- 1Department of Arctic Biology, The University Centre in Svalbard, Longyearbyen, Norway
- 2Faculty of Biosciences, Fisheries and Economics, The Arctic University of Norway, Tromsø, Norway
- 3Marine Botany, University of Bremen, Bremen, Germany
Vegetated coastal marine ecosystems are projected to expand northwards in the Arctic due to climate change, but the mechanisms for this expansion are complex and nuanced. Macroalgal biomass in the littoral areas of Svalbard has been increasing, but data at the glacier fronts are very scarce. In this study, we use hydroacoustics and video validation from an unmanned surface vehicle to survey macroalgal bed distribution along the coast of a High Arctic fjord (Billefjorden, Svalbard), including river bays and land- and sea- terminating glacier fronts, as well as oceanographic measurements to indicate physical drivers of macroalgal settlement. We found high variation of macroalgal coverage along the fjord coastline, with virtually no macroalgae in the river bays but abundant coverage in areas with little terrestrial runoff. Furthermore, the presence of kelp was found at the land-terminating glacier front which has recently retreated from the sea, which suggests the potential for rapid macroalgal establishment in newly available substrate following glacial retreat. These findings suggest large ecological implications throughout the Arctic, in which macroalgal expansion may lead to significant changes in the underwater coastal landscape and ecosystem. This study shows that the use of remote autonomous vehicles and hydroacoustic mapping with video validation has a high potential for sustainable and efficient ecological monitoring.
1 Introduction
The High Arctic is undergoing the most severe climate change of any region in the world and consequently marine ecosystems are being affected by an increase in temperature, precipitation, melting, river discharge and sedimentation (Box et al., 2019). Representing the boundary between marine and terrestrial environments where land and glacial inflow enter the marine system, littoral areas in fjord systems are particularly vulnerable to these changes, and the loss of the cryosphere is one of the main drivers for changes in biodiversity and ecosystem function in these areas (Bring et al., 2016; Hawkins et al., 2008; Hopwood et al., 2020; IPCC, 2022). The melting of tidewater glaciers and decrease in sea-ice is affecting biogeochemical parameters such as turbidity, salinity, as well as nutrient and light availability (Henson et al., 2023; Hopwood et al., 2020), and these changes may affect community composition in coastal fjord areas (Fredriksen et al., 2014; Weslawski et al., 2010).
Macroalgae are commonly found on rocky substrate and boulders in littoral and sublittoral fjord regions in the Svalbard archipelago (Fredriksen and Røst Kile, 2012; Hop et al., 2012), where they are key primary producers and represent important feeding and nursing sites for fish and benthic fauna (Carlsen et al., 2007; Lippert et al., 2001; Włodarska-Kowalczuk et al., 2009). The macroalgal community composition on Svalbard is similar to the Norwegian mainland and other regions of the North Atlantic, with few endemic Arctic species (e.g. Laminaria solidungula) (Fredriksen et al., 2015). Macroalgae have been shown to dominate at the sublittoral and littoral zones (2 - 15 m depth), with fucales in the shallow areas and species such as Saccharina latissima, Alaria esculenta, and Laminaria digitata commonly forming dense beds deeper in the euphotic zone in Svalbard fjords (Hop et al., 2012). In the Arctic, macroalgae are commonly absent in the uppermost sublittoral zone due to ice scouring (Bartsch et al., 2016; Gutt, 2001), which has an effect down to 5 m in Svalbard (Wiktor et al., 2022). Temperature and salinity are important drivers for macroalgal growth and vary significantly over the seasons in the high Arctic (Bischof et al., 2019). Macroalgae have been shown to have a high tolerance to variations in temperature and salinity, especially in the upper sublittoral zone, but freezing of the thalli and rapid changes in salinity have been shown to inhibit photosynthetic efficiency at least transiently (Becker et al., 2009; Hop et al., 2012; Karsten, 2007). Light availability is also an important factor determining the depth distribution of macroalgae (Bartsch et al., 2016; Düsedau et al., 2024), with high amounts of radiation in the uppermost littoral zone and light limitation deeper in the water column and under sea-ice (Svendsen et al., 2002). The lower depth limit for the most abundant kelp species in Svalbard is from 15 to 20 m, and is undergoing an upward shift as a consequence of a deteriorating light climate (Borum et al., 2002; Düsedau et al., 2024; Hop et al., 2012). Furthermore, mechanical factors such as tidal conditions and wave exposure are important drivers for macroalgal occurrence and community composition (Bird et al., 2013), with species like Laminaria digitata thriving in exposed sites (Gilson et al., 2023) while the opposite is observed for Saccharina latissima (Visch et al., 2020).
Changing environmental factors such as water temperature, salinity, sedimentation and light availability may have a direct impact on the spatial distribution of macroalgae (Bischof et al., 2019), and consequently affect associated species and ultimately the community composition of Arctic coastal ecosystems (Kortsch et al., 2012; Weslawski et al., 2010). The responses of macroalgal communities to changes in the cryosphere include complex interactions between environmental factors, macroalgal growth and distribution. High sediment load in the water column affects the light regime and decreases the availability of photosynthetically available radiation (Hanelt et al., 2001; Ronowicz et al., 2020). Species richness and biomass of macroalgae subsequently tend to decrease in close proximity to glacier fronts, and community composition changes with increased turbidity from glacier run-off (Ronowicz et al., 2020). Sea-terminating or tidewater glaciers flow into the ocean and their front is exposed to the marine system, while land-terminating glaciers do not have direct contact with the sea. The transition of sea-terminating to land-terminating glaciers due to increased glacial melting is changing the biogeochemical dynamics in these coastal areas, such as acidification state (Henson et al., 2023), which may impact macroalgal communities and the associated fauna by selecting those better suited for the changing conditions (Niedzwiedz and Bischof, 2023).
Heightened sedimentation due to increased river run-off and glacier melting may result in higher concentrations of dissolved bioavailable nutrients (Vonnahme et al., 2021), which potentially favours macroalgal growth. Conversely, higher river run-off can cause an accumulation of particles such as silt, sand or clay and lead to shifts in bottom sediments towards a soft-bottom seafloor which is not suitable for the settlement of macroalgae (Mann et al., 2022). Furthermore, glacial retreat is uncovering new hard substrate where macroalgae have the potential to settle, expanding their distribution (Deregibus et al., 2023). Reduced ice-scouring following sea-ice retreat may also allow for macroalgae settling in new regions (Krause-Jensen et al., 2020; Krause-Jensen and Duarte, 2014), and a northward expansion of macroalgae is expected (Assis et al., 2022).
There has been a recorded increase in littoral macroalgal biomass in Svalbard over the last 30 years (Bartsch et al., 2016; Fredriksen et al., 2015; Weslawski et al., 2010), which may be due to a decrease in mechanical disturbances such as sea-ice scouring, new substrate for settlement and higher bioavailability of nutrients (Krause-Jensen et al., 2020; Krause-Jensen and Duarte, 2014). Overall, studies show that macrophytes are generally expanding in the Arctic (IPCC, 2022; Krause-Jensen et al., 2020), but there is little research done at glacier fronts due to high difficulty to access and sample these areas.
Direct biological sampling, such as dive surveys, offers high detail and reliability to map underwater habitats and community composition, but it is rather expensive, localized and time-consuming. Acoustic methods are emerging as efficient tools for habitat assessment and ecosystem mapping and monitoring (Anderson et al., 2008; Brown et al., 2011), as they can be automated and used at large scales. Studies have shown that macroalgal beds significantly influence the strength of acoustic backscatter by dampening the return echo signal (Brown et al., 2011; Riegl et al., 2005), and hydroacoustic methods have already been used successfully to map macroalgal coverage in Arctic fjords (Kruss et al., 2017).
Due to their high susceptibility to environmental perturbations as well as their sedentary lifestyle, macroalgae are good indicators of changing conditions (Bischof et al., 2019; Fredriksen et al., 2014). Studying the spatial abundance and distribution of macroalgae at a local scale with high spatial resolution, and in relation to changes in the cryosphere and different fjord littoral habitats (glacier front, river bays and marine conditions with minimal land runoff), will contribute to inferring effects of climate change at a larger spatial scale.
The aim of this study was to map sublittoral cover of macroalgae beds in inner Billefjorden, Svalbard, and the Nordenskiöldbreen glacier front, which has recently partly transitioned from sea- to land- terminating, and investigate the role of turbidity, temperature and salinity in macroalgal presence in these areas. These oceanographical parameters were chosen as they are known drivers of macroalgal growth and are undergoing significant shifts due to climate changes, particularly in coastal fjord areas due to glacial melting and increased land runoff (Bischof et al., 2019; IPCC, 2022). We hypothesize that macroalgal biomass will decrease with increasing proximity to the glacier front and river bays due to high sedimentation rate and low light availability. Macroalgal distribution may be limited to shallower depths in these areas, where there is still enough available light to sustain growth. Finally, there might be an optimal proximity to the glacier front that allows for macroalgal growth, where there is increased nutrient concentration from subglacial upwelling and new substrate for settlement, but where the turbidity and mechanical disturbance is not high enough to prevent macroalgal settling. This study system may be used as a model to illustrate the changes taking place broadly in the Arctic.
