmol m−2 day−1, Croot et al., 2004; Lannuzel et al., 2007, 2008).
It should be noted that our flux calculations are conservative estimates. At present, there are no counts on the number of non-breeding pairs, which also feed and release iron-rich guano. Based on estimates for Adélie penguin populations, this could nearly double the overall population size (Southwell et al., 2017); hence, the flux of iron on land and at sea could double. Our estimates can also be improved by taking a finer resolution of the foraging range within a breeding season; for example, although we take account of differences between different life history stages (i.e., during the guard stage, the Adélie penguin forages relatively close to their breeding colonies (typically within 20 km) compared with the 400 km during their incubation foraging trips, Clarke et al., 2006), within these breeding stages, the penguins may concentrate guano within localised areas as they leave and return from their colonies. Moreover, we use the broader summer foraging area, which is dependent on sea-ice extent (i.e., foraging area is smaller earlier in the season compared to later in the season) and assume that penguin guano production (84.5 g individual−1 day−1 dry weight, Qin et al., 2014) is the same for emperor and Adélie penguins when it is highly possible that they may have different guano production rates (Burger et al., 1978).
Future studies: key uncertainties in the bioavailability of guano-derived iron
Our study demonstrates that penguin guano contains high iron concentrations (Figure 4), with a large flux being deposited at their colonies that requires mechanisms for entry into the seawater. Melting ice can wash the high flux of iron deposited in the colonies into the coastline (Figure 5). In contrast, their contribution directly into seawater during their foraging period is somewhat negligible (Table 2), because the penguins cover a very large foraging area. In a separate incubation experiment, the addition of iron from penguin guano to phytoplankton communities from sub-tropical, sub-Antarctic islands, and sub-Antarctic water masses resulted in an increase in phytoplankton biomass and shifted the community structure to more large-celled species (Shatova et al., 2017). Taken together, this suggests that penguin guano may be an additional source of iron along the marginal ice zone that could stimulate phytoplankton production over the spring/summer period alongside the other sources of iron in the region. However, many questions remain on the bioavailability of guano-derived iron which should be addressed in future studies to gain a holistic view of iron recycling in the region.
Despite its liquid-like consistency, guano is denser than seawater and as such may sink below the mixed layer relatively quickly. Although the sinking rate of guano is unknown, solubilisation, bacterial remineralisation and complexation by organic ligands could sustain guano-derived iron in the upper ocean. Experiments carried out on dust and whale faecal material, leached with seawater showed that iron leached is transferred from the particulate into the dissolved phase as it remained in contact with seawater over time (Ratnarajah et al., 2017; Perron et al., 2020). In parallel, guano can also be remineralised by the bacterial community similar to fast-sinking zooplankton faecal pellets and contribute to the recycling of iron in the upper ocean (Bowie et al., 2015) and/or complexed by organic ligands that make iron more bioavailable for uptake by phytoplankton. It is unclear if penguin guano contains organic ligands or is reliant on organic ligands present in seawater and/or sea ice. Antarctic sea ice contains some of the highest concentrations of organic ligands ever recorded in the marine environment that could bind with guano-derived iron (4.5–72 nmol L−1 iron-binding ligands in sea ice, Lannuzel et al., 2015).
In summary, Antarctic sea ice has been shown to incorporate iron at levels up to two orders of magnitude higher than the water column below (Lannuzel et al., 2015). Most of the iron found in sea ice is incorporated from seawater below, not from the top. Iron is not able to percolate into the sea ice cover (whether it is dust or guano) when it forms, because the typically low permeability of the ice in winter does not allow the brine pockets/channels to connect and allow the transfer of iron from the surface to deeper layers (Lannuzel et al., 2016). Penguin guano is primarily released directly into seawater during their foraging trips or washed and diluted into seawater during sea ice melt. Therefore, the guano enters the seawater during a period of ice melt rather than formation (Figure 2). The timing of guano-iron input is ideal, as it occurs when phytoplankton are not inhibited by light availability. Penguin guano may remain in the surface waters for longer time periods aided by solubilisation, remineralisation, and complexation by organic ligands in the water column as discussed above and incorporated into sea ice as it reforms.
