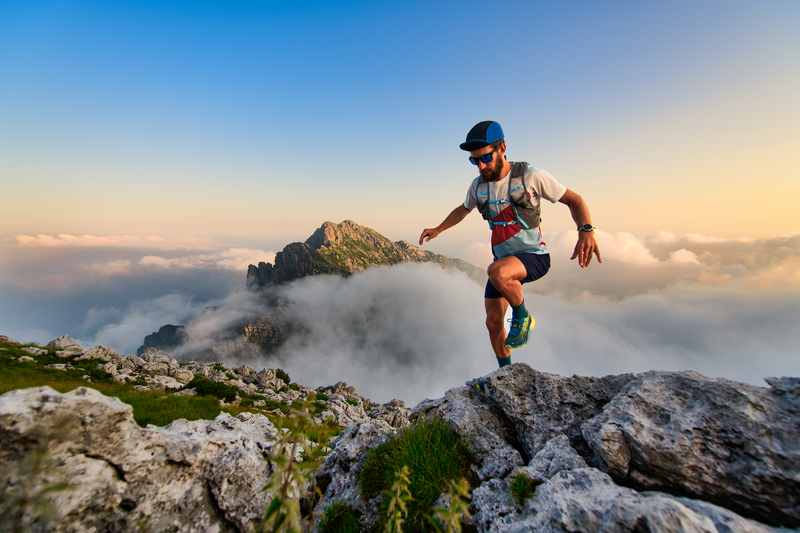
95% of researchers rate our articles as excellent or good
Learn more about the work of our research integrity team to safeguard the quality of each article we publish.
Find out more
ORIGINAL RESEARCH article
Front. Mar. Sci. , 25 November 2024
Sec. Marine Molecular Biology and Ecology
Volume 11 - 2024 | https://doi.org/10.3389/fmars.2024.1446998
This article is part of the Research Topic New Techniques for Providing a Window Into the Opaque Ocean View all 5 articles
Current climate change, particularly ocean warming, will induce shifts in marine species distribution and composition, affecting the marine food web and, thus, trophic interactions. Analyses of the stable isotopes 13C and 15N are commonly used to detect trophic markers for food web analyses. With the current standard methods used in food web ecology, it is still challenging to identify potential changes in the uptake and utilization of trophic markers. In this work, we present a 13C-enrichment analysis by NMR spectroscopy to track the uptake and utilization of dietary carbon in a simple laboratory experiment of a primary producer and its consumer (algae and bivalve). In particular, we tested the hypothesis of a temperature-dependent use of dietary carbon by tracing the incorporation of 13C-atoms. Unicellular phytoplankton, Phaeodactilum tricornutum, was reared in a medium containing 13C-labeled bicarbonate. The accompanying 13C-NMR spectra of labeled P. tricornutum showed a specific profile of 13C-labeled compounds, including typical trophic markers such as the polyunsaturated omega-3 fatty acid eicosapentaenoic acid (EPA). Afterwards, 13C-labeled P. tricornutum was fed to King scallops, Pecten maximus, kept at two different temperatures (15°C and 20°C). Tissue-specific NMR spectra of P. maximus revealed elevated 13C-NMR signals, particularly of the fatty acid EPA in the digestive gland, which was not evident in muscle tissue. The comparison between the two temperatures indicated a change in trophic markers. At the higher temperature, less unsaturated fatty acids were detected in the digested gland, but increased 13C-labels in sugars were detected in the adductor muscle. This might indicate a change in the uptake and utilization of the trophic marker EPA in P. maximus due to a shift in energy conversion from favored beta-oxidation at colder temperatures to conversion from carbohydrates in the warmth. Our approach indicates that besides the accumulation of trophic markers, their incorporation and conversion are additional important factors for the reliable interpretation of trophic linkages under climate change.
Current and ongoing climate change is accompanied by warming, ocean acidification, and deoxygenation, leading to oxygen minimum zones of the oceans (Pörtner et al., 2022). This directly impacts ectothermic organisms through increased energy demand and supply (Pörtner et al., 2017). In this context, the importance of the interactions between energy supply, energy storage, and energy allocation and their trade-offs in climate change has only recently been emphasized (Grunst et al., 2023). However, climate change will not only affect the organism`s energy budget. Still, it will also lead to shifts in the species diversity in the ecosystems, with species migrating out of an ecosystem and new species migrating in (Pinsky et al., 2020). In particular, phytoplankton communities will change their abundance and distribution (Hays et al., 2005). This results in earlier plankton blooms and a shift in distribution towards more thermophilic species, such as cyanobacteria and dinoflagellates (Paerl and Scott, 2010). This will have consequences for the quantity and quality of the phytoplankton as a food resource. Indeed, it is long known that the lipid composition of phytoplankton shifts to less unsaturated fatty acids at higher temperatures (Thompson et al., 1992). The potential impact of climate change changing diets is currently being extensively discussed for the availability of essential fatty acids, like omega-3 long-chain polyunsaturated fatty acids, such as eicosapentaenoic acid (EPA) and docosahexaenoic acid (DHA). These fatty acids are important for almost all organisms’ survival, development and growth (for a recent review, see Tan et al., 2023).
Last but not least, there will be alterations in behavior due to climate change, which will, for example, lead to changes in the feeding behavior of organisms. This factor has been given limited attention in the literature on the effects of climate change. Only recently, Gauzens et al., 2024 reported on potential changes in the foraging behavior of predators under warmer conditions, which can lead to unexpected effects, such as a lower consumption efficiency despite increased energy demand in the warmth (Sokolova et al., 2012). However, the underlying mechanisms for this are little or not understood. This might restructure marine food webs with potentially severe consequences, as already apparent in the Arctic (Kortsch et al., 2015). There is, therefore, an urgent need to better understand the potential changes in marine food webs and their trophic linkages to make reliable predictions about consequences for the biodiversity in an ecosystem. Food web ecology to describe trophic linkages and relationships is based on three basic techniques: stomach content analysis, stable isotope analysis, and fatty acid or sterol analysis. All three methods have advantages and disadvantages, extensively discussed in the relevant literature (for an overview, see Pasquaud et al., 2007).