2 Materials and methods
2.1 Study area
Billefjorden is located in Spitsbergen, Svalbard (Figure 1), and has a shallow sill at the mouth of the fjord, so that there is a layer of winter cold water at the bottom and a warmer shallow layer of Atlantic water above that enters via offshoots of the West Spitsbergen current (Nilsen et al., 2008). The inner part of the fjord is characterized by the river bay Petuniabukta and the glacier Nordenskiöldbreen in the adjacent bay Adolfbukta. This glacier has been retreating at a rate of 12-35 m per year (Szczuciński et al., 2009) and has recently receded to where the northern side is mostly land-terminating. The river bay, Petuniabukta, lies in the northwestern end of the fjord and receives a high amount of freshwater and sediment input due to the surrounding valley glaciers and mountains (Láska et al., 2012). Outwards from the river and glacier bays (Scottvika), river and glacier influence is minimal, which means that Billefjorden functions as a natural laboratory wherein the impact of both river and glacier systems can be systematically assessed. Geomorphological mapping showed that inner Billefjorden is characterized by soft bottom substrate in Petuniabukta, and both rocky and soft bottom in the glacier bay and surrounding coastline (Søreide et al., 2021). There has been a decrease in land-fast sea ice cover in Svalbard over the last decades, but inner Billefjorden is still ice-covered during the winter and spring seasons (Urbański and Litwicka, 2022).
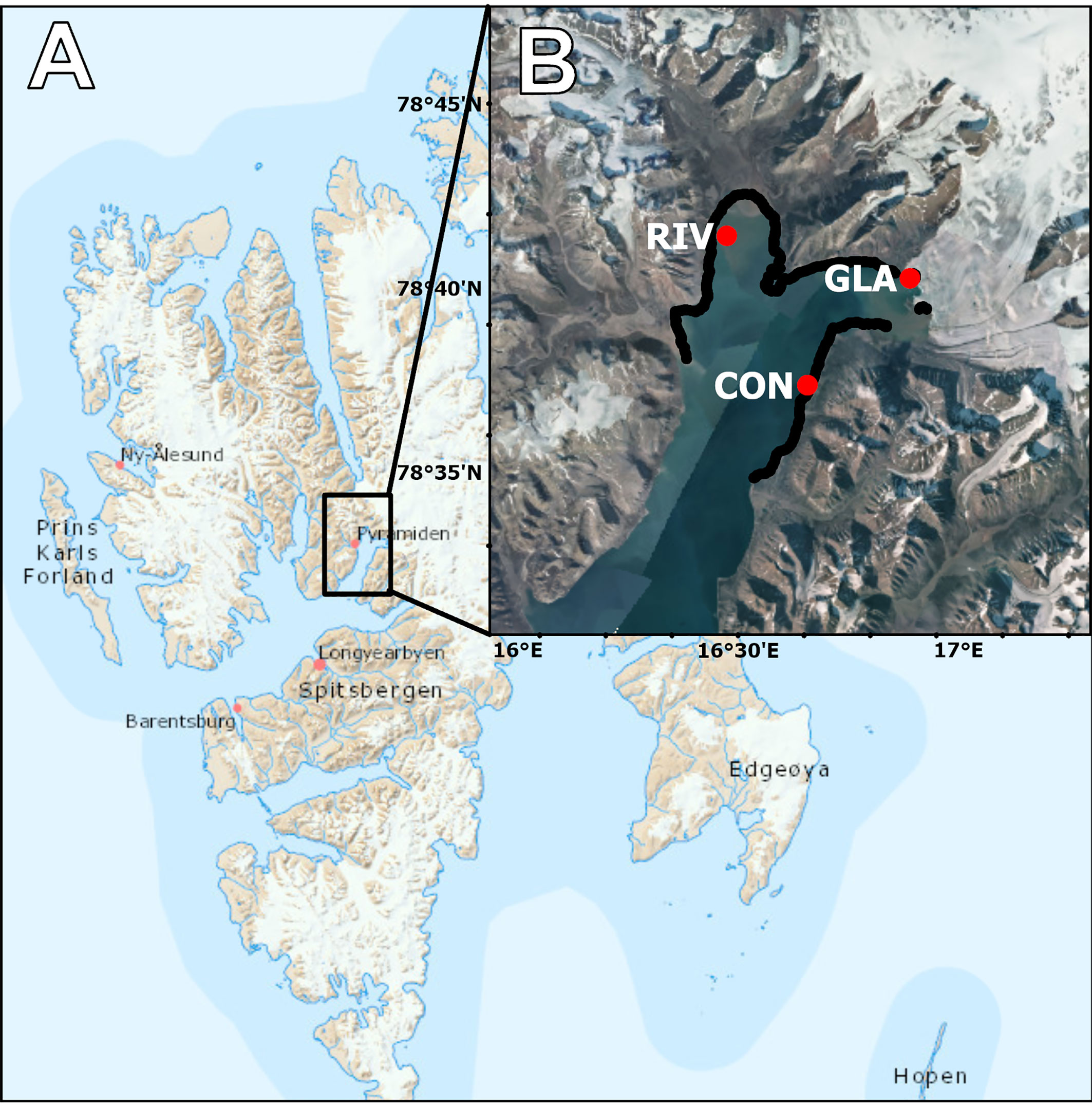
Figure 1. (A) Topographic map of Svalbard. (B) Satellite image of Billefjorden with the data transect from the coastal survey in Billefjorden (black line) and stations where CTD (Conductivity, Temperature and Density) water column profiles were done (red dots). Basemap source: Norwegian Polar Institute, Geodata AS, Kartverket, Geovekst og kommunene; Esri, USGS.
2.2 Coastal survey
A large-scale survey was done along the coastline of inner Billefjorden, Svalbard. Acoustic mapping was performed by split-beam echosounder (SBES) measurements mounted on an unmanned surface vehicle (USV) and the seafloor was imaged with a drop camera deployed from the USV for validation of the acoustic data. A Valeport SWiFT CTDplus with Turbidity (Valeport Ltd., Totnes, UK) was mounted on the USV to collect surface oceanographic data, which included temperature (degrees Celsius), salinity (practical salinity units; psu) and turbidity (nephelometric turbidity units; ntu).
The Otter USV (Maritime Robotics AS, Trondheim, Norway) is an electric 200 x 108 x 106 cm twin hull catamaran that can carry a variety of oceanographic sensors and cameras and was used to access very shallow areas and the glacier fronts, which cannot be sampled by traditional methods. The USV was controlled remotely via broadband communication radio (Direct link 5150 – 5875 MHz) from a nearby vessel and was equipped with a downward facing BioSonics DT-X scientific split-beam echosounder (BioSonics, Seattle, USA) mounted at the back of the vehicle. The USV’s internal GNSS system was used for georeferencing the echosounder data. The DT-X echosounder was used at an operating frequency of 200 kHz, a beam angle of 6.9°x 6.9°, a ping rate of 5 Hz and a pulse duration of 0.4 ms, and the USV was driven at a speed of approximately 3 knots. Autonomous vehicles have been used in previous studies to map the seafloor and study marine ecosystems (Johnsen et al., 2016; Ludvigsen et al., 2018), and provide an effective and sustainable methodology that is capable to reach understudied areas that are difficult to access.
A total of 40 km of coastline were surveyed over three separate days in July and August 2021, and the USV was manually run at a mean bottom depth isoline of 7 m (Figure 1). In August 2022, CTD profiles were taken down to bottom depth in Petuniabukta, the Nordenskiöldbreen glacier and Scottvika as locations indicating different environmental conditions (high freshwater and sedimentation from rivers – RIV, glacier front – GLA, and control marine station – CON, respectively), to complement the surface CTD measurements taken from the USV.
2.3 Acoustic detection of macroalgae
Acoustic detection of submerged flora is based on their physical properties, which scatter the acoustic signal differently than unvegetated bottom (Carbó and Molero, 1997; Kruss et al., 2017). Hydroacoustic mapping has been used extensively to map macroalgal coverage in coastal areas (Brown et al., 2011) and scientific split-beam echosounders have been used to study macroalgal settlement in Svalbard fjords (Kruss et al., 2017; Wiktor et al., 2022). The vegetation analyses were performed in BioSonics’ software Visual Aquatic v.1.0.0.12146 using an edge detection method. This method is based on analysing the strength of acoustic echoes to establish boundaries between the bottom layer, macroalgal cover and the water column. When macroalgae are present, the echogram shows a weak backscattering layer above the strong bottom signal (Figure 2).