Conclusion
Penguins are unique in that their contribution to nutrient recycling occurs both in their breeding colonies (land and ice) and in the ocean, and this is dependent on their breeding and foraging cycles. Our study finds that penguins redistribute a large flux of iron onto their colonies. Iron redistributed on fast ice by emperor penguins enters the ocean as the ice melts over the spring/summer season. However, iron redistributed on land by Adélie penguins largely remains at the site, as can be seen from satellite imagery. Because of the much larger foraging area they occupy, the flux of iron supplied during their foraging periods at sea is negligible compared to other iron sources in the region but may be concentrated where penguins access their breeding sites. Further studies should address the bioavailability of penguin guano, particularly the local importance around the marginal ice zone where seawater iron levels are generally low, and a large flux of iron is deposited daily by emperor penguins.
Data availability statement
The original contributions presented in the study are included in the article/Supplementary Material. Further inquiries can be directed to the corresponding author.
Ethics statement
Field procedures were approved by the Australian Antarctic Animal Ethics Committee and through Antarctic Treaty Environmental Protection (ATEP) permits to Australian Antarctic Science (AAS) #4087. The study was conducted in accordance with the local legislation and institutional requirements.
Author contributions
LR: Conceptualization, Data curation, Formal analysis, Investigation, Methodology, Project administration, Resources, Validation, Visualization, Writing – original draft, Writing – review & editing. LE: Conceptualization, Data curation, Formal analysis, Investigation, Methodology, Resources, Validation, Visualization, Writing – original draft, Writing – review & editing. CS: Conceptualization, Data curation, Formal analysis, Investigation, Methodology, Resources, Validation, Writing – original draft, Writing – review & editing. DL: Conceptualization, Formal analysis, Funding acquisition, Supervision, Writing – review & editing. AT: Formal analysis, Investigation, Resources, Supervision, Writing – review & editing. AB: Funding acquisition, Investigation, Project administration, Supervision, Writing – review & editing.
Funding
The author(s) declare financial support was received for the research, authorship, and/or publication of this article. This study was supported by the Australian Antarctic Division, Australian Government’s Cooperative Research Centres Programme through the Antarctic Climate and Ecosystem Cooperative Research Centre, the Australian Antarctic Partnership Program, and the Institute for Marine and Antarctic Studies and the Holsworth Wildlife Research Endowment. Access to ICP-MS instrumentation was supported through ARC LIEF funds (LE0989539). LR received funding from the European Research Council under the European Union’s Horizon 2020 research and innovation programme (Grant Agreement No. 724289 awarded to Alessandro Tagliabue). This investigation is a contribution to Australian Antarctic Science Projects #4087 and #4518.
Acknowledgments
We thank the Mawson seabird field team for collecting samples.
Conflict of interest
The authors declare that the research was conducted in the absence of any commercial or financial relationships that could be construed as a potential conflict of interest.
Publisher’s note
All claims expressed in this article are solely those of the authors and do not necessarily represent those of their affiliated organizations, or those of the publisher, the editors and the reviewers. Any product that may be evaluated in this article, or claim that may be made by its manufacturer, is not guaranteed or endorsed by the publisher.
Supplementary material
The Supplementary Material for this article can be found online at: https://www.frontiersin.org/articles/10.3389/fmars.2024.1465847/full#supplementary-material
References
Anderson O. R. J., Phillips R. A., Shore R. F., McGill R. A. R., McDonald R. A., Bearhop A. (2008). Diet, individual specialisation and breeding of brown skuas (Catharacta Antarctica lonnbergi): an investigation using stable isotopes. Polar Biol. 32, 27–33. doi: 10.1007/s00300-008-0498-9
Arrigo K. R., vanDijken G. L., Bushinsky S. (2008). Primary production in the southern ocean 1997–2006. J. Geophysical Res. 113, C08004. doi: 10.1029/2007JC004551
Ballance L. T., Ainley D. G., Ballard G., Ballard K. (2009). An energetic correlate between colony size and foraging effort in seabirds, an example of the Adélie penguin Pygocelis adeliae. J. Avian Biol. 40 (3), 279–288.
