- 1Magíster en Ciencias Mención Oceanografía, Universidad de Concepción, Concepción, Chile
- 2Departamento de Oceanografía y Centro de Investigación Oceanográfica en el Pacífico Sur-Oriental (COPAS COASTAL), Facultad de Ciencias Naturales y Oceanográficas, Universidad de Concepción, Concepción, Chile
- 3Laboratorio de Toxicología Acuática (LATAC), Departamento de Oceanografía, Facultad de Ciencias Naturales y Oceanográficas, Universidad de Concepción, Concepción, Chile
The effect of the combination of marine toxins produced by algal blooms, in conjunction with varying environmental characteristics on organisms in the water column, is a poorly explored research field. Pelagic fish species of commercial importance, such as anchoveta (Engraulis ringens) in central Chile, may be exposed to these combined factors in a climate change scenario. This is observed from documented changes in the length of the upwelling season, frequency of upwelling events, and the increased frequency of atmospheric rivers affecting the southern spawning zone of this species. This study evaluated the integrity of hair cells in neuromasts, mechanosensory organs present in fish larvae, under exposure to different combinations of the algal bloom-produced okadaic acid OA (1 ng mL−1), and two temperature (12 and 14°C), and salinity treatments (historically more frequent: 34 PSU- 12°C; expected: 32 PSU - 14°C). Viable hair cells were counted in newly hatched larvae from the Biobío region, central Chile. Results showed a significant decrease in the average number of viable hair cells per neuromast (from 6.1 ± 1.6 to 4.0 ± 1.2) under lower salinity treatments (32 PSU) compared to normal conditions. Additionally, a seasonal trend was observed with fewer viable cells (from 7.4 ± 1.2 to 4.4 ± 1.1) as the fish species’ reproductive period progressed. The combined effect of OA exposure and modifications with the environmental factors also resulted in a significant decrease of up to 70% in the number of viable hair cells in larvae exposed to OA and high temperatures, indicating damage influenced by the toxin along with a synergistic and/or additive role of temperature. These findings reveal how the lipophilic toxin okadaic acid, produced by harmful algal blooms, interacts with abiotic environmental factors affecting coastal ecologically and socio-economically important organisms. This emphasizes the need to consider multiple factors when studying the effects of marine toxins.
1 Introduction
The oceans are experiencing significant alterations due to the global climate change, which is altering coastal dynamics and intensifying the frequency and magnitude of events such as harmful algal blooms (HABs) (Kim, 2006; Anderson et al., 2015; Trainer et al., 2020). Among the organisms that can thrive during an HAB event are microalgae producing biotoxins, and these, along with the biotoxins themselves, have become the subject of recent research (Christensen and Khan, 2020; Anderson et al., 2021). Variations in physicochemical parameters of the water column, such as temperature and salinity, can alter the frequency, intensity, and duration of these HABs, as well as the production and toxic effects of associated toxins on organisms from the water column (Hinder et al., 2012; Kibler et al., 2015; Salgado et al., 2016; Basti et al., 2018). These observations are relevant in the context of current global changes, such as global warming and coastal pollution. These changes might lead to an increase in both the periodicity and geographic coverage of HAB occurrences; they may even occur in places where they were not previously reported (Glibert et al., 2014; Wells et al., 2020).
Biotoxins are usually classified within three groups named according to their toxicity: paralytic toxins, amnesic toxins, or lipophilic toxins (formerly known as shellfish diarrheal toxins due to their effects on human health). Lipophilic toxins are produced by dinoflagellates from the genera Dinophysis and Prorocentrum. They correspond to polyether compounds, including okadaic acid (OA) and its analogues, dinophysistoxins (DTXs), as well as pectenotoxins (PTX), yessotoxins (YTX), and azaspiracids (AZA) (Sar et al., 2002; Miles et al., 2004; Paz et al., 2004, Tillman et al., 2009). OA has a cyclic polyether structure with 38 carbons, highly lipophilic, which confers low polarity, strong hydrophobicity, and high stability in the water column (Zhao et al., 2016). The mechanism of action of OA is based on its inhibition of the protein phosphatases PP1 and PP2A, which are involved in relevant dephosphorylation reactions at the systemic level. Among other processes, they participate in germ cell maturation, embryonic development, and metabolic regulation. Additionally, they play a key role in metabolic pathways such as glycolysis, lipid metabolism, and catecholamine synthesis (Reynhout and Janssens, 2019).
Globally, the monitoring of lipophilic toxins in the natural environment indicates that OA, DTX, and PTX2 are the most common, with a high detection rate in seawater samples compared to other toxins and are present in samples studied worldwide (He et al., 2020; Wang et al., 2023). Their presence has even been observed in suspended particles and seafood (Bosch-Orea et al., 2017; Chen et al., 2018). Variable concentrations of OA have been recorded in seawater; while in some years the minimum detected concentration of this toxin in Haizhou Bay (Yellow Sea, China; 37°28’N 119°26’E) was 0.01 ng mL−1, the maximum concentration reached around 0.1 ng mL−1 in other years (Chen et al., 2018). On the western coast of the Mediterranean Sea, the detection of OA has reached 1.78 ng mL−1 (Bosch-Orea et al., 2017). In all cases, there is a particularly high incidence in coastal areas during summer (Liu et al., 2017; Chen et al., 2018; Hattenrath-Lehmann et al., 2018; Wang et al., 2023). The persistence time of OA under natural sunlight varies, reaching up to 20 days in autumn and longer times in winter, a season with lower solar radiation (Pan et al., 2020).
On Chilean coasts (41°28’S 72°56’W), the use of passive samplers, such as synthetic resins that adsorb toxins from water in a similar way to an artificial bivalve (MacKenzie et al., 2004), has enabled the detection of lipophilic toxins including the OA (Pizarro et al., 2011; Díaz et al., 2022; Möller et al., 2022). The OA producer Dinophysis acuminata is one of the most frequently recorded HAB species in monitoring efforts along the entire Chilean coast (Díaz et al., 2022; Paredes-Mella et al., 2022), therefore the presence of OA in seawater represents a potential threat to organism in the coastal marine ecosystem. However, this threat is not frequently detected probably because most preventive studies focus on monitoring shellfish meat or phytoplankton samples rather than directly analyzing seawater (Anderson et al., 2021). Additionally, the abundance of cells of a specific species is not necessarily a reliable indicator of toxin concentration due to variations in toxin content among microalgae cells (Roué et al., 2018).
The anchovy (Engraulis ringens), a small pelagic fish distributed over a wide latitudinal range along the Humboldt Current from northern Peru (Zorritos 4°30′S) to southern Chile (Chiloé 42°30′S), represents one of the largest fisheries in the world (Castro et al., 2020). Previous studies have reported that variations in environmental parameters, such as temperature and salinity, can affect the yolk sac larval stage, the rate of yolk consumption, larval growth rate (Llanos-Rivera and Castro, 2006), as well as gene expression related to the hatching process, the expression of hatching enzymes, and the success of larval hatching (Castro et al., 2021). In recent years, surface temperature values from July to December, the main spawning season form most anchovies on the coasts of Bío Bío, Central Chile, have fluctuated between 12 and 14°C and surface salinity values range between 32 and 34 (Castro et al., 2020, 2021). However, these environmental conditions are currently experiencing changes. Extreme environmental events, such as marine heatwaves, are increasing in frequency, duration, and intensity in recent decades (Oliver, 2019), particularly in this region (González-Reyes et al., 2023). There is also a trend of an increase in salinity due to the intensification of coastal upwelling as a result of the displacement of the South Pacific high-pressure center and the average decrease in precipitation (Schneider et al., 2017; Jacob et al., 2018; Castro et al., 2020). In contrast, an increase in extreme rainfall events during transitional periods (fall, spring) due to atmospheric rivers has also been reported, events that may reach up to 45-60% of the total annual precipitation in the region (Bustos-Espinoza et al., 2024). Whether these environmental changes affect the most vulnerable early life stages of small pelagic fishes and other coastal organisms, has not been assessed yet.
Environmental variations present also significant challenges for toxic microalgae. Temperature and salinity are factors that regulate the presence and production of toxins as they determine the growth and proliferation of the microalgae that produce them (Kamiyama et al., 2010; Basti et al., 2018). Some authors indicate that fluctuations in the ocean’s abiotic parameters can trigger responses related to toxin production, both an increase and a decrease, by the microalgae (Kamiyama et al., 2010; Bui et al., 2021; Wang et al., 2022). On the other hand, the stability and high persistence of toxins such as OA in the water column represent a stressing factor for coexisting marine organisms with negative effects on their growth, metabolism, and swimming performance (Corriere et al., 2020; Pan et al., 2020).
Other OA effects on marine vertebrates may include disruption of functions of the sensory system. In early fish larvae these functions are associated to neuromasts; mechanosensory organs arranged on the body surface. The haircells, specialized sensory cells within the neuromast, when in contact with the surrounding water, detect water movements and translate them into nerve impulses transmitted by neurons, creating a larval coordinated response to changes in the aquatic environment (Ghysen and Dambly-Chaudière, 2004). This system plays an essential role in various vital activities, such as detecting predators, prey, or other objects in the environment, making these mechanosensory cells ideal models for assessing the potential toxic effects of environmental pollutants because alteration in these functions becomes ecologically relevant (Froehlicher et al., 2009; Hung et al., 2023).
The study of fish lateral line neuromasts has been widely employed in toxicology to assess potentially harmful substances such as antibiotics or heavy metals in freshwater fish (Froehlicher et al., 2009). However, the potential use of the lateral line in marine organisms in toxicological studies remains less explored. Like most Teleosts, anchovy larvae hatch with a set of neuromasts on their head and body, with their hair cells exposed to the ambient water (Llanos-Rivera et al., 2014). Due to their proliferative capacity, neuromasts form throughout the fish’s life, not just during its early developmental stages (Kasumyan, 2003). The location, number, and development of neuromasts are well documented for anchovy larvae in the Bío-Bío region (Llanos-Rivera et al., 2014) reared under normal environmental conditions. Thus, the evaluation of neuromast integrity can be used to study the effect that phytoplankton derived toxins in the water column such as OA may have on larvae of small pelagic fish, such as anchovy, especially considering future scenarios of environmental temperature and salinity variations. No studies have been reported on the assessment of the response capacity to environmental variables related to motor responses of the sensory system in these organisms. In this context, this study aims to assess, through neuromast analyses, how OA affects early life stages of fish, such as anchovy larvae, while assessing the role of oceanographic variables (temperature and salinity) in this interaction. This approach will allow us to deepen our understanding of how biotoxins affect marine ecosystems, especially in the context of global climate change.
2 Materials and methods
2.1 Description of the study area
Anchovy (Engraulis ringens) eggs were collected during their reproductive season in the area off Coliumo Bay, Bío-Bío region in central Chile, at 3 coastal stations (Figure 1, Table 1), an area where high abundances of eggs of this species have historically been reported in central Chile (Soto-Mendoza et al., 2010). To characterize the oceanographic environment in which these stages take place, CTD hauls (SBE-19plus) were carried out up to depths of approximately 50 meters (depending on the bottom depth of each station) to obtain salinity and temperature profiles.