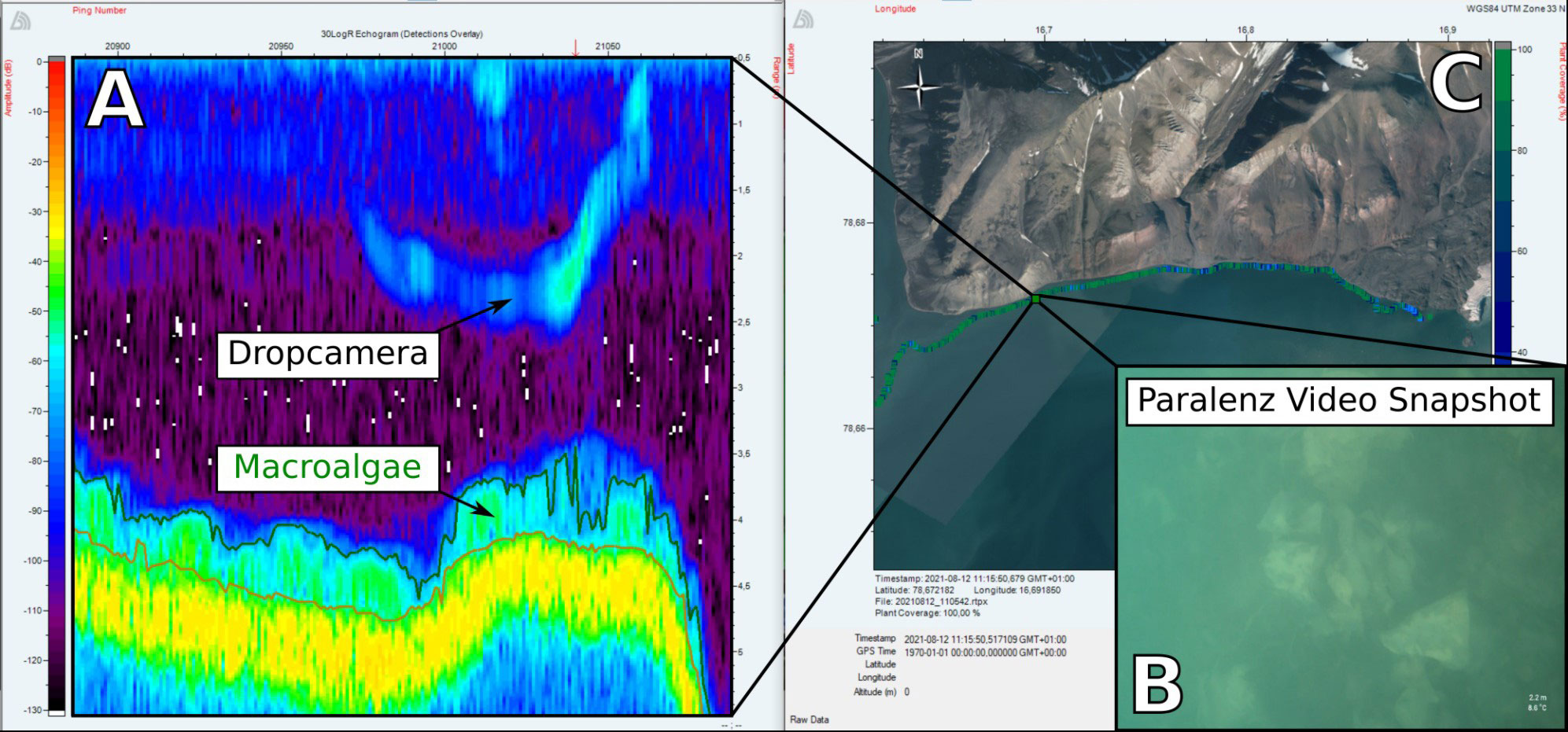
Figure 2. Process of validation of the acoustic data via drop camera samplings. (A) Echogram showing the acoustic signal from macroalgae. The signal from the drop camera is also shown. Brighter colours indicate stronger echo signal. (B) Video snapshot of the ocean floor at the same location. (C) Location of the respective sampling point.
The acoustic signal was recorded as Sv values (volume backscattering strength) and the files were inspected for noise and a threshold of -40 dB was used to determine bottom depth. The default threshold of -70 dB was used to detect presence of macroalgae. These thresholds were used based on existent literature and ground-truthing during field testing to determine the optimal values for bottom and plant acoustic boundaries (Radomski and Holbrook, 2015; Simmonds and MacLennan, 2005). A minimum detection distance of 0.3 m above the bottom was used to exclude the detection of smaller bottom structures and drift material and only include large macroalgal formations, as common macroalgae species in the Arctic can grow to >1 m in length (Diehl et al., 2023). Macroalgal detection was limited to a depth range from 1 to 20 m and minor manual editing of the bottom and vegetation lines was done to correct for noise and fish that merged with the bottom signal. The pings were clustered in groups of 50, which at a ping rate of 5 s-1 effectively equalled to one data point for every 10 seconds. The algorithm then detected macroalgal percentage cover of those ping clusters, and50% coverage and higher was assigned as macroalgal presence. Macroalgal presence/absence using a threshold of 50% coverage was used as a conservative approach to complement the height threshold and focus on large and established macroalgal beds and avoid over-representing macroalgal coverage by including detached and drift material and single units of macroalgae.
To ground-truth the acoustic data, a Paralenz Vaquita (Paralenz, Rødovre, Denmark) drop camera was deployed from the USV simultaneously to the echosounder recording. The camera was rigged to the USV’s winch system which was linked to the echosounder and programmed to be lowered to 1.5 m from the bottom and record video of the seafloor for 10 seconds.
A total of 30 drop camera deployments were used to validate the macroalgae detection algorithm. This footage was obtained at the land-terminating glacier front, along the northern coast of Adolfbukta and in Petuniabukta between 5 and 10 m bottom depth (Figure 3; Supplementary Table 1). Video that showed macroalgae covering more than 50% of the frame were assigned as indicating macroalgal presence to align with what constituted macroalgal presence in the acoustic algorithm, and this footage was cross-referenced with the acoustic data based on time stamps as well as visualizing the camera in the echogram.
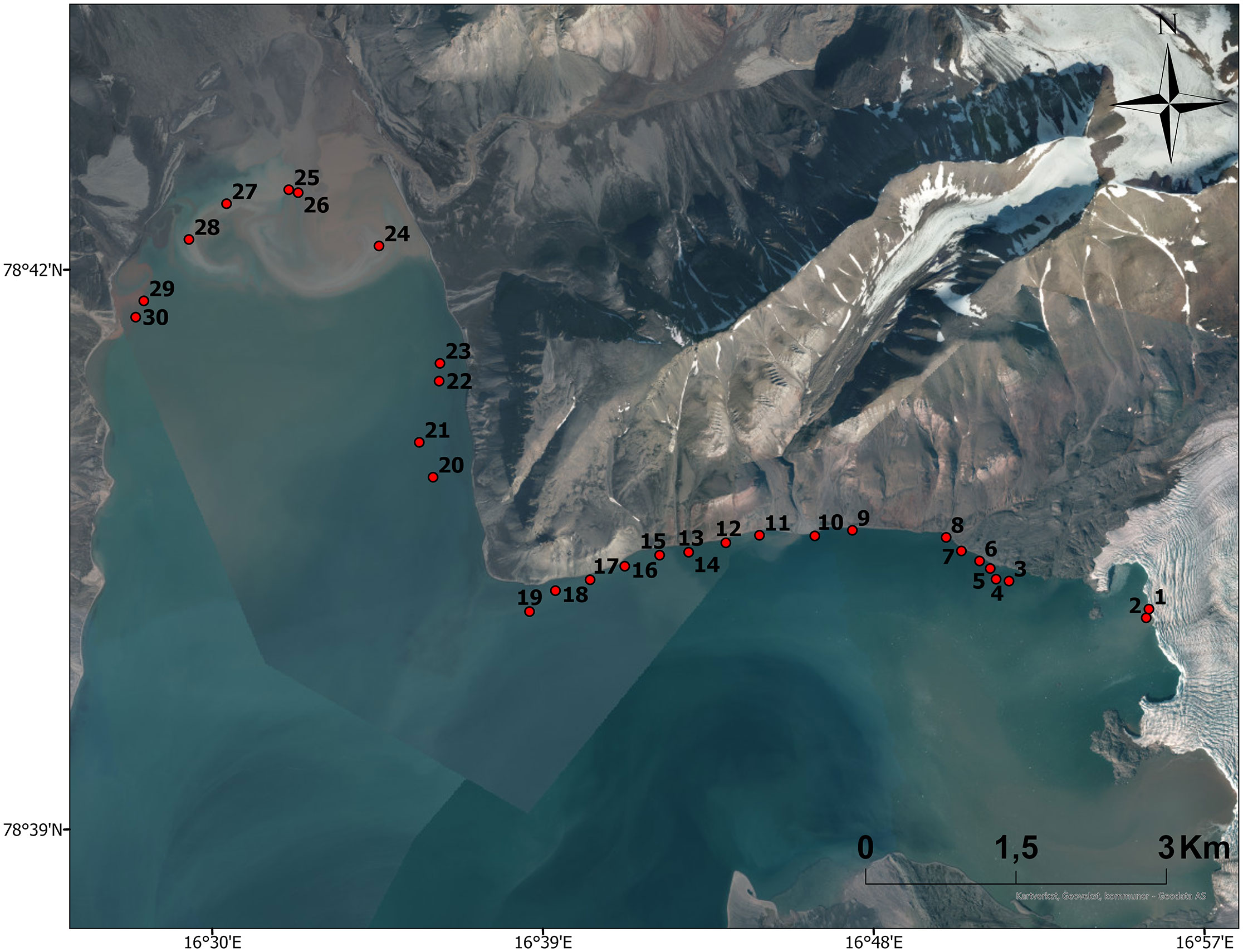
Figure 3. Drop camera locations along the Unmanned Surface Vehicle (USV) survey. Additional information on the environmental conditions and macroalgal coverage is presented in the Supplementary Material. Basemap: Norwegian Polar Institute, Geodata AS, Kartverket, Geovekst og kommunene; Esri, USGS.