Baune D., LeBohec C., Lucas F., Gauthier-Clerc M., Maho Y. L. (2008). Stomach stones in king penguin chicks. Polar Biol. 32, 593–597. doi: 10.1007/s00300-008-0558-1
Belyaev O., Sparaventi E., Navarro G., Rodríguez-Romero A., Tovar-Sánchez A. (2023). The contribution of penguin guano to the Southern Ocean iron pool. Nat. Commun. 14, 1781. doi: 10.1038/s41467-023-37132-5
Blain S., Sarthou G., Laan P. (2008). Distribution of dissolved iron during the natural iron-fertilization experiment KEOPS (Kerguelen Plateau, Southern Ocean). Deep Sea Res. Part II 55 594–, 605. doi: 10.1016/j.dsr2.2007.12.028
Bowie A. R., Lannuzel D., Remenyi T., Wagener T., Lam P. J., Boyd P. W., et al. (2009). Biogeochemical iron budgets of the Southern Ocean south of Australia: Decoupling of iron and nutrient cycles in the subantarctic zone by the summertime supply. Global Biogeochemical Cycles 23, 1–14.10. doi: 10.1029/2009GB003500
Bowie A. R., Maldonado M. T., Frew R., Croot P. L., Achterberg E. P., Fouzi R., et al. (2001). The fate of added iron during a mesoscale fertilisation experiment in the Southern Ocean. Deep Sea Res. II 48, 2703–2743. doi: 10.1016/S0967-0645(01)00015-7
Bowie A. R., Townsend A. T., Lannuzel D., Remenyi T., van der Merwe P. (2010). Modern sampling and analytical methods for the determination of trace elements in marine particulate material using magnetic sector ICP-MS. Analytica Chimica Acta 676, 15–27. doi: 10.1016/j.aca.2010.07.037
Bowie A. R., van der Merwe P., Quéroué F., Trull T., Fourquez M., Planchon F., et al. (2015). Iron budgets for three distinct biogeochemical sites around the Kerguelen archipelago (Southern Ocean) during the natural fertilisation experiment KEOPS-2. Biogeosciences 11, 17861–17923. doi: 10.5194/bg-12-4421-2015
Burger A. E., Lindeboom H. J., Williams A. J. (1978). The mineral and energy contributions of guano of selected species of birds to the Marion Island terrestrial ecosystem. South Afr. J. Antarctic Res. 8, 59–70.
Cabanes D. J. E., Norman L., Santos-Echeandía J., Iversen M. H., Trimborn S., Laglera L. M., et al. (2017). First evaluation of the role of salp fecal pellets on iron biogeochemistry. Front. Mar. Sci. 3, 1–11. doi: 10.3389/fmars.2016.00289
Charette M. A., Gonneea M. E., Morris P. J., Statham P., Fones G., Planquette H., et al. (2007). Radium isotopes as tracers of iron sources fueling a Southern Ocean phytoplankton bloom. Deep Sea Res. Part II 54 1989–, 1998. doi: 10.1016/j.dsr2.2007.06.003
Clarke A., Tyler P. A. (2008). Adult Antarctic Krill Feeding at Abyssal Depths Current Biology, Vol. 18 4. 282 –285.
