- 1Department of Biomedical Sciences, University of Padova, Padua, Italy
- 2Institute of Clinical Physiology, National Research Council (CNR), ASST Grande Ospedale Metropolitano Niguarda, Milan, Italy
- 3ATIP Hyperbaric Medical Center, Padua, Italy
- 4Sea Mammal Research Unit, Scottish Oceans Institute, University of St. Andrews, St. Andrews, Scotland, United Kingdom
- 5TEAMHealth Research Institute, Tampa General Hospital, Tampa, FL, United States
- 6Center for Hyperbaric Medicine and Environmental Physiology, Department of Anesthesiology, Duke University Medical Center, Durham, NC, United States
Background: Current diving physiology postulates that SCUBA divers’ arterial blood gas (ABG) levels vary proportionally to environmental pressure, but, to date, ABGs have only been obtained during simulated dives. Also, recent evidence supports the use of the arterial/alveolar (a:A) partial pressures of oxygen (PO2) ratio to predict the arterial PO2 (PaO2) under hyperbaric conditions from measurements obtained at 1 atmosphere absolute (ATA). This work summarizes ABGs obtained in SCUBA divers in real underwater conditions and aims to validate the a:A ratio in predicting PaO2 in this subset of individuals at depth.
Methods: The study was approved by the local ethics committee. After cannulating the radial artery of the non-dominant limb, ABGs were sampled at the surface before the dive (A), at depth (15 meters of freshwater (mfw) or 42 mfw) before (B) and after (C) pedaling on a submersed bicycle for 10 minutes, and back at surface (D). After calculating the surface alveolar PO2 for each subject, the a:A ratio was obtained and used to predict PaO2 at depth. A linear regression between measured and predicted PaO2 was reported, along with the goodness-of-fit F test.
Results: Six subjects performed the dive at 15 mfw, and four others at 42 mfw. The PaO2 proportionally increased at both depths, remaining stable before and after pedaling. The a:A calculated from the baseline ABG obtained at rest, out of the water, adequately predicted the PaO2 at depth (R2 = 0.97, p<0.001), better at 15 mfw but losing accuracy at 42 mfw.
Conclusions: The ABGs confirmed the proportional rise of PaO2 in SCUBA divers underwater. The a:A ratio could be used to predict the magnitude of PaO2 rise at depth to limit exposure to hyperoxia, especially in repetitive recreational dives and professional divers.
1 Introduction
Despite the shift to terrestrial living, the human body has retained its capacity to adapt to the underwater environment. Unique features such as cold temperatures, impaired breathing, and increased ambient pressure cause significant derangements in normal physiology, particularly affecting gas exchange in the lungs and distribution of oxygen (O2) and carbon dioxide (CO2) in the body. Breath-hold diving (BHD) is the first technique developed by humans to dive, allowing limited time underwater: during descent, arterial partial pressures (Pa) of O2 and CO2 increase while, during the ascent, PaCO2 slowly rises and PO2 progressively falls due to metabolic consumption and redistribution in re-expanding lungs (Bosco et al., 2018b; Lindholm and Lundgren, 2009). These principles have been established based on theory (Lindholm and Lundgren, 2009), arterial blood gases (ABGs) obtained from simulated dives in hyperbaric chambers (Linér et al., 1993), and dives in natural environmental conditions (Barković et al., 2022; Bosco et al., 2020, 2018a; Paganini et al., 2023; Scott et al., 2021).
The invention of self-contained underwater breathing apparatus (SCUBA) allowed humans to prolong their submersion thus exacerbating pathophysiological changes. While the increased pressure at depth compresses the thorax, the SCUBA circuit provides the diver with air at the same pressure, thus avoiding lung collapse. As per Dalton’s law, the partial pressures of gases in air breathed by the SCUBA diver increase proportionally to the depth reached. Thus, the partial pressure of oxygen in the SCUBA diver’s arterial blood is higher than at the surface at 1 absolute atmosphere (ATA).