Analyzing stable isotopes and determining fatty acids allows for insights into the energy transfer and energy flow in a food web and across predator-prey interactions. Both techniques can identify trophic markers and their linkages and relationships. In the following, we will present a method that integrates both analyses and is particularly suitable for detecting trophic markers. Stable isotope analysis has a long-standing tradition for tracing food- and trophic biomarkers to follow trophic linkages and energy flow (Peterson and Fry, 1987). Such investigations are extended to study the impact of environmental changes in ecosystems (for recent reviews, see Cresswell et al., 2020; Hobson, 2023). Nowadays, such studies on trophic biomarkers and linkages can be easily performed with the combined analysis of stable isotopes such as 13C and 15N, where carbon elucidates the energy flow, while nitrogen gives the position at the trophic level (Pasquaud et al., 2007). Stable isotope analysis recently gained a new renaissance due to the development of compound-specific isotope analysis (CSIA) tools, particularly for bulk stable isotope and fatty acid composition analysis (Twining et al., 2020).
13C-enrichment NMR spectroscopy is a unique tool to follow the uptake and incorporation of carbon in an organism due to the magnetic properties of the 13C-isotope in contrast to the 12C-isotope with a missing nuclear spin. 13C-enrichment NMR spectroscopy has been extensively used in studies (in vitro and in vivo) and has a long tradition in physiology and medical research. In particular, it allows the detection of 13C, which was incorporated in carbohydrates, such as glucose (Shulman and Rothman, 2001), amino acids (Morris and Bachelard, 2003), and fatty acids (Hwang et al., 2003). Since the 13C-isotope can be used to trace food sources and help to identify trophic markers in aquatic organisms, it is obvious that applications from biomedical research should be transferred to the challenges in trophic ecology. Therefore, the present study aimed to demonstrate the applicability of 13C-enrichment NMR spectroscopy to follow the energy flow in combination with the detection and analysis of incorporated carbohydrates, amino acids and fatty acids in a simple food chain model under global warming conditions. In the first step, 13C-enrichment NMR spectroscopy was used to follow the incorporation of 13C-atoms into energy-rich substances in the unicellular diatom, Phaeodactilum tricornutum, after cultivation in a medium containing 13C-labeled bicarbonate. In a second step, the algae were subsequently fed to our animal model consumer, the King scallop, Pecten maximus, at two different temperatures (temperate 15°C as the control temperature versus a moderate-warm temperature of 20°C). Finally, the incorporation of carbon sources into the scallop`s digestive gland and adductor muscle was screened using 13C-enrichment NMR spectroscopy.
Two objectives should be demonstrated in this work using 13C-enrichment NMR spectroscopy. Firstly, identifying trophic markers via 13C-tracing and their fate after absorption into another organism. Secondly, the potential for detecting changes in the use of metabolic substrates and energy conversion caused by climate warming.
Phaeodactylum tricornutum cultures were obtained from BlueBioTech GmbH (Büsum, Germany). Four batch cultures with 1*105 cells/mL starting concentrations were set up and incubated in f/2+Si medium (Guillard, 1975) with 0.2μm filtered seawater. In a trial experiment, unlabeled bicarbonate was replaced by 13C-labeled bicarbonate (Sigma/Aldrich, Germany) and added to the batch cultures in 1 mM and 3 mM (duplicate), respectively. Algae cultures were incubated inside a temperature control room with a constant temperature of 15°C. The light was constantly provided by a white fluorescent tube with a light intensity of 64.25 μEinstein m-2 s-1. Algae were left growing undisturbed for 7 days. Cell concentrations of each incubation bottle were determined using Utermoehl counting chambers and were manually counted under an Axio Observer (Zeiss, Germany). After incubation, the algae were transferred to falcon tubes and centrifuged for 10 minutes at 800rcf at 4°C. This procedure was repeated twice. The supernatant was discarded, and the algae pellet was stored at -80°C until further processing.
For the feeding experiments, P. tricornutum was incubated under the same conditions again, but only with a final 13C-labeled bicarbonate concentration of 3 mM and a starting concentration of 1*106 cells/mL. After harvesting the 13C-labeled cultivated algae, the algae were fed directly to the scallops.
Algae pellets were prepared for NMR spectroscopy using a methanol-chloroform extraction protocol (adapted from Bligh and Dyer, 1959). 2*108 cells were transferred to an ice-chilled precellys tube containing 400μL methanol and 125μL milli-Q water. The samples were homogenized using a precellys with 1 cycle of 20s at 6000 rpm (Precellys 24, Bertin technologies, France). Finally, 400μL chloroform and 400μL milli-Q water were added to the tubes and vortexed for 15s. After settling on ice for 10 min, the samples were centrifuged for 10 min with 3000 rpm at 4°C. The upper layer, containing the methanol soluble metabolites, was transferred to 1.5mL Eppendorf tubes and was dried in a vacuum concentrator (Speed-Vac, RVC 2-18 CD plus, Christ freeze dryers GmbH, Germany) for 12h. The lower part containing chloroform with the lipid phase was dried overnight in open brown glass bottles under a fume hood.
Extracts of muscle and digestive gland tissues of P. maximus were prepared for NMR spectroscopy using a similar extraction protocol as described by (Tripp-Valdez et al., 2017; Götze et al., 2020). Around 60 mg of grounded frozen tissue was taken and homogenized in methanol/water using a cell homogenizer (Precellys 24, Bertin Technology, France). In the second step, chloroform/water was added, vortexed, and settled on ice for 10 minutes. After centrifugation for 10 min at 3000 rpm and 4°C, the upper and lower layers were separated and dried as described for the algae extraction. The dried samples were stored in a fridge at -80°C until further processing.
A simple feeding experiment was designed for the giant scallop, Pecten maximus, under laboratory conditions (indoor simulated moderate-cold (15°C) and moderate-warm temperatures (20°C), in accordance with Bock et al., 2019. Both temperatures are typical temperatures, which these species experience during seasonal changes on the Atlantic coast.
For the feeding experiments, three individuals were placed in three separate water containers with a defined volume of 10 liters. The containers were continuously aerated with air and kept at their respective temperatures (15°C and 20°C, respectively). After one day of acclimation under starvation, a defined amount (4*109 cells) of 13C-labeled algae was added to each single container. Scallops were fed daily for 3 days. It was ensured that the animals were open during feeding and thus had the opportunity to filter feed. After the feeding experiments, the animals were sacrificed, and samples from the digestive gland and striated muscle tissue were snap-frozen in liquid nitrogen and stored at -80°C for later analysis.
