- Marine Ecology and Systematics (MarES), Department of Biology, University of the Balearic Islands, Palma de Mallorca, Spain
The environmental risk of coastal sunscreen pollution and ocean warming to seagrass meadows seems to be greatly intensified in the Mediterranean basin, due to its semi-enclosed nature that limits water renewal and the high influx of tourists it receives every year. Both stress factors could be interacting synergistically, thus, contributing to the current decline of Posidonia oceanica meadows. Our study aimed to determine the response of P. oceanica to the combined effects of elevated seawater temperature and sunscreen addition in a short-term laboratory experiment, testing an environmentally relevant sunscreen concentration in Mallorca, Spain (20 mg L-1) and a control (0 mg L-1) with the ambient temperature in spring (15°C) and a worst-case scenario of estimated temperature increase by 2100 (ambient + 5°C). Sunscreen addition promoted net primary production rates in the seagrass under ambient temperature, possibly due to nutrient enrichment from the mixture. Alkaline phosphatase activity (APA) in young leaves was enhanced under increased temperature only. Early-warning signs of the impacts of combined elevated temperature with sunscreen exposure in P. oceanica were the drastic decrease in leaf chlorophyll concentrations and inhibition of the nitrogen fixation associated with rhizomes (more than 50%), along with greater oxidative stress biomarkers in leaves (i.e., catalase activity and polyphenols content) and APA in roots (4-fold increase). The current investigation has revealed how the negative effects of coastal sunscreen pollution in this seagrass species may be exacerbated under climate change factors, such as ocean warming, with possible implications in the nutrient cycling and photosynthetic process of the plant. Investigations focused on determining the impacts of these contaminants in P. oceanica meadows and their interaction with additional stress factors in the environment is of great relevance for the future management of this declining ecosystem.
1 Introduction
Several environmental impacts brought by industrial development and anthropogenic activities are currently threatening marine ecosystems, including the increasing emissions of greenhouse gases that exacerbate climate change-related factors (i.e., ocean warming, acidification, deoxygenation, shifts of salinity and nutrients supply) and human activities that lead to chemical pollution, such as the release of whole sunscreen formulations into marine environments (He et al., 2023; IPCC, 2023). Ocean temperatures are projected to rise by an average of 1.6 to 4.3°C by the end of the century according to the Intergovernmental Panel on Climate Change (IPCC), with possible ranges of 0.9 to 2.4 and 3.2 to 5.4°C under the Representative Concentration Pathway - RCP 2.6 and RCP 8.5, respectively (IPCC, 2019). Ocean warming may interact with contaminants by increasing their release, degradation and transportation into marine environments, inducing higher bioaccumulation of these compounds and their inherent toxicity in the marine biota (Kibria et al., 2021; IPCC, 2023); thus, showing aggravated impacts when compared with the ones caused by pollutants acting alone (Bordalo et al., 2023). Moreover, according to Lopes et al. (2022), the bioavailability of contaminants in seawater can be directly controlled by ocean temperature. Past studies have discussed the possibility of seawater warming and exposure to pollutants acting additively or synergistically in the stress response of symbiotic cnidarians (Brown, 2000; Wijgerde et al., 2020). Experimental research has proven that temperature may affect the response of marine invertebrates to the toxicity of environmental contaminants, expressed through metabolic or oxidative stress (Pirone et al., 2019; Bai and Wang, 2020; Costa et al., 2020; Weber et al., 2020). However, these are among the few studies correlating the interaction between temperature rise and sunscreen components in seawater on marine organisms, and, in general, data involving multiple stressors considered together to assess their impacts is also scarce (Cuccaro et al., 2021; Rahi et al., 2021; Cuccaro et al., 2022; Bordalo et al., 2023).
Sunscreens are commercial products formulated for the protection of human skin against ultraviolet (UV) radiation damage, usually containing one or more UV filters, typically divided into physical and chemical agents, that absorb UV rays and convert them to thermal energy or reflect them, respectively (Sánchez-Quiles and Tovar-Sánchez, 2015; Schaap and Slijkerman, 2018). They are also composed of many other cosmetic ingredients, such as emollients, preservatives or stabilizers, emulsifiers, fragrances, and coloring compounds (Tovar-Sánchez et al., 2013; Fivenson et al., 2021). The extensive use of sunscreen products has led to the release of hundreds of tons of their components into several matrices of marine habitats, entering by directly washing off from human skin and clothing, and indirectly through treated wastewater, industrial discharges, and runoff (Molins-Delgado et al., 2014; Cadena-Aizaga et al., 2020). These chemicals represent an environmental risk for the integrity of marine ecosystems and their biota, being classified as pseudo-persistent pollutants and lately considered one of the most important contaminants of emerging concern designated by the U.S. Environmental Protection Agency (EPA) (Blitz and Norton, 2008; Díaz-Cruz and Barceló, 2015). Most studies to date are restricted to the effects of isolated UV filters in aquatic taxa, rather than the whole complex matrix that encompasses a sunscreen product, raising questions on how the combination of all their significant components altogether may enhance or alter the toxic effects of each component on its own (Tovar-Sánchez et al., 2019). In addition, most of the literature centers around the higher levels of the trophic web (Danovaro et al., 2008; Corinaldesi et al., 2017; Fel et al., 2019; Araújo et al., 2020; Cocci et al., 2020; Wong et al., 2020), leaving a greater gap of knowledge for marine primary producers. Past research on sunscreen pollution in seagrasses reports the accumulation of organic UV filters in their tissues (Agawin et al., 2022) and toxic effects, such as reduced photosynthetic efficiency and chlorophyll production, elevated oxidative stress indicators, leaf elongation inhibition, impairments in leaf cell structure and viability, and decreased nitrogen fixation associated with the seagrass epiphytes (Malea et al., 2019; Mylona et al., 2020a, Mylona et al., 2020b; García-Márquez et al., 2023b, García-Márquez et al., 2024).
Global warming, along with the increased intensity, frequency and duration over the past century of marine heatwaves (MHWs), i.e., prolonged discrete anomalously warm water events (Darmaraki et al., 2019a; Hobday et al., 2016; Oliver et al., 2018), may be influencing the decline of seagrass ecosystems, which are among the marine habitats with one of the most rapid recession rates in recent years, exhibiting global losses estimated at 2–5% year-1 (Duarte, 2002; Orth et al., 2006; Duarte et al., 2008; Waycott et al., 2009). These climate change-related factors implicate large-scale impacts on seagrass communities (Orth et al., 2006; Strydom et al., 2020) and on the ecosystem services that they provide (Aoki et al., 2021). Elevated seawater temperatures slow down the growth rates of temperate seagrass meadows and negatively affect their shoot survival, leading to stress responses, such as increased shoot population mortality rates (Oviatt, 2004; Reusch et al., 2005; Díaz-Almela et al., 2009) and mass flowering episodes (Díaz-Almela et al., 2007) promoted by heat waves. Temperatures above optimal also impair the light reception efficiency of photosystems, limiting nutrient uptake, protein synthesis, carbon assimilation and consequently raise the respiration rates of the plants (Berry and Bjorkman, 1980; Hammer et al., 2018), and lead to sublethal morphological modifications that induce leaf loss, which reduces leaf growth and triggers leaf necrosis (Guerrero-Meseguer et al., 2017; Chefaoui et al., 2018; Stipcich et al., 2022).
The environmental risk of sunscreen pollution and ocean warming seems to be intensified in the Mediterranean Sea basin, that on the one hand receives a massive influx of tourists every year and is conditioned by its semi-enclosed nature with limited exchanges with the ocean, high residence time of surface waters and its oligotrophic waters (Tovar-Sánchez et al., 2019), and on the other, experiences rates of seawater warming exceeding threefold those of the global ocean (IPCC, 2007; Vargas-Yáñez et al., 2007). One of the most relevant marine habitats that shelter the sediments of the Mediterranean are the Posidonia oceanica (L.) Delile meadows, declared as priority for conservation by the European Union (Annexe I, Dir. 92/43/EEC). The structural component that supports this complex habitat is an endemic and keystone marine angiosperm essential for maintaining the natural equilibrium of coastal ecosystems by oxygenating waters, cycling nutrients, sequestering carbon, providing nursery grounds, stabilizing sediments, and protecting the coastline from erosion (Procaccini et al., 1996; Duarte et al., 2005; Larkum et al., 2006; Fourqurean et al., 2017).
To our knowledge, there are no studies addressing the influence of warming on the effects of sunscreen components in seagrasses, despite the high vulnerability of these ecosystems to stress factors that could be interacting in a synergistic manner (Jackson, 2008), weakening the overall resistance and hindering the recovery of declining meadows. Therefore, the objective of our research was to determine the response of P. oceanica to the combined effects of elevated seawater temperature and sunscreen addition, testing the hypothesis that plants subjected to sunscreen exposure are more sensitive to increased temperature compared to those under no sunscreen addition. The interaction between elevated seawater temperature and sunscreen exposure on the health of P. oceanica was assessed through a short-term laboratory experiment in aquaria under spring conditions, recreating present and future temperatures (IPCC, 2019) with and without addition of commercial sunscreens.
2 Materials and methods
2.1 Sample collection and experimental design
Sunscreen treatments, i.e., 20 and 0 mg L-1, were factorially combined with the ambient temperature at the time of the seagrass collection (~15°C) and 5°C warmer (20°C). The sunscreen concentration selected was within environmentally relevant levels previously detected in Mallorca, Spain (range: 0–29 mg L−1; Agawin et al. unpub. data; García-Márquez et al., 2023b) and other Spanish beaches (range: 3.1–96.7 mg L−1; Tovar-Sánchez et al., 2020), while the temperature treatment simulated the worst case of estimated temperature increase by the end of the century according to the RCP 8.5 high emissions scenario (IPCC, 2019), and could represent an abnormally warm seawater episode as projected for future heat waves in the Mediterranean Sea (IPCC, 2014; Darmaraki et al., 2019b; García-Márquez et al., 2021, García-Márquez et al., 2022). Whole P. oceanica plants were collected from Caló Fort (39° 28′ 58.19″ N, 2° 43′ 59.95″ E) in March 2022 by freediving at 5 m depth, and carefully rinsed with seawater before being transported to the laboratory in containers filled with seawater. The seawater was collected on site and filtered through nylon Nitex filters of pore sizes of 1000 and 10 µm, to fill with 8 L each of 12 aquaria with 11 L of capacity. Three replicate aquaria without sediments were randomly assigned for the application of each treatment, and 8–10 seagrass shoots with roots and horizontal rhizome attached were introduced in each. A non-toxic underwater epoxy (D-D AquaScape) was applied in the cut ends of the horizontal rhizomes of the seagrasses to preserve their internal gas pressure. The aquaria were illuminated by diode lamps (Aquael Leddy Slim Sunny 5 W) to deliver optimal levels of photosynthetically active radiation (PAR) for P. oceanica (124 µmol photons m-2 s-1; Alcoverro et al., 1998; Lee et al., 2007) and configured with a timer for a photoperiod of lighting of 13 h light: 11 h dark cycles. Present day levels of carbon dioxide (CO2) were achieved by adjusting the partial pressure (pCO2) in the seawater of the aquaria via aeration with a mixture of air and CO2. The resulting gas mixture was controlled in each aquarium with flow meters of 2.5 L min-1 and flux diffusers. The incubations were done in a temperature-controlled room for 15 days, and the seagrasses were left to acclimatize under laboratory conditions for 48 h before applying the temperature/sunscreen treatments. The temperature of the seawater was regulated by connecting the aquaria to water chillers (HAILEA HC-130A) with a continuous circuit of water and heaters (Aquael EasyHeater 25 W) with the desired temperature previously set up in the devices. An assortment of eight (8) commercial sunscreens with varying formulations and properties (i.e., organic and inorganic UV filters, texture and solar protection factors) were used for the experiment (Table 1). A sunscreen standard stock solution of 5000 mg L-1 was prepared by mixing these sunscreens and dissolving them in filtered and autoclaved seawater (Tovar-Sánchez et al., 2020; García-Márquez et al., 2023b), and aliquots of the stock were diluted with the seawater of the aquaria to reach the desired sunscreen concentration.
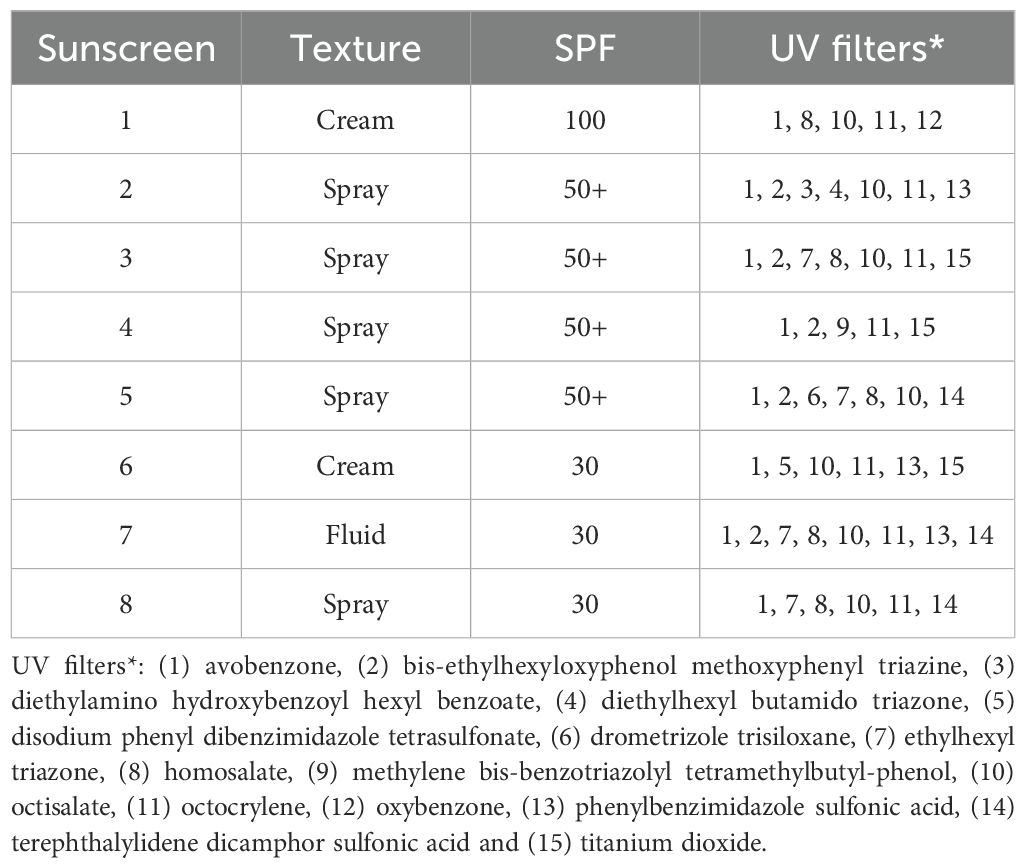
Table 1. Properties of the commercial sunscreens used in the experiment, specifying the brand, texture, sun protection factor (SPF) and UV filters indicated in the ingredients list.