A recent systematic review reports only three studies investigating blood gas variations in SCUBA divers (Paganini et al., 2022). In particular, the examined subjects exercised while submerged inside water-filled tanks during simulated dives in hyperbaric chambers to 4.7 atmosphere absolute (ATA), breathing different gas mixtures. The results of these studies show that both PaO2 and PaCO2 increased at the simulated depth (Cherry et al., 2009; Fraser et al., 2011; Peacher et al., 2010). Interestingly, the arterial/Alveolar oxygen partial pressure ratio (a:A) calculated at 1 ATA accurately predicted PaO2 at increased ambient pressure (Moon et al., 1987; Paganini et al., 2022). However, no studies have been conducted in the natural underwater environment at pressures usually attained by SCUBA divers. PaO2 values could not be exactly proportional to the reached depth, since several other external factors or compensatory mechanisms may affect this principle. For example, closing volume also is higher during immersion (which has been interpreted as due to some air trapping), consequently inverting the traditional cephalo-caudad distribution of blood and potentially altering ventilation/perfusion ratio (Moon et al., 2009). Moreover, alterations in the properties of inhaled gases (e.g., increased density and flow resistance, depending on mixture composition) or changes in respiratory mechanics and resistive and elastic load result in increased work of breathing (Bosco et al., 2018b; Moon et al., 2009). By measuring blood gases at surface and at two different depths, this study aimed at verifying the progressive PaO2 rise expected with increasing pressures and to confirm that the PaO2 at depth can be accurately predicted from the a:A ratio calculated at sea level.
2 Materials and methods
2.1 Subjects
Well-trained, healthy SCUBA divers were considered eligible for the study and recruited through public advertising. Inclusion criteria were age ≥ 18 years and no medical history of orthopedic, cardiovascular, liver, renal, or metabolic disorders. Exclusion criteria were pregnancy, allergy to local anesthetics, coagulation abnormalities, alterations in the arterial vascularization of upper limbs, or vasculopathy.
2.2 Experimental design and diving plan
The experimental protocol was approved by the Human Ethical Committee of the Department of Biomedical Science of the University of Padova (No. HEC-DSB/03-18; addendum: Nov/2022) and adhered to the principles of the Helsinki Declaration.
Before inclusion, all enrolled subjects underwent preliminary medical screenings, and written informed consent was collected.
A meeting was held fourteen days before the planned beginning of each experimental session to explain the protocol and help the included subjects familiarize themselves with the procedures to ensure their correct execution. All the experiments were carried out at the “Y-40 – The Deep Joy ®” pool in Montegrotto Terme (Padova, Italy) to ensure stable and safe environmental conditions and a controlled water temperature (31.5 ± 0.5°C). Each participant was medically screened again on the day of the experiment, including a standard set of vitals (non-invasive blood pressure, heart rate, pulse oximetry, axillary body temperature), age, height, and weight.
SCUBA (open-circuit) diving was the chosen technique for these experiments. Specifically, each participant wore a buoyancy compensator, a standard pair of fins, and a single 15-liter tank carrying compressed air. The divers reached the predefined depth (15 meters of freshwater (mfw) or 42 mfw, corresponding to some of the pool’s large steps) to pedal on a submerged bike (OKEO Srl, Genova, Italy), set at 100 W (plus an estimated 50 W to move legs underwater = 150 W total effort) at a rate of 60 rpm for ten minutes (Borg, 1982). The diving assistant checked the target depth on each diver’s diving computer, adjusting the bike’s saddle to accommodate for height differences among the subjects. SCUBA divers then ascended at 10 m/min, with a decompression stop as required and a final safety stop of 3 minutes at 5 mfw.
2.3 Arterial cannulation and sampling protocol
As previously described (Bosco et al., 2020, 2018a), the radial artery of the non-dominant limb was cannulated with a 20 G (3 Fr) Teflon catheter under local anesthesia after verifying radial and ulnar arteries patency through an Allen test (Fuhrman et al., 1992) and using a “catheter over the needle” technique. The cannula was fixed to the skin by an adhesive band and connected to a circuit with a Luer Lok-type fitting to avoid leakages. Differently from previous publications (Bosco et al., 2020, 2018a), the circuit was assembled – in a proximal to distal order – with (Figure 1):
- one semi-rigid plastic connection, single lumen, length 10 cm, ending with a stopcock;
- one stopcock manifold;
- two 3 ml heparinized plastic syringes for standard blood gas sampling connected on the first and second channel by one stopcock each (to avoid sample contamination with water after detachment);
- one 10 ml plastic syringe used for arterial blood aspiration before sampling (“dead space”) on the distal channel;
- one 10 ml plastic syringe filled with 10 ml of 0.9% NaCl solution on the distal channel for flushing and wash-out of the arterial line after the sample collection.
