- 1Geological Oceanography Division, CSIR-National Institute of Oceanography (NIO), Goa, India
- 2National Centre for Polar and Ocean Research (NCPOR), Ministry of Earth Sciences, Goa, India
- 3Department of Geology, Patna University, Bihar, India
Metal contamination in the Arctic region has increased over the years despite its remote and isolated location. Thus, to evaluate the bioavailable fractions of various metals and their effects on sediment-associated biota, the surface sediments from the fjords were analyzed for bulk concentration of metals and their speciation in different fractions. Metals concentrations were higher in the inner fjord region and decreased towards the outer fjord, supported by the terrigenous influence (TI%) calculated. Cr and Pb showed higher excess values attributed to their additional source other than the catchment rocks. So, to assess the metal-related ecological risk, the bulk concentration of metals was compared with Arctic sediment quality guidelines (ASQGs). Cr, Cd, and Pb concentrations were high, indicating potential adverse biological effects in the study. To avoid the risk of overestimation, metal speciation was conducted, showing that overall metal concentrations were higher in the residual fraction; however, higher concentrations of Mn in labile phases pose a moderate risk to the sediment-associated biota. Additionally, the population density of foraminifera in the sediments was calculated to assess the influence of bioavailable metal on benthic foraminifera. It was found that the presence of metals in bioavailable fractions affected the abundance of the foraminifera. However, no morphological abnormalities were observed in the species.
1 Introduction
The rise in atmospheric temperature in response to increasing greenhouse gas concentrations has led to the “Arctic amplification” phenomenon. Consequently, a significant reduction of sea ice extent and a large volume of fresh water is exported into the Arctic fjords. These fjords are located at the junction of the ocean, atmosphere, terrestrial, and cryospheric domains and are very sensitive to changes in climate (Bianchi et al., 2020). The source of freshwater in these Arctic fjords is primarily the marine-terminating/tidewater glaciers. The glacial meltwater runoff dominates these glaciers and regulates the primary productivity and terrestrial material flux influencing the delivery of metals accumulated in glacier ice to these fjords over the last century. Moreover, the West Spitsbergen Current (WSC) is gaining strength, transporting warm water to the fjords, responsible for the long-range transportation of significant soluble metals such as Cd and Pb (Maccali et al., 2013).
Along with the interplay of glacial fresh water and seawater, physicochemical variables such as pH, Eh, salinity, and dissolved oxygen determine the fate/form of metals in the sediments. The metals derived from different sources are adsorbed onto sorbent phases and preserved in the sediment. Due to changing chemical and hydrological conditions, metals undergo geochemical modifications and are recycled via sediment resuspension into the water column (Gibson et al., 2015; Zhang et al., 2014; Burton et al., 2006). Thus, sediments act as the sink and source of metals in the marine environment, and they play an essential role in transporting and accumulating metals.
Environmental pollution in the northern polar region has been studied for several decades (Poland et al., 2003; Barrie, 1986; Ottar, 1989); however, increasing varieties of toxic contaminants (such as pesticides and industrial chemicals) have been reported in the Arctic in recent years (Dietz et al., 2022). This indicates that pollutants from around the globe are being transported to the Arctic region by wind, sea ice, and long-range oceanic and atmospheric transport (Li et al., 2018). Due to the extreme environmental conditions in the Arctic, such as frigid temperatures, the contaminants accumulate and degrade slowly compared to tropical environments (Chen et al., 2018). Metal accumulation and their extended existence in this area may affect marine organisms, fish, birds, and mammals (Jakimska et al., 2011; Kock et al., 1996; Akeredolu et al., 1996) and sediment-associated benthic communities. Benthic foraminifera is less mobile and affected by natural and anthropogenic processes (Martins et al., 2019; Schintu et al., 2015) and variations in metal concentrations. Numerous researches have been carried out to assess the effect of metal pollution on benthic foraminifera (Alve, 1995; Yanko et al., 1994, 1998; Samir and El-Din, 2001; Geslin et al., 2002; Bergin et al., 2006; Ferraro et al., 2006; Jayaraju et al., 2008; Frontalini et al., 2009; Mendes et al., 2013; El Kateb et al., 2020; Gildeeva et al., 2021). Samir and El-Din (2001) reported that high concentrations of metals like Pb, Cu, Cr, Cd, and Zn deformed the foraminifera tests. Lintner et al. (2021) suggested that higher content of Mn and Zn ceased the growth of foraminifera. The population density and diversity of foraminifera are affected by increased metal pollution (Bergin et al., 2006; Debenay and Fernandez, 2009; Saalim et al., 2017; Barik et al., 2022) and developed morphological abnormalities (Frontalini and Coccioni, 2008). However, the studies on the impacts of metal concentrations on foraminiferal population and density in different geochemical phases are limited. Numerous studies have used bulk concentration to evaluate toxicity; however, the leachability of metals changes with varying environmental parameters, leading to an overestimation of the ecological risk associated. Therefore, the speciation of metals in different geochemical phases has been carried out to avoid the risk of overestimation and to assess the mobility, bioavailability, and toxicity of metals and their influence on benthic fauna. With this background, the objectives are i) to study the source and spatial variations of the metals in different fjords, ii) to assess the mobility, bioavailability, and toxicity of metals in different geochemical fractions, and iii) their influence on benthic fauna in the surface sediments of Arctic fjords.