Clarke J., Emmerson L., Otahal P. (2006). Environmental conditions and life history constraints determine for aging range in breeding Adélie penguins. Mar. Ecol. Prog. Ser. 310, 247–261. doi: 10.3354/meps310247
Critchley E. J., Grecian W. J., Kane A., Jessopp M. J., Quinn J. L. (2018). Marine protected areas show low overlap with projected distributions of seabird populations in Britain and Ireland. Biol. Conserv. 224, 309–317. doi: 10.1016/j.biocon.2018.06.007
Croot P. L., Andersson K., Öztürk M., Turner D. R. (2004). The distribution and speciation of iron along 6°E in the Southern Ocean. Deep Sea Res. Part II 51, 2857–2879. doi: 10.1016/j.dsr2.2003.10.012
Dalbec A., Twining B. S. (2009). Remineralization of bioavailable iron by a heterotrophic dinoflagellate. Aquat. Microbial Ecol. 54, 279–290. doi: 10.3354/ame01270
Deheyn D. D., Gendreau P., Baldwin R. J., Latz M. I. (2005). Evidence for enhanced bioavailability of trace elements in the marine ecosystem of Deception Island, a volcano in Antarctica. Mar. Environ. Res. 60, 1–33. doi: 10.1016/j.marenvres.2004.08.001
deJong J., Schoemann V., Lannuzel D., Croot P., de Baar H., Tison J.-L. (2012). Natural iron fertilisation of the Atlantic sector of the Southern Ocean by continental shelf sources of the Antarctic Peninsula. J. Geophysical Res. 117, G01029. doi: 10.1029/2011JG001679
Duce R. A., Tindale N. W. (1991). Atmospheric transport of iron and its deposition in the ocean. Limnology Oceanography 36, 1715–1726. doi: 10.4319/lo.1991.36.8.1715
Dulaiova H., Ardelan M. V., Henderson P. B., Charette M. A. (2009). Shelf-derived iron inputs drive biological productivity in the southern Drake Passage. Global Biogeochemical Cycles 23, GB4014. doi: 10.1029/2008GB003406
Edwards R., Sedwick P. (2001). Iron in East Antarctic snow: Implications for atmospheric iron deposition and algal production in Antarctic waters. Geophysical Res. Lett. 28, 3907–3910. doi: 10.1029/2001GL012867
Edwards R., Sedwick P., Morgan V., Boutron C. (2006). Iron in ice cores from Law Dome: A record of atmospheric iron deposition for maritime East Antarctica during the Holocene and Last Glacial Maximum. Geochem. Geophys. Geosyst. 7, Q12Q01. doi: 10.1029/2006GC001307
Emmerson L. M., Pike R., Southwell C. J. (2011). Reproductive consequences of environment-driven variation in Adélie penguin breeding phenology. Mar. Ecol. Prog. Ser. 440, 203–216. doi: 10.3354/meps09265
Emmerson L., Southwell C. (2008). Sea ice cover and its influence on Adélie penguin reproductive performance. Ecology 89, 2096–2102. doi: 10.1890/08-0011.1
Emmerson L., Walsh S., Southwell C. (2019). Non-breeder birds at colonies display qualitatively similar seasonal mass change patterns as breeders. Ecol. Evol. 9 (8), 4637–4650. doi: 10.1002/ece3.5067
Fretwell P. T., LaRue M., Morin P., Kooyman G., Wienecke B., Ratcliffe N., et al. (2012). An emperor penguin population estimate: the first global, synoptic survey of a species from space. PloS One 7. doi: 10.1371/annotation/32c246eb-3b73-4410-a44c-b41ddae11fc5
Fretwell P. T., Trathan P. N. (2009). Penguins from space: faecal stains reveal the location of emperor penguin colonies. Global Ecol. Biogeography 18, 543–552. doi: 10.1111/j.1466-8238.2009.00467.x
Gao Y., Yang L., Xie Z., Emmerson L., Southwell C., Wang Y., et al. (2018). Last millennium Adélie penguin mortality and colony abandonment events on Long Peninsula, East Antarctica. Journal of Geophysical Research. Biogeosciences 123, 2878–2889. doi: 10.1029/2018JG004550
Handley J., Rouyer M.-M., Pearmain E. J., Warwick-Evans V., Teschke K., Hinke J., et al. (2021). Marine Important Bird and Biodiversity Areas for penguins in Antarctica, targets for conservation action. Front. Mar. Sci. 7. doi: 10.3389/fmars.2020.602972
Harris C. M., Lorenz K., Fishpool L. D. C., Lascelles B., Cooper J., Coria N., et al. (2015). Important Birds Areas Antarctica Summary 2015 (Cambridge).