2.2 Control of physicochemical variables
Seawater temperature and pH in the aquaria were followed constantly during the experiment and registered every 30 min with IKS-Aquastar and ENV-40-pH calibrated (with 4.0 and 7.0 pH NBS standards) sensors, respectively, connected to a D130 data logger (Consort) and computer. The daily average of incident PAR for the seagrasses was monitored in each aquarium using light loggers (HOBO). Initial and final seawater samples were collected from each aquarium during the incubation period to determine the dissolved nutrients concentrations, which were filtered with sterile polypropylene filter holders (0.2 µm) using a peristaltic pump (Geotech Geopump) and stored frozen until their analysis. Concentrations of NO2-, NO3- and TDN were measured according to the optimized Griess assay and vanadium (III) reduction protocol of Pai et al. (2021) with a persulfate digestion pre-treatment for TDN, and NH4+ was detected fluorometrically based on the modified procedure of Horstkotte and Duarte (2012). The analyses of PO43- and TDP were done following the modified methods of Knap et al. (1997) and Weber-Shirk et al. (2001), considering the persulfate digestion for TDP samples.
2.3 Assessment of primary production and respiration rates of Posidonia oceanica
The primary production and respiration rates of P. oceanica were estimated through the dissolved oxygen (DO) evolution technique, as described by the modified Winkler method of Labasque et al. (2004). Four initial Exetainer vials (12 mL) and four 125 mL Winkler flasks, divided into two each for light and dark incubations, were carefully filled with filtered (0.2 µm) seawater from the aquaria, avoiding turbulences that could interfere with DO estimations. The second youngest leaves of four independent shoots per aquarium were extracted and cut into 5 cm segments from the top, scrapping off epiphytes when necessary. Leaf segments were distributed each per light and dark Winkler flask and submerged in their respective aquarium to incubate for three hours. Upon concluding the incubation period, two Exetainers were filled until overflowed with seawater from each Winkler bottle, reducing gas exchange as much as possible. Absorbances were measured at 466 nm in a Varian Cary 50 Conc UV-Visible spectrophotometer. Differences in DO concentrations from initial to final samples of light and dark Winkler flasks, respectively, provided the primary production parameters of the seagrass (i.e., net primary productivity, respiration and gross primary productivity), expressed as µmol O2 g DW-1 h-1.
2.4 Quantification of leaf chlorophyll contents in Posidonia oceanica
Chlorophyll concentrations were estimated with the method of Agawin et al. (1996), from the second youngest leaves of two independent P. oceanica shoots per aquarium. Approximately 0.1–0.3 g of wet weight of sample was ground in 1 ml of 96% ethanol and placed in 15 mL centrifuge tubes, filled up to 10 mL with 96% ethanol. Samples were stored in the dark for extraction for 12 h and centrifuged after at 2800×g for 10 min. Readings of absorbance were done at 665 and 649 nm with a Cary-50 Conc-UV Visible spectrophotometer and the equations presented by Wintermans and De Mots (1965) were used to estimate the final chlorophyll a and b concentrations.
2.5 Evaluation of the alkaline phosphatase activity associated with Posidonia oceanica
Rates of alkaline phosphatase activity (APA) were assessed in different tissues of P. oceanica with a fluorometric assay (Fernández-Juárez et al., 2019) that follows the hydrolysis of a fluorogenic substrate, i.e., (S) 4-methylumbelliferyl phosphate (MUF-P, Sigma-Aldrich) to 4-methylumbelliferyl (MUF). Seagrass tissues were sectioned in 5 cm pieces, consisting of the second oldest and youngest leaf from the top, unrinsed orthotropic rhizomes with scales and roots from two independent shoots from each aquarium. Leaf and root samples were placed in 15 mL centrifuge tubes filled with 10 mL of prefiltered (0.2 µm) and autoclaved seawater from their aquaria of origin, while the rhizomes were introduced in 50 mL tubes with 40 mL of the same seawater. A MUF-P solution (10 mM) was added in each tube to reach a final concentration of 3 µM and all samples were then incubated in agitation at room temperature and protected from light for 1 h. The MUF production in the water samples was determined after the incubation period in a microtiter plate with borate buffer at pH 10 (3:1 proportion of sample:buffer) with a Cary Eclipse spectrofluorometer (FL0902M009, Agilent Technologies) at 359 nm of excitation and 449 nm of emission, and final values were expressed as µM MUF g-1 dw h-1.
2.6 Biochemical analyses of oxidative stress in Posidonia oceanica leaves
The second oldest and youngest leaves of two independent shoots from each aquarium were extracted for the analyses. The seagrass leaves (approximately 0.8 g of wet weight) were homogenized with a disperser (IKA T 10 basic ULTRA-TURRAX and S 10 N - 10 G - ST) in five volumes (w/v) of 50 mM Tris-HCl buffer, 1 mM EDTA solution at pH 7.5, with liquid nitrogen and homogenates were centrifuged at 9000×g and 4°C for 4 min. Protein concentrations in the samples were estimated, to standardize the final data, with the colorimetric Thermo Scientific Coomassie (Bradford) Protein Assay Kit with Bovine Serum Albumin (BSA) as calibration reference. Reactive oxygen species (ROS) generation was analyzed measuring the oxidation rates of 2’,7’-dichlorofluorescein diacetate (DCFH-DA; Sigma) in sterilized seawater (expressed as arbitrary units-a.u. per mg of protein), reaching a concentration of 15 µg mL-1 in samples, at 25 °C in a FLx800 Microplate Fluorescence Reader (BioTek Instruments, Inc.) for 1 h, with an excitation of 480 nm and emission of 530 nm. Total phenolic content of P. oceanica leaves was quantified with the Folin-Ciocalteau colorimetric assay (Singleton et al., 1999) with tyrosine as standard, incubating the samples at room temperature for 90 min prior to measuring their absorbances at 760 nm in a UV-visible spectrophotometer Cary 100 Conc, Varian. Catalase activity was assessed following the protocol of Aebi (1984), based on the decomposition of hydrogen peroxide (H2O2) detected with the decrease in absorbance from samples in 50 mM phosphate buffer at 240 nm with a UV-Vis Cary 300 Bio spectrophotometer at 25°C. Activity rates were calculated using the equation of the rate constant of a first-order reaction (K) and expressed as mK per mg of protein.
2.7 Determination of N2 fixation rates associated with Posidonia oceanica
The N2 fixation activity associated with different tissues of P. oceanica were evaluated with the acetylene reduction assay (ARA; Stal, 1988; Capone, 1993; Agawin et al., 2014). Seagrass tissues were cut into 5 cm pieces, consisting of the second oldest leaf from the top, orthotropic rhizome with scales and roots from two independent seagrass shoots from each aquarium. Activity from root endophytes was determined from roots that underwent a surface sterilization process: 99% ethanol 1 min; 3.125% NaClO 6 min; 99% ethanol 30 s; autoclaved and filtered seawater final washing (Coombs and Franco, 2003). Leaf and root segments were introduced in 10 mL gas chromatograph (GC) vials and the rhizomes were placed in 50 mL centrifuge tubes. All samples were humidified with sterilized seawater from their aquaria of origin (1 mL in GC vials and 2.5 mL in centrifuge tubes) and sealed with gas-tight septum ports. Belowground tissues were flushed with helium gas for 1 min to obtain anoxic conditions. Acetylene gas was injected into the samples to reach 20% (v/v) concentrations with gas-tight Hamilton syringes and left to incubate in their aquaria for 3 h. Then, 10 mL of headspace was extracted from samples and injected into Hungate tubes sealed with hot melt glue to prevent gases from leaking (Agawin et al., 2014). Ethylene and acetylene concentrations were quantified with a gas chromatograph (7890A, Agilent Technologies). Finally, the equations of Stal (1988) were applied to transform the acetylene reduction rates were to N2 fixation rates using a factor of 4:1 (C2H4:N2 reduced; Jensen and Cox, 1983).
2.8 Statistical analysis of data
Data from all analyses are presented as average ± standard deviation of replicates per treatment (n=3). The normality of the datasets was evaluated with the Shapiro-Wilk (n<50) and Kolmogorov-Smirnov (n>50) goodness of fit tests, and the homogeneity of variances among groups was assessed using Levene’s test. Log-transformation was applied for data that did not satisfy these conditions. The effects of sunscreen addition under elevated temperature on the primary production and leaf chlorophyll concentrations of P. oceanica were assessed with linear mixed models (LMM), including the aquarium of the samples as random factor. For the rest of biological responses, i.e., APA, ROS production, total phenolic content, catalase activity and N2 fixation, LMM were also analyzed, considering the interaction between treatment and plant tissue. The a posteriori analyses were done following the Tukey test for multiple comparisons of means. All statistical analyses were performed in the R package, version 4.0.3.
3 Results
3.1 Fluctuations in physicochemical data
Differences in sunscreen concentrations in the dissolved and total fractions and temperature treatments were statistically significant (p<0.05). In the total fraction of seawater, background sunscreen concentrations in the aquaria without addition of commercial sunscreens were on average of 6.36 ± 2.84 and 9.73 ± 4.60 mg L-1 at ambient and elevated temperatures, respectively (Figure 1). Total sunscreen concentrations in aquaria under sunscreen treatment were on average of 18.84 ± 2.82 and 24.33 ± 4.66 mg L-1 at ambient and elevated temperatures, respectively. For treatments without sunscreen addition, mean values in the dissolved fraction were of 4.48 ± 3.15 and 6.38 ± 4.58 mg L-1 at ambient and elevated temperatures, respectively, while in aquaria under sunscreen exposure, values were of 15.17 and 19.21 mg L-1. Daily fluctuations in the seawater pH ranged from 0.16 to 0.52 units (Figure 2A). Mean values of pH were lower (p<0.05) in the aquaria at ambient temperature (7.80 ± 0.15 and 7.81 ± 0.16 at 0 and 20 mg L-1 of sunscreen, respectively) compared to those under elevated temperature treatments (7.94 ± 0.18 and 7.96 ± 0.18 at 0 and 20 mg L-1 of sunscreen, respectively). Average seawater temperatures in the ambient temperature treatments were 15.11 ± 0.24 and 15.24 ± 0.30°C at 0 and 20 mg L-1 of sunscreen exposure, respectively, and in the elevated temperatures ones were 20.12 ± 0.87 and 19.88 ± 0.32°C (Figure 2B). Nutrient analyses in seawater (Table 2) evidenced an increment in the final concentrations of NO2- (p<0.05), as well as PO43- and TDN (p>0.05), in comparison to the initial values. Final contents of dissolved NH4+ did not differ from the initials, although values were greater (p<0.05) in the aquaria under elevated temperatures with no sunscreen addition (7.36 ± 5.79 µM) than in those at ambient temperatures (1.94 ± 0.60 and 1.94 ± 0.39 µM at 0 and 20 mg L-1 of sunscreen, respectively). Concentrations of NO3- decreased in all treatments towards the end of the experiment (p>0.05), while final TDP content was lower compared with the initials in most aquaria, except for those under sunscreen exposure at 15°C.
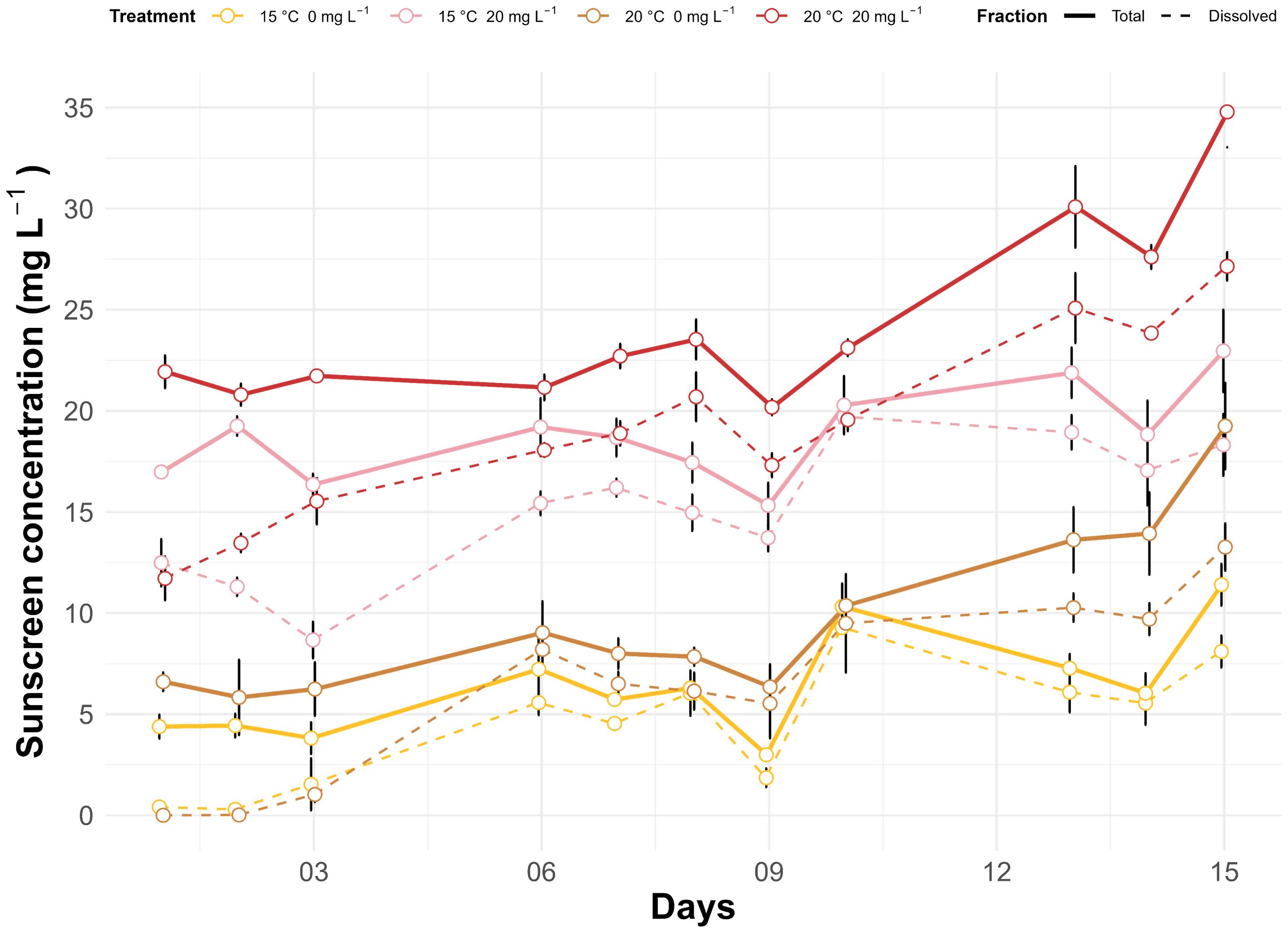
Figure 1. Average concentrations (n=3) of the total and dissolved fractions of commercial sunscreens in the seawater of the aquaria under different treatments during the incubation period. Error bars represent ± standard error.