2.2 Collection of biological material
A total of six zooplanktonic sampling cruises were carried out during the anchoveta spawning season between September and December 2023 (Table 1). To ensure an adequate number of eggs for each experiment (see below), between two and three oblique tows lasting 3 to 5 minutes each were conducted at each station using a bongo net (300 µm mesh, 60 cm mouth diameter), with the vessel maintained in motion at a speed of 2 to 3 knots. The depths of these tows varied depending on the station but most remained within the range of 20 to 40 meters deep, a depth range where anchovy eggs are usually found in this area (Castro et al., 2021). Water samples for laboratory incubations were collected at each station from 15 m using 5 L Niskin bottles.
Once each of the zooplankton samples was obtained, they were diluted in seawater from the same sampling area and individually stored in airtight plastic bags (27 × 28 cm). Each sample was stored in a sealed container and then rapidly transported to the laboratory at the University of Concepción. During transportation, the containers were maintained in the dark, avoiding temperature changes and abrupt movements, thereby ensuring the integrity of the plankton sample.
In the laboratory, using a stereoscopic microscope with a cold light source, the separation of eggs from the plankton was carried out. Early-stage eggs (Figures 2A, B) were then incubated in filtered seawater (0.2 microns) in 500 mL flasks, maintaining a density of 50 to 60 eggs per 100 mL. The samples were kept in an incubator chamber at 12°C, and the dead eggs were removed daily until larval hatching (Figure 2C). These recently hatched larvae were further utilized for all experimental treatments.
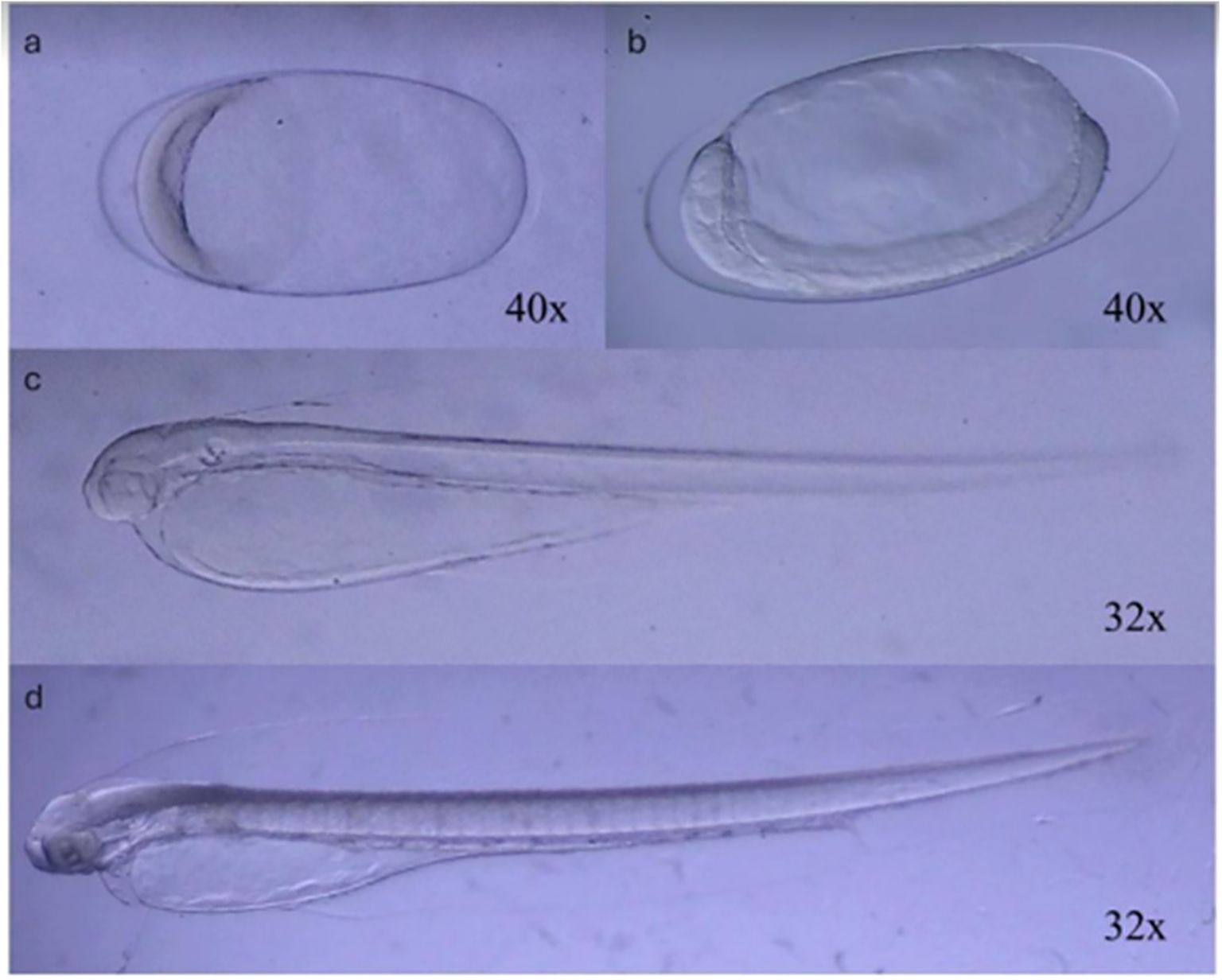
Figure 2. Developmental stages of Engraulis ringens observed during incubation. After the incubation of the eggs (A, B), the larvae (C, D) were observed during their growth until the stage defined for the setup of the experiments (D, partial absorption of the yolk sac).
Two sets of experiments were conducted (Table 1); the initial set (three first sampling dates) was made to standardize aspects of the experimental design (definition of positive control and appropriate OA concentration), and the second set consisted of three experiments with a complete experimental design (combinations of temperatures, salinities, and OA concentrations).
2.3 First set of experiments: standardization of methods
2.3.1 Standardization of neuromast cell counting as a method for damage assessment
In order to assess the direct interaction between organisms and the surrounding water, experiments were conducted by incubating anchovy larvae in filtered seawater (0.2 microns) prepared according to each of the treatments to be evaluated. Yolk sac larvae with approximately 50% of the yolk reserves already consumed (Figure 2D) were utilized to initiate all treatments. To achieve this, the larvae were monitored under a cold light magnifying glass, ensuring that they met the aforementioned conditions to start all treatments.
2.3.2 Positive control
A positive control was considered to be copper sulfate (CuSO4) at 50 μM, a concentration that has been shown to induce damage to neuromasts in other teleosts larvae (Hernández et al., 2006). Three replicates, each with 15 yolk sac larvae, were incubated in this solution for 20 minutes at a 12°C and 34 PSU in 12-well plates, with a density of 5 to 7 larvae per well. At the same time, an equal number of larvae were incubated in filtered seawater as a negative control, under the same experimental conditions.
To evaluate the structural integrity of the neuromasts, DiAsp staining (4-(4-diethylaminostyryl)-N-methylpyridinium iodide; SIGMA-Aldrich) was used. DiAsp is incorporated by cells that possess intact mechanotransduction channels, allowing visualization of those hair cells with active channels (Mardones, 2010; Nishikawa and Sasaki, 1996). After exposure to the experimental conditions, the larvae were exposed to DiAsp (5 ng mL−1 in seawater) for 10 minutes and then washed in seawater for another 10 minutes. Following staining, the larvae were anesthetized with 0.4% tricaine (Sigma Aldrich) and individually mounted on a slide with a coverslip provided with a stop of plasticine under a microscope.
A Nikon Eclipse E200 epifluorescence microscope was used, and under UV light, the larvae were observed individually. For each larva, live cells (identified as those showing positive staining with DiAsp) located in three lateral neuromasts (3, 4, 5) in the mid-body region of the larva (Figure 3A) were examined. These neuromasts were chosen following other authors who assessed functional neuromasts in other fish species (Hernández et al., 2006; Lin et al., 2023) and also because counting viable cells in neuromasts away from the yolk sac facilitates their examination (yolk autofluorescence interferes with the fluorescent signal of these structures). Following Hernández et al. (2006) those hair cells that tested positive for DiAsp were counted, and only those that exhibited uniform staining and a morphology similar to that of a flower petal were considered (Figure 3B).
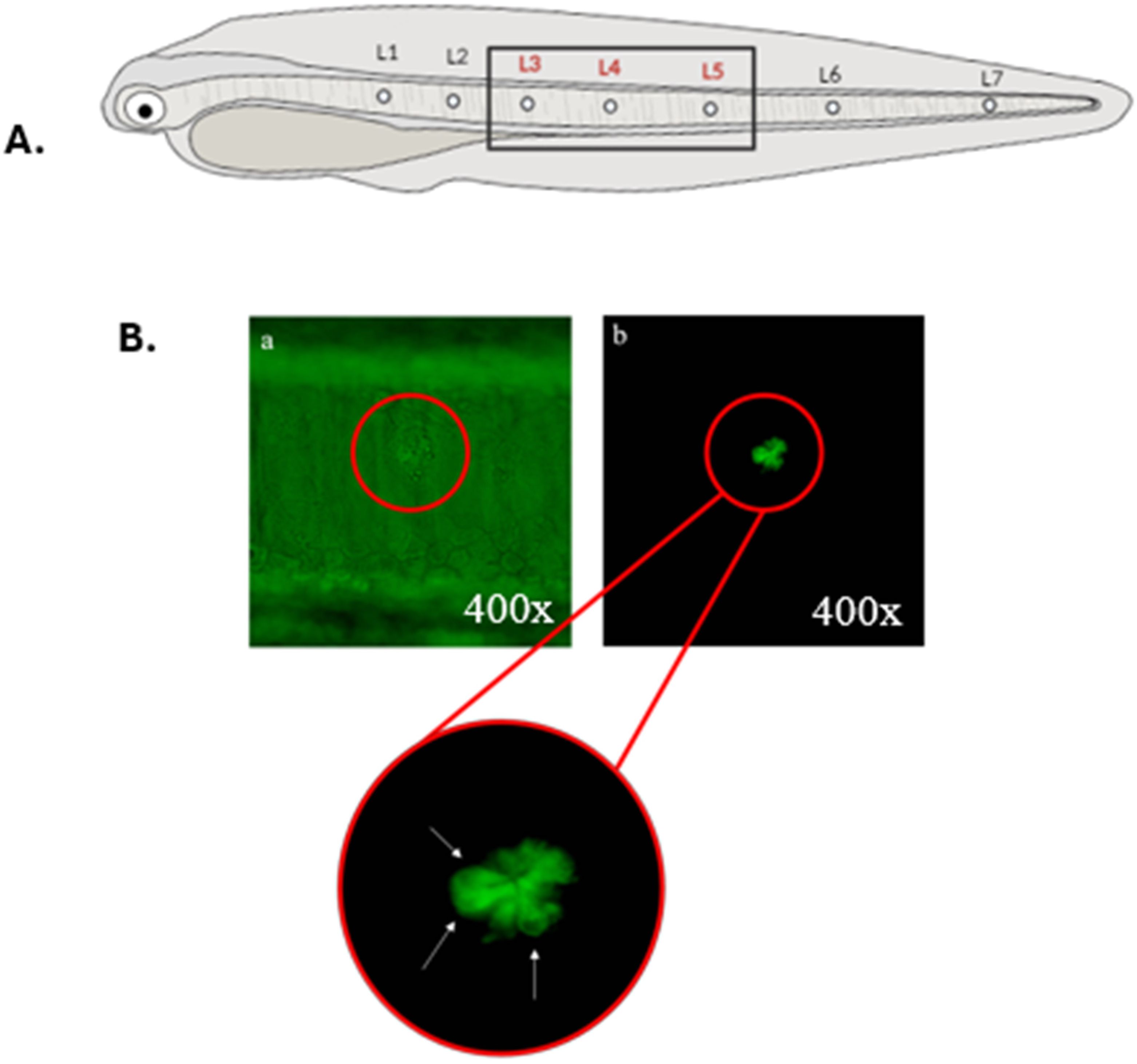
Figure 3. (A) Scheme showing the arrangement of neuromasts (L1 to L7) on the body of an anchovy larvae. Neuromasts where live cell counting was performed are highlighted in red. Neuromasts of the head are not represented. (B) In vivo visualization of the mid-body section of an anchovy larva exposed to seawater (negative control) and stained with DiAsp under a microscope (400x magnification). A single neuromast (L3) is distinguished, visible even without fluorescence (A), under fluorescence filter (B), and the detail of its cells. White arrows indicate cells considered viable for counting.