Herraiz-Borreguero L., Church J. A., Allison I., Peña-Molino B., Coleman R., Tomczak M., et al. (2016). Basal melt, seasonal water mass transformation, ocean current variability, and deep convection processes along the Amery Ice Shelf calving front, East Antarctica. J. Geophysical Res. 121, 4946–4965. doi: 10.1002/2016JC011858
Holmes T. M., Chase Z., van der Merwe P., Townsend A. T., Bowie A. R. (2017). Detection, dispersal and biogeochemical contribution of hydrothermal iron in the ocean. Mar. Freshw. Res. 68, 2184–22047. doi: 10.1071/MF16335
Kato A., Watanuki Y., Naito Y. (2003). Annual and seasonal changes in foraging site and diving behavior in Adélie penguins. Polar Biol. 26, 389–395.
Kirkwood R., Robertson G. (1997). Seasonal change in the foraging ecology of emperor penguins on the Mawson coast, Antarctica. Mar. Ecol. Prog. Ser. 156, 205–223.
Kokubun N., Emmerson L., McInnes J., Wienecke B., Southwell C. (2021). Sea-ice and density-dependent factors affecting foraging habitat and behaviour of Adélie penguins throughout the breeding season. Mar. Biol. 168, 97. doi: 10.1007/s00227-021-03899-8
Kooyman G. L., Hunke E. C., Ackley S. F., van Dam R. P., Robertson R. P. (2000). Moult of the emperor penguin: travel, location, and habitat selection. Mar. Ecol. Progress Series 204, 269–277. doi: 10.3354/meps204269
Landschutzer P., Gruber N., Haumann F. A., Rödenbeck C., Bakker D. C. E., van Heuven S., et al. (2015). The reinvigoration of the Southern Ocean carbon sink. Science 349, 1221–1224. doi: 10.1126/science.aab2620
Lannuzel D., Bowie A. R., Remenyi T., Lam P., Townsend A., Ibisanmi E., et al. (2011). Distributions of dissolved and particulate iron in the sub-Antarctic and Polar Frontal Southern Ocean (Australian sector). Deep Sea Res. Part II: Topical Stud. Oceanography 58, 2094–2112. doi: 10.1016/j.dsr2.2011.05.027
Lannuzel D., Grotti M., Abelmoschi M. L., van der Merwe P. (2015). Organic ligands control the concentrations of dissolved iron in Antarctic sea ice. Mar. Chem. 174, 120–130. doi: 10.1016/j.marchem.2015.05.005
Lannuzel D., Schoemann V., deJong J., Chou L., Delille B., Becquevort S., et al. (2008). Iron study during a time series in the western Weddell pack ice. Mar. Chem. 108, 85–95. doi: 10.1016/j.marchem.2007.10.006
Lannuzel D., Schoemann V., de Jong J., Tison J. L., Chou L. (2007). Distribution and biogeochemical behaviour of iron in the East Antarctic sea ice. Mar. Chem. 106, 18–32. doi: 10.1016/j.marchem.2006.06.010
Lannuzel D., Vancoppenolle M., van der Merve P., de Jong J., Meiners M., Grotti M., et al. (2016). Iron in sea ice: Review and new insights. Elementa: Sci. Anthropocene 4, 000130. doi: 10.12952/journal.elementa.000130
Lefèvre N., Watson A. J. (1999). Modeling the geochemical cycle of iron in the oceans and its impact on atmospheric CO2 concentrations. Global Biogeochemical Cycles 13, 727–736. doi: 10.1029/1999GB900034
Lin H., Rauschenberg S., Hexel C. R., Shaw T. J., Twining B. S. (2011). Free-drifting icebergs as sources of iron to the Weddell Sea. Deep Sea Res. Part II: Topical Stud. Oceanography 58, 1392–1406. doi: 10.1016/j.dsr2.2010.11.020
Marsay C., Sedwick P., Dinniman M. S., Barrett P. M., Mack S. L., McGillicuddy D. J. Jr. (2014). Estimating the benthic flux of dissolved iron on the Ross Sea continental shelf. Geophysical Res. Lett. 41, 7576–7583. doi: 10.1002/2014GL061684
Moore J. K., Doney S. C., Lindsay K. (2004). Upper ocean ecosystem dynamics and iron cycling in a global three-dimensional model. Global Biogeochemical Cycles 18, (4). doi: 10.1029/2004GB002220
Nicol S., Pauly T., Bindoff N. L., Wright S., Thiele D., Hosie G. W., et al. (2000). Ocean circulation off east Antarctica affects ecosystem structure and sea-ice extent. Nature 406, 504–507.