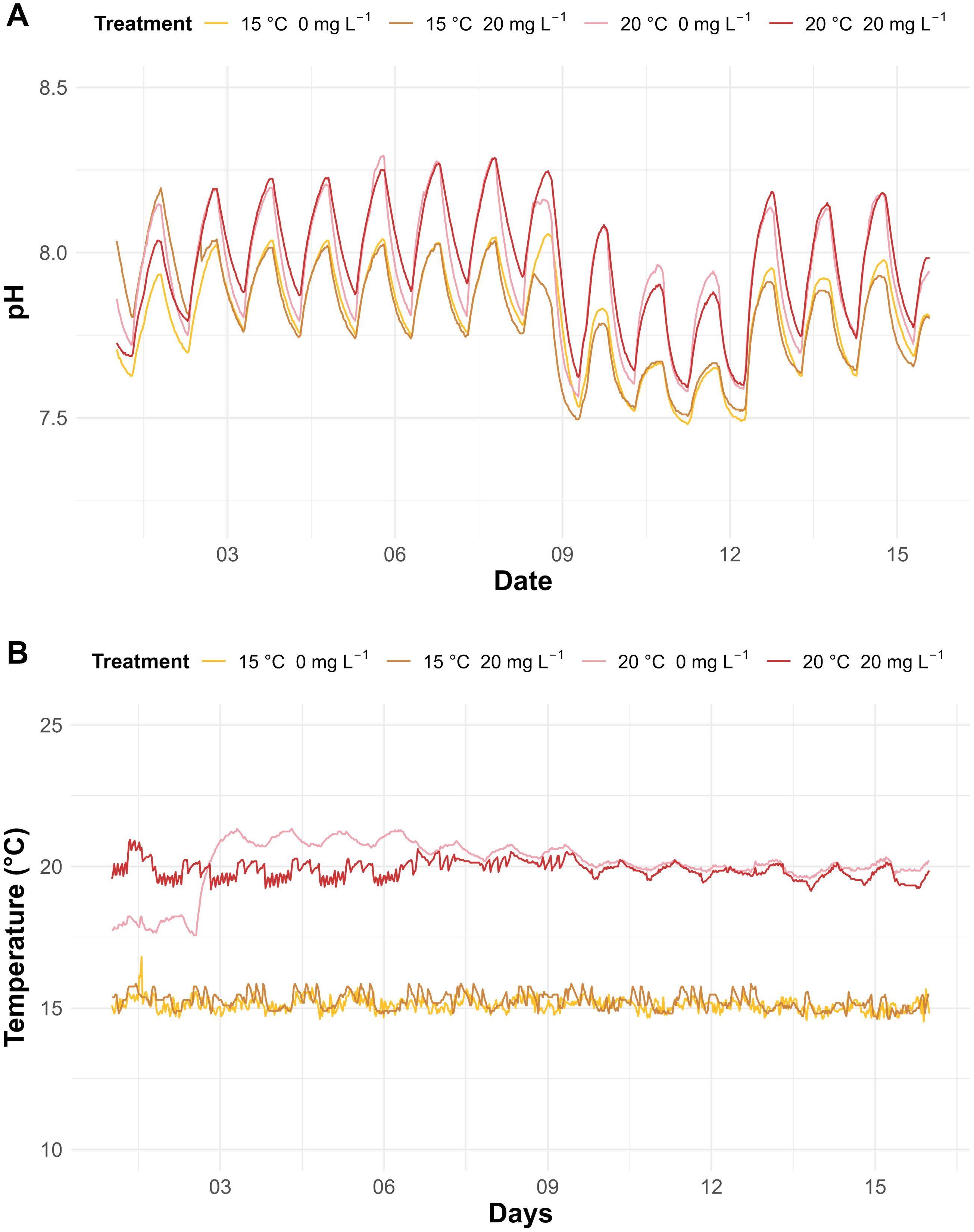
Figure 2. Diurnal changes in the seawater pH (A) and temperature (B) of the treatments during the experiment.
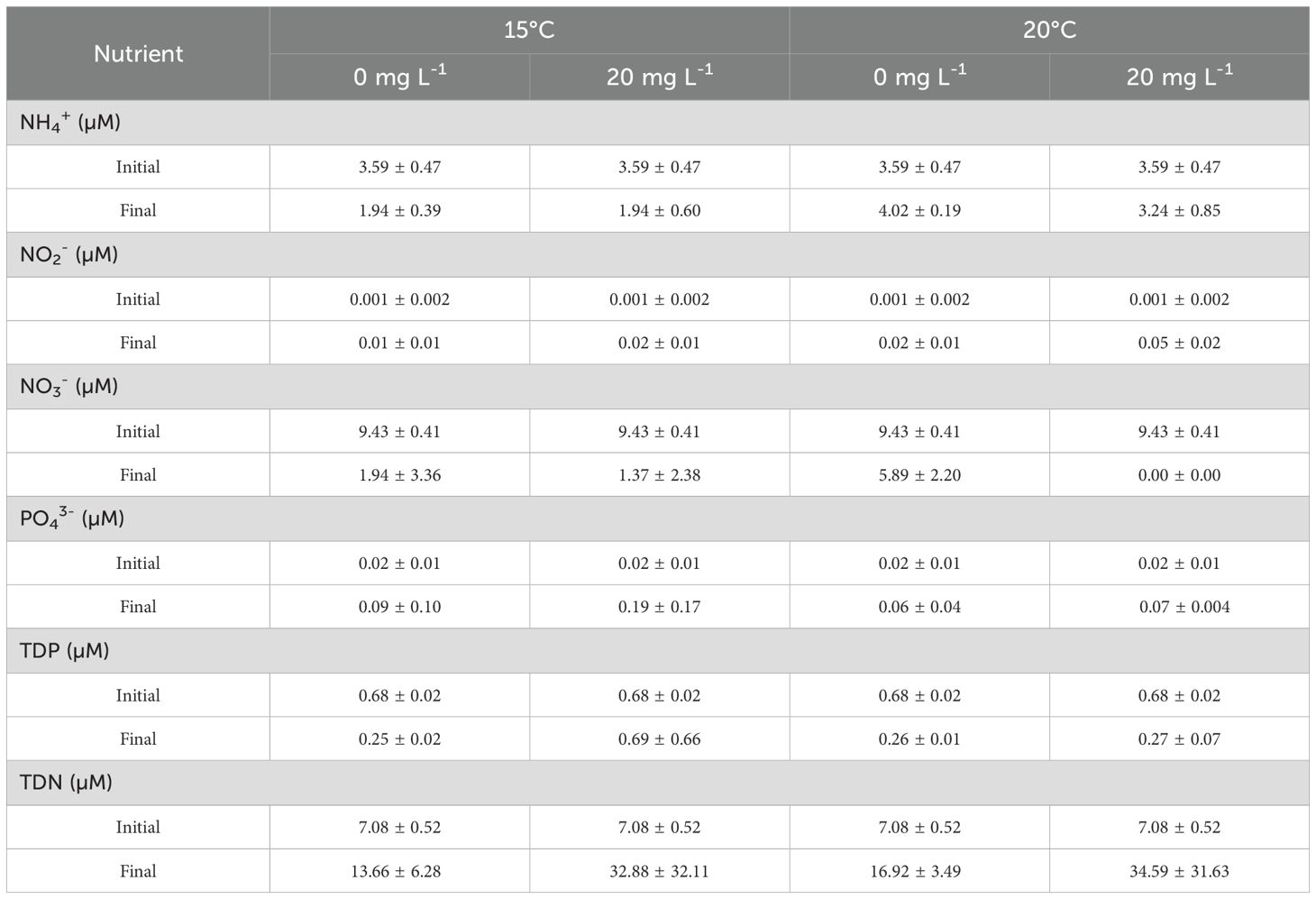
Table 2. Average concentrations (± SD, n=3) of ammonium (NH4+), nitrite (NO2-), nitrate (NO3-), phosphate (PO43-), total dissolved phosphorus (TDP) and nitrogen (TDN) in the experiment of sunscreen addition under different temperatures at the first and final days of the incubations.
3.2 Variations in primary productivity and respiration of Posidonia oceanica
Net primary production rates of P. oceanica were promoted by sunscreen under ambient temperature (3.53 ± 3.16 mg O2 g−1 dw h−1; Figure 3), compared to both temperature treatments without sunscreen exposure (0.91 ± 1.01 and 1.47 ± 0.62 mg O2 g−1 dw h−1 at 15 and 20°C, respectively). Respiration rates were relatively higher (p>0.05) in cut-leaf segments from both temperature treatments at 0 mg L-1 of sunscreen (-1.82 ± 1.45 and -2.09 ± 0.70 mg O2 g−1 dw h−1 under 15 and 20°C, respectively) than those at 20 mg L-1 of sunscreen (-0.75 ± 0.46 and -1.35 ± 0.84 mg O2 g−1 dw h−1 under 15 and 20°C, respectively). Differences in the gross primary productivity of P. oceanica were not statistically significant (p>0.05), although the lowest and highest values corresponded to both ambient temperature treatments (2.88 ± 1.32 and 4.03 ± 3.03 mg O2 g−1 dw h−1at 0 and 20 mg L-1 of sunscreen, respectively).
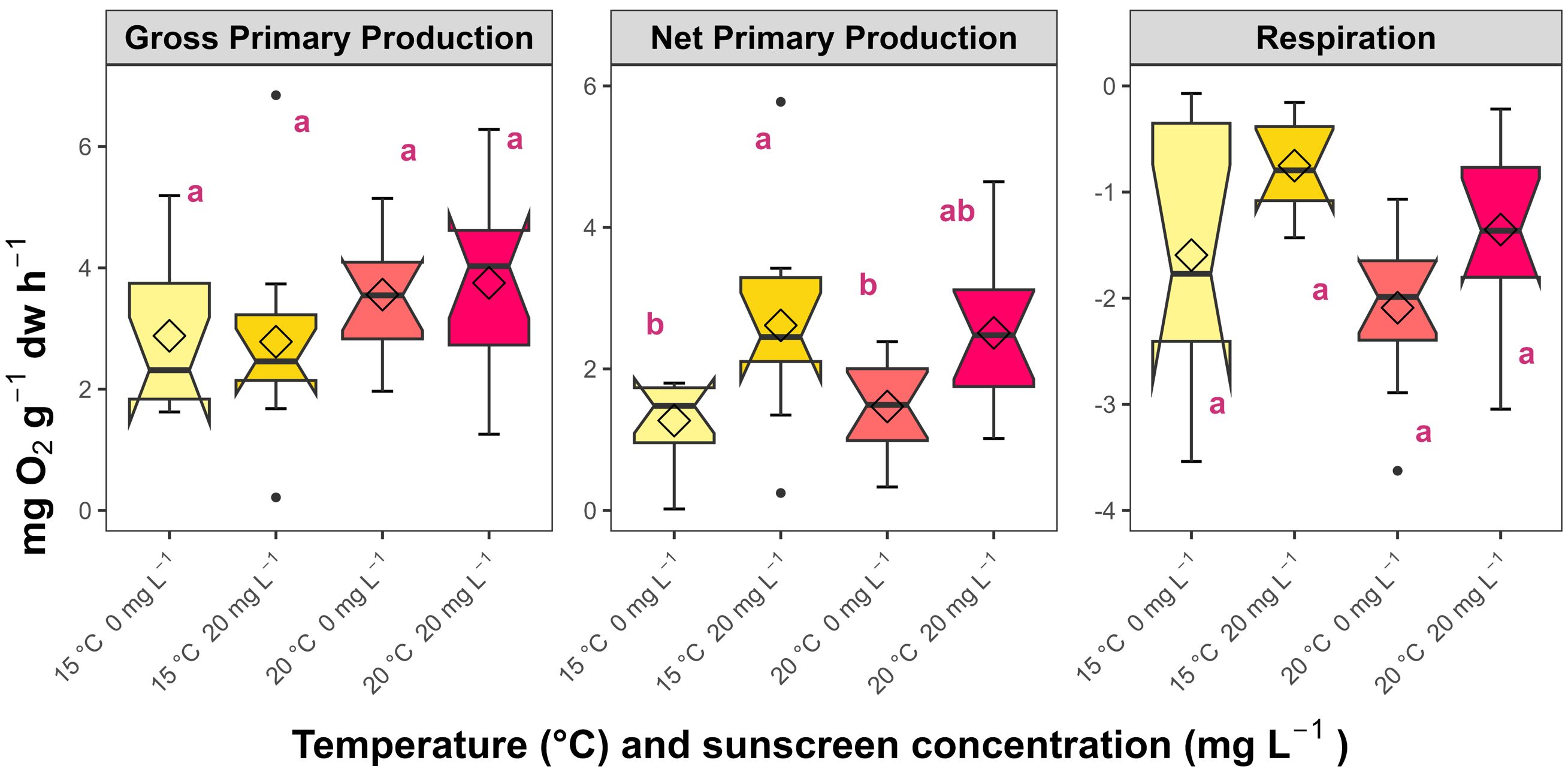
Figure 3. Average gross primary productivity, net primary productivity, and respiration of Posidonia oceanica in the treatments (n=6). Error bars represent ± standard deviation, while notches correspond with confidence intervals around medians and rhombi are mean values. Different letters reflect significant differences (p<0.05) among treatments (Tukey test).