2.3.3 Evaluation of ranges of OA concentrations inducing alterations on neuromasts
To assess the effect of OA on neuromast cells, three OA concentrations were evaluated based on bibliographic data from other geographical areas. Chen et al. (2018) mention that the maximum detected concentration of dissolved OA in Chinese coastal waters around 0.1 ng mL−1. On the other hand, Bosch-Orea et al. (2017) indicate that at Spanish coasts, detections of OA exceeded 1 ng mL−1. During HAB events, there is a higher phytoplankton cell density and therefore a higher concentration of toxins such as OA in the water column is expected. HAB events in the future are expected to increase in frequency and intensity (cell density) (Wells et al., 2015). Accordingly, for our treatments, our maximum concentrations reached values above those described by Bosch-Orea et al. (2017) (see below).
To prepare the solutions, aliquots of OA (National Research Council Canada pure standard solution (CRM-OA-d)) were evaporated using a sample concentrator coupled to a nitrogen tank to remove the methanol in which the toxin is dissolved. Subsequently, the OA was resuspended in seawater (0.2 um filtered), stored in amber glass vials, and left homogenizing with gentle agitation overnight.
For the experiment, anchovy yolk sac larvae (n= 15) were exposed to increasing concentrations of OA of 0.1, 1, and 10 ng mL−1 and incubated for 20 minutes at 12°C to evaluate the acute damage induced by the toxin. Larvae exposed to positive control (CuSO4; n=15) and negative control (seawater; n=15) were also included. To avoid any operator bias, observations were made randomly during the counting through a blind analysis.
2.4 Second set of experiments: assessment of temperature and salinity variations and OA on anchoveta larvae neuromasts
To determine if variations in environmental conditions (salinity and temperature) alone (without OA) might alter the integrity of the cells composing the neuromasts, temperature and salinity values were defined as normal by inspecting the hydrographic profiles (CTD casts) obtained during the first three sampling dates (See Table 1). Previous records during the anchovy spawning season in the study area determined average temperature values close to 12°C and salinity values between 33 and 34 PSU for the first 30 - 40 meters of depth (Castro et al., 2019). Using data collected by the CTD, we generated profiles that allowed us to calculate the average values of each parameter. These values were used as a reference to define our experimental conditions, which should reflect the natural environmental conditions in which anchovy eggs and larvae are found. Profile analyses revealed that temperatures ranged around 12°C and salinity around 34 PSU in the study area, in the first 30 meters of depth.
To evaluate scenarios beyond the current normal condition, variations of these temperature and salinity values were considered, defining 14°C for the second temperature treatment and 32 PSU for the second salinity treatment. The temperature variation (increase) was set considering the potential effects by heatwave events, and the salinity variation (reduction) on the observed extreme rainfall events caused by atmospheric rivers during transitional periods (spring and autumn) (Oliver, 2019; Bustos-Espinoza et al., 2024). Finally, a combined treatment with both values, 14°C and 32 PSU, was also considered.
Consequently, in each of the three experiments (corresponding to the egg batches from October, November, and December), the collected and separated eggs from the plankton were separately incubated under each of these combinations of environmental conditions. When reaching 50% vitelline absorption, 15 yolk sac larvae were selected to undergo the DiAsp staining procedure described in section 2.3. On the other hand, to assess the influence of OA on the integrity of the hair cells of the neuromasts, incubations in 1 ng mL−1 of OA were conducted for 20 minutes on 15 larvae from each treatment mentioned prior to DiAsp staining. After staining, all larvae underwent the same microscopic counting procedure described in section 2.3, and the counts were performed randomly through a blind analysis.
2.5 Statistical analysis
The within-group normality of each dataset was assessed using the Shapiro-Wilk test, whereas the homogeneity of variance was checked using the Bartlett test. Depending on the results, parametric or non-parametric statistical tests were utilized to evaluate the effect of CuSO4 and different concentrations of OA on the neuromasts, the average number of viable cells in the neuromasts observed per individual (n=3) was calculated. The effect of CuSO4 was assessed using the Mann-Whitney test, while the effect of different OA concentrations was compared by ANOVA and Tukey’s post-hoc tests using GraphPad Prism software (version 9.2.0).
For the last three experiments (second set, AO, T and S), the data on viable cells were sorted according to the treatments, and the average number of viable cells for each individual was calculated. Normality was assessed using the Shapiro-Wilk test, which indicated that the data did not follow a normal distribution. Outliers were removed from each set using the z-score method for each treatment (z-score = (value - mean)/SD), considering values with z-scores > 1 as outliers. After outlier removal, comparisons were made using the Kruskal-Wallis test followed by Dunn’s post-hoc tests using the basic package in R software (https://cran.r-project.org/).
The design of experiments 1, 2, and 3 incorporated environmental variables and okadaic acid (OA) exposure. However, for clarity in presenting the results, they were segregated into two figures. One figure exclusively presents the results of experimental conditions involving T and S, while another figure represents the experimental outcomes with OA.
3 Results
3.1 Environmental conditions
Temperature values ranged from 11.3°C at surface to 12.6°C at 40 m deep in the September-October sampled dates. Temperature in the first two samplings showed a gradual decrease with depth (Figure 4) but in October, the thermocline was more pronounced and located within the first 10 meters of depth. Salinity varied between 32 to 34.4 in all profiles, the lowest values occurred in shallow water early in the season (September). Late in September and in October, though, most of the water column deeper than 5 or 10 m depth remained with salinities around 34 or higher as the depth increased. In October, salinity profiles did not exhibit a pronounced halocline, which, along with the lower temperature, suggested that an upwelling event was occurring during the date sampled, thus, the most frequent ranges of values in the shallow layers ranged between 12° and 34 PSU.
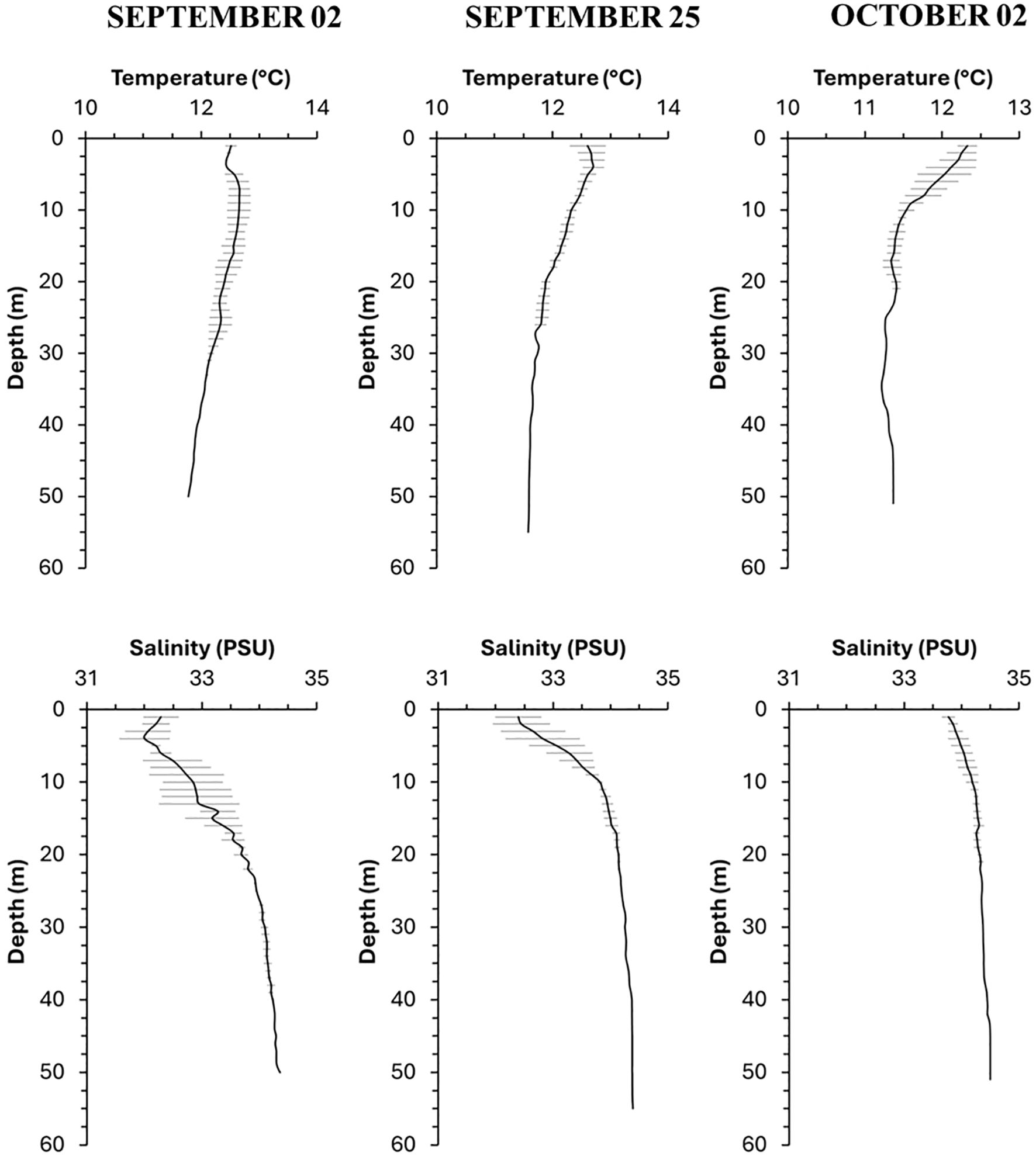
Figure 4. Temperature (°C) and salinity (PSU) profiles for the study area during samplings conducted in September and October. Average values are presented, with horizontal lines indicating standard deviation.
3.2 Standardization of neuromast cell counting: CuSO4 test as a possible positive control for neuromast cell damage
In larvae exposed to CuSO4 (50 uM), the number of viable cells in the analyzed neuromasts was lower (1.04 ± 0.99) than the number of viable cells in larvae exposed only to seawater (6.0 ± 2.2) (negative control). This nearly 85% decrease in the number of viable cells per neuromast was statistically significant (Mann-Whitney test; p < 0.0001, Figure 5), confirming that neuromasts of early anchovy larvae may be affected by toxic compounds, that the cell counting methodology with DiAsp staining is effective for evaluating this damage and is harmless to the larvae, since it did not generate mortality or any other type of damage. The treatment with copper was included in each of the subsequent experiments, and this result was observed in all of them.