The prepared extracts were analyzed to incorporated 13C-labeled compounds using 1H- and 13C-NMR spectroscopy. Using a methanol/chloroform protocol allowed us to analyze the cytosolic and lipid fractions within one sample, as described in (Amiel et al., 2020). In this way, we could detect carbohydrate and amino acid energy sources located in the cytosol and, in addition, the main and essential fatty acids located in the lipid fraction. The dried methanol phase, containing the cytosolic fraction of algae and tissue extracts, was dissolved in deuterated water (D2O) containing 0.05% trimethylsilylpropionic acid (TSP, Sigma-Aldrich, USA) as chemical shift standard. The dried lipid fractions were dissolved in deuterated chloroform (CDCL3) containing 0.03% tetramethylsilane (TMS) as the chemical shift standard for the lipid phase. All dried tissue samples were dissolved with a 1:1 ratio (initial tissue weight to volume) and transferred into 1.7 mm NMR tubes. Algae were either measured as a crude extract in the NMR spectrometer similar to Bock et al., 2024, or 2*108 cells/mL were extracted (see above) and re-suspended in 60μL D2O.
All NMR measurements were conducted on a wide bore vertical 9.4T NMR spectrometer (400 MHz) with Avance III HD electronics (Bruker Biospin, Germany). All samples were measured with a 1.7 mm diameter triple-tuned (1H-13C-15N) probe at room temperature (21°C).
For 1H-NMR measurements of cytosol samples, as well as for the measurements of the lipid phase, a classical Carr-Purcell-Meiboom-Gill, including f1 presaturation pulse sequence, was used. Subsequently, after each 1H-NMR measurement, 13C-enrichment NMR studies were performed using a 1D pulse and acquired sequence with gradient enhancement.
The acquisition parameters for the NMR measurements were as follows:
1H-NMR spectroscopy: pulse program: Bruker protocol cpmgpr1d: Acquisition time (AQ) 4.01s, time domain (TD) 70656, sweep width (SW) 8802 Hz (22 ppm), delay (D1) 4s, dummy scan (DS) 4, number of scans (ns) 256.
13C-enrichment NMR spectroscopy: pulse program: Bruker protocol zgig: TD 65536, SW 22055 Hz (220 ppm), AQ 1.485 s, D1 4s, DS 2, ns 8192.
1H- and 13C-NMR spectra were acquired from algae cultures raised under 1 mM and 3 mM concentrations of 13C-labeled bicarbonate to find the optimal incorporation of the 13C-label into the chemical compounds of P. tricornutum (n=3, respectively). NMR spectra of the cytosolic and lipid phases from tissue extracts from the muscle and digestive gland of P. maximus after feeding at the two temperatures (n=3, respectively) were acquired using 1H- and C13-NMR spectroscopy.
All NMR spectra were processed and analyzed using Bruker’s NMR spectrometer software TopSpin 3.1 pl. 1H-NMR spectra were zero-filled to 8k and processed using an exponential multiplication with a lb factor 0.5. Spectra were automatically phased, and the baseline was corrected using the TopSpin routine. 13C-NMR spectra were zero-filled to 32k, and the first 350 points of the free induction decay (FID) were discarded before Fourier transformation. Afterwards, an exponential multiplication on the FID with a line broadening (lb) factor of 5 was applied, the spectrum was automatically phased, and the baseline was corrected. All spectra were calibrated to TSP or TMS to 0.0 ppm, respectively. Integrals from selected 13C-labeled signals from 13C-NMR spectra were calculated using the integral routine within TopSpin to determine relative concentration changes. The noise levels for the 13C-NMR spectra of cytosol were set to a constant level; for the spectra of the lipid phase, the chloroform signal was set to a constant integral to assure a maximum comparability of integrals between spectra from different individuals. As a note, metabolite concentrations from 13C-NMR signals for comparisons between spectra can be achieved via the natural abundance of the 13C-NMR signal of the solvent as an internal calibration standard. In the case of chloroform, however, possible evaporation must be considered. Therefore, measuring the samples directly and consecutively is advisable to minimize the relative error. Integrals are given in arbitrary units of concentration and were saved in a csv file for comparison and further statistical analysis. The NMR spectra for the figures were prepared using MestReNova 14.2.0 (Mestrelab Research S. L., Santiago de Compostela, Spain).
13C-NMR signals were assigned to specific metabolites and fatty acids according to NMR libraries from the literature (Spectral Database for Organic Compounds (SDBS), https://sdbs.db.aist.go.jp/sdbs/cgi-bin/cre_index.cgi; Human Metabolome Database (HMDB), https://hmdb.ca/). In addition, 1H-13C-HSQC spectra were performed on some samples to confirm the identification of metabolites and lipid signals within the 1H- and 13C-NMR spectra (see Supplementary Figure S1).
Statistically significant differences between the integrals of 13C-labeled NMR signals of metabolites in the two temperature groups were determined using linear models. The linear models (function lm) were applied to the temperature groups using the statistical programming environment R (R Core Team, 2022), according to the tutorial by Winter (2013).
The main aim of this study was to implement 13C-enrichment NMR spectroscopy to detect trophic markers and track trophic linkages. To this end, under laboratory conditions, a simple feeding experiment was designed for the King scallop, Pecten maximus. Figure 1 compares 13C-NMR spectra of algal extracts grown in media containing 1 mM and 3 mM 13C-labeled bicarbonate, respectively. All 13C-NMR spectra show a variety of 13C-labeled NMR signals. The direct comparison of the spectra reveals an elevation of the most prominent signals in the 13C-NMR spectra from the algae extracts incubated in 3 mM 13C-labeled bicarbonate media. This is clear evidence for higher incorporation of 13C-atoms into metabolites and lipids with increasing concentration of 13C-labeled bicarbonate in the growth medium. All spectra were acquired with the same acquisition parameters and processed identically for a direct comparison. Despite the differences in concentration, no additional signals could be observed in the individual spectra, indicating that the incorporation of 13C depends on the metabolization and relies on the concentration of 13C-labeled bicarbonate. The contribution of 13C-signals from compounds with natural abundance can be neglected with the selected measurement parameters. This can be concluded from the relatively low signal-to-noise ratio of the algae extracts obtained from P. tricornutum incubated in the growth medium with 1 mM 13C-labeled bicarbonate, which is close to the detection limit (Figures 1A, C). Following the literature and to achieve the best possible incorporation of 13C into the algae, a concentration of 3 mM 13C-labeled bicarbonate was chosen for the upcoming feeding experiments.