3.3 Changes in leaf chlorophyll concentrations of Posidonia oceanica
Sunscreen exposure combined with elevated temperature induced a ~50% decrease in the total chlorophyll of P. oceanica (p<0.001; Figure 4), including chlorophyll a and b, with an average of 352.02 ± 82.31 µg g-1 ww, from 772.53 ± 281.36 µg g-1 ww under elevated temperature alone and 627.33 ± 199.60 µg g-1 ww at ambient temperature and 20 mg L-1 of sunscreen addition. Chlorophyll contents in the ambient temperature treatment without sunscreen (398.45 ± 165.28 µg g-1 ww) only differed (p<0.001) from those under elevated temperature alone, that overall exhibited greater chlorophyll production.
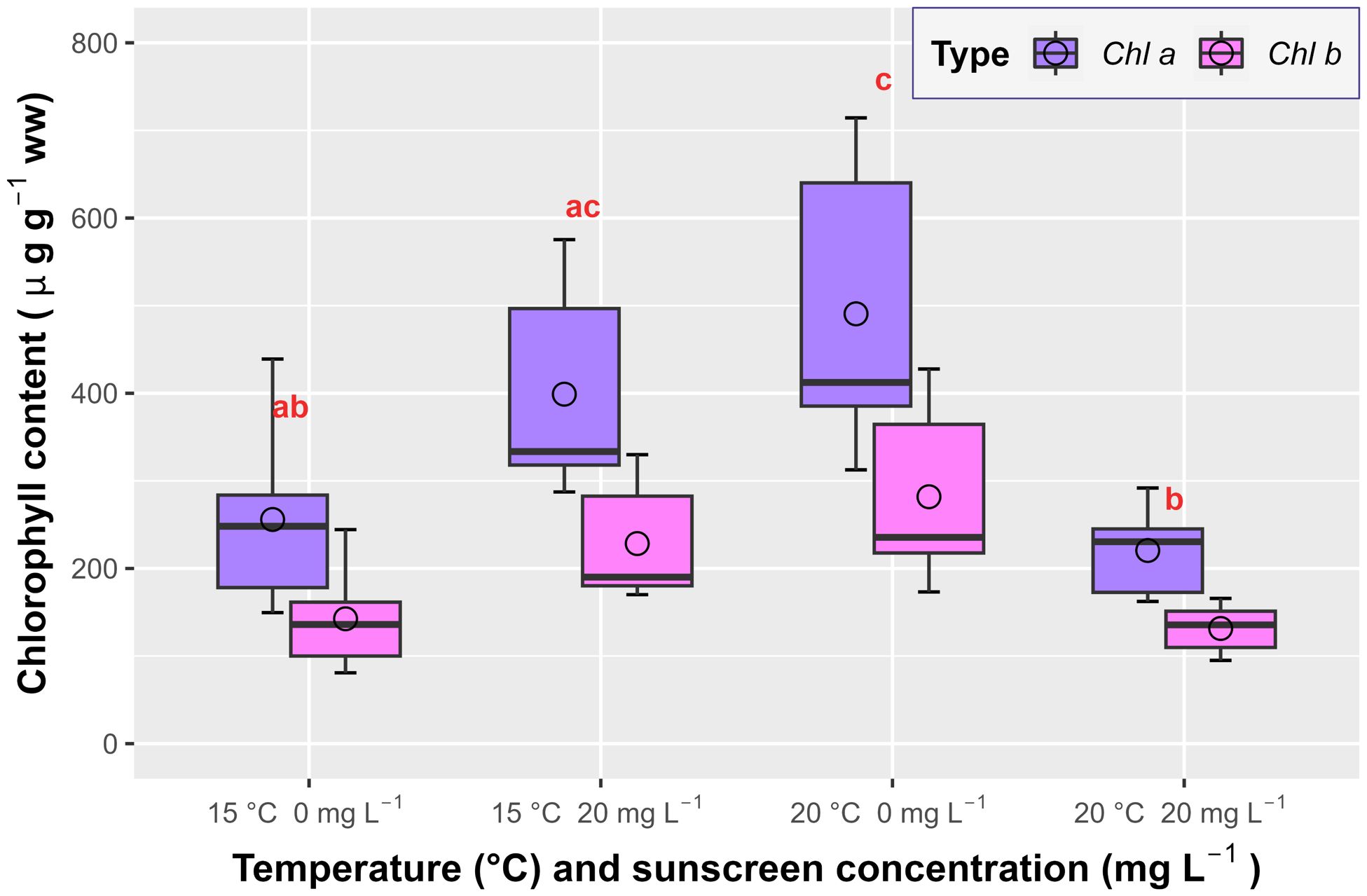
Figure 4. Average leaf chlorophyll a and b concentrations of Posidonia oceanica in the treatments (n=6). Error bars represent ± standard deviation and circles correspond with mean values. Different letters reflect significant differences (p<0.001) among treatments (Tukey test).
3.4 Responses of alkaline phosphatase activity in Posidonia oceanica tissues
The APA associated with P. oceanica young leaves and roots responded differently to the treatments (p<0.01). Under elevated temperature, sunscreen exposure seemed to inhibit the activity of the enzyme in the young leaves (Figure 5), with a 50% decrease from 59.66 ± 23.43 µM MUF g-1 dw h-1 without sunscreen, to 28.07 ± 14.28 µM MUF g-1 dw h-1. For the roots, APA rates were enhanced by the interaction of sunscreen addition with elevated temperature, showing increased rates by four-fold in this treatment (10.42 ± 9.23 µM MUF g-1 dw h-1) in comparison to those at ambient temperature and 0 mg L-1 of sunscreen (2.61 ± 1.31 µM MUF g-1 dw h-1). In general, the highest rates (p<0.001) per tissue corresponded to the young leaves (31.63 ± 20.69 µM MUF g-1 dw h-1), followed by the roots and old leaves (6.20 ± 6.74 and 3.25 ± 2.31 µM MUF g-1 dw h-1, respectively), and the lowest were evidenced in the rhizomes (1.19 ± 0.76 µM MUF g-1 dw h-1).
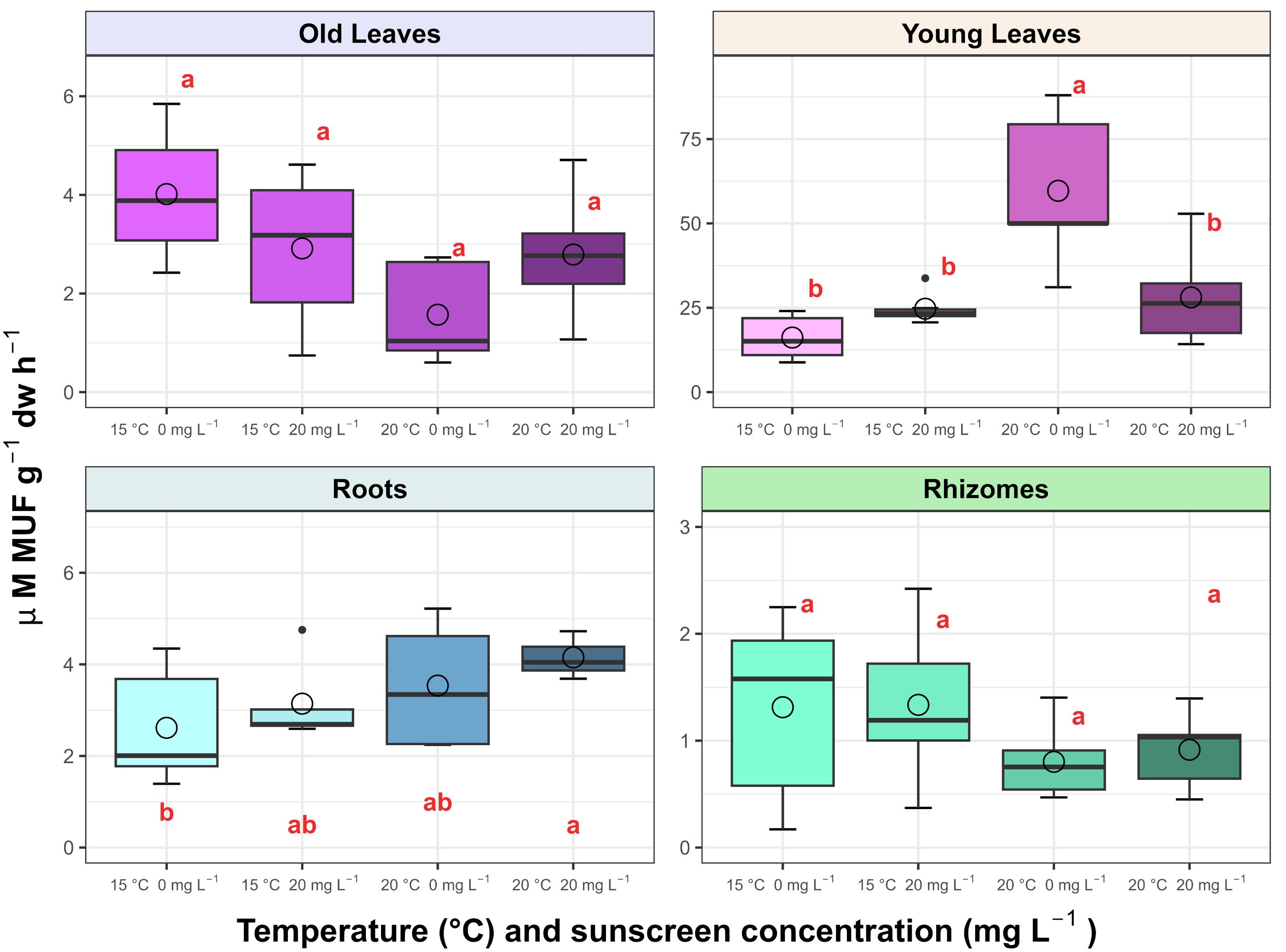
Figure 5. Average alkaline phosphatase activity (APA) associated with Posidonia oceanica leaves, roots and rhizomes in the treatments (n=6). Error bars represent ± standard deviation and circles correspond with mean values. Different letters reflect significant differences (p<0.01) among treatments (Tukey test).
3.5 Biochemical indicators of oxidative stress in Posidonia oceanica
Greater antioxidant defenses were induced in P. oceanica leaves by the combined treatments of sunscreen addition and elevated temperature (p<0.05; Figure 6). Catalase rates were incremented in old and young leaves under this treatment (7207 ± 3991 and 4503 ± 2699 mK mg-1 protein, respectively) in comparison to those at ambient temperature without sunscreen exposure (1383 ± 1346 and 546 ± 490 mK mg-1 protein, respectively). Comparing the elevated temperature treatments only, polyphenols concentrations in young leaves increased by ~100% with the addition of sunscreen, from 8.15 ± 1.57 to 16.16 ± 1.33 mg tyrosine mg-1 protein. Sunscreen exposure at ambient temperature promoted higher ROS production in young leaves (227.81 ± 58.68 a.u. mg-1 protein) than in both temperature conditions without sunscreen (48.73 ± 36.75 and 39.32 ± 21.93 a.u. mg-1 protein at 15 and 20°C, respectively).
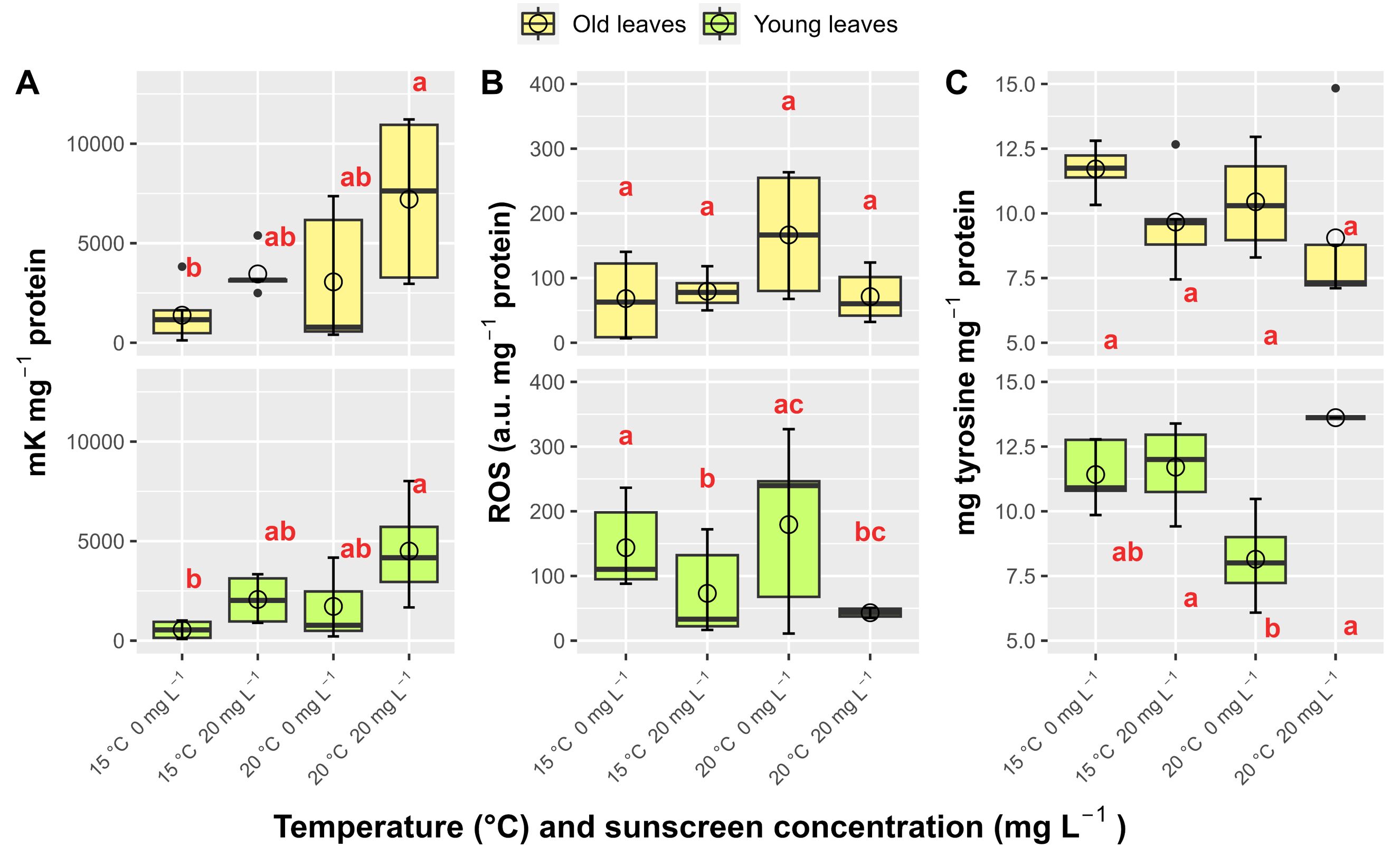
Figure 6. Average catalase activity (A), reactive oxygen species production-ROS (B) and polyphenols content (C) in leaves of Posidonia oceanica subjected to different treatments (n=6). Error bars represent ± standard deviation and circles correspond with mean values. Different letters reflect significant differences (p<0.05) among treatments (Tukey test).