2 Materials and methodology
2.1 Study area, sample collection, and storage
Sixteen surface sediment samples have been collected from the high Arctic fjords such as northernmost Raudfjord, Magdalenefjord, and St. Jonsfjord, situated on the west coast of Spitsbergen, Svalbard, Arctic (74 and 81°N and 10 to 28°E) (Figure 1). The sediment samples have been collected from various water depths between 20 and 115 m from the inner fjord to the outer fjord (Figure 1). Sediment samples were collected onboard RV Clione in July 2019 using a Van Veen grab sampler. The sediment samples were packed in clean and labeled plastic bags and stored at 4°C.
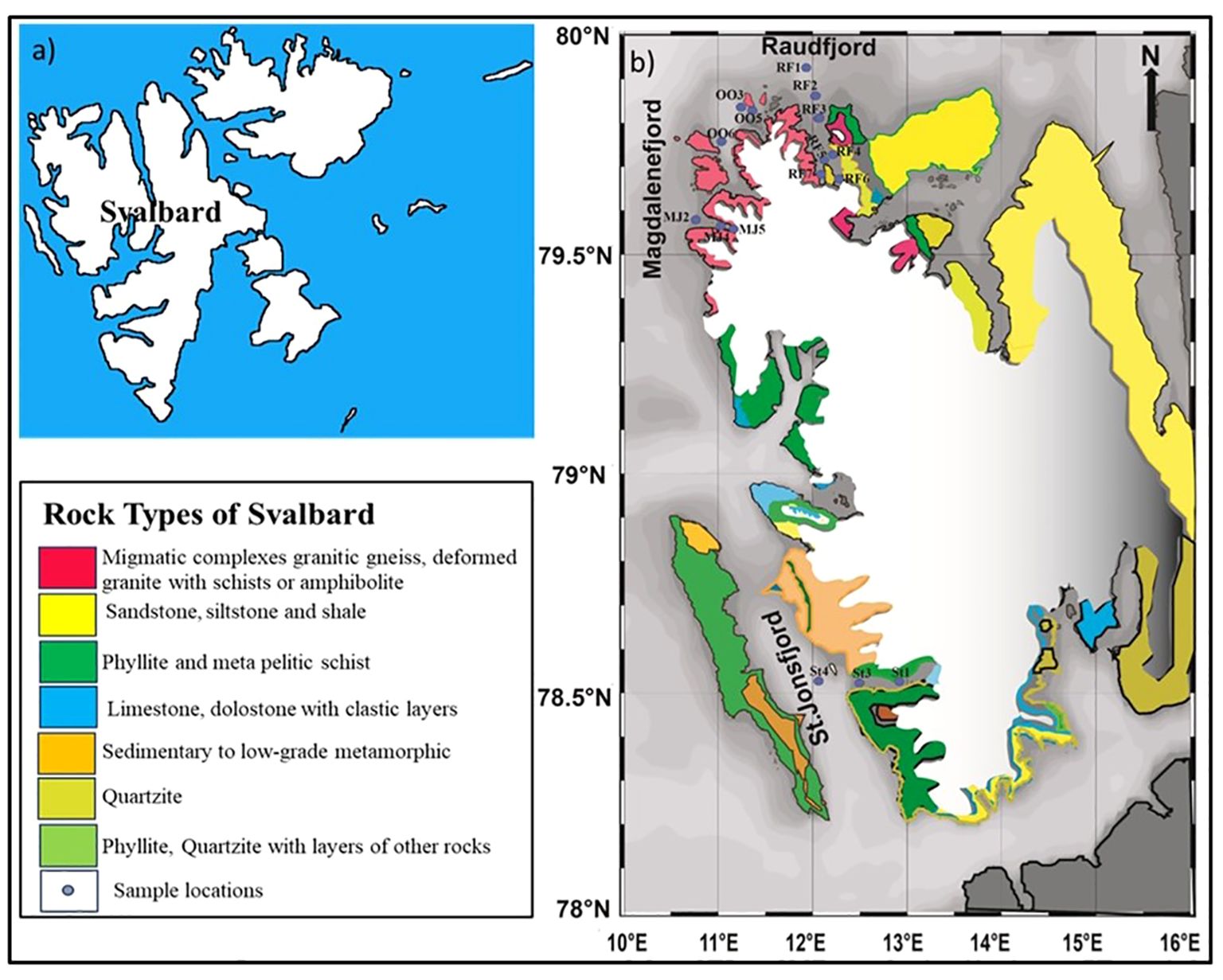
Figure 1. (A) Map showing the location of Svalbard (B) sampling locations in Raudfjord, Magdalenefjord, St. Jonsfjord and the open ocean in Svalbard.
2.2 Foraminiferal analysis
The surface sediment samples have been used to study the modern distribution of (living and dead) benthic foraminifera. The samples were divided into two halves, one half was stained onboard using rose-Bengal ethanol solution (2 g rose-Bengal in 1 l of ethanol) to identify living foraminifera, and the other half unstained was used for dead foraminifera. The rose-Bengal stained samples were stored for a minimum of two weeks and then processed following the standard sediment processing procedure (Saalim et al., 2022b). The samples were freeze-dried, weighed, and washed using a 63 μm sieve. The >63 μm sieved material (coarse fraction) was dried and stored in plastic vials. A small aliquot of the dried coarse fraction was weighed and used to pick living and dead benthic foraminifera. A minimum of 300 stained specimens were picked from each sample using a stereo-zoom microscope (Nikon SMZ 1500) and mounted on the micropaleontological slides. The relative abundance of each species was counted in all the surface samples. Foraminiferal density (FD) is the total number of foraminifera (live + dead) per gram of dry sediments (Barik et al., 2019).