Figure 1. Comparison of 13C-NMR spectra from Phaeodactilum tricornutum extracts grown in media with 1 mM (upper spectra) and 3 mM 13C-labeled bicarbonate (lower spectra). The cytosolic fraction is displayed in (A+B), and the lipid phase is in (C+D). The natural abundance of 13C-NMR signals from the solvent chloroform (CHCL3) were used to calibrate and scale the NMR spectra.
In total, 13C-labeled signals of seven main metabolites could be unambiguously assigned in the 13C-NMR spectrum of the cytosolic phase of P. tricornutum, which belongs to glucose and amino acids (see Table 1). The metabolites identified are organic osmolytes, typically appearing in considerable concentrations in 13C-NMR spectra of algae (e.g. Karsten et al., 1995). The signal pattern of the 13C-enrichment NMR spectrum of the lipid phase from the algae compares very well with typical 13C-spectra from classical oils, which are standardly used for the evaluation of 13C-NMR lipid measurements (e.g. Hwang et al., 2003; Amiel et al., 2020).
Table 1. Identified 13C-labeled NMR signals of the most prominent metabolites in the cytosolic extract phase of Phaeodactilum tricornutum.
13C-NMR spectra of fatty acids can be very similar, which makes it difficult to assign the signals to specific individual fatty acids. Therefore, in lipid analyses using NMR spectroscopy, only the ratios of the functional carbon groups are often given. In the present study, the 13C-NMR spectra of the most abundant fatty acids in P. tricornutum were taken from the literature (see Qiao et al., 2016) and compared with the labeled signals from the 13C-NMR spectra of the lipid phase. The five fatty acids taken from the literature with a proportion of more than 5% in the late linear growth phase of P. tricornutum could also be assigned from the 13C-NMR spectra (Qiao et al., 2016, see Table 2). In particular, the signals of the polyunsaturated omega-3 fatty acid eicosapentaenoic acid (EPA) in P. tricornutum could be identified in the 13C-NMR spectrum of the lipid phase of P. tricornutum (see bold numbers in Table 2), confirming the exceptionally high content of EPA of more than 30% in P. tricornutum during the late linear growth phase (Qiao et al., 2016; Ding et al., 2023).
Table 2. Chemical shift values of the functional groups of 13C-labeled fatty acids identified in the lipid extract phase of Phaeodactilum tricornutum.
The uptake and incorporation of 13C-labeled atoms from P. tricornutum into the digestive gland and muscle tissue of the King scallop P. maximus could be tracked clearly by 13C-enrichment NMR spectroscopy. Figure 2 shows the 13C-NMR spectra of the cytosolic and lipid phases obtained from the digestive gland (DG) tissue of P. maximus after feeding with unlabeled and 13C-labeled P. tricornutum. In the scallops spectra of the digestive gland fed with unlabeled P. tricornutum, there was a visible contribution of naturally abundant 13C-NMR signals from metabolites in the cytosolic and lipid phases (Figures 2A, C). However, the comparison with the spectra of the digestive gland of scallops fed with 13C-labeled algae shows a substantial enrichment in these naturally abundant 13C-NMR signals (Figures 2B, D), suggesting that these metabolites are preferentially synthesized. Interestingly, irrespective of the extract phase, only a few additional signals appeared in the 13C-NMR spectra after feeding with labeled algae. These extra signals belong most likely to the digestive gland’s specific metabolite pool, which was previously masked by noise and now became visible due to the increased incorporation of the 13C-label.
Figure 2. Comparison of 13C-NMR spectra of the specific cytosolic and lipid phases from digestive gland tissue of the scallop Pecten maximus fed with unlabeled algae (A+C) and after feeding with 13C-labeled Phaeodactilum tricornutum (B+D). Note the increase in 13C-NMR signals in the digestive gland tissue spectra of the scallop fed with 13C-labeled algae. Note the additional signals of the C1-atoms of the amino acids. The natural abundance of 13C-NMR signals from the solvent chloroform (CHCL3) and the noise level were used for scaling the NMR spectra.
A comparison of the 13C-enrichment NMR spectra from the digestive gland of scallops with the spectra of 13C-labeled algae indicates some substantial differences. The signals match between 45-80 ppm, while in the range between 170-180 ppm, the digestive gland shows additional signals compared to the algae spectra. In addition to the metabolites identified in P. tricornutum, the amino acids taurine, threonine and succinate could be assigned in the spectrum of the digestive gland of scallops fed with 13C-labeled algae. This is particularly visible in the NMR signals of the C1 atoms of amino acids around 170-180 ppm (Figure 2B, Table 1), which are not detected in P. tricornutum spectra. This is evidence of incorporating the 13C-label into de novo synthesized amino acids. The 13C-NMR spectrum from the cytosol of the muscle was almost identical to the digestive gland spectrum except for additional signals from the amino acid l-arginine (at 155 ppm; see spectra in the supplement). This result is not surprising, as phosphor-l-arginine is provided in the phasic muscle for rapid energy supply, for example, during swimming (e.g. Bock et al., 2019) and is therefore found in high concentrations in the phasic muscle of P. maximus compared to other tissues (e.g. Bock et al., 2024). All identified metabolites correspond very well with the literature data from metabolomics studies of digestive gland and muscle tissue from bivalves, including scallops (Cappello et al., 2018; Bock et al., 2024). Contrasting to the cytosol phase, the comparison of the 13C-NMR lipid spectra revealed no additional signals between the spectra obtained from algae and scallops. Indeed, the 13C-enriched NMR spectrum from the lipid phase of the digestive gland is very comparable with the spectrum of the lipid phase of P. tricornutum. In particular, the signals of the essential unsaturated fatty acids now appear prominently in the 13C-spectrum of the digestive gland (see region between 128-132 ppm in Figure 2D, Table 2). In contrast, the 13C-enrichment spectra of the lipid phase of muscle spectra showed no incorporation of 13C-labeled atoms (see Supplementary Figure S2).