If any technical issues related to arterial line placement were encountered during the procedure, the plan was to immediately halt the procedure and remove the catheter.
A first arterial blood sampling was drawn after arterial access placement while at rest, sitting on a stretcher (STEP A). The circuit was then attached and filled with a 0.9% NaCl solution, paying attention to carefully removing all gas bubbles inside. A safety briefing before the dives trained the subjects to turn the stopcock or apply direct and firm pressure on the vascular access site to prevent critical bleeding in case of disconnections or accidental removal. Further arterial blood samples were obtained at depth before (STEP B) and after (STEP C) pedaling on the submerged bike, in sitting position.
Blood collection sampling underwater in STEP B consisted of the following: 1) Opening of the most distal channel; 2) Slow aspiration of at least 5 ml of arterial blood with the distal plastic syringe; 3) Holding the plunger of the syringe firmly, closure of the distal channel; 4) Opening of the manifold’s most proximal channel, slow aspiration of at least 2 ml of arterial blood into the heparinized syringe; 5) Holding the plunger of the syringe firmly, closure of the first channel; 6) transient opening of the distal channel to flush the circuit; 7) Detachment of the first heparinized syringe plus one stopcock to avoid contamination of the sample by water. The procedure was repeated using the heparinized syringe on the second channel for STEP C. Both samples were sent to the surface inside a basket by inflating a lifting bag. The last arterial blood sample (STEP D) was obtained at the surface, within five minutes after the end of the dive and sitting out of the water.
At the end of the experiments, the arterial access was removed under aseptic conditions, and a compression bandage was applied for 2 hours. The insertion site was then monitored over the next 2 days for complications.
Arterial blood samples were preserved on ice and analyzed within 5 minutes, assuming that ABGs from normal individuals are stable for more than half an hour at room temperature and that this time can be extended to several hours if preserved on ice (Knowles et al., 2006). The retrieved blood samples were processed in the blood gas analyzer present on site (Epoc Blood Analysis System/NXS Host, model PD470SH-B, Siemens Healthcare AG, Erlangen, Germany), and the following values were tracked: pH; arterial partial pressure of oxygen (PaO2); and carbon dioxide (PaCO2).
2.4 Data analysis
All data obtained were coded onto a master sheet using a Microsoft Office Excel spreadsheet (Version 2016, Microsoft Corporation, Redmond, WA). Where appropriate, the Shapiro–Wilk test was utilized to determine the distribution of quantitative data; then, means and standard deviation (SD) were used in normally distributed samples, whereas median and interquartile range (IQR; 25% - 75%) were used in non-normal distributions. A paired t-test was used to test for differences between normally distributed groups; the Wilcoxon test for dependent samples was instead used for non-normal distributions (results described with sum of signed ranks (W), p-value, and rank biserial correlation (rrb)).
The a:A ratio was obtained as previously described by Moon et al. (Moon et al., 1987) and Paganini et al (Paganini et al., 2022). Briefly, the alveolar PO2 (PAO2) was calculated using the following formula:
where: FiO2=inspired oxygen fraction; Pb=ambient pressure; PaCO2=arterial PCO2 (assumed to be equal to alveolar PCO2); R (respiratory exchange ratio)=VCO2/VO2 (assumed equal to 0.8). Then, the basal a:A PO2 ratio was calculated at STEP A. The predicted PaO2 at increased ambient pressure (STEP B and C) was obtained from the basal a:A ratio and the calculated PAO2. Finally, a linear regression was calculated to assess the association between measured and predicted PaO2, along with the goodness-of-fit F test.
Significance was set at p<0.05. All statistical analysis was performed using R Statistical Software (version 3.6; R Foundation for Statistical Computing, Vienna, Austria).