2.3 Elemental analysis
A minimum of 1 g dry sediment was powdered and homogenized in a mortar pestle for the total carbon (TC), nitrogen (TN), and inorganic carbon (TIC) analysis. TC and TN were analyzed by using an elemental analyzer (Isoprime, Vario Isotope Cube). The precision for TC ± 0.3% and ± 0.63% (1σ standard deviation) for TN% was obtained by repeatedly running sulphanilamide as the standard. The inorganic carbon was measured by using a UIC CM 5017 coulometer. The organic carbon (Corg) was estimated by subtracting TIC from TC. The total phosphorus was determined following the procedure given by Murphy and Riley (1962). The accuracy of phosphorus analysis was determined using a digested sample of JLK-1 and relative error was noted to be less than 4%. The biogenic silica (BSi) was extracted following the wet alkaline extraction method, modified by Mortlock and Froelich (1989) and Muller and Schneider (1993). Each sample was measured in duplicate, with the relative error less than 3%.
2.4 Geochemical analysis
Further, the samples were transported to the laboratory and freeze-dried for further analysis.
For estimating bulk element concentration, sediment samples were finely ground and digested (Jarvis and Jarvis, 1985). 0.2 g of homogenized sediment sample was taken in acid-washed Teflon beakers. To this, a mixture of 10 ml HF–HClO4–HNO3 in the ratio of 7:3:1 was added, and the solution was completely digested on a hot plate at 150°C. If any particle persisted in the solution, the digestion steps were repeated to obtain a clear solution. The solution was then diluted up to 50 ml and preserved in a cool and dark place. The concentrations of trace elements were determined using Inductively Coupled Plasma Optical Emission Spectrometry (ICP-OES) (Thermo scientific iCAP 7000 series). Further, the salt-free and decarbonated sediments were analyzed for major elemental oxides using an X-ray fluorescence (XRF).
Sequential extraction of metals such as Fe, Mn, Co, Cr, Cu, Ni, Pb, Cd, and Zn was performed using the European Community Bureau of Reference (BCR) procedure (Supplementary Figure S1) (Lu and Kang, 2018). In this procedure metals have been extracted into four geochemical fractions viz. i) acid exchangeable phase (F1), ii) Fe-Mn oxide (F2, reducible phase), iii) organic matter/sulfide (F3, oxidizable) bound, and iv) residual phase (F4). The modified BCR method efficiently extracts trace metals like Cr, Cu, Pb, and Zn validated by certified reference material BCR-701, freshwater sediment, from the European Commission. In this study, metals like Fe, Mn, Co, Cr, Cu, Ni, Pb, Cd, and Zn have been considered for fractionation as these metals can pose a considerable ecological risk to the sediment-associated biota. All the extracted fractions were measured using ICP-OES (Thermo scientific iCAP 7000 series).
2.5 Quality assurance and quality control
All the glassware and labware used during the digestion and speciation were soaked in nitric acid and rinsed with Mili Q water thoroughly. During the extraction procedure, the blank was analyzed, and after every ten samples analysis was repeated to ensure the precision of the results, which was within ± 6%. The samples were spiked with known multielement standard solutions to validate the analysis. All the reagents used during the analysis were suprapure. With the samples, a certified reference standard (NIST-1646A, National Institute of Standards and Technology), US, was digested and run, to test the analytical accuracy of the bulk metal analysis method, which varied from 94.5% to 98.7%. Further, recovery (%) of the extracted metals is calculated by [(F1+F2+F3+F4)/total concentration]∗100 (Lu and Kang, 2018), and recovery is between 95 and 103% for all the extracted metals.
2.6 Data analysis using statistical methods
Principal component analysis was performed to assess the impact of metals in different fractions on the benthic foraminifera. The PCA was performed on different metal fractions as primary variables and foraminifera genus as secondary variables using the Statistical software (version 5.5), Statsoft. The three axes, axis 1 (Eigen value 3.26), axis 2 (Eigen value 2.52), and axis 3 (Eigen value 0.90) account for 50.68%, 15.53%, 12.25% of the total variation in the data, respectively making a total of 78.46%. On the biplot, the length of the arrow indicates the intensity of the metal fractions. Foraminifera genus are projected orthogonally on the arrows to understand the relationship between the metals and the foraminifera. The angle between the arrows suggests the correlation. Some figures have been plotted in the Ocean data view (Schlitzer, 2023).
2.7 Pollution load index
Pollution Load Index (PLI) is the aggregate of all contamination factors and the overall level of metal contamination in sediments. PLI >1 suggests metal contamination, whereas <1 indicates no metal pollution (Tomlinson et al., 1980; Choudhary et al., 2023; Salazar–Rojas et al., 2023).