The increased 13C-NMR signals in the labeled 13C-NMR spectra may arise from three contributors: 1. Signals from naturally abundant metabolites of the tissue. 2. Signals that belong to ingested 13C-labeled algae that were not removed during the extraction process and contributed to the 13C-NMR spectrum of the digestive gland; and 3. 13C-NMR signals that arise from de novo synthetized metabolites. The contribution from naturally abundant metabolites is unlikely, as the signals between the labeled and unlabeled digestive glands are too different in intensity to be explained by variations between individuals only. A possible signal contribution of undigested algae remaining that are still present in the digestive gland, is rather unlikely. During the extraction process (see M&M), the remaining undigested algae will most likely be removed. In addition, potential contamination cannot explain the increase in 13C-labels in amino acids that are not present in the cytosolic 13C-NMR spectrum of P. tricornutum. Furthermore, increased 13C-labeled signals were also observed in muscle tissue, where side effects due to contamination of undigested algae can be ruled out (see Supplementary Figure S2). Therefore, all indications support a de novo synthesis of metabolites in the scallops, especially of amino acids. However, a potential contribution by undigested algae in the spectra of the digestive gland can be regarded as insignificant.
The situation of the 13C-enriched NMR spectrum of the lipid phase differs from that of the cytosol. Here, very distinct signals from essential fatty acids of the algae appear. These signals are particularly visible in the 128-132 ppm range when comparing Figures 1D and 2C, D. These 13C-NMR signals can be attributed to the double-bonded carbon of unsaturated fatty acids (see above), in the case of P. tricornutum mainly to the polyunsaturated fatty acid EPA. Essential fatty acids such as EPA are necessary for the survival and growth of bivalves (da Costa et al., 2015). It is still unclear, however, whether scallops can directly synthesize essential fatty acids such as EPA (Knauer and Southgate, 1999), but it is well known that essential fatty acids can be selectively assimilated in scallops if the diet is unlimited (da Costa et al., 2015). EPA can, therefore, be identified as an unequivocal trophic marker for scallops, as shown in, e.g. Daphnia magna (Sperfeld and Wacker, 2009, 2011). In this way, the signals between 128-132 ppm in the 13C-NMR spectra of the lipid phase can be used as indicators for trophic markers. Interestingly, in the lipid phase of the phasic muscle, neither naturally abundant 13C-NMR signals nor labelled signals can be detected (see figure in supplement). This can be explained on the one hand by the fact that the lipid content in the phasic muscle of P. maximus is very low (Topić Popović et al., 2020), and on the other hand by the metabolism of the phasic muscle of P. maximus. The phasic muscle of scallops requires energy more immediately, e.g. for escape swimming. Since carbohydrate oxidation has a higher ATP synthesis rate than fatty acid oxidation (Hargreaves and Spriet, 2020), it is quite conceivable that fatty acids are only incorporated slowly or to a small extent. In addition, Guderley and co-workers who investigated isolated mitochondria from the phasic muscle of the tropic scallop Euvola ziczac, could show that carbohydrates rather than fatty acids are used for energy production (Guderley et al., 1995). This may explain the lack of incorporation of 13C-labeled atoms in fatty acids and the generally low to non-detection of 13C-atoms in the lipid phase of phasic muscle tissue under the present experimental conditions.
After discussing the applicability of 13C-enrichment NMR spectroscopy in a simple feeding experiment of 13C-labeled algae into bivalves, the next paragraph deals with our second approach to identify temperature-induced changes using metabolic substrates and energy conversion. Figure 3 presents 13C-NMR spectra from the cytosolic fraction of the phasic muscle of P. maximus after feeding with 13C-labeled algae at different temperatures, 15°C vs 20°C, respectively. At first glance, there are no major differences between the spectra from the cytosolic phase of the phasic muscle between the two temperatures. A closer inspection, however, showed a few but remarkable differences in the cytosolic fraction of the muscle tissue fed at 15°C compared with those fed at 20°C. In particular, there is an increase of a signal at 155 ppm (see bracket box in Figure 3) at 20°C in the cytosolic fraction of the phasic muscle, which can be attributed to the C6-atom of l-arginine and two more pronounced small signals in the region between 70 and 80 ppm (albeit not significant, see supplement for statistics). Carbon atoms of sugars, such as alpha- and beta-glucose, can be typically identified in this region of the 13C-NMR spectrum (70-80 ppm, see Table 1).
Figure 3. 13C-NMR spectra from the cytosolic fraction of scallop muscle tissue. Scallops were fed with 13C-labeled algae at 20°C (A) and 15°C (B). Spectra are the sum of spectra from three individual scallops from both groups. The bracket box points to the increased 13C-labeled signal at 155 ppm in the muscle tissue from scallops fed at 20°C. That signal is significantly elevated and could be attributed to l-arginine.
It is well known from the literature that scallops such as P. maximus have higher metabolic costs at higher temperatures (e.g. Schalkhausser et al., 2014), reflected in higher filtration rates. In the adductor muscle, particularly the phasic muscle, higher metabolic costs, such as swimming, are compensated via increased use of phospho-l-arginine (PLA) to fuel ATP (Tremblay et al., 2012). The 13C-label under sufficient food availability will be increasingly incorporated into l-arginine at the beginning of moderately higher temperatures to prepare for this under routine conditions. In the case of longer-term warming conditions and energy requirements, however, there is a reduction in PLA and l-arginine (Bock et al., 2019, 2024). Another compensation process for the increased energy requirement at warmer temperatures is the increased incorporation of carbohydrates such as glucose (signals at 76.2 and 78.0 ppm). These are then utilized for energy production in the mitochondria of the muscle (see above, Guderley et al., 1995). In addition, bivalves can show a seasonal-dependent preferred use of carbohydrates in summer at higher temperatures and fatty acids in autumn and winter at lower temperatures, as recently has been shown for Pecten jacobeus (Topić Popović et al., 2020). Furthermore, the triglyceride content in the muscle is an order of magnitude lower than in the digestive gland in P. jacobeus (Topić Popović et al., 2020). A scallop species that is closely related to P. maximus. The increased 13C-content on the sugars of the muscle tissue indicates that these sugars are incorporated from 13C-labeled algae. Therefore, the increased filtration rate at 20°C and higher energy demand at 20°C may explain the increased incorporation rate of the 13C-label into sugars in the muscle tissue of scallops from the 20°C group.