2.4 Mapping and statistical analyses
In order to test the correlation between surface temperature, salinity and turbidity with macroalgal occurrence, the acoustic and CTD data were merged together by aggregating the CTD data in intervals of 10 seconds and matching the start time stamp of each acoustic cluster of 50 pings. All statistical analyses were done in RStudio v2023.03.1, and the “dplyr” and “lubridate” packages were used for the data manipulation (Grolemund and Wickham, 2011; Wickham et al., 2023).
Box plots were made using the “ggplot2” package to visualize the frequency of presence and absence of macroalgae in relation to the range values for surface temperature, salinity and turbidity. Data were analysed for normality using a Saphiro-Wilk test and checked for multicollinearity and homoscedasticity. A correlation matrix was performed to analyse the relationships between temperature, salinity and turbidity. Logistic regression (Sperandei, 2014) was used on the merged dataset to analyse the relationship between temperature, salinity and turbidity with macroalgal presence/absence, and the model output exported using the “broom” package. In addition, an inflated beta regression model (Ospina and Ferrari, 2010) was used to analyse the relationship between temperature, salinity and turbidity with macroalgal percent coverage directly using the “gamlss” package. The CTD water column profile data was analysed and plotted using the “oce” package.
Macroalgal presence/absence, percent coverage and sampling locations were mapped in ArcGIS Pro v3.1.
3 Results
3.1 Acoustic survey and image validation
The bottom depth of the acoustic survey ranged from 1 to 20 m depth, with a mean of 7.1 m. Of a total of 3481 data points, there were 1085 reports of macroalgal presence (>50% coverage) and 2396 of absence (<50% coverage). Overall, the survey revealed macroalgal coverage to be quite heterogenous along the coast of Billefjorden (Figure 4). The eastern coastline showed patchy macroalgal coverage, decreasing with proximity to the sea-terminating glacier (southern side). The sea-terminating glacier front showed no coverage, while macroalgae were present adjacent to the land-terminating front (northern side). High macroalgal coverage was observed northwest of the glacier, decreasing with proximity to the river bay and with virtually no macroalgae in the inner part of the bay. Instances with coverage increase on the western coastline and drop in front of the river bay at the end of the survey.
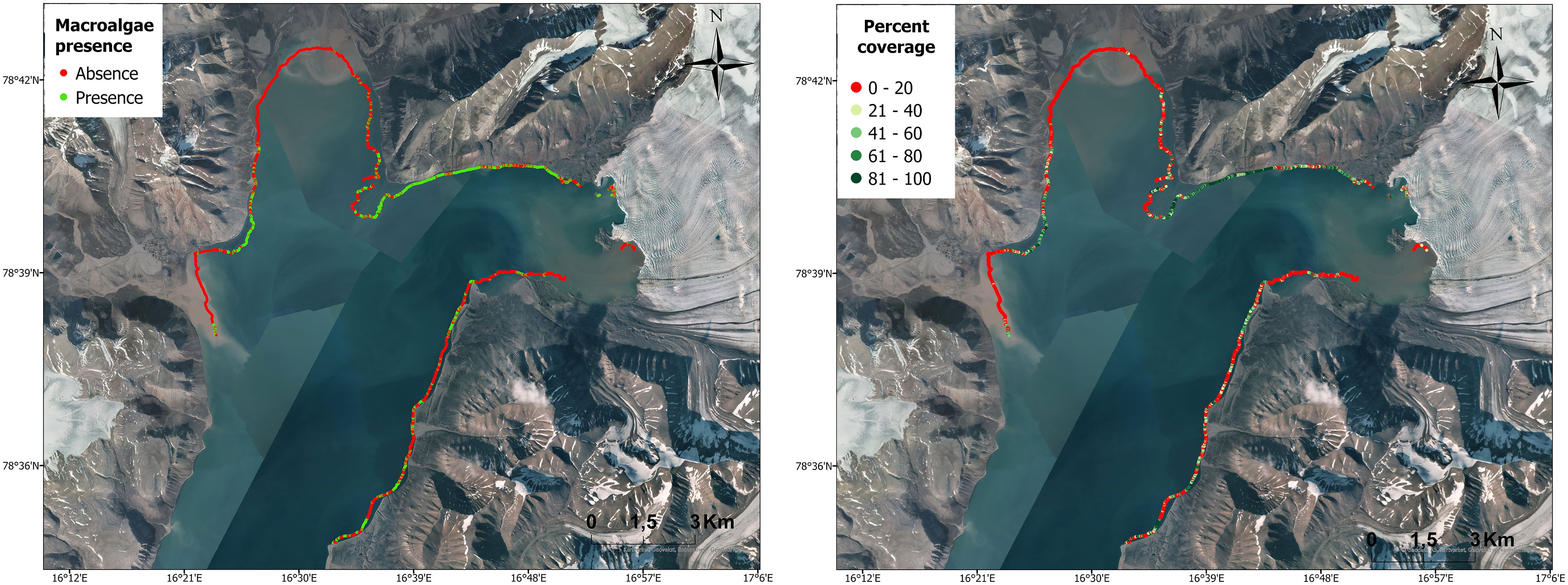
Figure 4. Macroalgal coverage along the inner coast of Billefjorden showing macroalgal presence (>50% coverage; green) and absence (<50% coverage; red) and percent coverage of macroalgae. Basemap source: Norwegian Polar Institute, Geodata AS, Kartverket, Geovekst og kommunene; Esri, USGS.
Out of 30 total camera drops, the echosounder correctly identified 15 cases of macroalgal presence and 13 of macroalgal absence, while 2 cases incorrectly identified macroalgal presence where the camera showed no coverage.
3.2 Surface CTD measurements
CTD surface turbidity measurements from the USV survey ranged from 1.7 to 497 ntu, with a mean of 17.9 ntu, temperature ranged from 3.8 to 9.2°C with a mean of 6.4°C, and salinity ranged from 7.2 to 33.2 psu with a mean of 26.6 psu (Figure 5). The highest turbidity measurements (243 – 497 ntu) were recorded at the sea-terminating glacier front, and the highest temperature (8 – 9°C) and lowest salinity (7 – 14 psu) were recorded at the southwestern river bay. The highest salinity values (31 – 33 psu) were recorded at inner Petuniabukta and along the eastern shoreline.
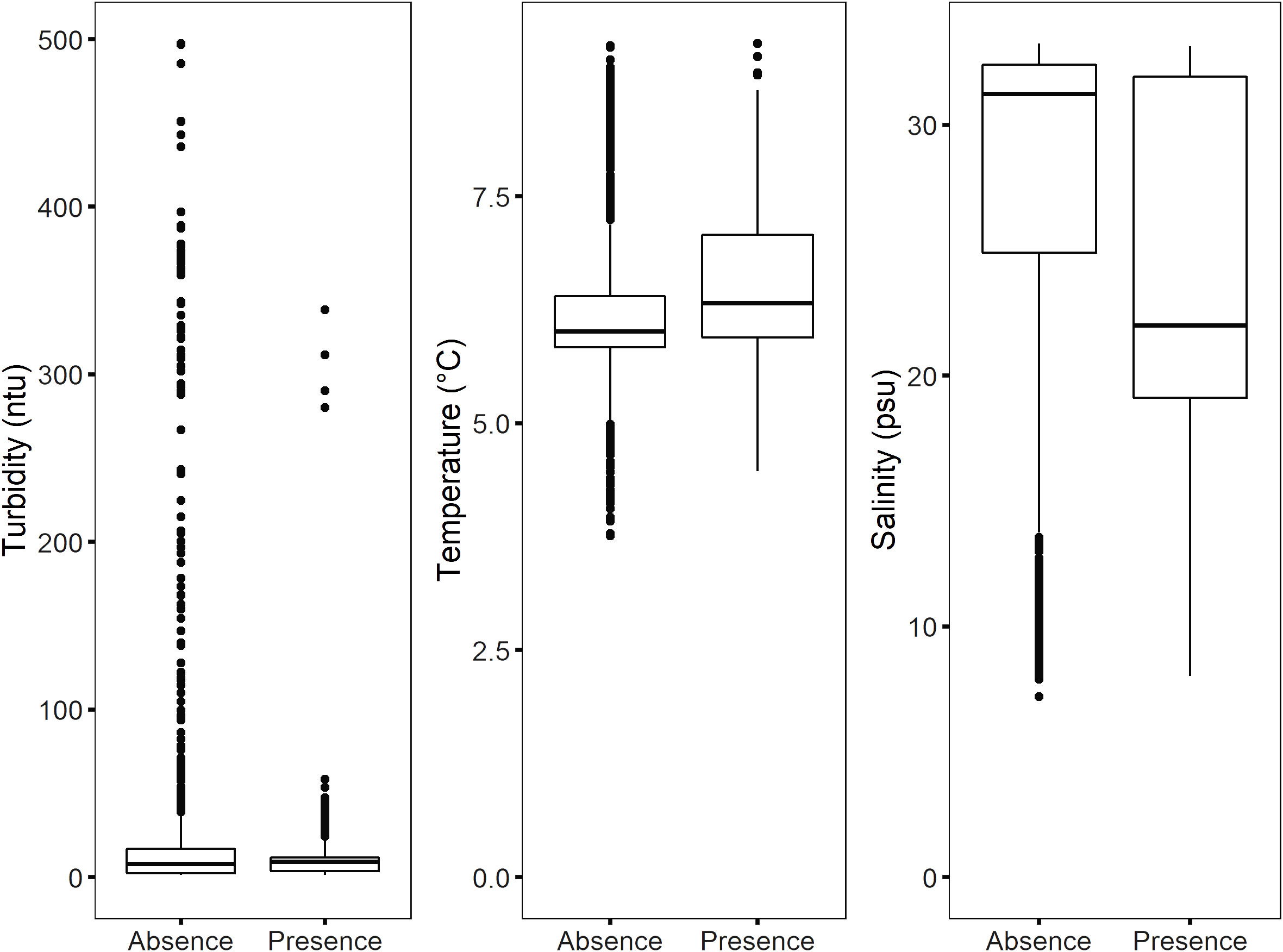
Figure 5. Boxplot showing the ranges of turbidity, temperature and salinity when macroalgae were present and absent. Each data point corresponds to the average values of 10-second intervals of CTD surface measurements. The box represents the interquartile range (IQR) and the line within indicates the median. The whiskers extend to the most extreme data points not considered outliers, which are represented by individual dots beyond the whiskers.