3 Results
Ten SCUBA divers, balanced for gender, were included in the study. Their characteristics are reported in Table 1. Six completed the experiments diving to 15 mfw and four others to 42 mfw, without reporting complications and with no missing data (listed in Table 2).
Basal samples obtained after positioning the arterial cannula at rest (STEP A) demonstrated values within normal ranges in both groups.
Diving to 15 mfw, a significant increase in PaO2 was registered from a median of 84 (82–98) mmHg in step A to a mean of 259 ± 12 and 257 ± 12 mmHg in STEPS B and C, respectively (Wilcoxon test; W=0.00, p=0.03, rrb= -1.0); then, PaO2 significantly returned to 102 ± 4 mmHg at surface (p<0.001). No statistically significant differences in PaO2 were found between the samples at depth before and after pedaling; there was a small but statistically significant 4 mmHg decrease in PaCO2 during pedaling (p=0.048). The divers in the 42 mfw group demonstrated similar PaO2 trends, with significantly higher mean values reached at depth (477 ± 19 and 483 ± 38 mmHg in STEPS B and C) than surface values, with no significant changes in PaCO2 during and after pedaling (Table 2).
No significant variations were found in the pH, except for a significant increase from 7.42 in the after-pedaling sample (C) to 7.46 at surface (D) (p=0.003) in the 15 mfw group. This alkalosis mirrored the hypocapnia found in STEP D (31 ± 3 mmHg), which was significantly less than other PaCO2 values measured in all the previous steps (with a higher significance versus STEP A: p<0.001). Despite lack of statistical significance, a similar trend can be noted in the 42 mfw dives, step D, with a median pH increasing to 7.45 (7.43–7.46) and a mean PaCO2 decreasing to 35 ± 3 mmHg.
The overall regression between measured and predicted PaO2 was statistically significant(F(1,38) = 1419, p<0.001) and showed a clear linear relationship (R2 = 0.97). Nevertheless, values collected at higher pressures (42 mfw) tended to be overestimated by the equation and have a higher weight in the estimation of the regression error, resulting in a slope< 1 (Figure 2).
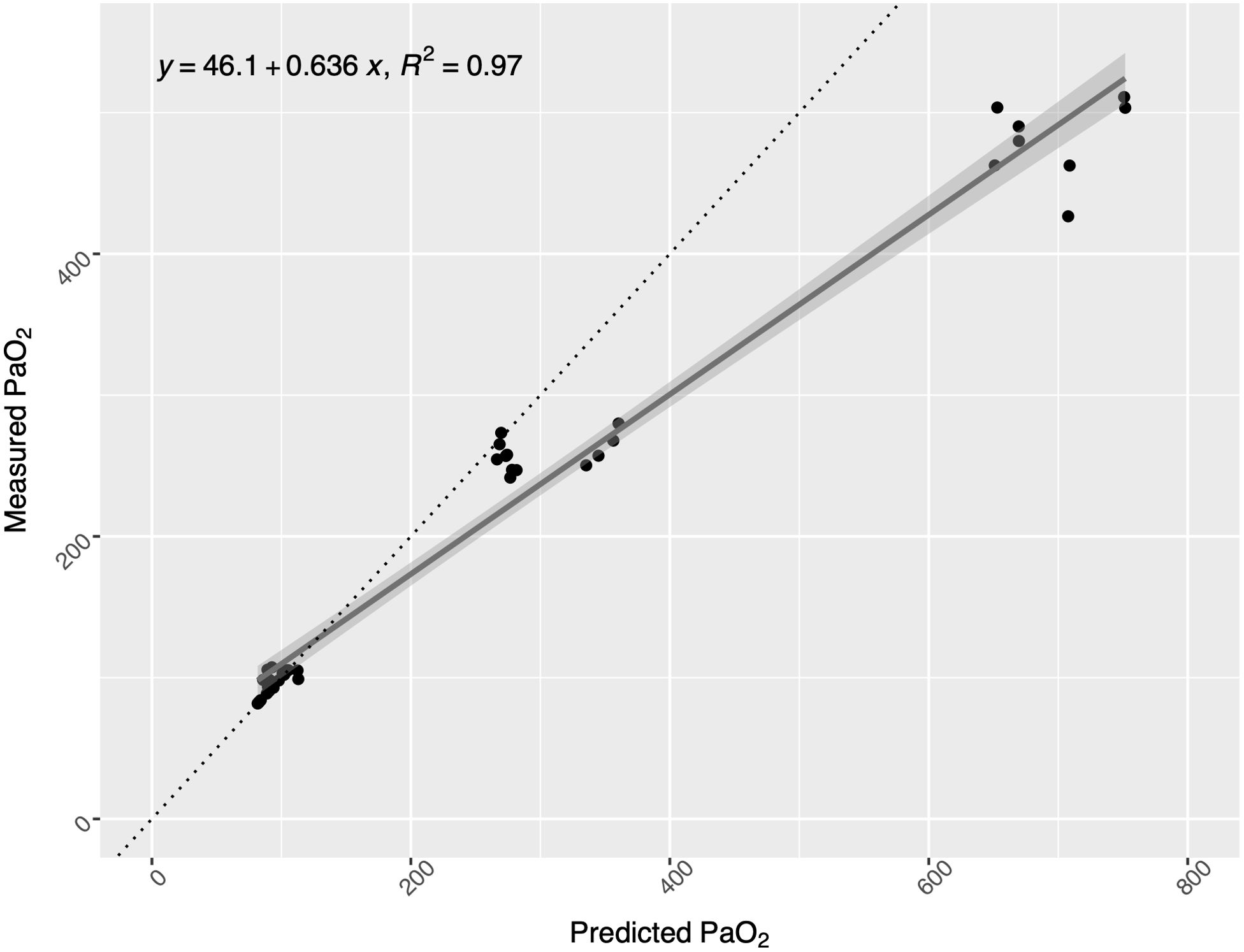