Figure 4 compares 13C-NMR spectra from the lipid fraction of the digestive gland from scallops fed at 15°C and 20°C. Here, the broad signal cluster at 127-129 ppm and the prominent signal cluster around 25 ppm are significantly higher in the 15°C spectrum than the sum spectrum at 20°C (see supplement for statistics). Together with the signals at 20 ppm and a signal at 14 ppm, these signals can be attributed to the polyunsaturated fatty acid EPA (see Table 2). EPA as a classical essential fatty acid was also identified in the 13C-NMR spectra from P. tricornutum extracts (Figure 1). As described above, EPA is an unequivocal trophic marker (Sperfeld and Wacker, 2009, 2011).
Figure 4. 13C-NMR spectra from the lipid fraction of scallop digestive gland tissue. Scallops were fed with 13C-labeled algae at 20°C (A) and 15°C (B). Spectra are the sum of three spectra from three individual scallops from both groups. The most notable changes of 13C-labeled atoms in the digestive gland from scallops can be observed inside the bracket boxes. Note the prominent decrease of double-bound 13C-atoms around 129 ppm, reflecting the double bonds of unsaturated fatty acids (in particular EPA, Table 2).
The higher levels of unsaturated fatty acids found in the digestive gland of the 15°C group can be explained by selectively incorporating more unsaturated fatty acids from the algae into their digestive gland than the 20°C group (da Costa et al., 2015). This interpretation is supported by the fact that scallops use more fatty acids for energy production at colder temperatures. A phenomenon that can be observed across the animal kingdom. At warmer temperatures, carbohydrates are used for energy provision, as shown for the tropical scallop E. ziczac (Guderley et al., 1995) and supported by the increased 13C-NMR glucose signals in the muscle tissue at 20°C (see above). Furthermore, unsaturated fatty acids are incorporated into cellular membranes at colder temperatures to keep the fluidity of cell membranes. A phenomenon frequently reported for polar organisms is called homeoviscious adaptation (Sinensky, 1974). However, it is most unlikely that it is necessary to modify the membrane fluidity in a moderate temperature difference range from 15° to 20°C. In addition, temperature-induced adaptations to membrane composition to keep membrane fluidity should affect all tissues. However, we did not observe such changes in scallop muscle tissue. Therefore, the observed increase in unsaturated fatty acids is more likely to be explained as a reserve for energy provision. This was also suggested in a recent metabolomic study on the impact of Ocean warming and acidification on the gills, mantle and adductor muscle of P. maximus (Bock et al., 2024). In summary, our simple experiment demonstrates the potential of NMR experiments with 13C-enrichment to detect environmentally induced changes in metabolic pathways (e.g. Lannig et al., 2010; Tikunov et al., 2014) or the specific use of metabolic substrates (Guderley et al., 1995) in laboratory experiments.
We showed that 13C-enrichment NMR spectroscopy can be used to monitor the uptake of 13C-labeled algae in filtration feeders. We could identify trophic markers in a very simple experiment with high reproducibility. The experimental approach indicates that besides the accumulation of trophic markers, their use and conversion might be an additional important factor for the reliable interpretation of trophic linkages under climate change scenarios. The experimental approach should be easily transferable to other and more complex systems, such as predator-prey interactions under more complex climate change scenarios. It should also be practicable in the field.
The datasets presented in this study can be found in online repositories. The names of the repository/repositories and accession number(s) can be found below: https://www.pangaea.de/.
Ethical approval was not required for the study involving animals in accordance with the local legislation and institutional requirements because it is not required for bivalves according to german legislature.
CB: Conceptualization, Data curation, Methodology, Project administration, Supervision, Writing – original draft, Writing – review & editing, Formal analysis. TZ: Investigation, Writing – original draft. SG: Investigation, Writing – review & editing. FW: Formal analysis, Visualization, Writing – review & editing. GL: Data curation, Formal Analysis, Writing – review & editing.
The author(s) declare financial support was received for the research, authorship, and/or publication of this article. The project was supported by the research program “Changing Earth—Sustaining our Future” in the program-oriented funding periods (PoF IV subtopic 6.2—Adaptation of marine life: from genes to ecosystems) of the Helmholtz Association.
We thank Charlotte Eymann, Fredy Véliz Moraleda and Dr. Barbara Niehoff for their advice and support during the rearing and 13C-incubation of P. tricornutum. Anette Tillmann and several trainees assisted with NMR measurements and analysis. The project was supported by the research program “Changing Earth—Sustaining our Future” in the program-oriented funding periods (PoF IV subtopic 6.2—Adaptation of marine life: from genes to ecosystems) of the Helmholtz Association. An earlier version of the results was presented as part of the IAEA CRP on “Applied radioecological tracers to assess coastal and marine ecosystem health” (K41019) at the IAEA Marine Environment Laboratories.
The authors declare that the research was conducted in the absence of any commercial or financial relationships that could be construed as a potential conflict of interest.
The author(s) declared that they were an editorial board member of Frontiers, at the time of submission. This had no impact on the peer review process and the final decision.
All claims expressed in this article are solely those of the authors and do not necessarily represent those of their affiliated organizations, or those of the publisher, the editors and the reviewers. Any product that may be evaluated in this article, or claim that may be made by its manufacturer, is not guaranteed or endorsed by the publisher.