3 Results
3.1 Elemental composition (Corg, TN, TP, and BSi)
The concentration of organic elements (Corg, TN, TP, and BSi) is provided in Figure 2. Among the organic elements, Corg ranges from 0.71 ± 0.43% to 1.13 ± 0.69% (avg. 0.96 ± 0.40%) (Figure 2). Corg concentration varied from 1.09% to 2.83% (Winkelmann and Knies, 2005; Koziorowska et al., 2016; Kumar et al., 2016; Choudhary et al., 2018a; Limoges et al., 2018; Saalim et al., 2022a) in the west Spitsbergen fjords which are higher than the Corg content in the study area. Organic carbon content generally increased in almost all fjords from the inner to the outer fjord. TN varied from 0.12 ± 0.02% to 0.19 ± 0.10% (avg. 0.15 ± 0.06) and showed a trend like Corg (Figure 2). Total Nitrogen (TN) concentration ranged from 0.12% to 0.28% (Winkelmann and Knies, 2005; Koziorowska et al., 2016; Choudhary et al., 2018a), in the west Spitsbergen fjords which is higher than the TN content reported in the study area. TP is almost constant at 0.02 ± 0.00% for all the samples (Figure 2). BSi ranges from 3.26 ± 0.40% to 5.61 ± 1.36% (avg. 5.06 ± 1.33%) (Figure 2). These values agree with concentrations from other high-Arctic fjords, such as the Young Sound-Tyrolefjord, Krossfjord and Kongsfjord, where the average value is almost similar (Ribeiro et al., 2017; Choudhary et al., 2018b; 2020).
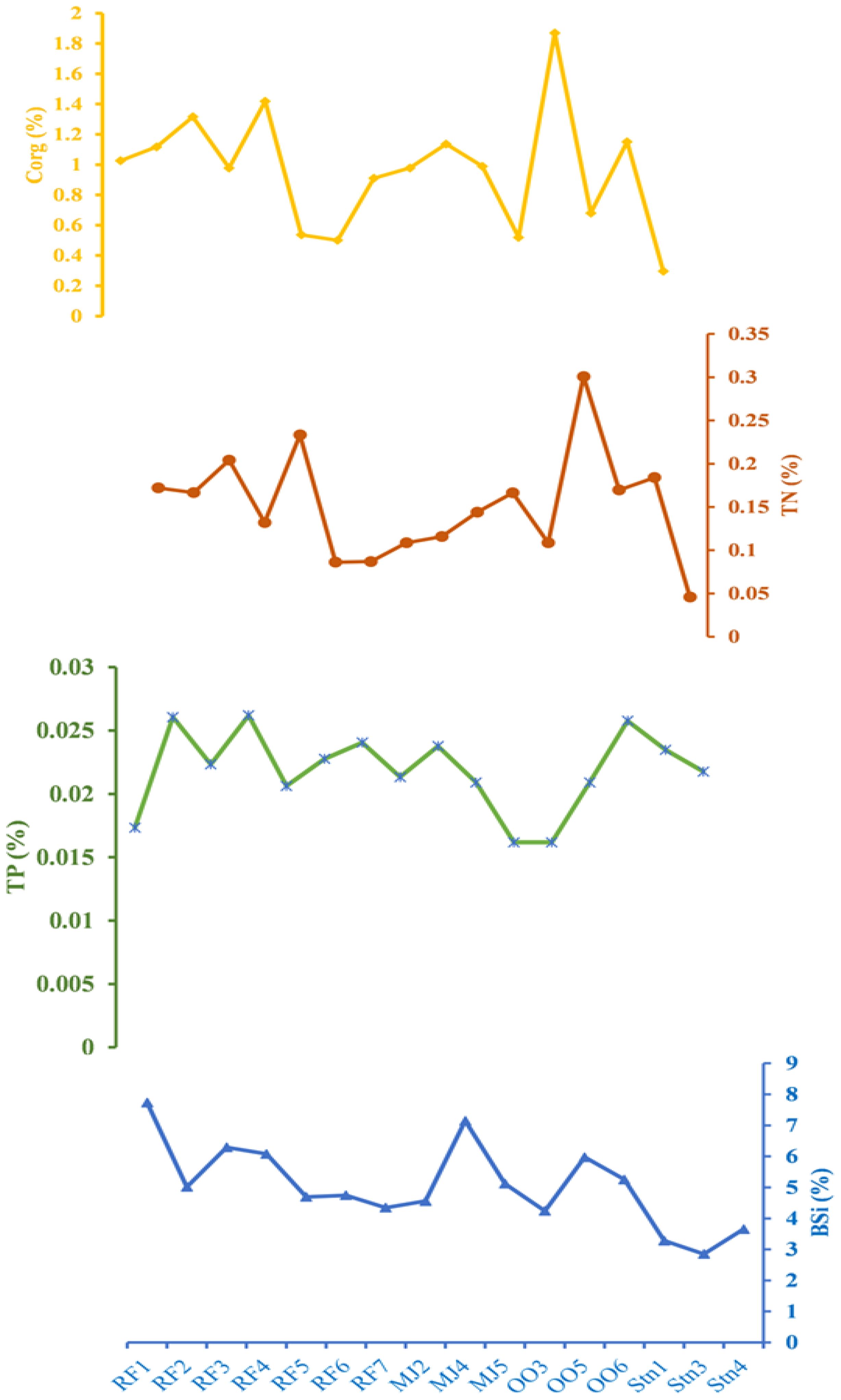
Figure 2. Spatial variations of organic components Corg (%), TN (%), TP (%), BSi (%) in the sediment of Raudfjord, Magdalenefjord and St. Jonsfjord and open ocean.