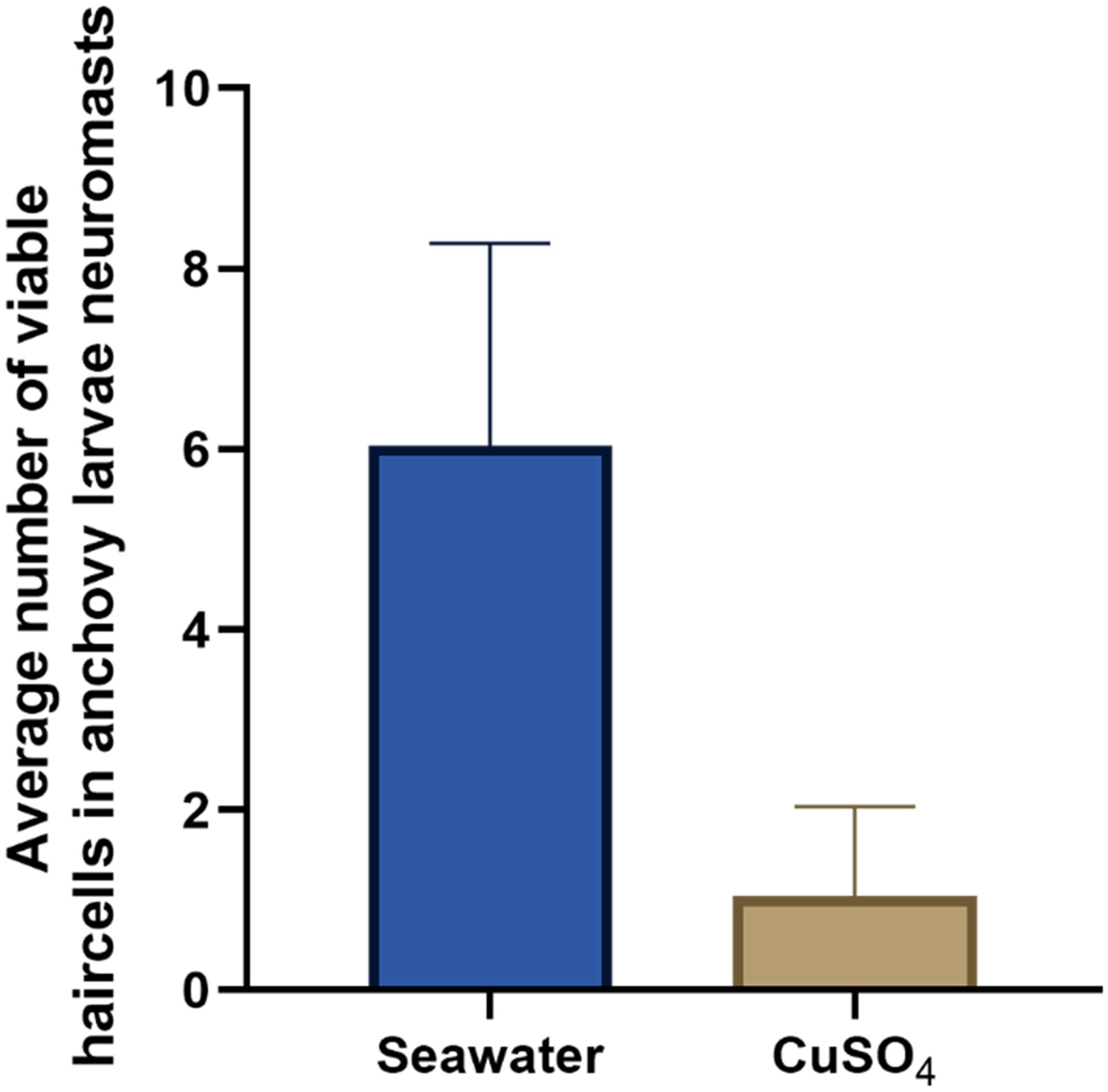
Figure 5. Average ( ± s.d.) number of viable cells in lateral neuromasts 3, 4, and 5 of anchovy larvae in positive control (CuSO4) and negative control (seawater) treatments; Mann-Whitney test, p<0,0001. The number of larvae analyzed per treatment was 43 and originated from 3 sampled stations or dates.
3.3 Evaluation of OA concentration ranges inducing alterations on neuromasts
The results of this experiment revealed an inverse relationship between the concentration of OA to which the larvae were exposed and the number of viable cells counted in the analyzed neuromasts (Figure 6, Table 2). As the concentration of OA increased, a decrease in the number of viable cells was observed. However, these differences were only significant when comparing larvae exposed to the negative control with those exposed to higher concentrations of OA (Tukey’s test; 1 ng mL−1, p= 0.0363; 10 ng mL−1, p<0.0001). When compared to the positive control (CuSO4), it was observed that the damage induced by the toxin in anchoveta larvae was lower than that induced by copper sulfate, with significant differences in the two lower concentrations (Tukey’s test; 0.1 ng mL−1, p<0.0001; 1 ng mL−1, p=0.0001). Based on these results, a concentration of 1 ng mL−1 of OA was selected for the subsequent experiments.
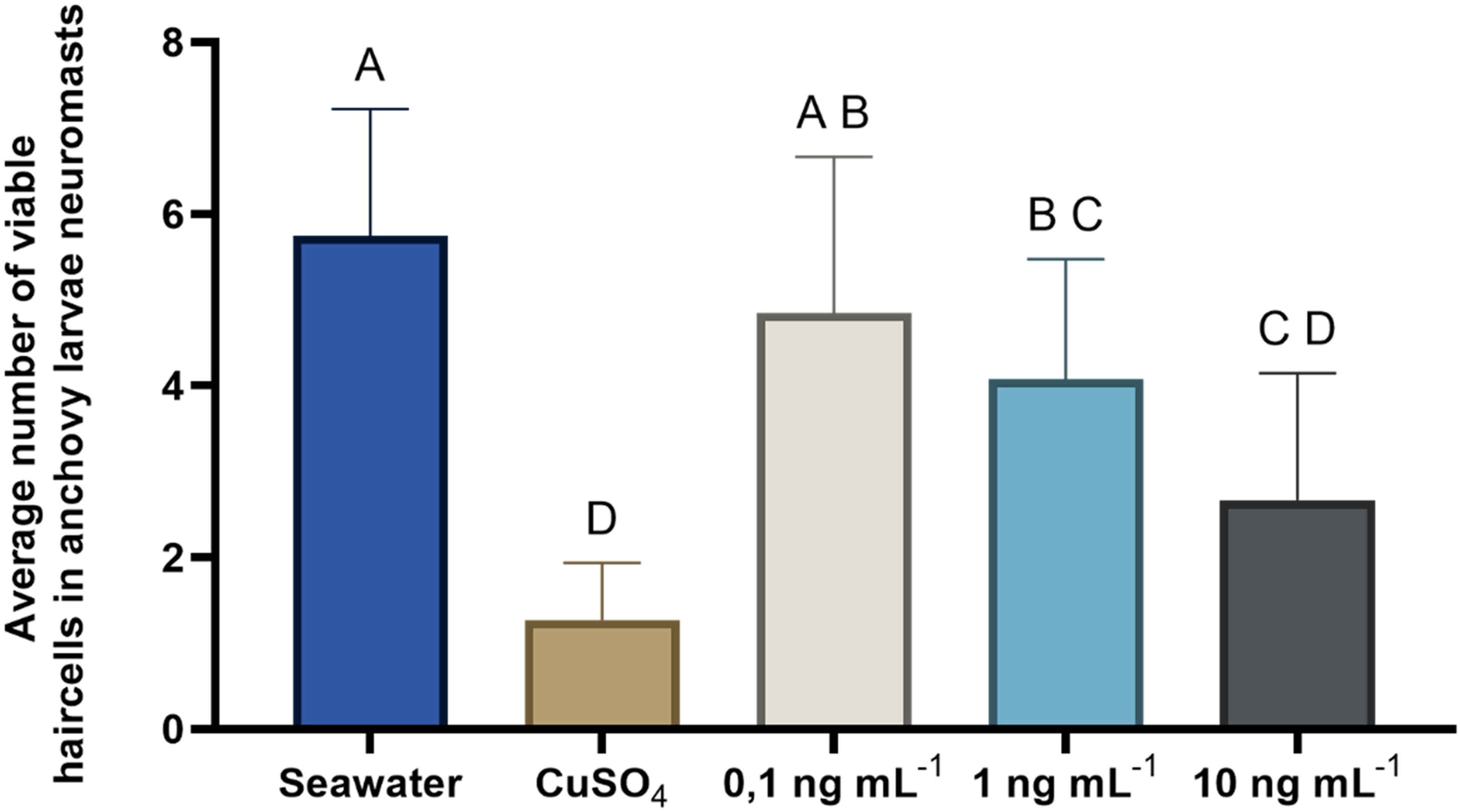
Figure 6. Evaluation of the effect of increasing concentrations of OA on neuromast cells. Average number of viable neuromast cells of anchoveta larvae exposed to OA at three concentrations. Statistical comparisons between treatments (Seawater, CuSO4, 0.1, 1, and 10 ng mL-1 OA) are represented with uppercase letters; treatments with the same letters indicate no significant differences between them. For example: 0.1 ng mL-1 does not differ significantly from the seawater treatment (A), nor from the 1 ng mL-1 treatment (B).
3.4 Evaluation of potential damage generated by temperature and salinity variations on anchoveta larvae neuromasts
To assess whether the projected environmental conditions for the study area alone might induce damage to neuromasts, anchoveta larvae were exposed to different combinations of temperature and salinity on three dates from October to December (Experiments 1-3). Three experiments were conducted: in the first experiment (Exp 1, corresponding to October; Table 1), a combined challenge treatment (14°C, 32 PSU) was not included (Figure 7). The results indicate that, under the environmental condition defined as normal (12°C, 34 PSU), the number of viable cells in the neuromasts varied, decreasing progressively as the spawning season advanced. The highest average values of viable cells were 7.4 (SD=3.77) in October (Exp. 1), and the lowest (4.4 cells, SD=2.27) were in December (Exp. 3) with which coincides with the final period of the anchoveta spawning season in the area.