Nicol S., Bowie A. R., Jarman S. N., Lannuzel D., Meiners K. M., van der Merwe P. (2010). Southern Ocean iron fertilization by baleen whales and Antarctic krill. Fish Fisheries 11, 203–209. doi: 10.1111/j.1467-2979.2010.00356.x
Otero X. L., Peña-Lastra S. D., Pérez-Alberti A., Ferreira O., Huerta-Diaz M. A. (2018). Seabird colonies as important global drivers in the nitrogen and phosphorus cycles. Nat. Commun. 9, 246. doi: 10.1038/s41467-017-02446-8
Péron C., Karine D., Phillips R., Charbonnier Y., Marteau C., Louzao M., et al. (2010). Seasonal variations in oceanographic habitat and behavior of White-chinned Petrels Procellaria aequinoctialis from Kerguelen Island. Mar. Ecol. Prog. Ser. 416, 267–284. doi: 10.3354/meps08785
Perron M., Strzelec M., Gault-Ringold M., Proemse B., Boyd P. W., Bowie A. R. (2020). Assessment of leaching protocols to determine the solubility of trace metals in aerosols. Talanta 208. doi: 10.1016/j.talanta.2019.120377
Planquette H., Statham P. J., Fones G. R., Charette M. A., Moore C. M., Salter I., et al. (2007). Dissolved iron in the vicinity of the Crozet Islands, Southern Ocean. Deep Sea Res. Part II 54, 1999–2019. doi: 10.1016/j.dsr2.2007.06.019
Poorvin L., Rinta-Kanto J., Hutchins D. A., Wilhelm S. W. (2004). Viral release of iron and its bioavailability to marine plankton. Limnology Oceanography 49, 1734–1741. doi: 10.4319/lo.2004.49.5.1734
Qin X., Sun L., Blais J. M., Wang Y., Huang T., Huang W., et al. (2014). From sea to land: assessment of the bio-transport of phosphorus by penguins in Antarctica. Chin. J. Oceanology Limnology 32, 148–154. doi: 10.1007/s00343-014-3115-5
Ratnarajah L., Lannuzel D., Townsend A. T., Meiners K. M., Nicol S., Friedlaender A. S., et al. (2017). Physical speciation and solubility of iron from baleen whale faecal material. Mar. Chem. 194, 78–88. doi: 10.1016/j.marchem.2017.05.004
Ratnarajah L., Nicol S., Kawaguchi S., Townsend A. T., Lannuzel D., Meiners K. M., et al. (2016). Understanding the variability in the iron concentration of Antarctic krill. Limnology Oceanography 61, 1651–1650. doi: 10.1002/lno.10322
Raymond B., Shaffer S. A., Sokolov S., Woehler E. J., Costa D. P., Einoder L., et al. (2010). Shearwater foraging in the southern ocean: the roles of prey availability and winds. PloS One 5, e10960. doi: 10.1371/journal.pone.0010960
Robertson G., Wienecke B., Emmerson L., Fraser A. D. (2013). Long-term trends in the population size and breeding success of emperor penguins at the Taylor Glacier colony, Antarctica. Polar Biol. 37 (2), 251–259.