3.3 CTD water column profiles
The highest surface turbidity (100 ntu) was found at the glacier station, along with the lowest salinity (below 20 psu) and lowest temperature (below 8°C; Figure 6). The control station was the most saline and least turbid station, with surface values above 25 psu and below 4 ntu respectively. The river station is the shallowest and was the only station with bottom water temperatures above 0°C.
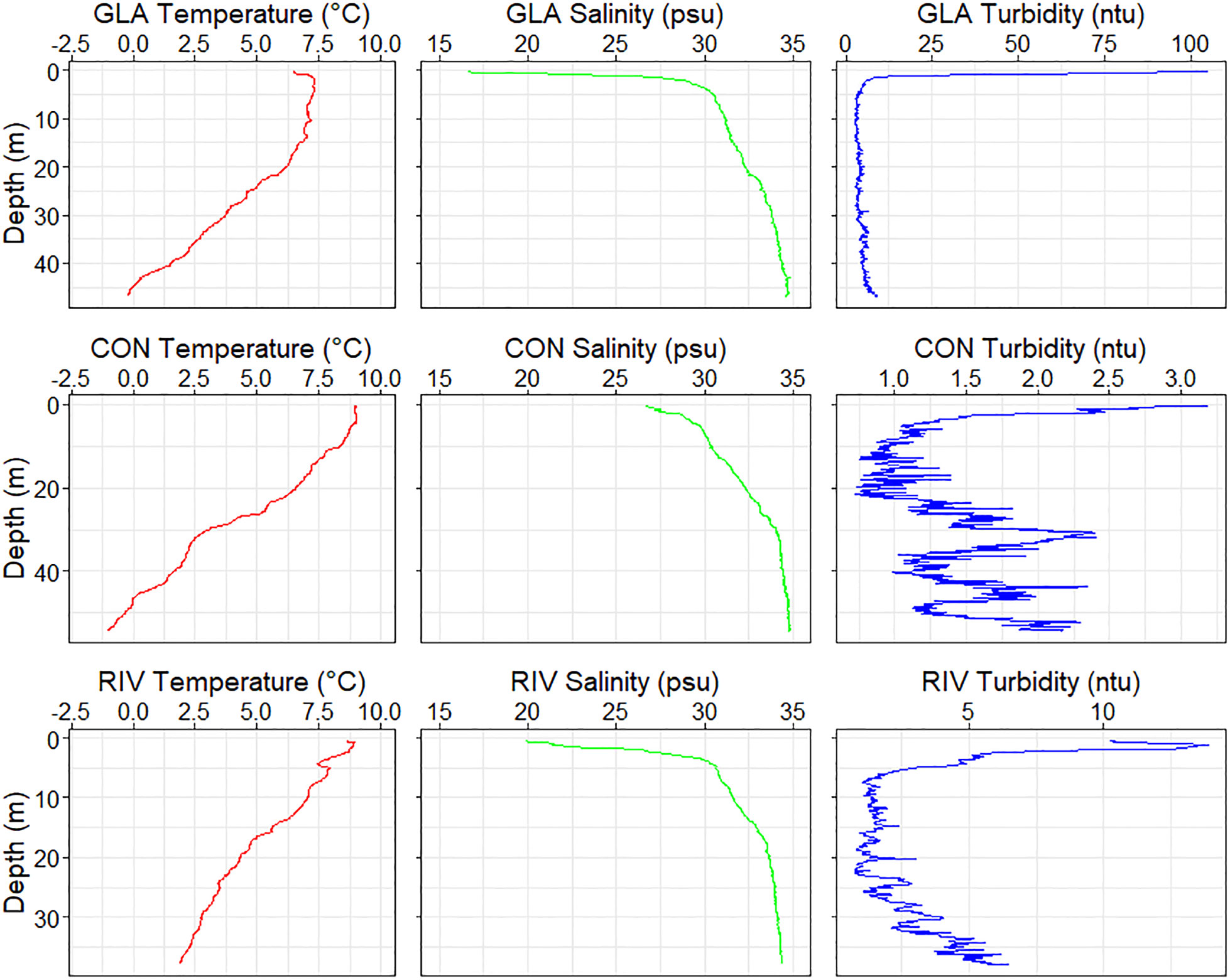
Figure 6. CTD depth profiles for the glacier (GLA), control (CON) and river (RIV) stations, with measures of temperature, salinity and turbidity. Turbidity scales are different between the three stations due to great variation in measurements.
The correlation matrix showed low correlations between turbidity and temperature and turbidity and salinity, 0.13 and -0.37 respectively, and a moderate correlation of -0.63 between temperature and salinity (Supplementary Table 2). All three predictor variables exhibited statistically significant associations with macroalgal presence in the logistic regression model (Table 1). Temperature exhibited a negative association, with a one-unit increase resulting in a 53.49% decrease in the odds of macroalgal presence, as indicated by the odds ratio (OR) of 0.465 (95% CI: 0.405, 0.535, z = -10.74, p < 0.001). Similarly, salinity displayed a negative association, with a one-unit increase corresponding to an 16.36% decrease in odds (OR: 0.836, 95% CI: 0.820, 0.853, z = -17.28, p < 0.001). Turbidity also showed a negative relationship, with a one-unit increase leading to a 3.06% decrease in odds (OR: 0.969, 95% CI: 0.961, 0.978, z =-7.19, p < 0.001). All predictors had highly significant p-values, indicating the robustness of these associations. A McFadden’s R-squared value of 0.1 was obtained for the model. Similarly to the logistic regression, all three predictors were found to be statistically significant in the inflated beta regression model (Table 2), and all predictors exhibited negative associations with macroalgal percent coverage. A one-unit increase in temperature resulted in a decrease in the expected value of macroalgal coverage, as indicated by the estimate of -0.3903 (t = -10.95, p < 0.001). Similarly, a one-unit increase in salinity corresponded to a decrease in the expected value of coverage, with an estimate of -0.0850 (t = -18.44, p < 0.001). Turbidity also showed a negative relationship, with a one-unit increase leading to a decrease in the expected value of coverage, as indicated by the estimate of -0.0066 (t = -10.71, p < 0.001).
4 Discussion
Macroalgae-dominated coastal marine ecosystems are projected to expand northwards and become more common in the Arctic due to climate change (Krause-Jensen et al., 2020; Krause-Jensen and Duarte, 2014). Despite increasing temperatures and greater availability of substrate for macroalgal growth, higher sedimentation rates from melting glaciers and the subsequent changes to the light regime can limit the expansion of macroalgae in the Arctic (Assis et al., 2022). Exploring the interactions between the different changes in oceanographical conditions and their relationships with macroalgal settling and growth is crucial to understanding the new underwater landscape in littoral areas of a changing Arctic. This study focused on the shallow sublittoral area and the middle of the macroalgal depth distribution zone on Svalbard (Bartsch et al., 2016), at an average survey depth of 7 m. We aimed at exploring these relationships further and developing a sustainable monitoring protocol for surveys in shallow littoral areas and glacier fronts. We found high variation in macroalgal coverage along inner Billefjorden, with river bays such as Petuniabukta with virtually no macroalgae and the adjacent bay (Adolfbukta) with abundant biomass. Furthermore, kelp was found at the land-terminating glacier front. The image validation of the acoustic mapping of macroalgae showed a high rate of success and points at the potential of such methodology for sustainable ecological mapping.