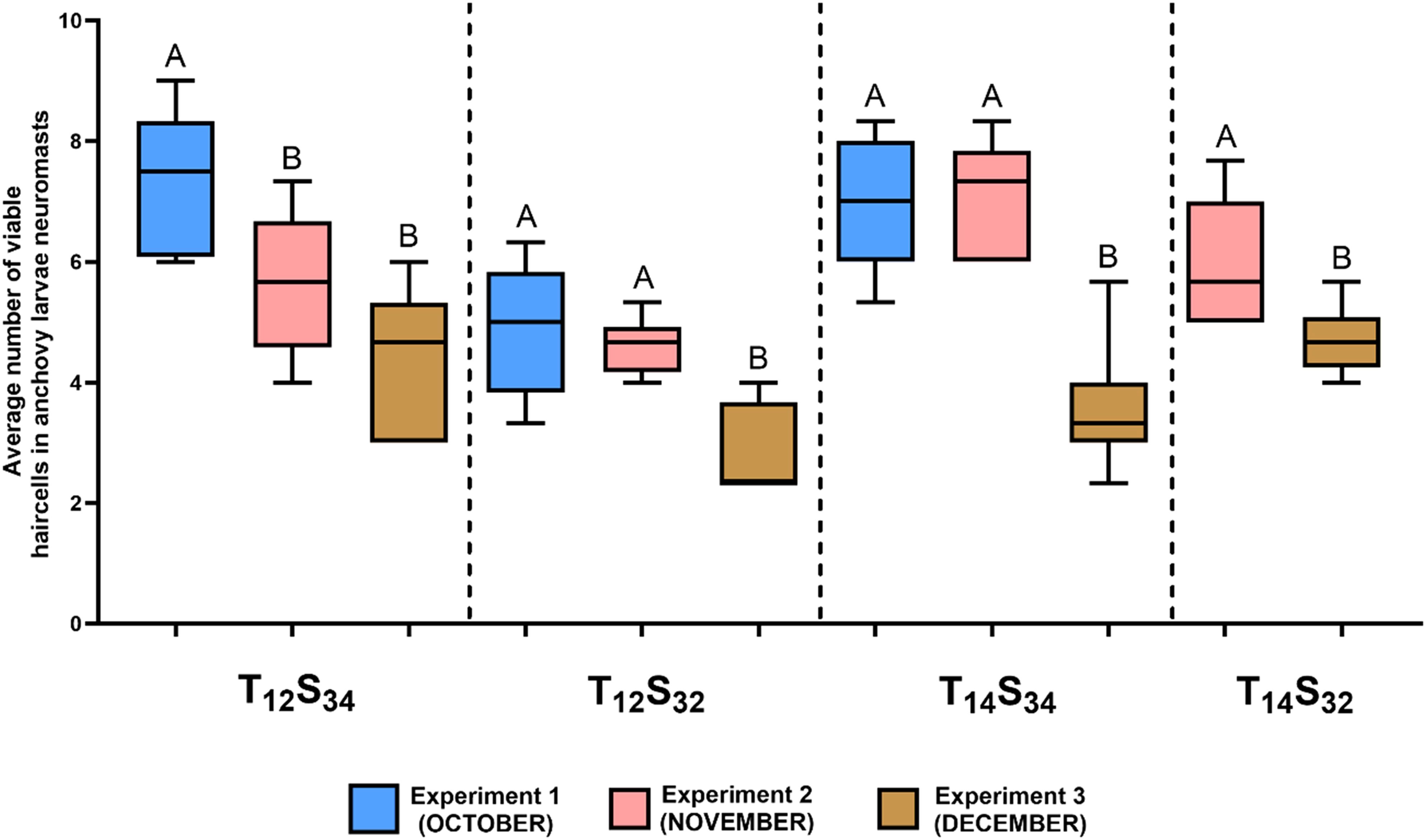
Figure 7. Effect of different combinations of temperature and salinity (treatments) on the number of viable neuromast cells of anchoveta larvae in the three experiments conducted. The ends of the boxes show the upper (Q3) and lower (Q1) quartiles, the horizontal line represents the median of the data, and the vertical lines represent the maximum and minimum values for each treatment. Statistical comparisons of the same treatment between experiments are represented with uppercase letters; treatments with the same letters indicate no significant differences between them. For example: in the T12 S32 treatment, there were no significant differences between experiments 1 and 2 (A), but both differed significantly from experiment 3 (B).
The comparison between treatments of environmental conditions indicated a decrease in the number of viable cells in larvae exposed to the salinity challenge treatment (T12S32) compared to those under normal environmental conditions (T12S34) with statistically significant differences in the first (October) and third (December) experiments (Table 3, Exp 1: Dunn’s Test, p= 0.0046, Exp 3: Dunn’s Test, p= 0.0078) (Figure 8). This could indicate a negative effect on neuromasts due to lower salinity in the environment; however, this difference was not observed in treatments where along with the decrease in salinity, there was a higher ambient temperature (Combined challenge, T14S32). Under this condition, the number of viable cells in the neuromasts was equal to what was quantified in larvae exposed to the normal condition (Exp 2: Dunn’s Test, p= 0.5739; Exp 3: Dunn’s Test, p= 0.6228). In larvae exposed to the temperature challenge treatment (T14S34), the results were similar to those quantified under normal conditions (Exp 2: Dunn’s Test, p= 0.0943, Exp 3: Dunn’s Test, p=0.3526).
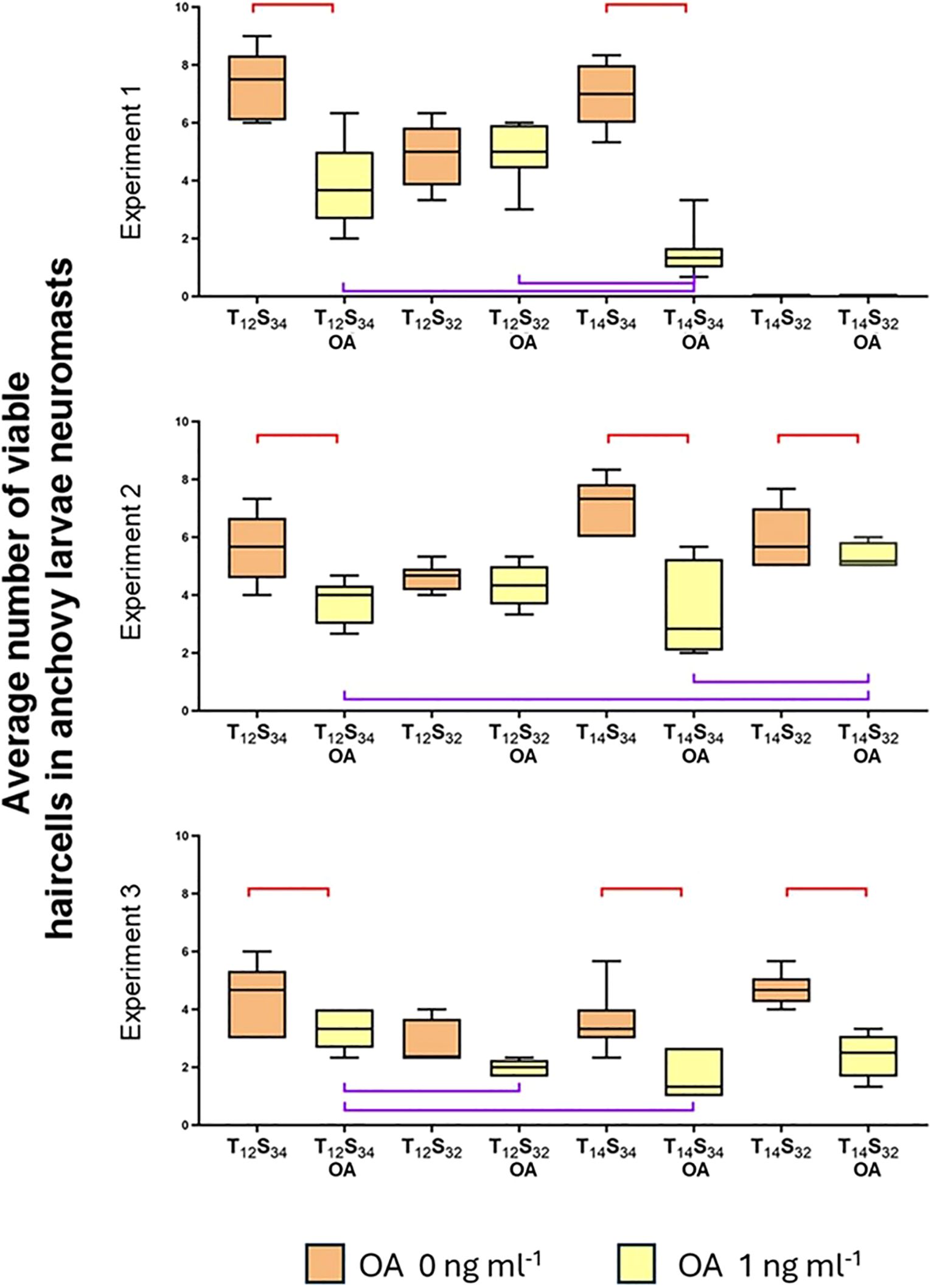
Figure 8. Effect of OA and different combinations of temperature and salinity on the number of viable neuromast cells. The ends of the boxes show the upper (Q3) and lower (Q1) quartiles, the horizontal line represents the median of the data, and the vertical lines represent the highest and lowest values for each treatment. The brackets indicate statistical comparisons where significant differences were found: the red brackets indicate significant differences within the same treatment with and without okadaic acid. The purple brackets indicate significant differences between different treatments with OA only.
Both treatments defined as salinity challenge (T12S32) and temperature challenge (T14S34) in experiments 1 and 2 showed significant differences between them (Table 3, Exp. 1: Dunn’s Test. p=0.0161, Exp 2: Dunn’s Test p=0.0019), indicating a lower average number of viable hair cells in the salinity challenge treatment (Figure 8).
3.5 Evaluation of the effect of OA with variations in ambient temperature and salinity on neuromasts of anchoveta larvae
In all three experiments where larvae were exposed to OA along with different combinations of temperature and salinity, a consistent trend is observed: OA induces a decrease in the number of viable cells in all cases, except when the salinity challenge was applied (T12S32) (Figure 8).
The results indicate that temperature seems to play a significant role in reducing the number of hair cells in neuromasts in the presence of OA in at least two out of the three experiments conducted, showing significant differences in both cases (Table 3, Exp 1: Dunn’s test, p=0.0560; Exp 3: Dunn’s test, p=0.0170). It is important to note that OA treatment exhibited the lowest average values of hair cells in neuromasts (Exp 1: 1.48; Exp 2: 3.5; Exp 3: 1.64 cells).
However, in the second experiment, no significant differences were found between the treatment of normal conditions (T12S34) and the temperature treatment (T14S34). There were also no significant differences between the normal treatment (T12S34) and the salinity treatment (Exp 2: T14S34, Dunn’s test, p=0.7093; T12S32, Dunn’s test, p=0.4586).
4 Discussion
Given the heterogeneous nature and the different mechanisms of action of the factors that can be stressful or be directly harmful to aquatic organisms, the coexistence of several factors can result in additive, synergistic, or antagonistic effects on the organisms facing them (Velasco et al., 2018). With the purpose of investigating possible structural variations in the lateral line neuromasts of early anchoveta larvae in response to exposure to okadaic acid (OA), as well as to different temperature and salinity values, experiments were conducted with different combinations of these three variables. The results show an influence of seasonal variability on the quality of the larvae and their neuromasts, as well as a significant influence of the salinity variation on the number of living cells in these sensorial organs. Additionally, when evaluating the combined effects of the toxin presence and environmental variations, we observed that an increase in temperature intensifies the harmful effects of OA on hair cells, suggesting greater damage.
Studies on effects generated by variations in environmental conditions, such as temperature and salinity, on larvae of aquatic organisms usually reveal alterations in survival, growth, and vital biological processes such as swimming speed (Collazos-Lasso et al., 2014; Montory et al., 2014; Politis et al., 2018). For young life stages of anchoveta (Engraulis ringens), it has been reported that temperature modulates the embryo duration, the yolk sac larval phase duration, yolk consumption rate, and larval growth rate (Llanos-Rivera and Castro, 2006). Salinity variations have been related to changes in the hatching gene expression, the hatching enzyme expression and larval hatching success (Castro et al., 2021). However, while these studies provide evidence of the effects of environmental variations on relevant embryonic and larval processes, to date, there has been no reported evidence of the effect that temperature and salinity variations could have on the mechanoreceptor system of the lateral line in the larval stage of this economically important fish species.