3.6 Rates of N2 fixation associated with Posidonia oceanica tissues
The N2 fixation activity associated with P. oceanica was affected by the treatments only in the rhizomes analyzed (p<0.05; Figure 7), with a more than 50% inhibition in rates at combined sunscreen addition with elevated temperature (0.0063 ± 0.0036 nmol N2 fixed g-1 dw h-1) compared to those subjected to the warming treatment only (0.0170 ± 0.0091 nmol N2 fixed g-1 dw h-1). Activity rates were greater (p<0.05) in the roots and old leaves (0.55 ± 1.01 and 0.29 ± 0.06 nmol N2 fixed g-1 dw h-1, respectively), followed by the sterilized roots (0.19 ± 0.10 nmol N2 fixed g-1 dw h-1), and lower in the rhizomes (0.01 ± 0.01 nmol N2 fixed g-1 dw h-1).
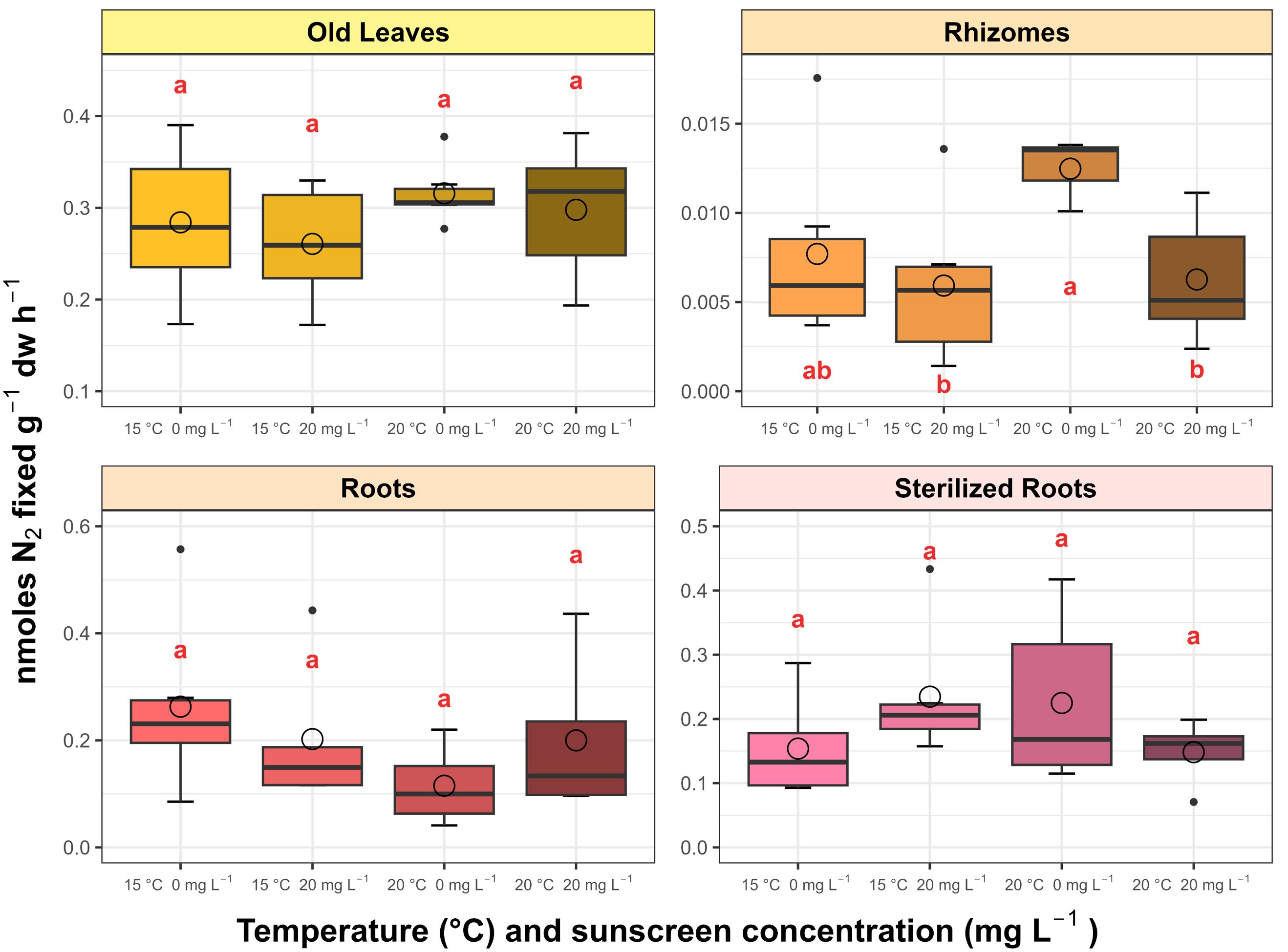
Figure 7. Average N2 fixation rates associated with Posidonia oceanica leaves, roots and rhizomes incubated in the treatments (n=6). Error bars represent ± standard deviation and circles correspond with mean values. Different letters reflect significant differences (p<0.05) among treatments (Tukey test).
4 Discussion
4.1 Effects of nutrient release from sunscreens and temperature on primary productivity
Sunscreen exposure alone (i.e., without increment of temperature) promoted the net primary productivity of P. oceanica, while the gross productivity and respiration rates did not respond to any of the treatments. Our past research (García-Márquez et al., 2023b) evidenced similar results, with enhanced gross and net rates under 35 and 45 mg L-1 of the same sunscreen mixture, rich in inorganic nutrients, such as NH4+, PO43- and NO2-, that may be assimilated by the seagrasses to sustain the photosynthesis process. Nutrient analyses in our study show higher (p>0.05) final TDN concentrations in the seawater of aquaria subjected to sunscreen addition under both temperature treatments. Sources of nitrogen contribute significantly to the high productivity of seagrasses (Agawin et al., 2017), thus, it can be expected for the net primary production rates, that directly measure the oxygen evolution from photosynthesis, to increase with nutrient inputs from the environment. Other studies (Tovar-Sánchez et al., 2013; Rodríguez-Romero et al., 2019) also consider sunscreen application from recreational activities in coastal areas a relevant additional source of nutrients in nearshore waters, with foreseeable implications for primary production and phytoplankton growth in oligotrophic environments, as the Mediterranean Sea (Vaulot et al., 1996). On the other hand, the differences detected in the pH measurements between the temperature conditions tested, could be related with the gross primary productivity evidenced in these respective treatments. The gross primary production rates of P. oceanica were relatively higher (p>0.05) under elevated temperature in both sunscreen treatments, possibly suggesting that the more alkaline conditions (i.e., higher pH) evidenced in these aquaria reflect the buffering effect promoted by the seagrasses through photosynthetic activity, which reduces the CO2 concentration that is being metabolically consumed (Invers et al., 2001; Hendriks et al., 2013; García-Márquez et al., 2022). A consistent linear increase can be observed in the gross photosynthesis of different seagrass species, including P.oceanica, with increased temperature below the point where thermal damage may occur (Drew, 1979; Masini and Manning, 1997; Agawin et al., 2021). Therefore, elevated temperature might have stimulated the gross primary production rates of P. oceanica, consequently modifying the seawater pH through enhanced CO2 uptake rates for photosynthesis.
4.2 Interaction of sunscreen and temperature in the synthesis of photosynthetic pigments
Combined sunscreen exposure and increased temperature seemed to cause leaf chlorophyll concentrations in P. oceanica to drop, while the elevated temperature treatment induced chlorophyll production without sunscreen addition. One of the well-known effects of sunscreen components, particularly UV filters, is the inhibition of chlorophyll production in marine photosynthetic organisms (Liu et al., 2019; Zhong et al., 2019; Baharlooeian and Haq, 2020; Thorel et al., 2020; Xing et al., 2022; García-Márquez et al., 2023a; García-Márquez et al., 2023b; García-Márquez et al., 2024) due to degradation of different cellular structures, including the chloroplast ribosomes/thylakoid membranes and the electron transport machinery, by the excessive accumulation of ROS generated in the system with the presence of toxic chemicals. Although the addition of the commercial sunscreen mixture alone at 20 mg L-1 did not affect the pigment biosynthesis, contrary to our previous results obtained when experimenting with a higher concentration (45 mg L-1; García-Márquez et al., 2023b), the incorporation of the elevated temperature factor in the incubations may have intensified the toxicity of the sunscreen components. Therefore, based on the current and previous investigation, the concentration threshold from 20 to 35 mg L-1 of commercial sunscreens seem less impactful on the chlorophyll production of this seagrass species. Increased temperatures may also be responsible for reductions in the leaf chlorophyll content of P. oceanica, as a general side effect of induced leaf senescence (Drew, 1978) or due to cellular stress (Rotini et al., 2013; Repolho et al., 2017). However, chlorophyll concentrations responded positively to elevated temperature alone, possibly because it is below the thermal tolerance threshold of this seagrass species (i.e., 11.5 to 26°C; Olsen et al., 2012; Pazzaglia et al., 2020) and, thus, temperature may have accelerated metabolic rates in P. oceanica, as it is reported to occur during winter and spring months (Pirc, 1986; Agawin et al., 2021).
4.3 Regulation of alkaline phosphatase activity under stressing conditions
An upregulation of the APA in young leaves and roots of P. oceanica was evidenced under different treatments, with the first responding to increased temperature only and the latter to the interaction between sunscreen addition and elevated temperature. Past studies have shown that rising temperatures benefit the activity of this enzyme in P. oceanica tissues until a specific threshold (i.e.,24°C; Invers et al., 1995; García-Márquez et al., 2021, García-Márquez et al., 2022), after which an inhibitory effect may be observed. The temperature treatment falls within the optimal range for the functioning of the alkaline phosphatases in the seagrass, thus, supporting previous reports on the promoting influence that temperature can exert on this enzyme. Sunscreen components may also induce the APA not only in seagrasses (García-Márquez et al., 2023a, García-Márquez et al., 2023b), but also in other marine taxa (Danovaro et al., 2008; Huang et al., 2018), acting up as a defense mechanism to cope with dysfunctions provoked by toxic substances and generally associated with biochemical indicators of oxidative stress. The ROS generation was relatively higher (p>0.05) in the young leaves subjected to the elevated temperature treatment without sunscreen exposure and may also partially reflect the lower tolerance to increased temperature from this part of the plant that is still under growth and development. The roots analyzed were the only sensitive tissue, in terms of APA, to the combined temperature and sunscreen treatment, suggesting potential cellular damage occurring in this tissue due to the interaction of both factors. Stressing conditions in seagrass ecosystems (e.g. chemical pollution, ocean warming or decreases in irradiance) may encourage phosphorus (P) deficiency in these plants (Ferrat et al., 2003; García-Márquez et al., 2023a, García-Márquez et al., 2023b, García-Márquez et al., 2024), enhancing the activity of the alkaline phosphatases, which sequester and hydrolyze organic P (i.e., phosphomonoesters) that release inorganic P into the environment and increase its bioavailability (Koch et al., 2009). The activity of this enzyme, tightly regulated by P metabolism, plays an essential role in providing energy for stressed cells and can constitute a physiological response to damages in cell membranes and tissues (Agrahari et al., 2007; Saha and Kaviraj, 2009; Banaee, 2020).
4.4 Activation of antioxidant defenses under combined environmental stressors
In general, the oxidative stress indicators evaluated in P. oceanica could suggest the vulnerability of the species to both elevated sunscreen concentrations and seawater warming during spring, with increased catalase activity in old and young leaves, as well as polyphenols production in young leaves. An oxidative stress situation normally occurs when ROS is excessively generated in an organism, causing malfunctioning and deterioration of cellular structures (Vale et al., 2016; Khan et al., 2017), and marine organisms must rely on different types of antioxidant systems, primarily centered around non-enzymatic and enzymatic antioxidants (Núñez-Pons et al., 2018). Organic UV filters in sunscreens may produce ROS when introduced in the marine environment (Varrella et al., 2022), and due to their high lipophilicity and stability, their associated harmful effects to the marine biota could be aggravated by ocean warming (Fastelli and Renzi, 2019; Wijgerde et al., 2020). Heat stress alone may also result in ROS formation and strong oxidative damage in seagrasses, inducing changes in cell membranes and photosynthetic pigments (Campbell et al., 2006; Chalanika De Silva and Asaeda, 2017). In our experiments, P. oceanica plants incubated under sunscreen exposure and elevated temperature could have resorted to activating protection mechanisms against oxidative stress, since survival under these conditions depend on the equilibrium between production and quenching of ROS by detoxification (Chalanika De Silva and Asaeda, 2017). Moreover, under unfavorable conditions, intracellular ROS levels not only can damage important biomolecules in an organism, but their overproduction beyond equilibrium can lead to the death of cells induced by programmed cell death (Shah et al., 2001; Gill and Tuteja, 2010). Catalase is an enzymatic antioxidant that removes H2O2 catalytically under saturating levels (Gunawan et al., 2013; Halliwell and Gutteridge, 2015), thus, the enhancement of its activity may indicate the generation of high levels of this oxyradical in P. oceanica due to the sunscreen and temperature treatment. The seagrasses in our study can also have produced more polyphenols to overcome these conditions, since phenolic compounds activate antioxidant or ROS scavenging properties under oxidative stress (Milito et al., 2021; Martins and Marto, 2023).