Figure 2. Scatter plot of measured vs. predicted arterial partial pressure of oxygen (PaO2) values: at surface (on the left), at 15 mfw (middle values), and at 42 (on the right). The regression model (with the 95% confidence interval represented in gray) accurately fits the data (R2 = 0.96, F(1,30) = 672.8, p<0.001), although PaO2 is overestimated at 40 msw depth.
4 Discussion
In this experiment, blood gas variations were investigated on SCUBA divers in the underwater environment. The most important results include arterial blood sampled at depth before and after pedaling on a submerged bike to reproduce recreational diving activity.
As expected, PaO2 significantly increased when SCUBA divers reached both 15 or 42 mfw, returning to normal values when resurfacing (Table 2). During submersion breathing from a SCUBA regulator, despite an increase in cardiac output and redistribution of blood into the thorax, pulmonary gas exchange remains stable. The results are also consistent with values obtained from SCUBA divers in previous experiments performed in wet tanks in hyperbaric chambers as simulated dives and not in the real underwater environment (Cherry et al., 2009; Fraser et al., 2011; Freiberger et al., 2016; Peacher et al., 2010). The first case of underwater ABG analysis on a SCUBA diver pedaling in the same setting (warm-water pool) demonstrated a similar PaO2 trend – reaching 236.7 mmHg after pedaling at depth (Di Pumpo et al., 2023).
Moreover, no relevant differences were found between mean PaO2 values before and after pedaling at 150 W on the submerged bike at both depths. Previous studies have already demonstrated this relative stability of PaO2; in particular, moderate exercise on land, at sea level, did not change pH, PaO2, and PaCO2 (Wasserman et al., 1967; Whipp and Wasserman, 1969). Underwater, the redistribution of blood from the extremities to the central circulation causes pulmonary vascular pressure elevation, leading to pulmonary edema in a small group of susceptible individuals. Blood pooling into the thorax reduces vital capacity (Moon et al., 2009), potentially influencing gas exchange while exercising at depth. However, Peacher et al. found no significant variations in PaO2 before and after a standardized underwater exercise at simulated pressure (Peacher et al., 2010) consistently with the present results.