Our results suggest a change in larval neuromasts integrity along the reproductive season. For this species, a primary spawning period is recognized between winter and spring, with a reproductive peak in August (Cubillos et al., 2001), and our experiments were conducted with eggs collected in months following this peak (October, November, and December). The results showed that larvae from eggs collected in the middle of the spawning season had a higher average number of viable hair cells compared to those obtained from eggs spawned towards the end of the reproductive period. Additionally, for the later period, we observed greater larval fragility, making their handling considerably more complex. Seasonal variation in the quality of eggs and larvae has been reported for small pelagic fishes using various indicators. For example, for this species, both the biochemical composition (proteins, lipids, or free amino acids), the size of the egg and larval hatch success indicate a decrease in the quality of propagules as the spawning season progresses (Llanos-Rivera and Castro, 2004; Castro et al., 2009, 2021). For Strangomera bentincki, another Clupeiform species present in the same area, there is a trend towards an increase in embryonic anomalies as the reproductive season progresses (Vásquez et al., 2010).
Salinity, as one of the most important physicochemical parameters in marine ecosystems, varies in different habitats and seasons, affecting young stages survival, metabolism, distribution, and oxygen consumption rates of various taxa (Varsamos et al., 2005; Montory et al., 2014; Castro et al., 2021). In this study, we provide evidence on that a decrease in salinity in experimental conditions affect the structure and integrity of the hair cells present in the lateral neuromasts of anchoveta larvae. Neuromasts maintain hair cells immersed in fluid microenvironments maintained electrogenically; covered by a gelatinous cupula that provides an electric and ionic microenvironment that remains active and is necessary for hair cells to depolarize when mechanically activated, initiating sensory transduction (Peloggia et al., 2021). In zebrafish larvae (Danio rerio), another teleost, changes in salinity have been identified to induce a signaling cascade in isotonic environments, causing epithelial cells to differentiate into ionocytes; which maintain ionic balance ensuring conditions for hair cell functionality (Stawicki et al., 2014; Peloggia et al., 2022, 2024). Thus, genetic ablation of these ionocytes as well as exposure to acidified water impair hair cell function, suggesting that hair cells require a buffered ionic environment to function properly (Stawicki et al., 2014; Lin et al., 2019).
In recent decades, potential toxic effects of OA have been investigated beyond its role as a Diarrhetic Shellfish Poisoning (DSP) toxin. Alterations in DNA and cellular components have been reported, as well as effects on the immune and nervous systems, and even on embryonic development (Valdiglesias et al., 2013). In fish, acute exposure to this toxin leads to alterations in oxidative parameters inducing malformations in medaka (Oryzias latipes) larvae (Figueroa et al., 2023), embryonic development alterations in marine species such as Oryzias melastigma (Yang et al., 2023), and changes in swimming, buoyancy, and ataxia in adult zebrafish (Danio rerio) (Barrio et al., 2015). Our results indicate that this toxin has a dose-dependent negative effect on the integrity of hair cells in neuromasts. These hair cells are formed by specialized extensions called stereocilia which are composed of actin filaments, and a group of stereocilia will be associated with a single kinocilium, a central microtubule (Tanimoto et al., 2011), both structures being fundamental for the sensory function of the neuromast. Okadaic acid inhibits the activity of phosphatase 2A (PP2A), which among other functions may affect the structural states that define the cellular function of actin, such as polymerization and depolymerization (Canals et al., 2012). On the other hand, there is evidence that OA affects microtubule support proteins in primary neuron cultures, resulting in decreased neuronal function (Zhao et al., 2016). These findings suggest that the potential mechanism of toxicity in neuromasts could be related to the structural stability of the cells composing neuromasts mediated by the inhibition of PP2A activity on proteins. Further subcellular level research is needed to improve our understanding on the underlying biochemical mechanisms involved in regulating the quantity and integrity of hair cells in response to environmental variations and the presence of toxic agents such as OA.
Lin et al. (2019) and Stawicki et al. (2014) have shown how damage to hair cell structures, induced by factors such as acidic water, can impair the mechanosensory capabilities of neuromasts. Consequently, structural damage to these organs can lead to their functional impairment. To evaluate the impact of OA on the functionality of the mechanoreceptor system, Faria et al. (2020) assessed how this toxin affects the escape behavior of 7 days post fertilization (dpf) zebrafish larvae, which is partly regulated by the normal functioning of neuromasts. The results showed that startle and habituation responses (the ability to ignore irrelevant stimuli) were not altered after exposure to 0.036 ng mL−1 of this toxin; a concentration below which our results showed that it would not induce structural damage of neuromasts (1 ng mL−1) and thus it is expected not to detect functional alterations. Thus, our findings indicate that the higher concentrations of okadaic acid, starting from 1 ng mL−1, which are ecologically relevant, can cause hair cell damage. This damage suggests a possible functional impairment of these organs in fish larvae that are exposed to these toxins dissolved in their surrounding water.
When comparing the combined effect of the toxin and environmental parameters, we observed the synergistic and/or additive role of temperature with the toxin. A stressed thermoregulatory system in any organism can alter the physiological response to toxic agents, and generally, high temperatures exacerbate the effects of many environmental toxins (Gordon, 2003). In other fish, an increase in water temperature has been associated with an increase in the acute toxicity of substances such as paracetamol, enrofloxacin, and chlortetracycline (Kim et al., 2010). This phenomenon is likely attributed to changes in the toxicokinetics of these substances, i.e., how they are absorbed, distributed, metabolized, and excreted in the fish’s body (Kim et al., 2010). In our experience, in two out of the three experiments conducted, the combination of temperature and OA resulted in a decrease in the number of viable hair cells compared to normal conditions with the presence of the toxin. Additionally, in Experiment 1, these variations were significant even when compared to the effects of salinity, suggesting that the interaction between temperature and OA may potentiate adverse effects on neuromast integrity. This contrasts with observations by Xu et al. (2004), who report that a thermal variation can mitigate the effects of OA through the hyperphosphorylation of cytoskeletal proteins, implying that the effect of OA would be less when applied together with a thermal change. However, it is important to highlight that this study was conducted on mammalian cell cultures (neuroblastomas), which might explain differences in the observed responses compared to whole organisms, as in our case. Moreover, the experimental conditions used by Xu et al. including toxin concentration, exposure time, and temperature, were much higher than those in our study (25 ng mL−1, 10 hours, and 42°C). Therefore, differences in the results could be due to these discrepancies in experimental conditions and may not necessarily reflect what occurs in the natural environment of anchovy larvae that we attempted to replicate in the laboratory.
Among the characteristics of OA, its thermal stability stands out, along with a relatively slow degradation under natural solar radiation, allowing it to remain in the water column for more than 20 days (Jimenez, 2019, Pan et al., 2020). Most harmful algal bloom (HAB) events reported in central-southern Chile have occurred during spring-summer periods, where there is usually an increase in seawater temperature (Barría et al., 2022). This makes it possible for an HAB event with OA production during spring-summer to coincide with the final main spawning period of anchoveta.
The results obtained in this study show that this toxin in the water column could induce damage to neuromasts and that this damage could be greater as the reproductive period progresses. The deterioration of these sensory organs could compromise the larvae’s ability to respond to key environmental stimuli, which in turn could affect their survival. This becomes particularly relevant when considering that the early life stages of these organisms naturally experience high mortality rates. The success of recruitment is closely tied to the survival of larvae during these critical periods, where any additional stressors, such as the damage induced by marine toxins, could further diminish their chances of reaching maturity. On a broader perspective, these results underscore the importance of considering multiple environmental factors (e.g., variations in temperature and salinity) when studying the effects of marine toxins on fish larvae, providing new insights into how toxins produced in HAB events can interact with abiotic elements and have repercussions on organisms coexisting in the water column.
5 Conclusions
Our study highlights the potential effect of OA on the sensory organs of fish larvae, particularly on neuromasts. We found that exposure to OA can lead to negative effects in these critical sensory structures, potentially compromising the larvae’s ability to respond to environmental cues and affect their survival. Salinity alone may also induce changes in the viability of haircells. Additionally, our results suggest a synergistic effect between OA and temperature, which could exacerbate the detrimental effects on neuromast integrity. In a broader context, these results underscore the importance of considering multiple environmental variables when assessing the effects of marine toxins on fish larvae, especially considering environmental variations anticipated under a climate change scenario.
Data availability statement
The raw data supporting the conclusions of this article will be made available by the authors, without undue reservation.
Ethics statement
The animal study was approved by Comité de Ética, Bioética y Bioseguridad. Universidad de Concepción. The study was conducted in accordance with the local legislation and institutional requirements.
Author contributions
PP: Data curation, Investigation, Methodology, Writing – original draft. LC: Conceptualization, Funding acquisition, Investigation, Methodology, Project administration, Resources, Writing – review & editing. CA: Data curation, Formal analysis, Methodology, Writing – review & editing. AL: Conceptualization, Formal analysis, Methodology, Writing – original draft.
Funding
The author(s) declare financial support was received for the research, authorship, and/or publication of this article. Financial support for the study and to PP-V, LC, and CA-D-S was provided by COPAS COASTAL Center FB10021, and for AL-R by FONDEF IDEA ID20I10078.
Acknowledgments
We appreciate the field and laboratory work conducted by personnel at LOPEL (P Barrientos, C Veroe, C Orellana, R. León, V. Palma) and LATAC (P Yañez, N Zambrano) as well as the technical support aboard the RV KayKay from the Universidad de Concepción. S. Soto prepared Figure 1.
Conflict of interest
The authors declare that the research was conducted in the absence of any commercial or financial relationships that could be construed as a potential conflict of interest.
Publisher’s note
All claims expressed in this article are solely those of the authors and do not necessarily represent those of their affiliated organizations, or those of the publisher, the editors and the reviewers. Any product that may be evaluated in this article, or claim that may be made by its manufacturer, is not guaranteed or endorsed by the publisher.
References
Anderson C., Moore S., Tomlinson M., Silke J., Cusack C. (2015). “Chapter 17: living with harmful algal blooms in a changing world: strategies for modeling and mitigating their effects in coastal marine ecosystems,” in Coastal and marine hazards, risks, and disasters (Netherlands: Elsevier). doi: 10.1016/B978-0-12-396483-0.00017-0
Anderson D., Fensin E., Gobler C., Hoeglund A., Hubbard K., Kulis D., et al. (2021). Marine harmful algal blooms (HABs) in the United States: History, current status and future trends. Harmful Algae 102, 101975. doi: 10.1016/j.hal.2021.101975
Barría C., Vásquez-Calderón P., Lizama C., Herrera P., Canto A., Conejeros P., et al. (2022). Spatial temporal expansion of harmful algal blooms in Chile: A review of 65 years records. J. Mar. Sci. Eng. 10, 1868. doi: 10.3390/jmse10121868
Barrio D., Avila M., Alejo A., Solimano P., Piñuel M., Boeri P., et al. (2015). El pez cebra (Danio rerio) como un sistema modelo para la valoración biológica de las toxinas producidas por la marea roja. Servicio Nacional Sanidad y Calidad Agroalimentaria 8, 1–9. Available at: http://hdl.handle.net/11336/44947.