4.1 Macroalgal coverage in relation to temperature, salinity and turbidity
The logistic and beta inflated regression models, which studied the relationships between environmental conditions and macroalgal presence/absence and percent coverage respectively, showed similar results indicating that temperature, salinity and turbidity significantly predict macroalgal presence, with increasing values correlated with decreasing odds for macroalgal presence and coverage. However, the low McFadden’s R-squared value indicates that the model only explains a small part of the variance, and other factors such as bottom type and nutrient availability likely play a bigger role in determining macroalgal presence. This is consistent with studies showing that macroalgae in Svalbard can thrive in wide ranges of temperature and salinity which vary seasonally (Bischof et al., 2019), and therefore these drivers are of lesser importance than substrate type and light and nutrient availability. Exposure to wave action can be a critical driver for macroalgal occurrence, but it likely does not play a major role as the coastline in inner Billefjorden is sheltered due to seasonal sea-ice cover and has low exposure to waves (Urbański and Litwicka, 2022).
Both the CTD water column profile and the drop camera footage showed high turbidity in the surface layer of the river bay Petuniabukta, which suggest high rates of run-off from the surrounding rivers. Kelp relies on rocky substrates to adhere to and grow (Steneck et al., 2002; Wulff et al., 2009) and the scarce macroalgal abundance observed in Petuniabukta may be explained by a soft bottom seafloor created by high rates of sedimentation from land, as is shown in geomorphological maps of the Billefjorden coastline (Søreide et al., 2021). Furthermore, high concentration of particles in the water and increased turbidity in the water column may have a negative effect on macroalgal growth by decreasing the available photosynthetically available radiation that reaches the bottom. Studies have shown that high sedimentation has negative effects on the germination rates of kelp by altering the recruitment of sporophytes (Traiger and Konar, 2018; Zacher et al., 2016), and the results of the acoustic survey are consistent with this area having adverse conditions for macroalgal growth. Similarly adverse conditions for macroalgal growth were expected at the glacier front, due to high sedimentation and turbidity (Szczuciński et al., 2009). The survey at the sea-terminating front was consistent with that hypothesis, as no macroalgae were detected and the surface turbidity was extremely high.
However, the hydroacoustic analyses and video validation showed considerable kelp growth on the land-terminating glacier side. This area is separated from the sea-terminating side by a small peninsula that may provide shelter from the glacial sediment plume, which combined with a rocky substrate may constitute more favourable conditions for macroalgal communities to establish. Moreover, as this section of the glacier front keeps retreating into land, there will be less mechanical disturbance from calving events. Surface turbidity measurements from the USV survey were low, while the water column profile taken further from the shore indicated more turbid surface waters, which supports that the northern, land-terminating side of the glacier may be sheltered from the sediment plume that originates at the sea-terminating side. It is of note that the drop camera footage showed a high amount of sediment on the kelp, which indicates that despite some degree of sheltering, the land-terminating side does nonetheless have appreciable sedimentation rates. The presence of macroalgae may indicate that it can thrive in areas with high sedimentation if there is enough light availability and suitable substrate. In such cases we would expect a shift in the depth range of kelp towards shallower areas, which is consistent with recent findings (Niedzwiedz and Bischof, 2023). It is important to note that environmental conditions affect macroalgae throughout the growth period, and therefore variability in temperature, salinity and turbidity over time plays a key role. Although constrained over time, the observations of oceanographic parameters in this study are representative of the summer and fall period of the year when inner Billefjorden is not ice-covered.
4.2 Ecological implications of macroalgal expansion at retreating glacier fronts
As a glacier retreats, new rocky bottom is exposed that can potentially be exploited by macroalgal communities. Furthermore, as the glacier continues to retreat and sedimentation rates and mechanical disturbances decline, the conditions will become more favourable for macroalgae and will potentially lead to higher abundance of kelp. Satellite imagery shows that the northern section of the Nordenskiöldbreen glacier became partly land-terminating in 2017-2018 (Sentinel Playground, Sinergise LTD), which implies a potential colonisation time by macrophytes of just a few years once the substrate becomes available. An acoustic survey study by Kruss et al. (2017) mapping macroalgae in Kongsfjorden, Svalbard similarly observed macroalgae close to the glacier front, and it may become common throughout the Arctic following rapid glacial retreat.
Kelp beds are important ecosystem engineers, playing a key role in nutrient cycling and energy transfer and provide shelter and substrate for many associated species (Teagle et al., 2017; Włodarska-Kowalczuk et al., 2009). Furthermore, macroalgae act as nursery areas for fish and facilitate complex interactions and trophic linkages (James and Whitfield, 2023), and play a key role in carbon cycles in coastal areas by storing CO2 in their tissues, which is transferred through the food web (Chung et al., 2011). Glacier fronts are highly productive due to sub-glacial upwelling circulating bottom nutrient-rich water to the surface (Vonnahme et al., 2021), but this process will disappear as sea-terminating glaciers retreat into land, to be replaced by assemblages driven by the establishment of kelp as biogenic habitats. Macroalgal expansion in Arctic fjords may therefore result in an enhanced local carbon sink as well as an increase in local primary production. As this study has shown, macroalgal communities are rapidly establishing in retreating glacier fronts, and this may cause a shift from a top-down phytoplankton-dominated primary production to a bottom-up production based on kelp. Hence, as glacier fronts throughout the Arctic retreat and macroalgae settle in this new substrate, there is a potential for large scale implications and dramatic changes in the underwater landscape of Arctic fjord coastal systems.
4.3 Hydroacoustics and remote vehicles as mapping tools
Hydroacoustic mapping is highly efficient for surveying and monitoring submerged vegetation, as it can be automated and cover large areas. This methodology allows to survey very shallow areas and glacier fronts, where it is very difficult and dangerous to conduct traditional sampling. Due to this, it was possible to sample at the very edge of the glacier front, and survey these newly uncovered areas for the first time. However, image validation is essential as the acoustic properties of every site will differ according to environmental variables such as weather conditions and bottom and vessel types. Nonetheless, our results demonstrate that validating acoustic data with underwater video and performing manual corrections can result in an efficient mapping of macroalgae. The main challenge encountered was the disturbance of the acoustic signal due to waves and steep bottom slopes, which can affect echo shapes and acoustic backscatter (von Szalay and McConnaughey, 2002). Furthermore, acoustic mapping may not distinguish dead from living macroalgae, and in areas with very low macroalgal presence, such as inner Petuniabukta, the occasional incorrect reports of vegetation most likely refer to drift material, which also appeared in the validation footage. To avoid misidentification of detached kelp as macroalgal coverage, we imposed a threshold of >50% cover to determine the presence of dense kelp beds and filter out drift material. However, where macroalgae coverage was patchy and the camera showed only partial coverage, the algorithm also reflected that patchiness, which indicates that this methodology can result in high resolution mapping of macroalgae.
It’s important to note that the surface CTD measurements from the USV survey offer only a snapshot of the oceanographical conditions and that this shallow water layer varies according to weather conditions and current systems in the fjord. The logistic regression model using surface CTD data in combination with the water column profiles gives a more complete picture of the conditions and how that may relate to macroalgal coverage. Moreover, this study focused on macroalgal coverage in very shallow areas and kelp may be present in deeper areas along the coast that were not covered by the acoustic beam during mapping.
This study demonstrates that macroalgal communities can rapidly establish in newly available substrate after glacial retreat, dramatically changing the coastal underwater landscape. These shifts have large scale implications as primary production and carbon cycling pathways are changing in Arctic fjords, and it is likely that these changes are taking place all throughout the Arctic. Overall, this methodology demonstrates potential for macroalgal mapping and monitoring surveys, but ground-truthing is still essential. Furthermore, such a survey is considerably more cost-efficient and sustainable than traditional methods (e.g. dredging and diving surveys) and has virtually no impact on the ecosystem. This study contributes to further understanding the links between a melting cryosphere and the expansion of macroalgae in the High Arctic, and to fill a research gap in monitoring glacier fronts and shallow littoral areas.
Data availability statement
The acoustic data generated for this study falls under confidentiality regulations of the Norwegian Mapping Authority. Requests to access the datasets should be directed to dmljdG9ydHJAdW5pcy5ubw==.
Author contributions
VT: Conceptualization, Data curation, Formal analysis, Investigation, Methodology, Software, Visualization, Writing – original draft. MB: Conceptualization, Data curation, Investigation, Methodology, Software, Visualization, Writing – review & editing. AS: Project administration, Supervision, Writing – review & editing. KB: Conceptualization, Funding acquisition, Project administration, Supervision, Writing – review & editing. BD: Conceptualization, Data curation, Funding acquisition, Methodology, Project administration, Supervision, Writing – review & editing.
Funding
The author(s) declare financial support was received for the research, authorship, and/or publication of this article. This study was financed through the FACE-IT project which has received funding from the European Union’s Horizon 2020 research and innovation programme under grant agreement No. 869154.
Acknowledgments
We thank the boat drivers and logistics personnel at the University Centre in Svalbard for their support during the data collection. We would also like to thank the reviewers for their valuable and helpful input.
Conflict of interest
The authors declare that the research was conducted in the absence of any commercial or financial relationships that could be construed as a potential conflict of interest.