4.5 Inhibition of N2 fixation activity in rhizome epiphytes of P. oceanica
The N2 fixation of rhizome epiphytes in P. oceanica was reduced under sunscreen addition at elevated temperature. Sunscreen components can exert an inhibitory effect on the nitrogenase activity in legumes rhizosphere (Fan et al., 2014) and P. oceanica leaves and rhizomes (García-Márquez et al., 2023a, García-Márquez et al., 2023b, García-Márquez et al., 2024), which is possibly attributed to damages induced by toxic ROS in the bacteroid membranes associated with the N2-fixing apparatus, resulting in less efficient rates of N2 fixation. On the contrary, temperature is known to favor this process, directly controlling the functioning of the nitrogenase enzyme (Brauer et al., 2013; Agawin et al., 2017; Garcias-Bonet et al., 2019; García-Márquez et al., 2021, García-Márquez et al., 2022), which could be evidenced in our study with the increased rates recorded at elevated temperature only. The N2 fixation activity was relatively lower with sunscreen exposure at ambient temperature, while the decrease potentially induced by the sunscreen mixture was statistically significant under elevated temperature. This response also supports the previously discussed results, in which the interaction between sunscreen and temperature likely encouraged oxidative damage in cellular components of the seagrass. The disruption of the N2-fixing activity of rhizome epiphytic microorganisms could have implications in the nitrogen exchange required to sustain the growth and high productivity of P. oceanica, since the nitrogenase activity in the rhizosphere tends to be greater during the most active growth period of seagrasses (McGlathery et al., 1998).
5 Conclusions
A synergistic interaction resulted from the combination of sunscreen exposure at 20 mg L-1 and increased seawater temperature (i.e., plus 5°C) in spring, with overall negative responses for the physiology of P. oceanica. Sensitive parameters that indirectly reflected the stressed conditions of the seagrasses under the sunscreen and temperature treatment, apart from the incremented oxidative stress indicators, were the synthesis of chlorophyll pigments and N2 fixation associated with the rhizomes, which declined in these conditions. These findings could provide early evidence of potentially harmful dynamics that may be occurring in P. oceanica meadows, and aquatic ecosystems overall. The additive impacts of continued rising sea temperatures under greenhouse warming and coastal sunscreen pollution can be degrading the environmental quality of seagrass ecosystems and possibly taking part in their deteriorating health and eventual mortality. However, the conclusions driven are tentative and must be further discussed with discretion given the limitations of our experimental setup, which cannot recreate in every aspect the complexity of the natural environment. Responses from the plants were measured only at one point during a relatively short-term period, leaving possibilities open for different outcomes in the analyses performed (e.g. resilience, recovery or mortality of the seagrasses). Moreover, it would be appropriate for future research to determine which components in commercial sunscreens are inducing these negative consequences under elevated temperature, as well as other factors that could be aggravating their toxic effects in the marine environment.
Data availability statement
The raw data supporting the conclusions of this article will be made available by the authors, without undue reservation.
Author contributions
MG: Writing – review & editing, Writing – original draft, Visualization, Validation, Methodology, Investigation, Formal analysis, Data curation, Conceptualization. SM: Writing – review & editing, Funding acquisition, Visualization, Validation, Methodology, Formal analysis, Data curation, Conceptualization. CC: Writing – review & editing, Visualization, Validation, Methodology, Formal analysis, Data curation, Conceptualization. NA: Writing – review & editing, Visualization, Validation, Supervision, Resources, Project administration, Methodology, Investigation, Funding acquisition, Conceptualization.
Funding
The author(s) declare financial support was received for the research, authorship, and/or publication of this article. MG-M is recipient of the CAIB (2021) predoctoral grant (BOIB No. 103) funded by the Govern de les Illes Balears - Conselleria de Fons Europeus, Universitat i Cultura. This work has been partially sponsored and promoted by the Comunitat Autonoma de les Illes Balears through the Servei de Recerca i Desenvolupament and the Conselleria de Fons Europeus, Universitat i Cultura and by the European Union-Next Generation EU (BIO/001). Nevertheless, the views and opinions expressed are solely those of the authors, and do not necessarily reflect those of the European Union or the European Commission. Neither the European Union nor the European Commission are to be held responsible”.
Acknowledgments
We acknowledge the collaboration of José Carlos Rodríguez-Castañeda in the execution of the experimental setup and laboratory analyses. We also thank the Scientific Technical Service of the University of the Balearic Islands for their support in the gas chromatography (Maria Trinidad Garcia Barceló) and spectrofluorimetric (Biel Martorell Crespí) analyses.
Conflict of interest
The authors declare that the research was conducted in the absence of any commercial or financial relationships that could be construed as a potential conflict of interest.
The author(s) declared that they were an editorial board member of Frontiers, at the time of submission. This had no impact on the peer review process and the final decision.
Publisher’s note
All claims expressed in this article are solely those of the authors and do not necessarily represent those of their affiliated organizations, or those of the publisher, the editors and the reviewers. Any product that may be evaluated in this article, or claim that may be made by its manufacturer, is not guaranteed or endorsed by the publisher.
References
Aebi H. (1984). Catalase in vitro. Methods Enzymol. 105, 121–126. doi: 10.1016/S0076-6879(84)05016-3
Agawin N. S. R., Benavides M., Busquets A., Ferriol P., Stal L. J., Arístegui J. (2014). Dominance of unicellular cyanobacteria in the diazotrophic community in the Atlantic Ocean. Limnol. Oceanogr. 59, 623–637. doi: 10.4319/lo.2014.59.2.0623
Agawin N. S. R., Duarte C. M., Fortes M. D. (1996). Nutrient limitation of Philippine seagrasses (Cape Bolinao, NW Philippines): in situ experimental evidence. Mar. Ecol. Prog. Ser. 138, 233–243. doi: 10.3354/meps138233
Agawin N. S. R., Ferriol P., Sintes E., Moyà G. (2017). Temporal and spatial variability of in situ nitrogen fixation activities associated with the Mediterranean seagrass Posidonia oceanica meadows. Limnol. Oceanogr. 62, 2575–2592. doi: 10.1002/lno.10591
Agawin N. S. R., Gil Atorrasagasti M. G., Frank Comas A., Fernández-Juárez V., López-Alforja X., Hendriks I. E. (2021). Response of the seagrass Posidonia oceanica and its associated N2 fixers to high business-as-usual climate change scenario in winter. Limnol. Oceanogr. 1, 1–14. doi: 10.1002/lno.11758
Agawin N. S. R., Sunyer-Caldú A., Díaz-Cruz M. S., Frank-Comas A., García-Márquez M. G., Tovar-Sánchez A. (2022). Mediterranean seagrass Posidonia oceanica accumulates sunscreen UV filters. Mar. pollut. Bull. 176, 113417. doi: 10.1016/j.marpolbul.2022.113417
Agrahari S., Pandey K. C., Gopal K. (2007). Biochemical alteration induced by monocrotophos in the blood plasma of fish, Channa punctatus (Bloch). Pestic Biochem. Physiol. 88, 268–272. doi: 10.1016/j.pestbp.2007.01.001
Alcoverro T., Manzanera M., Romero J. (1998). Seasonal and age-dependent variability of Posidonia oceanica (L.) Delile photosynthetic parameters. J. Exp. Mar. Biol. Ecol. 230, 1–13. doi: 10.1016/S0022-0981(98)00022-7
Aoki L. R., McGlathery K. J., Wiberg P. L., Oreska M. P. J., Berger A. C., Berg P., et al. (2021). Seagrass recovery following marine heat wave influences sediment carbon stocks. Front. Mar. Sci. 7. doi: 10.3389/fmars.2020.576784
Araújo C. V. M., Rodríguez-Romero A., Fernández M., Sparaventi E., Márquez Medina M., Tovar-Sánchez A. (2020). Repellency and mortality effects of sunscreens on the shrimp Palaemon varians: toxicity dependent on exposure method. Chemosphere 257, 127190. doi: 10.1016/j.chemosphere.2020.127190
Baharlooeian M., Haq M. A. B. (2020). Toxic effect of nano and bulk TiO2 on growth, chlorophyll a content and oxidative stress of marine diatom Chaetoceros muelleri. Nipp. J. Environ. Sci. 1, 1001. doi: 10.46266/njes.1001
Bai Z., Wang M. (2020). Warmer temperature increases mercury toxicity in a marine copepod. Ecotoxicol Environ. Saf. 201, 110861. doi: 10.1016/j.ecoenv.2020.110861
Banaee M. (2020). Alkaline phosphatase activity as a biochemical biomarker in aqua-toxicological studies. Int. J. Aquat. Biol. 8, 143–147. doi: 10.22034/ijab.v8i2.880
Berry J., Bjorkman O. (1980). Photosynthetic response and adaptation to temperature in higher plants. Annu. Rev. Plant Physiol. 31, 491–543. doi: 10.1146/annurev.pp.31.060180.002423
Blitz J. B., Norton S. A. (2008). Possible environmental effects of sunscreen run-off. J. Am. Acad. Dermatol. 59, 898. doi: 10.1016/j.jaad.2008.06.013
Bordalo D., Cuccaro A., Meucci V., De Marchi L., Soares A. M. V. M., Pretti C., et al. (2023). Will warmer summers increase the impact of UV filters on marine bivalves? Sci. Total Environ. 872, 162108. doi: 10.1016/j.scitotenv.2023.162108
Brauer V. S., Stomp M., Rosso C., van Beusekom S. A. M., Emmerich B., Stal L. J., et al. (2013). Low temperature delays timing and enhances the cost of nitrogen fixation in the unicellular cyanobacterium Cyanothece. ISME J. 7, 2105–2115. doi: 10.1038/ismej.2013.103
Brown B. E. (2000). The significance of pollution in eliciting the “’bleaching’” response in symbiotic cnidarians. Int. J. Environ. pollut. 13, 392. doi: 10.1504/IJEP.2000.002328
Cadena-Aizaga M. I., Montesdeoca-Esponda S., Torres-Padrón M. E., Sosa-Ferrera Z., Santana-Rodríguez J. J. (2020). Organic UV filters in marine environments: An update of analytical methodologies, occurrence and distribution. Trends Environ. Analytical Chem. 25, e00079. doi: 10.1016/j.teac.2019.e00079
Campbell S. J., McKenzie L. J., Kerville S. P. (2006). Photosynthetic responses of seven tropical seagrasses to elevated seawater temperature. J. Exp. Mar. Biol. Ecol. 330, 455–468. doi: 10.1016/j.jembe.2005.09.017
Capone D. G. (1993). “Determination of nitrogenase activity in aquatic samples using the acetylene reduction procedure,” in Handbook of methods in aquatic microbial ecology. Eds. Kemp P., Sherr B., Sherr E., Cole J. (Lewis Publishers, Boca Raton, FL), 621–631.