Instead of the expected PaCO2 rise, comparisons at 15 showed a slightly significant decrease between steps B and C and a consistent drop in resurfacing samples (STEP D); a similar trend, not reaching respiratory alkalosis, can be noted in the 42 mfw group A possible explanation is the relatively low work rate set in this study to simulate regular recreational activity underwater, which could have led to lower PaCO2 values than those obtained in other simulated dives using higher effort levels (Cherry et al., 2009; Fraser et al., 2011; Peacher et al., 2010) and probably generating higher respiratory effort and fatigue. Furthermore, these experiments showing hypercapnia after exercising at simulated depth were performed in the prone position, and in cold (Fraser et al.: 19.9 – 20.9°C (Fraser et al., 2011)) or thermoneutral water (Cherry et al.: 30.0 ± 0.7°C (Cherry et al., 2009); Peacher et al.: 28.4 – 30.8°C (Peacher et al., 2010)) than in the present setting where subjects pedaled upright and in warm water (32°C). Finally, the subjects did not wear a suit underwater. Marabotti et al. suggested that wetsuits can affect respiratory function by adding an external pressure on the chest; experiments on non-immersed subjects demonstrated a shift towards a restrictive pattern after wearing a wetsuit, with a reduced functional residual capacity and expiratory reserve volume on spirometry, but an increased tidal volume (Marabotti et al., 2017).
As previously demonstrated (Paganini et al., 2022), the a:A ratio provided an adequate estimate of PaO2 at depth starting from surface PaO2, losing accuracy at higher depths. By minimizing potential sources of preanalytical error, such as calibrating the instrument as per manufacturer’s indications, or reducing time between sampling and analysis–which could allow diffusion of oxygen out of the blood sample–a possible explanation is the inaccuracy of blood gas analyzers at values currently not seen in the clinical context in which such instruments are used and calibrated. In this experiment, the investigated subjects breathed compressed air, developing significant hyperoxemia.
Limitations of the present study include the small sample size due to the difficult recruitment of participants, which currently hampers a generalization of the findings. Also, warm water could have mitigated blood shift and redistribution to the thorax. Future experiments will include further subjects and will be performed in natural conditions (open seawater) to reproduce real-environmental changes in SCUBA divers. Despite strictly adhering to the protocol to minimize preanalytical errors, samples obtained at depth could have been affected by analytical errors. Given that constancy of the PaO2/PAO2 ratio has already been demonstrated at 40 mfw (Cherry et al., 2009; Fraser et al., 2011; Paganini et al., 2022; Peacher et al., 2010) and in this study, the expected PaO2 values in the range of 350-750 mmHg exceed the highest possible calibration point of a blood gas analyzer, these measured values may have been outside the calibration range and hence artifactually low.
Current experiments confirmed the rise in arterial partial pressures of oxygen in SCUBA divers at depth. Instead, the PaCO2 decrease after pedaling at depth and after resurfacing still needs explanation. Finally, the a:A ratio adequately predicted PaO2 values at depth except at high predicted values between 650-750 mmHg.
Data availability statement
The original contributions presented in the study are included in the article/supplementary material. Further inquiries can be directed to the corresponding authors.
Ethics statement
The studies involving humans were approved by the Human Ethical Committee of the Department of Biomedical Science of the University of Padova, Italy (No. HEC-DSB/03-18; addendum: Nov/2022). The studies were conducted in accordance with the local legislation and institutional requirements. The participants provided their written informed consent to participate in this study.
Author contributions
MP: Writing – review & editing, Writing – original draft, Visualization, Validation, Resources, Methodology, Investigation, Funding acquisition, Formal Analysis, Data curation, Conceptualization. LZ: Writing – review & editing, Investigation. TG: Writing – review & editing, Investigation. LM: Writing – review & editing, Investigation. SM: Writing – review & editing, Investigation. GG: Writing – review & editing, Investigation. JM: Writing – review & editing, Resources, Methodology, Investigation, Conceptualization. EC: Writing – review & editing, Writing – original draft, Visualization, Validation, Supervision, Methodology, Investigation, Conceptualization. RM: Writing – review & editing, Writing – original draft, Visualization, Validation, Supervision, Project administration, Methodology, Investigation, Formal Analysis, Conceptualization. GB: Writing – review & editing, Writing – original draft, Visualization, Validation, Supervision, Resources, Project administration, Methodology, Investigation, Funding acquisition, Formal Analysis, Data curation, Conceptualization.