Publisher’s note
All claims expressed in this article are solely those of the authors and do not necessarily represent those of their affiliated organizations, or those of the publisher, the editors and the reviewers. Any product that may be evaluated in this article, or claim that may be made by its manufacturer, is not guaranteed or endorsed by the publisher.
Supplementary material
The Supplementary Material for this article can be found online at: https://www.frontiersin.org/articles/10.3389/fmars.2024.1438332/full#supplementary-material
References
Anderson J. T., Van Holliday D., Kloser R., Reid D. G., Simard Y. (2008). Acoustic seabed classification: Current practice and future directions. ICES J. Mar. Sci. 65, 1004–1011. doi: 10.1093/icesjms/fsn061
Assis J., Serrão E. A., Duarte C. M., Fragkopoulou E., Krause-Jensen D. (2022). Major expansion of marine forests in a warmer arctic. Front. Mar. Sci. 9. doi: 10.3389/fmars.2022.850368
Bartsch I., Paar M., Fredriksen S., Schwanitz M., Daniel C., Hop H., et al. (2016). Changes in kelp forest biomass and depth distribution in Kongsfjorden, Svalbard, between 1996–1998 and 2012–2014 reflect Arctic warming. Polar Biol. 39, 2021–2036. doi: 10.1007/s00300-015-1870-1
Becker S., Walter B., Bischof K. (2009). Freezing tolerance and photosynthetic performance of polar seaweeds at low temperatures. Botanica Marina 52, 609–616. doi: 10.1515/BOT.2009.079
Bird C., Franklin E., Smith C., Toonen R. (2013). Between tide and wave marks: A unifying model of physical zonation on littoral shores. PeerJ 1, e154. doi: 10.7717/peerj.154
Bischof K., Buschbaum C., Fredriksen S., Gordillo F. J. L., Heinrich S., Jiménez C., et al. (2019). “Kelps and environmental changes in Kongsfjorden: stress perception and responses,” in The Ecosystem of Kongsfjorden, Svalbard, vol. 2. Eds. Hop H., Wiencke C. (Cham, Switzerland: Springer International Publishing), 373–422. doi: 10.1007/978-3-319-46425-1_10
Borum J., Pedersen M., Krause-Jensen D., Christensen P., Nielsen K. (2002). Biomass, photosynthesis and growth of Laminaria saccharina in a high-arctic fjord, NE Greenland. Mar. Biol. 141, 11–19. doi: 10.1007/s00227-002-0806-9
Box J. E., Colgan W. T., Christensen T. R., Schmidt N. M., Lund M., Parmentier F.-J. W., et al. (2019). Key indicators of Arctic climate change: 1971–2017. Environ. Res. Lett. 14, 045010. doi: 10.1088/1748-9326/aafc1b
Bring A., Fedorova I., Dibike Y., Hinzman L., Mård J., Mernild S. H., et al. (2016). Arctic terrestrial hydrology: A synthesis of processes, regional effects, and research challenges. J. Geophys. Res.: Biogeosci. 121, 621–649. doi: 10.1002/2015JG003131
Brown C. J., Smith S. J., Lawton P., Anderson J. T. (2011). Benthic habitat mapping: A review of progress towards improved understanding of the spatial ecology of the seafloor using acoustic techniques. Estuar. Coast. Shelf Sci. 92, 502–520. doi: 10.1016/j.ecss.2011.02.007
Carbó R., Molero A. C. (1997). Scattering strength of a gelidium biomass bottom. Appl. Acous. 51, 343–351. doi: 10.1016/S0003-682X(97)00012-1
Carlsen B. P., Johnsen G., Berge J., Kuklinski P. (2007). Biodiversity patterns of macro-epifauna on different lamina parts of Laminaria digitata and Saccharina latissima collected during spring and summer 2004 in Kongsfjorden, Svalbard. Polar Biol. 30, 939–943. doi: 10.1007/s00300-007-0272-4
Chung I. K., Beardall J., Mehta S., Sahoo D., Stojkovic S. (2011). Using marine macroalgae for carbon sequestration: A critical appraisal. J. Appl. Phycol. 23, 877–886. doi: 10.1007/s10811-010-9604-9
Deregibus D., Campana G. L., Neder C., Barnes D. K. A., Zacher K., Piscicelli J. M., et al. (2023). Potential macroalgal expansion and blue carbon gains with northern Antarctic Peninsula glacial retreat. Mar. Environ. Res. 189, 106056. doi: 10.1016/j.marenvres.2023.106056
Diehl N., Steiner N., Bischof K., Karsten U., Heesch S. (2023). Exploring intraspecific variability – biochemical and morphological traits of the sugar kelp Saccharina latissima along latitudinal and salinity gradients in Europe. Front. Mar. Sci. 10. doi: 10.3389/fmars.2023.995982
Düsedau L., Fredriksen S., Brand M., Fischer P., Karsten U., Bischof K., et al. (2024). Kelp forest community structure and demography in Kongsfjorden (Svalbard) across 25 years of Arctic warming. Ecol. Evol. 14, e11606. doi: 10.1002/ece3.11606
Fredriksen S., Bartsch I., Wiencke C. (2014). New additions to the benthic marine flora of Kongsfjorden, western Svalbard, and comparison between 1996/1998 and 2012/2013. Botanica Marina 57, 203–216. doi: 10.1515/bot-2013-0119
Fredriksen S., Gabrielsen T. M., Kile M. R., Sivertsen K. (2015). Benthic algal vegetation in Isfjorden, Svalbard. Polar Res. 34, 25994. doi: 10.3402/polar.v34.25994
Fredriksen S., Røst Kile M. (2012). The algal vegetation in the outer part of Isfjorden, Spitsbergen: Revisiting Per Svendsen’s sites 50 years later. Polar Res. 31, 17538. doi: 10.3402/polar.v31i0.17538
Gilson A. R., White L. J., Burrows M. T., Smale D. A., O’Connor N. E. (2023). Seasonal and spatial variability in rates of primary production and detritus release by intertidal stands of Laminaria digitata and Saccharina latissima on wave-exposed shores in the northeast Atlantic. Ecol. Evol. 13, e10146. doi: 10.1002/ece3.10146
Grolemund G., Wickham H. (2011). Dates and times made easy with lubridate. J. Stat. Softw. 40, 1–25. doi: 10.18637/jss.v040.i03
Gutt J. (2001). On the direct impact of ice on marine benthic communities, a review. Polar Biol. 24, 553–564. doi: 10.1007/s003000100262
Hanelt D., Tüg H., Bischof K., Groß C., Lippert H., Sawall T., et al. (2001). Light regime in an Arctic fjord: A study related to stratospheric ozone depletion as a basis for determination of UV effects on algal growth. Mar. Biol. 138, 649–658. doi: 10.1007/s002270000481
Hawkins S., Moore P., Burrows M., Poloczanska E., Mieszkowska N., Herbert R., et al. (2008). Complex interactions in a rapidly changing world: Responses of rocky shore communities to recent climate change. Climate Res. 37, 123–133. doi: 10.3354/cr00768
Henson H. C., Holding J. M., Meire L., Rysgaard S., Stedmon C. A., Stuart-Lee A., et al. (2023). Coastal freshening drives acidification state in Greenland fjords. Sci. Total Environ. 855, 158962. doi: 10.1016/j.scitotenv.2022.158962
Hop H., Wiencke C., Vögele B., Kovaltchouk N. A. (2012). Species composition, zonation, and biomass of marine benthic macroalgae in Kongsfjorden, Svalbard. Botm 55, 399–414. doi: 10.1515/bot-2012-0097
Hopwood M. J., Carroll D., Dunse T., Hodson A., Holding J. M., Iriarte J. L., et al. (2020). Review article: How does glacier discharge affect marine biogeochemistry and primary production in the Arctic? Cryosphere 14, 1347–1383. doi: 10.5194/tc-14-1347-2020
IPCC. (2022). The ocean and cryosphere in a changing climate: special report of the intergovernmental panel on climate change. 1st ed (Cambridge, UK: Cambridge University Press). doi: 10.1017/9781009157964
James N. C., Whitfield A. K. (2023). The role of macroalgae as nursery areas for fish species within coastal seascapes. Cambridge Prisms: Coast. Futures 1, e3. doi: 10.1017/cft.2022.3
Johnsen G., Ludvigsen M., Sørensen A., Aas L. (2016). The use of underwater hyperspectral imaging deployed on remotely operated vehicles—Methods and applications. IFAC-PapersOnLine 49, 476–481. doi: 10.1016/j.ifacol.2016.10.451
Karsten U. (2007). Research note: Salinity tolerance of Arctic kelps from Spitsbergen. Phycol. Res. 55, 257–262. doi: 10.1111/j.1440-1835.2007.00468.x
Kortsch S., Primicerio R., Beuchel F., Renaud P. E., Rodrigues J., Lønne O. J., et al. (2012). Climate-driven regime shifts in Arctic marine benthos. Proc. Natl. Acad. Sci. 109, 14052–14057. doi: 10.1073/pnas.1207509109