Schine C. M. S., Alderkamp A.-C., van Dijken G., Gerringa L. J. A., Sergi S., Laan P., et al. (2021). Massive Southern Ocean phytoplankton bloom fed by iron of possible hydrothermal origin. Nat. Commun. 12, 1211. doi: 10.1038/s41467-021-21339-5
Schmidt K., Atkinson A., Steigenberger S., Fielding S., Lindsay M. C. M., Pond D. W., et al. (2011). Seabed foraging by Antarctic krill: Implications for stock assessment, bentho-pelagic coupling, and the vertical transfer of iron. Limnology Oceanography 56, 1411–1428. doi: 10.4319/lo.2011.56.4.1411
Schmidt K., Schlosser C., Atkinson A., Fielding S., Venebles H. J., Waluda C. M., et al. (2016). Zooplankton gut passage mobilizes lithogenic iron for ocean productivity. Curr. Biol. 26, 1–7. doi: 10.1016/j.cub.2016.07.058
Sedwick P. N., Bowie A. R., Trull T. W. (2008). Dissolved iron in the Australian sector of the Southern Ocean (CLIVAR SR3 section): Meridional and seasonal trends. Deep Sea Res. Part I: Oceanographic Res. Papers 55, 911–925. doi: 10.1016/j.dsr.2008.03.011
Shatova O., Wing S. R., Hoffmann L., Wing L., Gault-Ringold M. (2017). Phytoplankton community structure is influenced by seabird guano enrichment in the Southern Ocean. Estuarine Coast. Shelf Sci. 191, 125–135. doi: 10.1016/j.ecss.2017.04.021
Shaw T. J., Raiswell R., Hexel C. R., Vu H. P., Moore W. S., Dudgeon R., et al. (2011). Input, composition, and potential impact of terrigenous material from free-drifting icebergs in the Weddell Sea. Deep Sea Res. Part II: Topical Stud. Oceanography 58, 1376–1383. doi: 10.1016/j.dsr2.2010.11.012
Smith K. L., Robinson B. H., Helly J. J., Kaufman R. S., Ruhl H. A., Shaw T. J., et al. (2007). Free-drifting icebergs: hot spots of chemical and biological enrichment in the weddell sea. Science 317, 478–482. doi: 10.1126/science.1142834
Southwell D., Emmerson L., Forcada J., Southwell C. (2015). A bioenergetics model for estimating prey consumption throughout the breeding season for Adélie penguins. Mar. Ecol. Prog. Ser. 526, 183–197.
Southwell C., Emmerson L., Takahashi A., Barbraud C., Delord K., Weimerskirch H. (2017). Large-scale population assessment informs conservation management for seabirds in Antarctica and the Southern Ocean: A case study of Adélie penguins. Global Ecol. Conserv. 9, 104–115. doi: 10.1016/j.gecco.2016.12.004
Tagliabue A., Bopp L., Dutay J. C., Bowie A. R., Chever F., Jean-Baptiste P., et al. (2010). Hydrothermal contribution to the oceanic dissolved iron inventory. Nat. Geosci. 3, 252–256. doi: 10.1038/ngeo818
Tagliabue A., Mtshali T., Aumont O., Bowie A. R., Klunder M. B., Roychoudhury A. N., et al. (2012). A global compilation of dissolved iron measurements: focus on distribution and processes in the Southern Ocean. Biogeosciences 9, 2333–2349.