Chalanika De Silva H. C., Asaeda T. (2017). Effects of heat stress on growth, photosynthetic pigments, oxidative damage and competitive capacity of three submerged macrophytes. J. Plant Interact. 12, 228–236. doi: 10.1080/17429145.2017.1322153
Chefaoui R. M., Duarte C. M., Serrão E. A. (2018). Dramatic loss of seagrass habitat under projected climate change in the Mediterranean Sea. Glob Chang Biol. 24, 4919–4928. doi: 10.1111/gcb.14401
Cocci P., Mosconi G., Palermo F. A. (2020). Sunscreen active ingredients in loggerhead turtles (Caretta caretta) and their relation to molecular markers of inflammation, oxidative stress and hormonal activity in wild populations. Mar. pollut. Bull. 153, 111012. doi: 10.1016/j.marpolbul.2020.111012
Coombs J. T., Franco C. M. M. (2003). Isolation and identification of actinobacteria from surface-sterilized wheat roots. Appl. Environ. Microbiol. 69, 5603–5608. doi: 10.1128/AEM.69.9.5603
Corinaldesi C., Damiani E., Marcellini F., Falugi C., Tiano L., Brugè F., et al. (2017). Sunscreen products impair the early developmental stages of the sea urchin Paracentrotus lividus. Sci. Rep. 7, 7815. doi: 10.1038/s41598-017-08013-x
Costa S., Coppola F., Pretti C., Intorre L., Meucci V., Soares A. M. V. M., et al. (2020). Biochemical and physiological responses of two clam species to Triclosan combined with climate change scenario. Sci. Total Environ. 724, 138143. doi: 10.1016/j.scitotenv.2020.138143
Cuccaro A., De Marchi L., Oliva M., Monni G., Miragliotta V., Fumagalli G., et al. (2022). The influence of salinity on the toxicity of chemical UV-filters to sperms of the free-spawning mussel Mytilus galloprovincialis (Lamark 1819). Aquat. Toxicol. 250, 106263. doi: 10.1016/j.aquatox.2022.106263
Cuccaro A., De Marchi L., Oliva M., Sanches M. V., Freitas R., Casu V., et al. (2021). Sperm quality assessment in Ficopomatus enigmaticus (Fauvel 1923): Effects of selected organic and inorganic chemicals across salinity levels. Ecotoxicol Environ. Saf. 207, 111219. doi: 10.1016/j.ecoenv.2020.111219
Danovaro R., Bongiorni L., Corinaldesi C., Giovannelli D., Damiani E., Astolfi P., et al. (2008). Sunscreens cause coral bleaching by promoting viral infections. Environ. Health Perspect. 116, 441–447. doi: 10.1289/ehp.10966
Darmaraki S., Somot S., Sevault F., Nabat P. (2019a). Past variability of Mediterranean sea marine heatwaves. Geophys Res. Lett. 46, 9813–9823. doi: 10.1029/2019GL082933
Darmaraki S., Somot S., Sevault F., Nabat P., Cabos Narvaez W. D., Cavicchia L., et al. (2019b). Future evolution of marine heatwaves in the Mediterranean Sea. Clim Dyn 53, 1371–1392. doi: 10.1007/s00382-019-04661-z
Díaz-Almela E., Marbà N., Duarte C. M. (2007). Consequences of Mediterranean warming events in seagrass (Posidonia oceanica) flowering records. Glob Chang Biol. 13, 224–235. doi: 10.1111/j.1365–2486.2006.01260.x
Díaz-Almela E., Marbà N., Martínez R., Santiago R., Duarte C. M. (2009). Seasonal dynamics of Posidonia oceanica in Magalluf Bay (Mallorca, Spain): Temperature effects on seagrass mortality. Limnol Oceanogr 54, 2170–2182. doi: 10.4319/lo.2009.54.6.2170
Díaz-Cruz M. S., Barceló D. (Eds.) (2015). Personal Care Products in the Aquatic Environment (Cham: Springer International Publishing). doi: 10.1007/978–3-319–18809-6
Drew E. A. (1978). Factors affecting photosynthesis and its seasonal variation in the seagrasses Cymodocea nodosa (Ucria) Aschers, and Posidonia oceanica (L.) Delile in the mediterranean. J. Exp. Mar. Biol. Ecol. 31, 173–194. doi: 10.1016/0022-0981(78)90128-4
Drew E. A. (1979). Physiological aspects of primary production in seagrasses. Aquat Bot. 7, 139–150. doi: 10.1016/0304-3770(79)90018-4
Duarte C. M. (2002). The future of seagrass meadows. Environ. Conserv. 29, 192–206. doi: 10.1017/S0376892902000127
Duarte C. M., Dennison W. C., Orth R. J. W., Carruthers T. J. B. (2008). The charisma of coastal ecosystems: addressing the imbalance. Estuaries Coasts 31, 233–238. doi: 10.1007/s12237-008-9038-7
Duarte C. M., Middelburg J. J., Caraco N. (2005). Major role of marine vegetation on the oceanic carbon cycle. Biogeosciences 2, 1–8. doi: 10.5194/bg-2-1-2005
Fan R., Huang Y. C., Grusak M. A., Huang C. P., Sherrier D. J. (2014). Effects of nano-TiO2 on the agronomically-relevant Rhizobium–legume symbiosis. Sci. Total Environ. 466–467, 503–512. doi: 10.1016/j.scitotenv.2013.07.032
Fastelli P., Renzi M. (2019). Exposure of key marine species to sunscreens: Changing ecotoxicity as a possible indirect effect of global warming. Mar. pollut. Bull. 149, 110517. doi: 10.1016/j.marpolbul.2019.110517
Fel J. P., Lacherez C., Bensetra A., Mezzache S., Béraud E., Léonard M., et al. (2019). Photochemical response of the scleractinian coral Stylophora pistillata to some sunscreen ingredients. Coral Reefs 38, 109–122. doi: 10.1007/s00338-018-01759-4
Fernández-Juárez V., Bennasar-Figueras A., Tovar-Sanchez A., Agawin N. S. R. (2019). The role of iron in the P-acquisition mechanisms of the unicellular N2-fixing cyanobacteria Halothece sp., found in association with the Mediterranean seagrass Posidonia oceanica. Front. Microbiol. 10. doi: 10.3389/fmicb.2019.01903
Ferrat L., Pergent-Martini C., Roméo M. (2003). Assessment of the use of biomarkers in aquatic plants for the evaluation of environmental quality: application to seagrasses. Aquat. Toxicol. 65, 187–204. doi: 10.1016/S0166-445X(03)00133-4
Fivenson D., Sabzevari N., Qiblawi S., Blitz J., Norton B. B., Norton S. A. (2021). Sunscreens: UV filters to protect us: Part 2-Increasing awareness of UV filters and their potential toxicities to us and our environment. Int. J. Womens Dermatol. 7, 45–69. doi: 10.1016/j.ijwd.2020.08.008
Fourqurean J. W., Duarte C. M., Kennedy H., Marbà N., M. H., Mateo M. A., et al. (2017). Seagrass ecosystems as a globally significant carbon stock. Nat. Geosci. 5, 505–509. doi: 10.1038/ngeo1477
García-Márquez M. G., Fernández-Juárez V., Rodríguez-Castañeda J. C., Agawin N. S. R. (2022). Response of Posidonia oceanica (L.) delile and its associated N2 fixers to different combinations of temperature and light levels. Front. Mar. Sci. 8. doi: 10.3389/fmars.2021.757572
García-Márquez M. G., Fernández-Juárez V. F., Rodríguez-Castañeda J. C., Ruiz-Llobera I., Garriga Aguas Q., Agawin N. S. R. (2021). “Response of Posidonia oceanica (L.) Delile and their associated N2 fixers to different combinations of light levels and temperature,” in ASLO 2021 Virtual Meeting - Environmental Change. (Spain: Association for the Sciences of Limnology and Oceanography).
García-Márquez M. G., Rodríguez-Castañeda J. C., Agawin N. S. R. (2023a). “Effects of the sunscreen ultraviolet filter oxybenzone (benzophenone-3) on the seagrass Posidonia oceanica (L.) Delile,” in ASLO 2023 Aquatic Sciences Meeting(Palma de Mallorca, Spain: Association for the Sciences of Limnology and Oceanography).
García-Márquez M. G., Rodríguez-Castañeda J. C., Agawin N. S. R. (2023b). Sunscreen exposure interferes with physiological processes while inducing oxidative stress in seagrass Posidonia oceanica (L.) Delile. Mar. pollut. Bull. 187, 114507. doi: 10.1016/j.marpolbul.2022.114507
García-Márquez M. G., Rodríguez-Castañeda J. C., Agawin N. S. R. (2024). Effects of the sunscreen ultraviolet filter oxybenzone (benzophenone-3) on the seagrass Posidonia oceanica (L.) Delile and its associated N2 fixers. Sci. Total Environ. 918, 170751. doi: 10.1016/j.scitotenv.2024.170751
Garcias-Bonet N., Vaquer-Sunyer R., Duarte C. M., Marbà N. (2019). Warming effect on nitrogen fixation in Mediterranean macrophyte sediments. Biogeosciences 16, 167–175. doi: 10.5194/bg-16-167-2019
Gill S. S., Tuteja N. (2010). Reactive oxygen species and antioxidant machinery in abiotic stress tolerance in crop plants. Plant Physiol. Biochem. 48, 909–930. doi: 10.1016/j.plaphy.2010.08.016
Guerrero-Meseguer L., Marín A., Sanz-Lázaro C. (2017). Future heat waves due to climate change threaten the survival of Posidonia oceanica seedlings. Environ. pollut. 230, 40–45. doi: 10.1016/j.envpol.2017.06.039
Gunawan C., Sirimanoonphan A., Teoh W. Y., Marquis C. P., Amal R. (2013). Submicron and nano formulations of titanium dioxide and zinc oxide stimulate unique cellular toxicological responses in the green microalga Chlamydomonas reinhardtii. J. Hazard Mater 260, 984–992. doi: 10.1016/j.jhazmat.2013.06.067
Halliwell B., Gutteridge J. M. C. (2015). Free Radicals in Biology and Medicine. 5th Edn (New York, EEUU: Oxford University Press). doi: 10.1093/acprof:oso/9780198717478.001.0001
Hammer K., Borum J., Hasler-Sheetal H., Shields E., Sand-Jensen K., Moore K. (2018). High temperatures cause reduced growth, plant death and metabolic changes in eelgrass Zostera marina. Mar. Ecol. Prog. Ser. 604, 121–132. doi: 10.3354/meps12740
He T., Tsui M. M. P., Mayfield A. B., Liu P.-J., Chen T.-H., Wang L.-H., et al. (2023). Organic ultraviolet filter mixture promotes bleaching of reef corals upon the threat of elevated seawater temperature. Sci. Total Environ. 876, 162744. doi: 10.1016/j.scitotenv.2023.162744
Hendriks I. E., Olsen Y. S., Ramajo L., Basso L., Steckbauer A., Moore T. S., et al. (2013). Photosynthetic activity buffers ocean acidification in seagrass meadows. Biogeosci. Discuss. 10, 12313–12346. doi: 10.5194/bgd-10-12313-2013
Hobday A. J., Alexander L. V., Perkins S. E., Smale D. A., Straub S. C., Oliver E. C. J., et al. (2016). A hierarchical approach to defining marine heatwaves. Prog. Oceanogr 141, 227–238. doi: 10.1016/j.pocean.2015.12.014
Horstkotte B., Duarte C. M. (2012). “Análisis de amonio en aguas marinas y aguas dulces por fluorimetría,” in Expedición de circunnavegación Malaspina 2010. Cambio global y exploración de la biodiversidad del océano. Libro blanco de métodos y técnicas de trabajo oceanográfico. Ed. Moreno-Ostos E. (Consejo Superior de Investigaciones Científicas, Madrid, Spain), 123–132.
Huang X., Liu Y., Liu Z., Zhao Z., Dupont S., Wu F., et al. (2018). Impact of zinc oxide nanoparticles and ocean acidification on antioxidant responses of Mytilus coruscus. Chemosphere 196, 182–195. doi: 10.1016/j.chemosphere.2017.12.183
Invers O., Pérez M., Romero J. (1995). Alkaline phosphatase activity as a tool for assessing nutritional conditions in the seagrass Posidonia oceanica. Sci. Mar. 59, 41–47.
Invers O., Zimmerman R. C., Alberte R. S., Perez M., Romero J. (2001). Inorganic carbon sources for seagrass photosynthesis: an experimental evaluation of bicarbonate use in species inhabiting temperate waters. J. Exp. Mar. Biol. Ecol. 265, 203–217. doi: 10.1016/S0022-0981(01)00332-X
IPCC (2007). Climate change 2007: The Physical Science Basis. Eds. Solomon S., Qin D., Manning M., Chen Z., Marquis M., Averyt K. B., et al (Cambridge, United Kingdom and New York, NY, USA: Cambridge University Press).
IPCC (2014). Climate Change 2014: Impacts, Adaptation, and Vulnerability. Part A: Global and Sectoral Aspects. Contribution of Working Group II to the Fifth Assessment Report of the Intergovernmental Panel on Climate Change. Eds. Field C. B., Barros V. R., Dokken D. J., Mach K. J., Mastrandrea M. D., Bilir T. E., et al (Cambridge, United Kingdom and New York, NY, USA: Cambridge University Press).
IPCC (2019). “Summary for policymakers,” in IPCC Special Report on the Ocean and Cryosphere in a Changing Climate. Eds. Pörtner H.-O., Roberts D. C., Masson-Delmotte V., Zhai P., Tignor M., Poloczanska E., et al (Cambridge University Press, UK and New York, NY, USA), 3–35. doi: 10.1017/9781009157964.001
IPCC (2023). Climate Change 2022 – Impacts, Adaptation and Vulnerability. Contribution of Working Group II to the Sixth Assessment Report of the Intergovernmental Panel on Climate Change. Eds. Pörtner H.-O., Roberts D. C., Tignor M., Poloczanska E. S., Mintenbeck K., Alegría A., et al (Cambridge, UK and New York, NY, USA: Cambridge University Press). doi: 10.1017/9781009325844
Jackson J. B. C. (2008). Ecological extinction and evolution in the brave new ocean. Proc. Natl. Acad. Sci. 105, 11458–11465. doi: 10.1073/pnas.0802812105
Jensen B. B., Cox R. P. (1983). Direct measurements of steady-state kinetics of cyanobacterial N2 uptake by membrane-leak mass spectrometry and comparisons between nitrogen fixation and acetylene reduction. Appl. Environ. Microbiol. 45, 1331–1337. doi: 10.1128/aem.45.4.1331-1337.1983
Khan M. N., Mobin M., Abbas Z. K., AlMutairi K. A., Siddiqui Z. H. (2017). Role of nanomaterials in plants under challenging environments. Plant Physiol. Biochem. 110, 194–209. doi: 10.1016/j.plaphy.2016.05.038
Kibria G., Nugegoda D., Rose G., Haroon A. K. Y. (2021). Climate change impacts on pollutants mobilization and interactive effects of climate change and pollutants on toxicity and bioaccumulation of pollutants in estuarine and marine biota and linkage to seafood security. Mar. pollut. Bull. 167, 112364. doi: 10.1016/j.marpolbul.2021.112364
Knap A. H., Michaels A. F., Steinberg D. K., Bahr F., Bates N. R., Bell S., et al. (1997). “The determination of phosphorus in sea water,” in BATS Methods Manual, Version 4 (U.S. JGOFS Planning Office, Woods Hole, MA, USA), 71–74.
Koch M. S., Kletou D. C., Tursi R. (2009). Alkaline phosphatase activity of water column fractions and seagrass in a tropical carbonate estuary, Florida Bay. Estuar. Coast. Shelf Sci. 83, 403–413. doi: 10.1016/j.ecss.2009.04.007
Labasque T., Chaumery C., Aminot A., Kergoat G. (2004). Spectrophotometric Winkler determination of dissolved oxygen: re-examination of critical factors and reliability. Mar. Chem. 88, 53–60. doi: 10.1016/j.marchem.2004.03.004
Larkum A. W. D., Drew E. A., Ralph P. J. (2006). “Photosynthesis and metabolism in seagrasses at the cellular level,” in Seagrasses: Biology, Ecology and Conservation. Eds. Larkum A. W. D., Orth R. J., Duarte C. M. (Springer Netherlands, Dordrecht, The Netherlands), 323–345.