Krause-Jensen D., Archambault P., Assis J., Bartsch I., Bischof K., Filbee-Dexter K., et al. (2020). Imprint of climate change on pan-arctic marine vegetation. Front. Mar. Sci. 7. doi: 10.3389/fmars.2020.617324
Krause-Jensen D., Duarte C. M. (2014). Expansion of vegetated coastal ecosystems in the future Arctic. Front. Mar. Sci. 1. doi: 10.3389/fmars.2014.00077
Kruss A., Tęgowski J., Tatarek A., Wiktor J., Blondel P. (2017). Spatial distribution of macroalgae along the shores of Kongsfjorden (West Spitsbergen) using acoustic imaging. Polish Polar Res. 38, 205–229. doi: 10.1515/popore-2017-0009
Láska K., Witoszová D., Prošek P. (2012). Weather patterns of the coastal zone of Petuniabukta, central Spitsbergen in the period 2008–2010. Polish Polar Res. 33, 297–318. doi: 10.2478/v10183-012-0025-0
Lippert H., Iken K., Rachor E., Wiencke C. (2001). Macrofauna associated with macroalgae in the Kongsfjord (Spitsbergen). Polar Biol. 24, 512–522. doi: 10.1007/s003000100250
Ludvigsen M., Berge J., Geoffroy M., Cohen J. H., de la Torre P. R., Nornes S. M., et al. (2018). Use of an Autonomous Surface Vehicle reveals small-scale diel vertical migrations of zooplankton and susceptibility to light pollution under low solar irradiance. Sci. Adv. 4, eaap9887. doi: 10.1126/sciadv.aap9887
Mann P. J., Strauss J., Palmtag J., Dowdy K., Ogneva O., Fuchs M., et al. (2022). Degrading permafrost river catchments and their impact on Arctic Ocean nearshore processes. Ambio 51, 439–455. doi: 10.1007/s13280-021-01666-z
Niedzwiedz S., Bischof K. (2023). Glacial retreat and rising temperatures are limiting the expansion of temperate kelp species in the future Arctic. Limnol. Oceanogr. 68, 816–830. doi: 10.1002/lno.12312
Nilsen F., Cottier F., Skogseth R., Mattsson S. (2008). Fjord–shelf exchanges controlled by ice and brine production: The interannual variation of Atlantic Water in Isfjorden, Svalbard. Continental Shelf Res. 28, 1838–1853. doi: 10.1016/j.csr.2008.04.015
Ospina R., Ferrari S. L. P. (2010). Inflated beta distributions. Stat. Papers 51, 111–126. doi: 10.1007/s00362-008-0125-4
Radomski P. J., Holbrook B. V. (2015). A comparison of two hydroacoustic methods for estimating submerged macrophyte distribution and abundance: A cautionary note. J. Aquat. Plant Manag. 53, 151–159. Available online at: https://apms.org/wp-content/uploads/japm-53-02-151.pdf.
Riegl B. M., Moyer R. P., Morris L. J., Virnstein R. W., Purkis S. J. (2005). Distribution and seasonal biomass of drift macroalgae in the Indian River Lagoon (Florida, USA) estimated with acoustic seafloor classification (QTCView, Echoplus). J. Exp. Mar. Biol. Ecol. 326, 89–104. doi: 10.1016/j.jembe.2005.05.009
Ronowicz M., Włodarska-Kowalczuk M., Kukliński P. (2020). Glacial and depth influence on sublittoral macroalgal standing stock in a high-Arctic fjord. Continental Shelf Res. 194, 104045. doi: 10.1016/j.csr.2019.104045
Simmonds E. J., MacLennan D. N. (2005). Fisheries acoustics: Theory and practice. 2nd ed (Oxford, UK: Blackwell Science).
Søreide J., Pitusi V., Vader A., Damsgård B., Nilsen F., Skogseth R., et al. (2021). Environmental status of Svalbard coastal waters: Coastscapes and focal ecosystem components (SvalCoast). State. Environ. Sci. Svalbard. Rep. 2020, 143–174. doi: 10.5281/zenodo.4293849
Sperandei S. (2014). Understanding logistic regression analysis. Biochem. Med. 24, 12–18. doi: 10.11613/BM.2014.003
Steneck R. S., Graham M. H., Bourque B. J., Corbett D., Erlandson J. M., Estes J. A., et al. (2002). Kelp forest ecosystems: Biodiversity, stability, resilience and future. Environ. Conserv. 29, 436–459. doi: 10.1017/S0376892902000322
Svendsen H., Beszczynska-Møller A., Hagen J. O., Lefauconnier B., Tverberg V., Gerland S., et al. (2002). The physical environment of Kongsfjorden–Krossfjorden, an Arctic fjord system in Svalbard. Polar Res. 21, Article 1. doi: 10.3402/polar.v21i1.6479
Szczuciński W., Zajączkowski M., Scholten J. (2009). Sediment accumulation rates in subpolar fjords – Impact of post-Little Ice Age glaciers retreat, Billefjorden, Svalbard. Estuar. Coast. Shelf Sci. 85, 345–356. doi: 10.1016/j.ecss.2009.08.021
Teagle H., Hawkins S. J., Moore P. J., Smale D. A. (2017). The role of kelp species as biogenic habitat formers in coastal marine ecosystems. J. Exp. Mar. Biol. Ecol. 492, 81–98. doi: 10.1016/j.jembe.2017.01.017
Traiger S. B., Konar B. (2018). Mature and developing kelp bed community composition in a glacial estuary. J. Exp. Mar. Biol. Ecol. 501, 26–35. doi: 10.1016/j.jembe.2017.12.016
Urbański J. A., Litwicka D. (2022). The decline of Svalbard land-fast sea ice extent as a result of climate change. Oceanologia 64, 535–545. doi: 10.1016/j.oceano.2022.03.008
Visch W., Nylund G. M., Pavia H. (2020). Growth and biofouling in kelp aquaculture (Saccharina latissima): The effect of location and wave exposure. J. Appl. Phycol. 32, 3199–3209. doi: 10.1007/s10811-020-02201-5
Vonnahme T. R., Persson E., Dietrich U., Hejdukova E., Dybwad C., Elster J., et al. (2021). Early spring subglacial discharge plumes fuel under-ice primary production at a Svalbard tidewater glacier. Cryosphere 15, 2083–2107. doi: 10.5194/tc-15-2083-2021
von Szalay P. G., McConnaughey R. A. (2002). The effect of slope and vessel speed on the performance of a single beam acoustic seabed classification system. Fisheries Res. 56, 99–112. doi: 10.1016/S0165-7836(02)00020-6
Weslawski J. M., Wiktor J., Kotwicki L. (2010). Increase in biodiversity in the arctic rocky littoral, Sorkappland, Svalbard, after 20 years of climate warming. Mar. Biodivers. 40, 123–130. doi: 10.1007/s12526-010-0038-z
Wickham H., François R., Henry L., Müller K., Vaughan D. (2023).dplyr: A grammar of data manipulation. Available online at: https://dplyr.tidyverse.org. (accessed August 20, 2024).
Wiktor J. M., Tatarek A., Kruss A., Singh R. K., Wiktor J. M., Søreide J. E. (2022). Comparison of macroalgae meadows in warm Atlantic versus cold Arctic regimes in the high-Arctic Svalbard. Front. Mar. Sci. 9. doi: 10.3389/fmars.2022.1021675
Włodarska-Kowalczuk M., Kukliński P., Ronowicz M., Legeżyńska J., Gromisz S. (2009). Assessing species richness of macrofauna associated with macroalgae in Arctic kelp forests (Hornsund, Svalbard). Polar Biol. 32, 897–905. doi: 10.1007/s00300-009-0590-9
Wulff A., Iken K., Quartino M. L., Al-Handal A., Wiencke C., Clayton M. N. (2009). Biodiversity, biogeography and zonation of marine benthic micro- and macroalgae in the Arctic and Antarctic. Botm 52, 491–507. doi: 10.1515/BOT.2009.072
Keywords: algae, autonomous vehicles, coastal mapping, climate change, hydroacoustics, Svalbard
Citation: Gonzalez Triginer V, Beck M, Sen A, Bischof K and Damsgård B (2024) Acoustic mapping reveals macroalgal settlement following a retreating glacier front in the High Arctic. Front. Mar. Sci. 11:1438332. doi: 10.3389/fmars.2024.1438332
Received: 25 May 2024; Accepted: 22 August 2024;
Published: 10 September 2024.
Edited by:
Bernardo Antonio Perez Da Gama, Fluminense Federal University, BrazilReviewed by:
Jan Marcin Weslawski, Polish Academy of Sciences, PolandTrine Bekkby, Norwegian Institute for Water Research (NIVA), Norway
Copyright © 2024 Gonzalez Triginer, Beck, Sen, Bischof and Damsgård. This is an open-access article distributed under the terms of the Creative Commons Attribution License (CC BY). The use, distribution or reproduction in other forums is permitted, provided the original author(s) and the copyright owner(s) are credited and that the original publication in this journal is cited, in accordance with accepted academic practice. No use, distribution or reproduction is permitted which does not comply with these terms.
*Correspondence: Victor Gonzalez Triginer, dmljdG9ydHJAdW5pcy5ubw==