Tagliabue A., Sallee J. B., Bowie A. R., Levy M., Swart S., Boyd P. W. (2014). Surface-water iron supplies in the Southern Ocean sustained by deep winter mixing. Nat. Geosci. 7, 314–320. doi: 10.1038/ngeo2101
Takahashi T., Sutherland S. C., Wanninkhof R., Sweeney C., Feely R. A., Chipman D. W., et al. (2009). Climatological mean and decadal change in surface ocean pCO2, and net sea–air CO2 flux over the global oceans. Deep Sea Res. Part II: Topical Stud. Oceanography 56, 554–577. doi: 10.1016/j.dsr2.2008.12.009
Tovar-Sanchez A., Duarte C. M., Hernández-León S., Sañudo-Wilhelmy S. A. (2007). Krill as a central node for iron cycling in the Southern Ocean. Geophysical Res. Lett. 34, L11601. doi: 10.1029/2006GL029096
Tovar-Sanchez A., Duarte C. M., Hernández-León S., Sañudo-Wilhelmy S. A. (2009). Impact of submarine hydrothermal vents on the metal composition of krill and its excretion products. Mar. Chem. 113, 129–136. doi: 10.1016/j.marchem.2009.01.010
Townsend A. T. (2000). The accurate determination of the first-row transition metals in water, urine, plant, tissue and rock samples by sector field ICP-MS. J. Analytical Spectometry 15, 307–314. doi: 10.1039/a904933i
van der Merwe P., Lannuzel D., Bowie A. R., Mancuso Nichols C. A., Meiners K. M. (2011). Iron fractionation in pack and fast ice in EastAntarctica: Temporal decoupling between the release of dissolved and particulate iron during spring melt. Deep Sea Res. II 58, 1222–1236. doi: 10.1016/j.dsr2.2010.10.036
Wagener T., Guieu C., Losno R., Bonnet S., Mahowald N. (2008). Revisiting atmospheric dust export to the Southern Hemisphere ocean: Biogeochemical implications. Global Biogeochemical Cycles. 22, 2. doi: 10.1029/2007GB002984
Whitehead M. D., Johnston G. W., Burton H. R. (1990). Annual fluctuations in productivity and breeding success of Adélie penguins and fulmarine petrels in Prydz Bay, East Antarctica. Antarctic ecosystems Ecol. Change Conserv. (Berlin).
Wienecke B., Kirkwood R., Robertson G. (2004). Pre-moult foraging trips and moult locations of emperor penguins at the Mawson Coast. Polar Biol. 27, 83–91.
Wienecke B., Raymond B., Robertson G. (2010). Maiden journey of fledgling emperor penguins. Mar. Ecol. Prog. Ser. 410, 269–282. doi: 10.3354/meps08629
Wienecke B., Robertson (1997). Foraging space of emperor penguins Aptenodytes forsteri in Antarctic shelf waters in winter. Mar. Ecol. Prog. Ser. 159, 249–263. doi: 10.3354/meps159249
Wienecke B., Robertson G. (2016). Foraging ecology of emperor penguins in summer and potential overlap with fisheries, Ver. 1, Australian Antarctic Data Centre. Available online at: https://data.aad.gov.au/metadata/records/ASAC_1252.
Wing S. R., Gault-Ringold M., Stirling C. H., Wing L. C., Shatova O. A., Frew R. D. (2017). [amp]]delta;56Fe in seabird guano reveals extensive recycling of iron in the Southern Ocean ecosystem. Limnology Oceanography 62, 1671–1681. doi: 10.1002/lno.10524
Wing S. R., Jack L., Shatova O., Leichter J. J., Barr D., Frew R. D., et al. (2014). Seabirds and marine mammals redistribute bioavailable iron in the Southern Ocean. Mar. Ecol. Prog. Ser. 510, 1–13. doi: 10.3354/meps10923
Keywords: iron, recycling, seabirds, Southern Ocean, Antarctica
Citation: Ratnarajah L, Emmerson L, Southwell C, Lannuzel D, Townsend AT and Bowie AR (2024) Quantifying the role of iron recycling by Adélie and Emperor penguins over the austral spring and summer in Prydz Bay. Front. Mar. Sci. 11:1465847. doi: 10.3389/fmars.2024.1465847
Received: 17 July 2024; Accepted: 14 August 2024;
Published: 10 October 2024.
Edited by:
Sunil Kumar Singh, Physical Research Laboratory, IndiaReviewed by:
David Ainley, H.T. Harvey & Associates, United StatesFrank Dehairs, Vrije University Brussels, Belgium
Copyright © 2024 Ratnarajah, Emmerson, Southwell, Lannuzel, Townsend and Bowie. This is an open-access article distributed under the terms of the Creative Commons Attribution License (CC BY). The use, distribution or reproduction in other forums is permitted, provided the original author(s) and the copyright owner(s) are credited and that the original publication in this journal is cited, in accordance with accepted academic practice. No use, distribution or reproduction is permitted which does not comply with these terms.
*Correspondence: Lavenia Ratnarajah, TGF2ZW5pYS5SYXRuYXJhamFoQHV0YXMuZWR1LmF1