Lee K. S., Park S. R., Kim Y. K. (2007). Effects of irradiance, temperature, and nutrients on growth dynamics of seagrasses: A review. J. Exp. Mar. Biol. Ecol. 350, 144–175. doi: 10.1016/j.jembe.2007.06.016
Liu J., Yin P., Zhao L. (2019). Adverse effect of TiO2 nanoparticles on antioxidant system and antitumor activities of macroalgae Gracilaria lemaneiformis. J. Ocean Univ. China 18, 1130–1138. doi: 10.1007/s11802-019-3819-4
Lopes F. C., de Castro M. R., Patrocinio G. T. A., da Silveira Guerreiro A., Barbosa S. C., Primel E. G., et al. (2022). Detoxification and effects of the UV filter Benzophenone-3 in the digestive gland and hemocytes of yellow clam (Amarilladesma mactroides) under a perspective of global warming scenario. Mar. pollut. Bull. 185, 114188. doi: 10.1016/j.marpolbul.2022.114188
Malea P., Charitonidou K., Sperdouli I., Mylona Z., Moustakas M. (2019). Zinc uptake, photosynthetic efficiency and oxidative stress in the seagrass Cymodocea nodosa exposed to ZnO nanoparticles. Materials 12, 2101. doi: 10.3390/ma12132101
Martins A. M., Marto J. M. (2023). A sustainable life cycle for cosmetics: From design and development to post-use phase. Sustain Chem. Pharm. 35, 101178. doi: 10.1016/j.scp.2023.101178
Masini R. J., Manning C. R. (1997). The photosynthetic responses to irradiance and temperature of four meadow-forming seagrasses. Aquat Bot. 58, 21–36. doi: 10.1016/S0304-3770(97)00008-9
McGlathery K., Risgaard-Petersen N., Christensen P. (1998). Temporal and spatial variation in nitrogen fixation activity in the eelgrass Zostera marina rhizosphere. Mar. Ecol. Prog. Ser. 168, 245–258. doi: 10.3354/meps168245
Milito A., Castellano I., Damiani E. (2021). From sea to skin: is there a future for natural photoprotectants? Mar. Drugs 19, 379. doi: 10.3390/md19070379
Molins-Delgado D., Díaz-Cruz M. S., Barceló D. (2014). “Introduction: personal care products in the aquatic environment,” in Personal Care Products in the Aquatic Environment. The Handbook of Environmental Chemistry. Eds. Díaz-Cruz M., Barceló D. (Springer, Cham), 1–34. doi: 10.1007/698_2014_302
Mylona Z., Panteris E., Kevrekidis T., Malea P. (2020a). Effects of titanium dioxide nanoparticles on leaf cell structure and viability, and leaf elongation in the seagrass Halophila stipulacea. Sci. Total Environ. 719, 137378. doi: 10.1016/j.scitotenv.2020.137378
Mylona Z., Panteris E., Kevrekidis T., Malea P. (2020b). Physiological and structural responses of the seagrass Cymodocea nodosa to titanium dioxide nanoparticle exposure. Botanica Marina 63, 493–507. doi: 10.1515/bot-2020-0047
Núñez-Pons L., Avila C., Romano G., Verde C., Giordano D. (2018). UV-protective compounds in marine organisms from the Southern ocean. Mar. Drugs 16, 336. doi: 10.3390/md16090336
Oliver E. C. J., Donat M. G., Burrows M. T., Moore P. J., Smale D. A., Alexander L. V., et al. (2018). Longer and more frequent marine heatwaves over the past century. Nat. Commun. 9, 1324. doi: 10.1038/s41467-018-03732-9
Olsen Y. S., Sánchez-Camacho M., Marbà N., Duarte C. M. (2012). Mediterranean seagrass growth and demography responses to experimental warming. Estuaries Coasts 35, 1205–1213. doi: 10.1007/s12237-012-9521-z
Orth R. J., Carruthers T. J. B., Dennison W. C., Duarte C. M., Fourqurean J. W., Heck K. L., et al. (2006). A global crisis for seagrass ecosystems. Bioscience 56, 987–996. doi: 10.1641/0006-3568(2006)56[987:AGCFSE]2.0.CO;2
Oviatt C. A. (2004). The changing ecology of temperate coastal waters during a warming trend. Estuaries 27, 895–904. doi: 10.1007/BF02803416
Pai S.-C., Su Y.-T., Lu M.-C., Chou Y., Ho T.-Y. (2021). Determination of nitrate in natural waters by vanadium reduction and the griess assay: reassessment and optimization. ACS ES&T Water 1, 1524–1532. doi: 10.1021/acsestwater.1c00065
Pazzaglia J., Santillán-Sarmiento A., Helber S. B., Ruocco M., Terlizzi A., Marín-Guirao L., et al. (2020). Does warming enhance the effects of eutrophication in the seagrass Posidonia oceanica? Front. Mar. Sci. 7. doi: 10.3389/fmars.2020.564805
Pirc H. (1986). Seasonal aspects of photosynthesis in Posidonia oceanica: Influence of depth, temperature and light intensity. Aquat. Bot. 26, 203–212. doi: 10.1016/0304-3770(86)90021-5
Pirone G., Coppola F., Pretti C., Soares A. M. V. M., Solé M., Freitas R. (2019). The effect of temperature on Triclosan and Lead exposed mussels. Comp. Biochem. Physiol. B Biochem. Mol. Biol. 232, 42–50. doi: 10.1016/j.cbpb.2019.02.007
Procaccini G., Alberte R., Mazzella L. (1996). Genetic structure of the seagrass Posidonia oceanica in the Western Mediterranean: ecological implications. Mar. Ecol. Prog. Ser. 140, 153–160. doi: 10.3354/meps140153
Rahi D., Dzyuba B., Policar T., Malinovskyi O., Rodina M., Dzyuba V. (2021). Bioenergetic pathways in the sperm of an under-ice spawning fish, burbot (Lota lota): the role of mitochondrial respiration in a varying thermal environment. Biol. (Basel) 10, 739. doi: 10.3390/biology10080739
Repolho T., Duarte B., Dionísio G., Paula J., Lopes A., Rosa I., et al. (2017). Seagrass ecophysiological performance under ocean warming and acidification. Sci. Rep. 7, 41443. doi: 10.1038/srep41443
Reusch T. B. H., Ehlers A., Hämmerli A., Worm B. (2005). Ecosystem recovery after climatic extremes enhanced by genotypic diversity. Proc. Natl. Acad. Sci. 102, 2826–2831. doi: 10.1073/pnas.0500008102
Rodríguez-Romero A., Ruiz-Gutiérrez G., Viguri J. R., Tovar-Sánchez A. (2019). Sunscreens as a new source of metals and nutrients to coastal waters. Environ. Sci. Technol. 53, 10177–10187. doi: 10.1021/acs.est.9b02739
Rotini A., Belmonte A., Barrote I., Micheli C., Peirano A., Santos R. O., et al. (2013). Effectiveness and consistency of a suite of descriptors for assessing the ecological status of seagrass meadows (Posidonia oceanica L. Delile). Estuar. Coast. Shelf Sci. 130, 252–259. doi: 10.1016/j.ecss.2013.06.015
Saha S., Kaviraj A. (2009). Effects of cypermethrin on some biochemical parameters and its amelioration through dietary supplementation of ascorbic acid in freshwater catfish Heteropneustes fossilis. Chemosphere 74, 1254–1259. doi: 10.1016/j.chemosphere.2008.10.056
Sánchez-Quiles D., Tovar-Sánchez A. (2015). Are sunscreens a new environmental risk associated with coastal tourism? Environ. Int. 83, 158–170. doi: 10.1016/j.envint.2015.06.007
Schaap I., Slijkerman D. M. E. (2018). An environmental risk assessment of three organic UV-filters at Lac Bay, Bonaire, Southern Caribbean. Mar. pollut. Bull. 135, 490–495. doi: 10.1016/j.marpolbul.2018.07.054
Shah K., Kumar R. G., Verma S., Dubey R. S. (2001). Effect of cadmium on lipid peroxidation, superoxide anion generation and activities of antioxidant enzymes in growing rice seedlings. Plant Sci. 161, 1135–1144. doi: 10.1016/S0168-9452(01)00517-9
Singleton V. L., Orthofer R., Lamuela-Raventós R. M. (1999). Analysis of total phenols and other oxidation substrates and antioxidants by means of Folin-Ciocalteu reagent. Methods Enzymol. 299, 152–178. doi: 10.1016/S0076-6879(99)99017-1
Stal L. J. (1988). Nitrogen fixation in cyanobacterial mats. Methods Enzymol. 167, 474–484. doi: 10.1016/0076-6879(88)67052-2
Stipcich P., Marín-Guirao L., Pansini A., Pinna F., Procaccini G., Pusceddu A., et al. (2022). Effects of current and future summer marine heat waves on Posidonia oceanica: plant origin matters? Front. Climate 4. doi: 10.3389/fclim.2022.844831
Strydom S., Murray K., Wilson S., Huntley B., Rule M., Heithaus M., et al. (2020). Too hot to handle: Unprecedented seagrass death driven by marine heatwave in a World Heritage Area. Glob Chang Biol. 26, 3525–3538. doi: 10.1111/gcb.15065
Thorel E., Clergeaud F., Jaugeon L., Rodrigues A. M. S., Lucas J., Stien D., et al. (2020). Effect of 10 UV filters on the brine shrimp Artemia salina and the marine microalga Tetraselmis sp. Toxics 8, 29. doi: 10.3390/toxics8020029
Tovar-Sánchez A., Sánchez-Quiles D., Basterretxea G., Benedé J. L., Chisvert A., Salvador A., et al. (2013). Sunscreen products as emerging pollutants to coastal waters. PloS One 8, e65451. doi: 10.1371/journal.pone.0065451
Tovar-Sánchez A., Sánchez-Quiles D., Rodríguez-Romero A. (2019). Massive coastal tourism influx to the Mediterranean Sea: the environmental risk of sunscreens. Sci. Total Environ. 656, 316–321. doi: 10.1016/j.scitotenv.2018.11.399
Tovar-Sánchez A., Sparaventi E., Gaudron A., Rodríguez-Romero A. (2020). A new approach for the determination of sunscreen levels in seawater by ultraviolet absorption spectrophotometry. PloS One 15, e0243591. doi: 10.1371/journal.pone.0243591
Vale G., Mehennaoui K., Cambier S., Libralato G., Jomini S., Domingos R. F. (2016). Manufactured nanoparticles in the aquatic environment-biochemical responses on freshwater organisms: A critical overview. Aquat. Toxicol. 170, 162–174. doi: 10.1016/j.aquatox.2015.11.019
Vargas-Yáñez M., García-Martínez M., Moya- Ruiz F., Tel E., Parrilla G., Plaza F., et al. (2007). Cambio Climático en el Mediterráneo español. Ed. Instituto Español de Oceanografía and Ministerio de Educación y Ciencia (Madrid, Spain: Impresos y Revistas, S.A).
Varrella S., Danovaro R., Corinaldesi C. (2022). Assessing the eco-compatibility of new generation sunscreen products through a combined microscopic-molecular approach. Environ. pollut. 314, 120212. doi: 10.1016/j.envpol.2022.120212
Vaulot D., Lebot N., Marie D., Fukai E. (1996). Effect of phosphorus on the synechococcus cell cycle in surface mediterranean waters during summer. Appl. Environ. Microbiol. 62, 2527–2533. doi: 10.1128/aem.62.7.2527-2533.1996
Waycott M., Duarte C. M., Carruthers T. J. B., Orth R. J., Dennison W. C., Olyarnik S., et al. (2009). Accelerating loss of seagrasses across the globe threatens coastal ecosystems. Proc. Natl. Acad. Sci. 106, 12377–12381. doi: 10.1073/pnas.0905620106
Weber A., Jeckel N., Wagner M. (2020). Combined effects of polystyrene microplastics and thermal stress on the freshwater mussel Dreissena polymorpha. Sci. Total Environ. 718, 137253. doi: 10.1016/j.scitotenv.2020.137253
Weber-Shirk M. L., Lion L. W., Bisogni J. J. Jr. (2001). “Phosphorus determination using the colorimetric ascorbic acid technique,” in Laboratory Research in Environmental Engineering, Laboratory Manual (Cornell University, Ithaca, USA), 64–69.
Wijgerde T., van Ballegooijen M., Nijland R., van der Loos L., Kwadijk C., Osinga R., et al. (2020). Adding insult to injury: Effects of chronic oxybenzone exposure and elevated temperature on two reef-building corals. Sci. Total Environ. 733, 139030. doi: 10.1016/j.scitotenv.2020.139030
Wintermans J., De Mots A. (1965). Spectrophotometric characteristics of chlorophylls a and b and their pheophytins in ethanol. Biochim. Biophys. Acta 109, 448–453. doi: 10.1016/0926-6585(65)90170-6
Wong S. W. Y., Zhou G.-J., Leung P. T. Y., Han J., Lee J.-S., Kwok K. W. H., et al. (2020). Sunscreens containing zinc oxide nanoparticles can trigger oxidative stress and toxicity to the marine copepod Tigriopus japonicus. Mar. pollut. Bull. 154, 111078. doi: 10.1016/j.marpolbul.2020.111078
Xing Q., Kim Y. W., Kim D., Park J.-S., Yoo H. I. L., Yarish C., et al. (2022). Effects of the ultraviolet filter oxybenzone on physiological responses in a red macroalga, Gracilaria vermiculophylla. Aquat Bot. 179, 103514. doi: 10.1016/j.aquabot.2022.103514
Keywords: sunscreen pollution, ocean warming, seagrass meadows, Mediterranean, stress factors
Citation: García-Márquez MG, Muñoz Entrena S, Clément C and Agawin NSR (2024) Effects of sunscreen exposure on Posidonia oceanica (L.) Delile under a perspective of increased seawater temperature scenario. Front. Mar. Sci. 11:1443692. doi: 10.3389/fmars.2024.1443692
Received: 04 June 2024; Accepted: 26 July 2024;
Published: 09 August 2024.
Edited by:
Michael Alan Rasheed, James Cook University, AustraliaReviewed by:
Simone Strydom, Conservation and Attractions (DBCA), AustraliaJinlin Liu, Tongji University, China
Copyright © 2024 García-Márquez, Muñoz Entrena, Clément and Agawin. This is an open-access article distributed under the terms of the Creative Commons Attribution License (CC BY). The use, distribution or reproduction in other forums is permitted, provided the original author(s) and the copyright owner(s) are credited and that the original publication in this journal is cited, in accordance with accepted academic practice. No use, distribution or reproduction is permitted which does not comply with these terms.
*Correspondence: Manuela Gertrudis García-Márquez, bWFudWVsYWcuZ2FyY2lhbUB1aWIuZXM=