The Supplementary Material for this article can be found online at: https://www.frontiersin.org/articles/10.3389/fmars.2024.1446998/full#supplementary-material
Amiel A., Tremblay-Franco M., Gautier R., Ducheix S., Montagner A., Polizzi A., et al. (2020). Proton NMR enables the absolute quantification of aqueous metabolites and lipid classes in unique mouse liver samples. Metabolites. 10, 9. doi: 10.3390/metabo10010009
Bligh E. G., Dyer W. J. (1959). A rapid method of total lipid extraction and purification. Can. J. Biochem. Physiol. 37, 911–917. doi: 10.1139/y59-099
Bock C., Goütze S., Poürtner H. O., Lannig G. (2024). Exploring the mechanisms behind swimming performance limits to ocean warming and acidification in the Atlantic king scallop, Pecten maximus. Front. Ecol. Evol. 12. doi: 10.3389/fevo.2024.1347160
Bock C., Wermter F. C., Schalkhausser B., Blicher M. E., Pörtner H. O., Lannig G., et al. (2019). In vivo31P-MRS of muscle bioenergetics in marine invertebrates: Future ocean limits scallops’ performance. Magn. Reson. Imaging. 61, 239–246. doi: 10.1016/j.mri.2019.06.003
Cappello T., Giannetto A., Parrino V., Maisano M., Oliva S., De Marco G., et al. (2018). Baseline levels of metabolites in different tissues of mussel Mytilus galloprovincialis (Bivalvia: Mytilidae). Comp. Biochem. Physiol. Part. D. Genomics Proteomics. 26, 32–39. doi: 10.1016/j.cbd.2018.03.005
Cresswell T., Metian M., Fisher N. S., Charmasson S., Hansman R. L., Bam W., et al. (2020). Exploring new frontiers in marine radioisotope tracing-Adapting to new opportunities and challenges. Front. Mar. Sci 7, 406. doi: 10.3389/fmars.2020.00406
da Costa F., Robert R., Quéré C., Wikfors G. H., Soudant P. (2015). Essential fatty acid assimilation and synthesis in larvae of the bivalve crassostrea gigas. Lipids 50, 503–511. doi: 10.1007/s11745-015-4006-z
Ding W., Ye Y., Yu L., Liu M., Liu J. (2023). Physiochemical and molecular responses of the diatom Phaeodactylum tricornutum to illumination transitions. Biotechnol. Biofuels bioproducts. 16, 103. doi: 10.1186/s13068-023-02352-w
Gauzens B., Rosenbaum B., Kalinkat G., Boy T., Jochum M., Kortsch S., et al. (2024). Flexible foraging behaviour increases predator vulnerability to climate change. Nat. Clim. Change 14, 387–392. doi: 10.1038/s41558-024-01946-y
Götze S., Bock C., Eymann C., Lannig G., Steffen J. B. M., Pörtner H. O. (2020). Single and combined effects of the “Deadly trio” hypoxia, hypercapnia and warming on the cellular metabolism of the great scallop Pecten maximus. Comp. Biochem. Physiol. B Biochem. Mol. Biol. 243-244, 110438. doi: 10.1016/j.cbpb.2020.110438
Grunst M. L., Grunst A. S., Grémillet D., Fort J. (2023). Combined threats of climate change and contaminant exposure through the lens of bioenergetics. Global Change Biol. 29, 5139–5168. doi: 10.1111/gcb.16822
Guderley H. E., Rojas F. M., Nusetti O. A. (1995). Metabolic specialization of mitochondria from scallop phasic muscles. Mar. Biol. 122, 409–416. doi: 10.1007/BF00350873
Guillard R. R. L. (1975). “Culture of phytoplankton for feeding marine invertebrates,” in Culture of marine invertebrate animals. Eds. Smith W. L., Chanley M. H. (Springer, Boston, MA).
Hargreaves M., Spriet L. L. (2020). Skeletal muscle energy metabolism during exercise. Nat. metabol 2, 817–828. doi: 10.1038/s42255-020-0251-4
Hays G. C., Richardson A. J., Robinson C. (2005). Climate change and marine plankton. Trends Ecol. Evolution. 20, 337–344. doi: 10.1016/j.tree.2005.03.004
Hobson K. A. (2023). Stable isotopes and a changing world. Oecologia. 203, 233–250. doi: 10.1007/s00442-023-05387-w
Hwang J. H., Bluml S., Leaf A., Ross B. D. (2003). In vivo characterization of fatty acids in human adipose tissue using natural abundance 1H decoupled 13C MRS at 1.5 T: clinical applications to dietary therapy. NMR Biomed. 16, 160–167. doi: 10.1002/nbm.824
Karsten U., Bock C., West J. A. (1995). 13C-NMR spectroscopy as a tool to study organic osmolytes in the mangrove red algal genera Bostrychia and Stictosiphonia (Ceramiales). Phycol Res. 43, 241–247. doi: 10.1111/j.1440-1835.1995.tb00030.x
Knauer J., Southgate P. C. (1999). A review of the nutritional requirements of bivalves and the development of alternative and artificial diets for bivalve aquaculture. Rev. Fish. Sci. 7, 241–280. doi: 10.1080/10641269908951362
Kortsch S., Primicerio R., Fossheim M., Dolgov A. V., Aschan M. (2015). Climate change alters the structure of arctic marine food webs due to poleward shifts of boreal generalists. Proc. Biol. Sci. 282, 20151546. doi: 10.1098/rspb.2015.1546
Lannig G., Eilers S., Pörtner H. O., Sokolova I., Bock C. (2010). Impact of Ocean Acidification on Energy Metabolism of Oyster, Crassostrea gigas. Changes in metabolic pathways and thermal response. Mar. Drugs 8, 2318–2339. doi: 10.3390/md8082318
Morris P., Bachelard H. (2003). Reflections on the application of 13C-MRS to research on brain metabolism. NMR Biomed. 16, 303–312. doi: 10.1002/nbm.844
Paerl H. W., Scott J. T. (2010). Throwing fuel on the fire: Synergistic effects of excessive nitrogen inputs and global warming on harmful algal blooms. Environ. Sci. Technology. 44, 7756–7758. doi: 10.1021/es102665e
Pasquaud S., Lobry J., Elie P. (2007). Facing the necessity of describing estuarine ecosystems: a review of food web ecology study techniques. Hydrobiologia. 588, 159–172. doi: 10.1007/s10750-007-0660-3
Peterson B. J., Fry B. (1987). Stable isotopes in ecosystem studies. Annu. Rev. Ecology Evolution Systematics. 18, 293–320. doi: 10.1146/annurev.es.18.110187.001453
Pinsky M. L., Selden R. L., Kitchel Z. J. (2020). Climate-driven shifts in marine species ranges: scaling from organisms to communities. Ann. Rev. Mar. Sci. 3, 153–179. doi: 10.1146/annurev-marine-010419-010916
Pörtner H. O., Bock C., Mark F. C. (2017). Oxygen-and capacity-limited thermal tolerance: bridging ecology and physiology. J. Exp. Biol. Cambridge University Press 220, 2685–2696. doi: 10.1242/jeb.134585
Pörtner H. O., Roberts D. C., Tignor M. M., Poloczanska E., Mintenbeck K., Nicolai M., et al. (2022). “The ocean and cryosphere in a changing climate,” in IPCC special report on the ocean and cryosphere in a changing climate (IPCC).