3.2 Major Oxides and trace elements in fjord sediments
The major oxide concentrations of the sediments from the northernmost Raudfjord, Magdalenefjord, and St. Jonsfjord have been analyzed. The bulk sediment composition indicates primarily a felsic source based on SiO2 (66.41 wt.%) and Al2O3 (16.23 wt.%) abundances. The SiO2 and Al2O3 abundances are lower than the upper continental crust (UCC) (Taylor and McLennan, 1995; McLennan, 2001). The rest of the major oxides in descending order of concentration are Fe2O3> MgO> K2O>CaO> Na2O>P2O5 and MnO with abundances of 5.39, 3.74, 2.98, 2.89, 1.44, 0.17 and 0.06 wt.% respectively. Among these oxides, most of them (SiO2, TiO2, MnO, K2O) have concentrations like average UCC values except for Na2O (1.76 wt.%), which is approximately half of the UCC value (3.25 wt.%). In contrast, MgO shows a higher abundance (3.63 wt.%) in the sediments of fjords, approximately double as compared to the UCC value (1.33 wt.%).
The Fe concentration of the fjord sediments ranges from 1.38 ± 0.52 to 2.39 ± 0.82%, with an average concentration of 2.14 ± 0.71% (Table 1; Figure 3A). The Mn concentration varies from 0.02 ± 0.01 to 0.04 ± 0.01% with an average concentration of 0.04 ± 0.01%. Cr concentration ranges from 25.98 ± 13.98 to 74.36 ± 13.07 ppm with an average concentration of 51.14 ± 21.96 ppm lower than the UCC value (Table 1). Co concentration ranges from 6.02 ± 3.28 ppm to 13.69 ± 4.99 ppm with an average concentration of 11.56 ± 4.65 ppm (Figure 3B). The Cu concentration varies from 2.53 ± 0.92 to 7.40 ± 2.74 with an average concentration of 5.12 ± 2.24 ppm, which is very low compared to the UCC values. The Zn concentration varied from 45.79 ± 13.30 ppm to 89.63 ± 31.30 ppm with an average concentration of 71.24 ± 23.92 ppm. Pb concentration ranges from 10.06 ± 2.08 to 24.66 ± 12.87 ppm with an average concentration of 15.29 ± 9.65 ppm. Their concentrations are comparable to the UCC values (Table 1). Cd concentration ranges from 1.77 ± 0.85 to 4.11 ± 1.53 ppm with an average concentration of 3.30 ± 1.35 ppm, two to three times lower than UCC values. Sr and Ba concentration varies from 178.55 ± 67.07 and 316.20 ± 43.87 to 286.39 ± 209.03 and 389 ± 50.63 ppm with an average concentration of 245.51 ± 149.53 and 354.78 ± 83.81 ppm, respectively, which is compared lower than average crustal abundance (350 and 550 ppm respectively, Taylor and McLennan, 1995; McLennan, 2001).
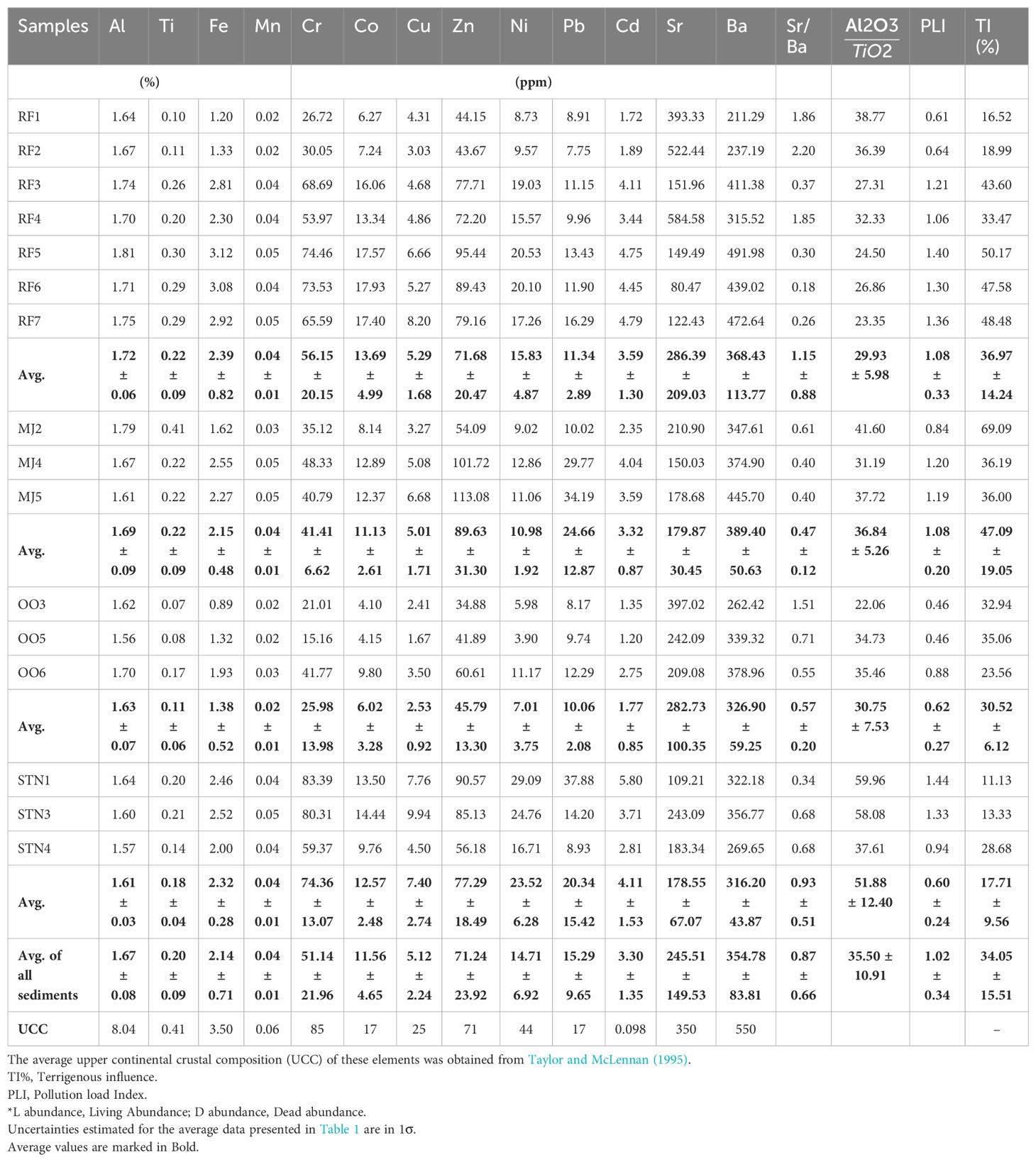
Table 1. Elemental concentrations, pollution load Index and terrigenous influence (%) in Raudfjord, Magdalenefjord and St. Jonsfjord and the open ocean.