Funding
The author(s) declare financial support was received for the research, authorship, and/or publication of this article. This study was funded by the Office of Naval Research (Grant No. N00014-23-1-2757). Open Access funding provided by Università degli Studi di Padova | University of Padua, Open Science Committee.
Acknowledgments
We are grateful to dr. Giorgio Melloni for statistical advice provided.
Conflict of interest
The authors declare that the research was conducted in the absence of any commercial or financial relationships that could be construed as a potential conflict of interest.
Publisher’s note
All claims expressed in this article are solely those of the authors and do not necessarily represent those of their affiliated organizations, or those of the publisher, the editors and the reviewers. Any product that may be evaluated in this article, or claim that may be made by its manufacturer, is not guaranteed or endorsed by the publisher.
References
Barković I., Jurilj Z., Marinelli F., Maričić V., Pavlović M., Turk Wensveen T., et al. (2022). Arterial blood gases’ analysis in elite breath-hold divers at extreme depths. Eur. J. Appl. Physiol. 123 (4), 857–865. doi: 10.1007/s00421-022-05110-2
Borg G. A. (1982). Psychophysical bases of perceived exertion. Med. Sci. Sports Exercise 14, 377–381. doi: 10.1249/00005768-198205000-00012
Bosco G., Paganini M., Rizzato A., Martani L., Garetto G., Lion J., et al. (2020). Arterial blood gases in divers at surface after prolonged breath-hold. Eur. J. Appl. Physiol. 120, 505–512. doi: 10.1007/s00421-019-04296-2
Bosco G., Rizzato A., Martani L., Schiavo S., Talamonti E., Garetto G., et al. (2018a). Arterial blood gas analysis in breath-hold divers at depth. Front. Physiol. 9. doi: 10.3389/fphys.2018.01558
Bosco G., Rizzato A., Moon R. E., Camporesi E. M. (2018b). Environmental physiology and diving medicine. Front. Psychol. 9. doi: 10.3389/fpsyg.2018.00072
Cherry A. D., Forkner I. F., Frederick H. J., Natoli M. J., SChinazi E. A., Longphre J. P., et al. (2009). Predictors of increased PaCO2 during immersed prone exercise at 4.7 ATA. J. Appl. Physiol. 106, 316–325. doi: 10.1152/japplphysiol.00885.2007
Di Pumpo F., Meloni G., Paganini M., Cialoni D., Garetto G., Cipriano A., et al. (2023). Comparison between arterial blood gases and oxygen reserve indexTM in a SCUBA diver: A case report. Healthcare 11, 1102. doi: 10.3390/healthcare11081102
Fraser J., Peacher D. F., Freiberger J. J., Natoli M. J., SChinazi E. A., Beck I. V., et al. (2011). Risk factors for immersion pulmonary edema: Hyperoxia does not attenuate pulmonary hypertension associated with cold water-immersed prone exercise at 4.7 ATA. J. Appl. Physiol. (Bethesda Md.: 1985) 110, 610–618. doi: 10.1152/japplphysiol.01088.2010
Freiberger J. J., Derrick B. J., Natoli M. J., Akushevich I., SChinazi E. A., Parker C., et al. (2016). Assessment of the interaction of hyperbaric N2, CO2, and O2 on psychomotor performance in divers. J. Appl. Physiol. 121, 953–964. doi: 10.1152/japplphysiol.00534.2016
Fuhrman T. M., Pippin W. D., Talmage L. A., Reilley T. E. (1992). Evaluation of collateral circulation of the hand. J. Clin. Monit. 8, 28–32. doi: 10.1007/BF01618084
Knowles T. P., Mullin R. A., Hunter J. A., Douce F. H. (2006). Effects of syringe material, sample storage time, and temperature on blood gases and oxygen saturation in arterialized human blood samples. Respir. Care 51, 732–736.
Lindholm P., Lundgren C. E. (2009). The physiology and pathophysiology of human breath-hold diving. J. Appl. Physiol. 106, 284–292. doi: 10.1152/japplphysiol.90991.2008
Linér M. H., Ferrigno M., Lundgren C. E. (1993). Alveolar gas exchange during simulated breath-hold diving to 20 m. Undersea Hyperbaric Medicine: J. Undersea Hyperbaric Med. Society Inc 20, 27–38.