Basti L., Hégaret H., Shumway S. (2018). “Chapter 4: harmful algal blooms and shellfish,” in En Harmful Algal Blooms: A Compendium Desk Reference (USA: John Wiley and Sons). doi: 10.1002/9781118994672.ch4
Bosch-Orea C., Sanchís J., Farré M., Barceló D. (2017). Analysis of lipophilic marine biotoxins by liquid chromatography coupled with high-resolution mass spectrometry in seawater from the Catalan Coast. Anal. Bioanal Chem. 409, 5451–5462. doi: 10.1007/s00216-017-0536-y
Bui Q., Kim H., Park H., Ki J. S. (2021). Salinity Affects Saxitoxins (STXs) Toxicity in the Dinoflagellate Alexandrium pacificum, with Low Transcription of SXT-Biosynthesis Genes sxtA4 and sxtG. Toxins 13, 733. doi: 10.3390/toxins13100733
Bustos-Espinoza L., Torres-Ramírez P., Figueroa S., González P., Pavez M., Jerez R., et al. (2024). Biogeochemical response of the water column of concepción bay, Chile, to a new regime of atmospheric and oceanographic variability. Geosciences 14, 125. doi: 10.3390/geosciences14050125
Canals D., Roddy P., Hannun Y. (2012). Protein phosphatase 1α mediates ceramide-induced ERM protein dephosphorylation: a novel mechanism independent of phosphatidylinositol 4, 5-biphosphate (PIP2) and myosin/ERM phosphatase. J. Biol. Chem. 287, 10145–10155. doi: 10.1074/jbc.M111.306456
Castro L., Claramunt G., Krautz M., Llanos-Rivera A., Moreno P. (2009). Egg trait variation in anchoveta Engraulis ringens: a maternal response to changing environmental conditions in contrasting spawning habitats. Mar. Ecol. Prog. Ser. 381, 237–248. doi: 10.3354/meps07922
Castro L., Claramunt G., Espinoza R., Azocar C., Soto-Mendoza S. (2019). Vertical distribution, specific gravity, and free amino acids in anchoveta Engraulis ringens eggs under contrasting spawning habitat conditions. Marine Ecology Progress Series 617, 7-24. doi: Marine Ecology Progress Series
Castro L., González V., Claramunt G., Barrientos P., Soto S. (2020). Stable isotopes (δ13C, δ15N) seasonal changes in particulate organic matter and in different life stages of anchoveta (Engraulis ringens) in response to local and large scale oceanographic variations in north and central Chile. Prog. Oceanography 186, 102342. doi: 10.1016/j.pocean.2020.102342
Castro L., Morin V., Tiznado O., Miranda A., Soto S., Gonzalez M. (2021). Effects of salinity changes on hatching, hatching-gene expression, and hatching-enzyme expression in anchoveta Engraulis ringens eggs. Mar. Ecol. Prog. Ser. 658, 181–194. doi: 10.3354/meps13548
Chen J., Han T., Li X., He X., Wang Y., Chen F., et al. (2018). Occurrence and distribution of marine natural organic pollutants: Lipophilic marine algal toxins in the Yellow Sea and the Bohai Sea, China. Sci. Total Environ. 612, 931–939. doi: 10.1016/j.scitotenv.2017.08.304
Christensen V., Khan E. (2020). Freshwater neurotoxins and concerns for human, animal, and ecosystem health: A review of anatoxin-a and saxitoxin. Sci. Total Environ. 736, 139515. doi: 10.1016/j.scitotenv.2020.139515
Collazos-Lasso L., Gutierrez-Espinosa M., Restrepo-Betancour L. (2014). Supervivencia de larvas de cachama blanca, Piaractus brachypomus Cuvier 1818, sometidas a cambios experimentales de temperatura. Orinoquia 18, 193–197. doi: 10.22579/20112629.375
Corriere M., Baptista M., Paula J., Repolho T., Rosa R., Reis-Costa P., et al. (2020). Impaired fish swimming performance following dietary exposure to the marine phycotoxin okadaic acid. Toxicon 179, 53–59. doi: 10.1016/j.toxicon.2020.02.022
Cubillos L., Arcos D., Bucarey D., Canales M. (2001). Seasonal growth of small pelagic fish off Talcahuano, Chile (37°S, 73°W): a consequence of their reproductive strategy to seasonal upwelling? Aquat. Living Resour. 14, 115–124. doi: 10.1016/S0990-7440(01)01112-3
Díaz P., Álvarez G., Pizarro G., Blanco J., Reguera B. (2022). Lipophilic toxins in Chile: history, producers and impacts. Mar. Drugs 20, 122. doi: 10.3390/md20020122
Faria M., Wu X., Luja-Mondragón M., Prats E., Gómez-Oliván L., Piña B., et al. (2020). Screening anti-predator behaviour in fish larvae exposed to environmental pollutants. Sci. Total Environ. 714, 136759. doi: 10.1016/j.scitotenv.2020.136759
Figueroa D., Ríos J., Araneda O., Contreras H., Concha M., García C. (2023). Oxidative Stress Parameters and Morphological Changes in Japanese Medaka (Oryzias latipes) after Acute Exposure to OA-Group Toxins. Life 13, 15. doi: 10.3390/life13010015
Froehlicher M., Liedtke A., Groh K. J., Neuhauss S. C., Segner H., Eggen R. I. (2009). Zebrafish (Danio rerio) neuromast: Promising biological endpoint linking developmental and toxicological studies. Aquat. Toxicol. 95, 307–319. doi: 10.1016/j.aquatox.2009.04.007
Ghysen A., Dambly-Chaudière C. (2004). Development of the zebrafish lateral line. Curr. Opin. Neurobiol. 14, 67–73. doi: 10.1016/j.conb.2004.01.012
Glibert P., Allen J., Artioli Y., Beusen A., Bouwman L., Harle J., et al. (2014). Vulnerability of coastal ecosystems to changes in harmful algal bloom distribution to climate change: projections based on model analysis. Global Change Biol. 20, 3845–3858. doi: 10.1111/gcb.12662
González-Reyes Á, Jacques-Coper M., Bravo C., Rojas M., Garreaud R. (2023). Evolution of heatwaves in Chile since 1980. Weather Climate Extremes 41, 100588. doi: 10.1016/j.wace.2023.100588
Gordon C. (2003). Role of environmental stress in the physiological response to chemical toxicants. Environ. Res. 92, 1–7. doi: 10.1016/S0013-9351(02)00008-7
Hattenrath-Lehmann T., Lusty M., Wallace R., Haynes B., Wang Z., Broadwater M., et al. (2018). Evaluation of rapid, early warning approaches to track shellfish toxins associated with dinophysis and alexandrium blooms. Mar. Drugs 16, 28. doi: 10.3390/md16010028
He X., Chen J., Wu D., Wang D., Xin X., Liu L., et al. (2020). Occurrence, distribution, source, and influencing factors of lipophilic marine algal toxins in Laizhou Bay, Bohai Sea, China. Mar. pollut. Bull. 150, 110789. doi: 10.1016/j.marpolbul.2019.110789
Hernández P., Moreno V., Olivari F., Allende M. (2006). Sub-lethal concentrations of waterborne copper are toxic to lateral line neuromasts in zebrafish (Danio rerio). Hear Res. 2006 213, 1–10. doi: 10.1016/j.heares.2005.10.015
Hinder S., Hays G., Edwards M., Roberts E., Walnes A., Gravenor B. (2012). Changes in marine dinoflagellate and diatom abundance under climate change. Nat. Climate Change 2, 271–275. doi: 10.1038/nclimate1388
Hung G. Y., Pan Y. C., Horng J. L., Lin L. Y. (2023). Sublethal effects of methylmercury on lateral line sensory and ion-regulatory functions in zebrafish embryos. Comp. Biochem. Physiol. Part C: Toxicol. Pharmacol. 271, 109700. doi: 10.1016/j.cbpc.2023.109700
Jacob B., Tapia F., Quiñones R., Montes R., Sobarzo M., Schneider W. (2018). Major changes in diatom abundance, productivity, and net community metabolism in a windier and dryer coastal climate in the southern Humboldt Current. Prog. oceanography 168, 196–209. doi: 10.1016/j.pocean.2018.10.001
Jimenez D. (2019). Efecto protumoral del écido okadaico y sus análogos DTX-1 Y DTX-2 en líneas celulares de epitelio de colon. Universidad de Chile. Chile. Available at: https://repositorio.uchile.cl/handle/2250/196857. Master's thesis.
Kamiyama T., Nagai S., Suzuki T., Miyamura K. (2010). Effect of temperature on production of okadaic acid, dinophysistoxin-1, and pectenotoxin-2 by Dinophysis acuminata in culture experiments. Aquat Microb. Ecol. 60, 193–202. doi: 10.3354/ame01419
Kasumyan A. O. (2003). The lateral line in fish: structure, function, and role in behavior. Journal of Ichthyology 43 (2), S175.
Kibler S., Tester P., Kunkel K., Moore S., Litaker R. (2015). Effects of ocean warming on growth and distribution of dinoflagellates associated with ciguatera fish poisoning in the Caribbean. Ecol. Model. 316, 194–210. doi: 10.1016/j.ecolmodel.2015.08.020
Kim H. (2006). Mitigation and controls of HABs. En Ecol. Harmful Algae 189, 327–338. doi: 10.1007/978-3-540-32210-8_25
Kim J., Park J., Kim P., Lee C., Choi K., Choi K. (2010). Implication of global environmental changes on chemical toxicity-effect of water temperature, pH, and ultraviolet B irradiation on acute toxicity of several pharmaceuticals in Daphnia magna. Ecotoxicology 19, 662–669. doi: 10.1007/s10646-009-0440-0
Lin L., Hung G., Yeh Y., Chen S., Horng J. (2019). Acidified water impairs the lateral line system of zebrafish embryos. Aquat. Toxicol. 217, 105351. doi: 10.1016/j.aquatox.2019.105351
Lin L., Kantha P., Horng J. (2023). Toxic effects of polystyrene nanoparticles on the development, escape locomotion, and lateral-line sensory function of zebrafish embryos. Comp. Biochem. Physiol. Toxicol. Pharmacol. 272, 109701. doi: 10.1016/j.cbpc.2023.109701
Liu Y., Yu R., Kong F., Li C., Dai L., Chen Z., et al. (2017). Lipophilic marine toxins discovered in the Bohai Sea using high performance liquid chromatography coupled with tandem mass spectrometry. Chemosphere 183, 380–388. doi: 10.1016/j.chemosphere.2017.05.073
Llanos-Rivera A., Castro L. (2004). Latitudinal and Seasonal Egg-Size Variation of the Anchoveta (Engraulis ringens) off the Chilean Coast. Fishery Bull. 102, 207–212.
Llanos-Rivera A., Castro L. (2006). Inter-population differences in temperature effects on Engraulis ringens yolk-sac larvae. Mar. Ecol. Prog. Ser. 312, 245–253. doi: 10.3354/meps07922
Llanos-Rivera A., Herrera G., Tarifeño E., Castro L. (2014). Development of free neuromasts in Engraulis ringens and Strangomera bentincki (Teleostei, Clupeiformes) early larvae. Lat. Am. J. Aquat. Res. 42, 264–270. Available at: http://dx.doi.org/103856/vol42-issue1-fulltext-22.