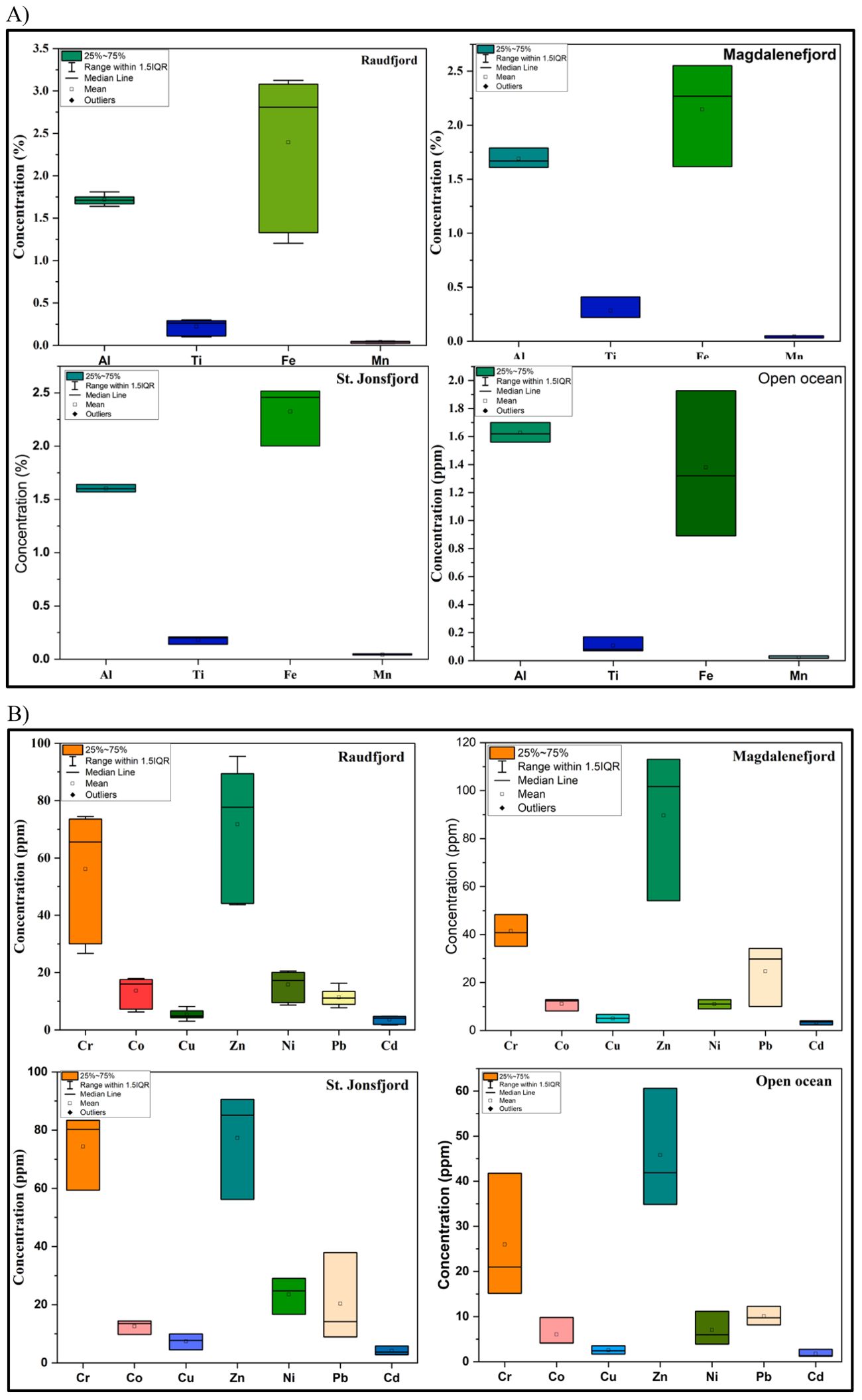
Figure 3. (A) Major such as Al, Ti, Fe and Mn and (B) Trace elements such as Cr, Co, Cu ,Ni, Zn, Pb and Cd in the sediment of Raudfjord, Magdalenefjord and St. Jonsfjord and open ocean.