Marabotti C., Prediletto R., Scalzini A., Pingitore A., Passera M., Laurino M., et al. (2017). Cardiovascular and respiratory effects of the neoprene wetsuit in non-immersed divers. Undersea Hyperbaric Med. 44, 141–147. doi: 10.22462/3.4.2017.7
Moon R., Camporesi E., Shelton D. (1987). “Prediction of arterial PO2 during hyperbaric treatment,” in Proceedings of the Ninth International Symposium on Underwater and Hyperbaric Physiology. Bethesda, MD: Undersea & Hyperbaric Medical Society 1127–1131.
Moon R. E., Cherry A. D., Stolp B. W., Camporesi E. M. (2009). Pulmonary gas exchange in diving. J. Appl. Physiol. 106, 668–677. doi: 10.1152/japplphysiol.91104.2008
Paganini M., Moon R. E., Boccalon N., Melloni G. E. M., Giacon T. A., Camporesi E. M., et al. (2022). Blood gas analyses in hyperbaric and underwater environments: A systematic review. J. Appl. Physiol. 132, 283–293. doi: 10.1152/japplphysiol.00569.2021
Paganini M., Moon R. E., Giacon T. A., Cialoni D., Martani L., Zucchi L., et al. (2023). Relative hypoxemia at depth during breath-hold diving investigated through arterial blood gas analysis and lung ultrasound. J. Appl. Physiol 135 (4), 863–871. doi: 10.1152/japplphysiol.00777.2022
Peacher D. F., Pecorella S. R. H., Freiberger J. J., Natoli M. J., SChinazi E. A., Doar P. O., et al. (2010). Effects of hyperoxia on ventilation and pulmonary hemodynamics during immersed prone exercise at 4.7 ATA: Possible implications for immersion pulmonary edema. J. Appl. Physiol. 109, 68–78. doi: 10.1152/japplphysiol.01431.2009
Scott T., van Waart H., Vrijdag X. C. E., Mullins D., Mesley P., Mitchell S. J. (2021). Arterial blood gas measurements during deep open-water breath-hold dives. J. Appl. Physiol. 130, 1490–1495. doi: 10.1152/japplphysiol.00111.2021
Wasserman K., Van Kessel A. L., Burton G. G. (1967). Interaction of physiological mechanisms during exercise. J. Appl. Physiol. 22, 71–85. doi: 10.1152/jappl.1967.22.1.71
Keywords: SCUBA diving, arterial blood gas, environmental physiology, diving physiology, oxygen
Citation: Paganini M, Zucchi L, Giacon TA, Martani L, Mrakic-Sposta S, Garetto G, McKnight JC, Camporesi EM, Moon RE and Bosco G (2024) Arterial blood gases in SCUBA divers at depth. Front. Mar. Sci. 11:1445692. doi: 10.3389/fmars.2024.1445692
Received: 07 June 2024; Accepted: 09 October 2024;
Published: 29 October 2024.
Edited by:
Erik Seedhouse, Embry–Riddle Aeronautical University, United StatesReviewed by:
Richard Mahon, Henry M Jackson Foundation for the Advancement of Military Medicine (HJF), United StatesPeter E. Westerweel, Albert Schweitzer Ziekenhuis, Netherlands
Copyright © 2024 Paganini, Zucchi, Giacon, Martani, Mrakic-Sposta, Garetto, McKnight, Camporesi, Moon and Bosco. This is an open-access article distributed under the terms of the Creative Commons Attribution License (CC BY). The use, distribution or reproduction in other forums is permitted, provided the original author(s) and the copyright owner(s) are credited and that the original publication in this journal is cited, in accordance with accepted academic practice. No use, distribution or reproduction is permitted which does not comply with these terms.
*Correspondence: Matteo Paganini, bWF0dGVvLnBhZ2FuaW5pQHVuaXBkLml0; Gerardo Bosco, Z2VyYXJkby5ib3Njb0B1bmlwZC5pdA==