MacKenzie L., Beuzenberg V., Holland P., McNabb P., Selwood A. (2004). Solid phase adsorption toxin tracking (SPATT): a new monitoring tool that simulates the biotoxin contamination of filter feeding bivalves. Toxicon 44, 901–918. doi: 10.1016/j.toxicon.2004.08.020
Mardones C. (2010). Seguimiento in vivo del proceso regenerativo de la línea lateral caudal en pez cebra adulto (Danio rerio). Universidad de Chile. Available at: https://repositorio.uChile.cl/handle/2250/192537. Bachelor’s thesis.
Miles C., Wilkins A., Munday R., Dines M., Hawkes A., Briggs L., et al. (2004). Isolation of pectenotoxin-2 from Dinophysis acuta and its conversion to pectenotoxin-2 seco acid, and preliminary assessment of their acute toxicities. Toxicon 43, 1–9. doi: 10.1016/j.toxicon.2003.10.003
Möller K., Pinto-Torres M., Mardones J., Krock B. (2022). Distribution of phycotoxins in Última Esperanza Province during the PROFAN expedition 2019. Prog. Oceanography 206, 102851. doi: 10.1016/j.pocean.2022.102851
Montory J., Pechenik J., Diederich C., Chaparro O. (2014). Effects of low salinity on adult behavior and larval performance in the intertidal gastropod crepipatella Peruviana (Calyptraeidae). PloS One 9, e103820. doi: 10.1371/journal.pone.0103820
Nishikawa S., Sasaki F. (1996). Internalization of styryl dye FM1-43 in the hair cells of lateral line organs in Xenopus larvae. J. Histochem Cytochem. 44, 733–741. doi: 10.1177/44.7.8675994
Oliver E. (2019). Mean warming not variability drives marine heatwave trends. Clim Dyn 53, 1653–1659. doi: 10.1007/s00382-019-04707-2
Pan L., Chen J., He X., Zhan T., Shen H. (2020). Aqueous photodegradation of okadaic acid and dinophysistoxin-1: Persistence, kinetics, photoproducts, pathways, and toxicity evaluation. Sci. Total Environ. 743, 140593. doi: 10.1016/j.scitotenv.2020.140593
Paredes-Mella J., Mardones J., Norambuena L., Fuenzalida G., Labra G., Nagai S. (2022). Toxic Dinophysis acuminata in southern Chile: A comparative approach based on its first local in vitro culture and offshore-estuarine bloom dynamics. Prog. Oceanography 209, 102918. doi: 10.1016/j.pocean.2022.102918
Paz B., Riobó P., Fernández M., Fraga S., Franco J. (2004). Production and release of yessotoxins by the dinoflagellates Protoceratium reticulatum and Lingulodinium polyedrum in culture. Toxicon 44, 251–258. doi: 10.1016/j.toxicon.2004.05.021
Peloggia J., Lush M., Tsai Y. Y., Wood C., Piotrowski T. (2024). Environmental and molecular control of tissue-specific ionocyte differentiation in zebrafish. bioRxiv. doi: 10.1101/2024.01.12.575421. Preprint.
Peloggia J., Münch D., Meneses-Giles P., Romero-Carvajal A., Lush M., Lawson N., et al. (2022). Adaptive cell invasion maintains lateral line organ homeostasis in response to environmental changes. Dev. Cell 56, 1296–1312. doi: 10.1016/j.devcel.2021.03.027
Peloggia J., Münch D., Meneses-Giles P., Romero-Carvajal A., McClain M., Pan Y. A., et al. (2021). Adaptive cell invasion maintains organ homeostasis. bioRxiv. doi: 10.1101/2020.12.01.404954. Preprint.
Pizarro G., Alarcón C., Franco J., Palma M., Escalera L., Reguera B., et al. (2011). Distribución espacial de Dinophysis spp. y detección de toxinas DSP en el agua mediante resinas DIAION (verano 2006, Región de Los Lagos, Chile). Cienc. Tecnol. Mar. 34, 31–48.
Politis S., Mazurais D., Servili A., Zambonino-Infante J. L., Miest J., Tomkiewicz J., et al. (2018). Salinity reduction benefits European eel larvae: Insights at the morphological and molecular level. PloS One 13, e0198294. doi: 10.1371/journal.pone.0198294
Reynhout S., Janssens V. (2019). Physiologic functions of PP2A: Lessons from genetically modified mice. Biochim. Biophys. Acta (BBA) - Mol. Cell Res. 1866, 31–50. doi: 10.1016/j.bbamcr.2018.07.010
Roué M., Darius H. T., Chinain M. (2018). Solid phase adsorption toxin tracking (SPATT) technology for the monitoring of aquatic toxins: A review. Toxins 10 (4), 167. doi: 10.3390/toxins10040167
Salgado P., Vázquez J., Riobó P., Franco J., Figueroa R., Kremp A., et al. (2016). A kinetic and factorial approach to study the effects of temperature and salinity on growth and toxin production by the dinoflagellate alexandrium ostenfeldii from the baltic sea. PloS One 11, e0147461. doi: 10.1371/journal.pone.0147461
Sar E., Ferrario M., Reguera B. (2002). Floraciones Algales Nocivas en el Cono Sur Americano (España: Instituto Español de Oceanografía).
Schneider W., Donoso D., Garcés-Vargas J., Escribano R. (2017). Water-column cooling and sea surface salinity increase in the upwelling region off central-south Chile driven by a poleward displacement of the South Pacific High. Prog. Oceanography 151, 38–48. doi: 10.1016/j.pocean.2016.11.004
Soto-Mendoza S., Castro L., Llanos-Rivera A. (2010). Variabilidad espacial y temporal de huevos y larvas de Strangomera bentincki y Engraulis ringens, asociados a la desembocadura del río Itata, Chile. Rev. biología marina y oceanografía 45, 471–487. doi: 10.4067/S0718-19572010000300012
Stawicki T., Owens K., Linbo T., Reinhart K., Rubel E., Raible D. (2014). The zebrafish merovingian mutant reveals a role for pH regulation in hair cell toxicity and function. Dis. Model. Mech. 7, 847–856. doi: 10.1242/dmm.016576
Tanimoto M., Ota Y., Inoue M., Oda Y. (2011). Origin of inner ear hair cells: morphological and functional differentiation from ciliary cells into hair cells in zebrafish inner ear. J. Neurosci. 31, 3784–3794. doi: 10.1523/JNEUROSCI.5554-10.2011
Tillmann U., Elbrächter M., Krock B., John U., Cembella A. (2009). Azadinium spinosum gen. et sp. nov. (Dinophyceae) identified as a primary producer of azaspiracid toxins. European Journal of Phycology 44, 63–79. doi: 10.1080/09670260802578534
Trainer V., Moore S., Hallegraeff G., Kudela R., Clement A., Mardones J., et al. (2020). Pelagic harmful algal blooms and climate change: Lessons from nature’s experiments with extremes. Harmful Algae 91, 101591. doi: 10.1016/j.hal.2019.03.009
Valdiglesias V., Prego-Faraldo M., Pásaro E., Méndez J., Laffon B. (2013). Okadaic acid: more than a diarrheic toxin. Mar. Drugs 11, 4328–4349. doi: 10.3390/md11114328
Varsamos S., Nebel C., Charmantier G. (2005). Ontogeny of osmoregulation in postembryonic fish: A review. Comp. Biochem. Physiol. Part A: Mol. Integr. Physiol. 141, 401–429. doi: 10.1016/j.cbpb.2005.01.013
Vásquez P., Llanos-Rivera A., Castro L. (2010). Abnormalities during the embryonic development in araucanian herring, Strangomera bentincki, in natural environment. Rev. biología marina y oceanografía 45, 177–185. doi: 10.4067/S0718-19572010000100020
Velasco J., Gutiérrez-Cánovas C., Botella-Cruz M., Sánchez-Fernández D., Arribas P., Carbonell J. A., et al. (2018). Effects of salinity changes on aquatic organisms in a multiple stressor context. Phil. Trans. R. Soc 374, 20180011. doi: 10.1098/rstb.2018.0011
Wang H., Kim H., Park H., Ki J. (2022). Temperature influences the content and biosynthesis gene expression of saxitoxins (STXs) in the toxigenic dinoflagellate Alexandrium pacificum. Sci. Total Environ. 802, 149801. doi: 10.1016/j.scitotenv.2021.149801
Wang J., Li R., Liu B., Zhang Q., Wang X., Zhu Y., et al. (2023). Occurrence and distribution of lipophilic marine algal toxins in the coastal seawater of Southeast China and the South China Sea. Mar. pollut. Bull. 187, 114584. doi: 10.1016/j.marpolbul.2023.114584
Wells M., Trainer V., Smayda T., Karlson B., Trick C., Kudela R., et al. (2015). Harmful algal blooms and climate change: Learning from the past and present to forecast the future. Harmful Algae 49, 68-93. doi: 10.1016/j.hal.2019.101632
Wells M., Karlson B., Wulff A., Kudela R., Trick C., Asnaghi V., et al. (2020). Future HAB science: Directions and challenges in a changing climate. Harmful Algae 91, 101632. doi: 10.1016/j.hal.2019.101632
Xu Y., Zhang Y., Zhang A., Zhang Q., Wu T., Wang J. (2004). Attenuation of okadaic acid-induced hyperphosphorylation of cytoskeletal proteins by heat preconditioning and its possible underlying mechanisms. Cell Stress Chaperones 9, 304–312. doi: 10.13792/FCSC-23R1.1
Yang Y., Li A., Qiu J., Yan W., Han L., Li D. (2023). Effects of lipophilic phycotoxin okadaic acid on the early development and transcriptional expression of marine medaka Oryzias melastigma. Aquat. Toxicol. 260, 106576. doi: 10.1016/j.aquatox.2023.106576
Keywords: hair cells, neuromasts, okadaic acid, Engraulis ringens, environmental variations, small pelagic fish
Citation: Pepe-Vargas P, Castro LR, Alves-de-Souza C and Llanos-Rivera A (2024) Effects of the harmful algal bloom toxin, okadaic acid, on the mechanoreceptors of larval anchoveta (Engraulis ringens) under varying environmental conditions. Front. Mar. Sci. 11:1446509. doi: 10.3389/fmars.2024.1446509
Received: 10 June 2024; Accepted: 02 September 2024;
Published: 07 October 2024.
Edited by:
Ana Maulvault, Portuguese Institute for Sea and Atmosphere (IPMA), PortugalReviewed by:
Wanchun Guan, Wenzhou Medical University, ChinaRui Cereja, Center for Marine and Environmental Sciences (MARE), Portugal
Copyright © 2024 Pepe-Vargas, Castro, Alves-de-Souza and Llanos-Rivera. This is an open-access article distributed under the terms of the Creative Commons Attribution License (CC BY). The use, distribution or reproduction in other forums is permitted, provided the original author(s) and the copyright owner(s) are credited and that the original publication in this journal is cited, in accordance with accepted academic practice. No use, distribution or reproduction is permitted which does not comply with these terms.
*Correspondence: Alejandra Llanos-Rivera, YWxsbGFub3NAdWRlYy5jbA==