3.3 Terrigenous (TI%) and non-terrigenous (Excess) influence in sediments
To determine the terrigenous matter percentage available in the sediments, titanium (Ti) concentration can be used (Schroeder et al., 1997; Sensarma et al., 2017). Ti is mostly considered detrital with a composition similar to Post‐Archean Australian Shale (PAAS; Taylor and McLennan, 1985) and is the most conservative element under changing physicochemical conditions. To determine the terrigenous influence percentage (TI%) of sediments, the following equation has been used:
————————— (Schroeder et al., 1997)
where the concentration of Ti in PAAS is 0.6 (wt.%).
Thus, a higher percentage of the terrigenous influx in a given area is attributed to more terrigenous influence. The highest values of terrigenous percentage were reported from the inner fjords, and the lowest values were reported from the outer fjords (Table 1; Figure 4) suggesting higher terrigenous supply in the inner fjord region or it is diluted in the outer fjord region.
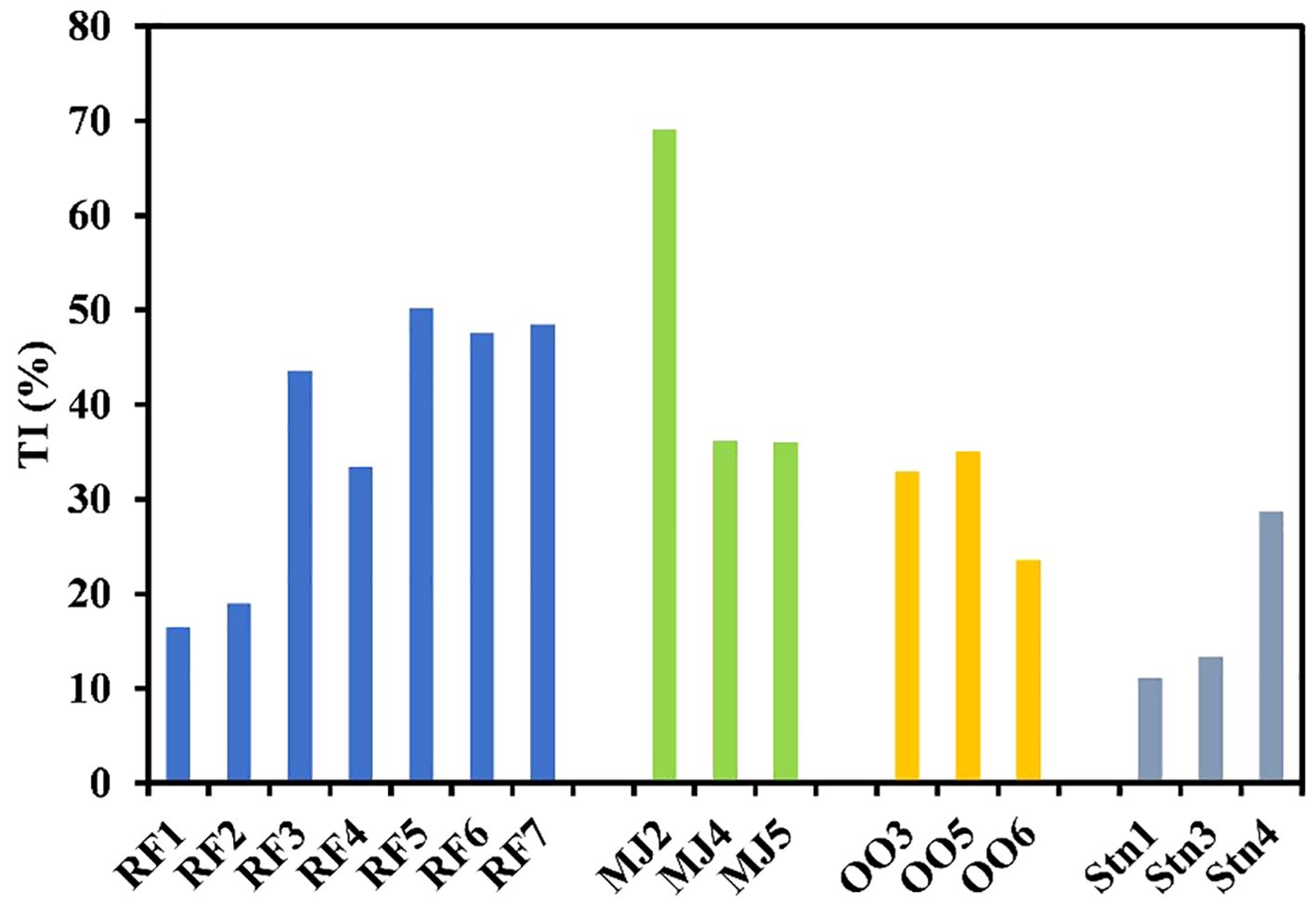
Figure 4. Terrigenous influence (TI%) in sediment in Raudfjord, Magdalenefjord and St. Jonsfjord and open ocean.
The amount of an element not supported by the structurally bound detrital terrigenous fraction or derived from sources other than terrestrial influx is called “excess,” i.e., non-terrigenous fraction, and evaluated using the equation proposed by Murray and Leinen (1996).
———————— Schroeder et al. (1997)
In the present study, Cu and Ni showed negative excess values in all the fjords at all the stations suggesting their detrital source. Fe, Mn, and Co showed very low excess values indicating a minor contribution from another source besides terrigenous (Figure 5).