Qiao H., Cong C., Sun C., Li B., Wang J., Zhang L. (2016). Effect of culture conditions on growth, fatty acid composition and DHA/EPA ratio of Phaeodactylum tricornutum. Aquaculture. 452, 311–317. doi: 10.1016/j.aquaculture.2015.11.011
R Core Team. (2022). R: A language and environment for statistical computing (Vienna, Austria: R Foundation for Statistical Computing). Available at: https://www.R-project.org/.
Schalkhausser B., Bock C., Pörtner H. O., Lannig G. (2014). Escape performance of temperate king scallop, Pecten maximus under ocean warming and acidification. Mar. Biol. 161, 2819–2829. doi: 10.1007/s00227-014-2548-x
Shulman R. G., Rothman D. L. (2001). 13C NMR of intermediary metabolism: Implications for systemic physiology. Annu. Re. Physiol. 63, 15–48. doi: 10.1146/annurev.physiol.63.1.15
Sinensky M. (1974). Homeoviscous adaptation—a homeostatic process that regulates the viscosity of membrane lipids in Escherichia coli. Proc. Natl. Acad. Sci. United States America. 71, 522–525. doi: 10.1073/pnas.71.2.522
Sperfeld E., Wacker A. (2009). Effects of temperature and dietary sterol availability on growth and cholesterol allocation of the aquatic keystone species Daphnia. J. Exp. Biol. 212, 3051–3059. doi: 10.1242/jeb.031401
Sperfeld E., Wacker A. (2011). Temperature- and cholesterol-induced changes in eicosapentaenoic acid limitation of Daphnia magna determined by a promising method to estimate growth saturation thresholds. Limnology Oceanography 56, 1273–1284. doi: 10.4319/lo.2011.56.4.1273
Sokolova I. M., Frederich M., Bagwe R., Lannig G., Sukhotin A. A. (2012) Energy homeostasis as an integrative tool for assessing limits of environmental stress tolerance in aquatic invertebrates. Mar. Environ. Res. 79, 1–15. doi: 10.1016/j.marenvres.2012.04.003
Tan K., Ransangan J., Tan K., Cheong K. L. (2023). The impact of climate change on Omega-3 long-chain polyunsaturated fatty acids in bivalves. Crit. Rev. Food Sci. Nutr. 64, 11661–11671. doi: 10.1080/10408398.2023.2242943
Thompson P. A., Guo M., Harrison P. J., Whyte J. N. C. (1992). Effects of variation in temperature. II. On the fatty acid composition of eight species of marine phytoplankton. J. Phycol. 28, 488–497. doi: 10.1111/j.0022-3646.1992.00488.x
Tikunov A. P., Stoskopf M. K., Macdonald J. M. (2014). Fluxomics of the eastern oyster for environmental stress studies. Metabolites. 4, 53–70. doi: 10.3390/metabo4010053
Topić Popović N., Beer Ljubić B., Strunjak-Perović I., Babić S., Lorencin V., Jadan M., et al. (2020). Seasonal antioxidant and biochemical properties of the Northern Adriatic Pecten jacobaeus. PloS One 15, e0230539. doi: 10.1371/journal.pone.0230539
Tremblay I., Guderley H. E., Himmelman J. H. (2012). Swimming away or clamming up: the use of phasic and tonic adductor muscles during escape responses varies with shell morphology in scallops. J. Exp. Biol. 215, 4131–4143. doi: 10.1242/jeb.075986
Tripp-Valdez M. A., Bock C., Lucassen M., Lluch-Cota S. E., Sicard M. T., Lannig G., et al. (2017). Metabolic response and thermal tolerance of green abalone juveniles (Haliotis fulgens: Gastropoda) under acute hypoxia and hypercapnia. J. Exp. Mar. Biol. Ecol. 497, 11–18. doi: 10.1016/j.jembe.2017.09.002
Twining C. W., Taipale S. J., Ruess L., Bec A., Martin-Creuzburg D., Kainz M. J. (2020). Stable isotopes of fatty acids: current and future perspectives for advancing trophic ecology. Phil. Trans. R. Soc B 375, 20190641. doi: 10.1098/rstb.2019.0641
Winter B. (2013).Linear models and linear mixed effects models in R with linguistic applications. Available online at: http://arxiv.org/pdf/1308.5499.pdf.
Keywords: nuclear magnetic resonance, stable isotopes, 13C-labeling, food web, climate change
Citation: Bock C, Zhao T, Götze S, Wermter FC and Lannig G (2024) 13C-enrichment NMR spectroscopy: a tool to identify trophic markers and linkages. Front. Mar. Sci. 11:1446998. doi: 10.3389/fmars.2024.1446998
Received: 10 June 2024; Accepted: 05 November 2024;
Published: 25 November 2024.
Edited by:
Paco Bustamante, Université de la Rochelle, FranceReviewed by:
Andrey Tikunov, University of North Carolina at Chapel Hill, United StatesCopyright © 2024 Bock, Zhao, Götze, Wermter and Lannig. This is an open-access article distributed under the terms of the Creative Commons Attribution License (CC BY). The use, distribution or reproduction in other forums is permitted, provided the original author(s) and the copyright owner(s) are credited and that the original publication in this journal is cited, in accordance with accepted academic practice. No use, distribution or reproduction is permitted which does not comply with these terms.
*Correspondence: Christian Bock, Q2hyaXN0aWFuLkJvY2tAYXdpLmRl
Disclaimer: All claims expressed in this article are solely those of the authors and do not necessarily represent those of their affiliated organizations, or those of the publisher, the editors and the reviewers. Any product that may be evaluated in this article or claim that may be made by its manufacturer is not guaranteed or endorsed by the publisher.
Research integrity at Frontiers
Learn more about the work of our research integrity team to safeguard the quality of each article we publish.