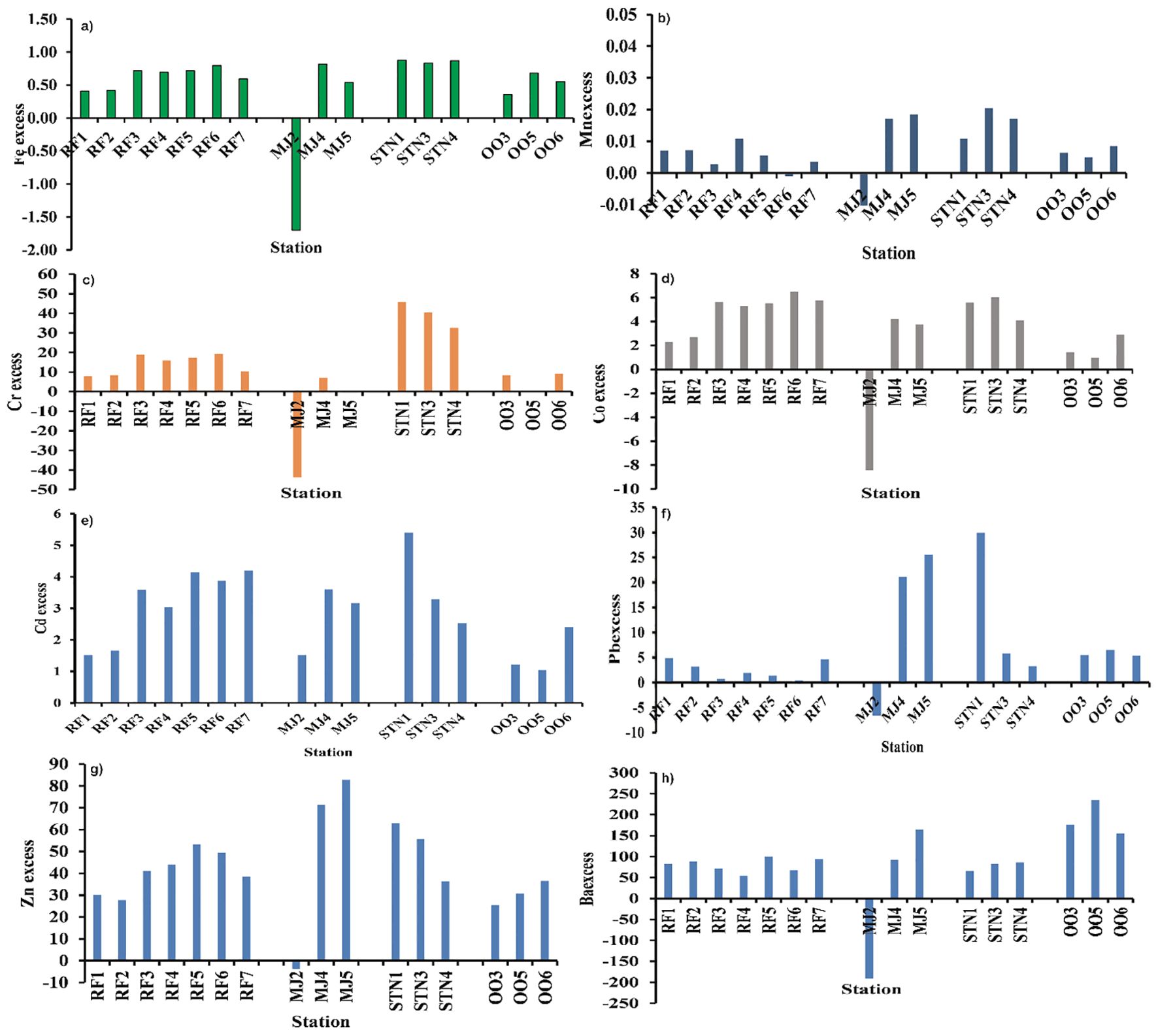
Figure 5. (A) Fe excess, (B) Mn excess, (C) Cr excess, (D) Co excess, (E) Cd excess (F) Pb excess (G) Zn excess and (H) Ba excess in sediments of Raudfjord, Magdalenefjord, St. Jonsfjord and open ocean.
3.4 Speciation of metals
The fractionation of metals Fe, Mn, Cr, Co, Cu, Zn, Ni, Pb, and Cd varied spatially in different fractions (F1 to F4). The overall concentration of the analyzed elements like Fe, Ni, Cr, and Co was high in the residual fraction (F4), Mn, Cd, and Zn were high in the organic-sulfide fraction (F3), while Cu and Pb were found to be high in Fe-Mn hydroxide fraction (F2).
In Raudfjord, metals like Fe, Ni, Pb, and Co showed the highest concentration in the residual phase (F4). However, in other fractions, Fe, Mn, Cr, Co, Cu, Zn, Ni, Pb, and Cd had an average concentration of 1.84%, 20.75%, 4.27%, 5.99%, 14.49%,7.11%, 7.79%, 5.06%, and 2.89% respectively in F1 fraction. In the F2 fraction, Fe, Mn, Cr, Co, Cu, Zn, Ni, Pb, and Cd had an average concentration of 21.14%, 20.92%, 17.54%, 15.58%, 37.10%, 24.35%, 9.57, 47.70 and 21.26% respectively. F3 fraction has an average concentration of 37.51%, 29.40%, 39.41%, 19.93%, 22.09%, 42.87%, 31.43%, 8.17%, and 50.99% of Fe, Mn, Cr, Co, Cu, Zn, Ni, Pb and Cd respectively (Figure 6)
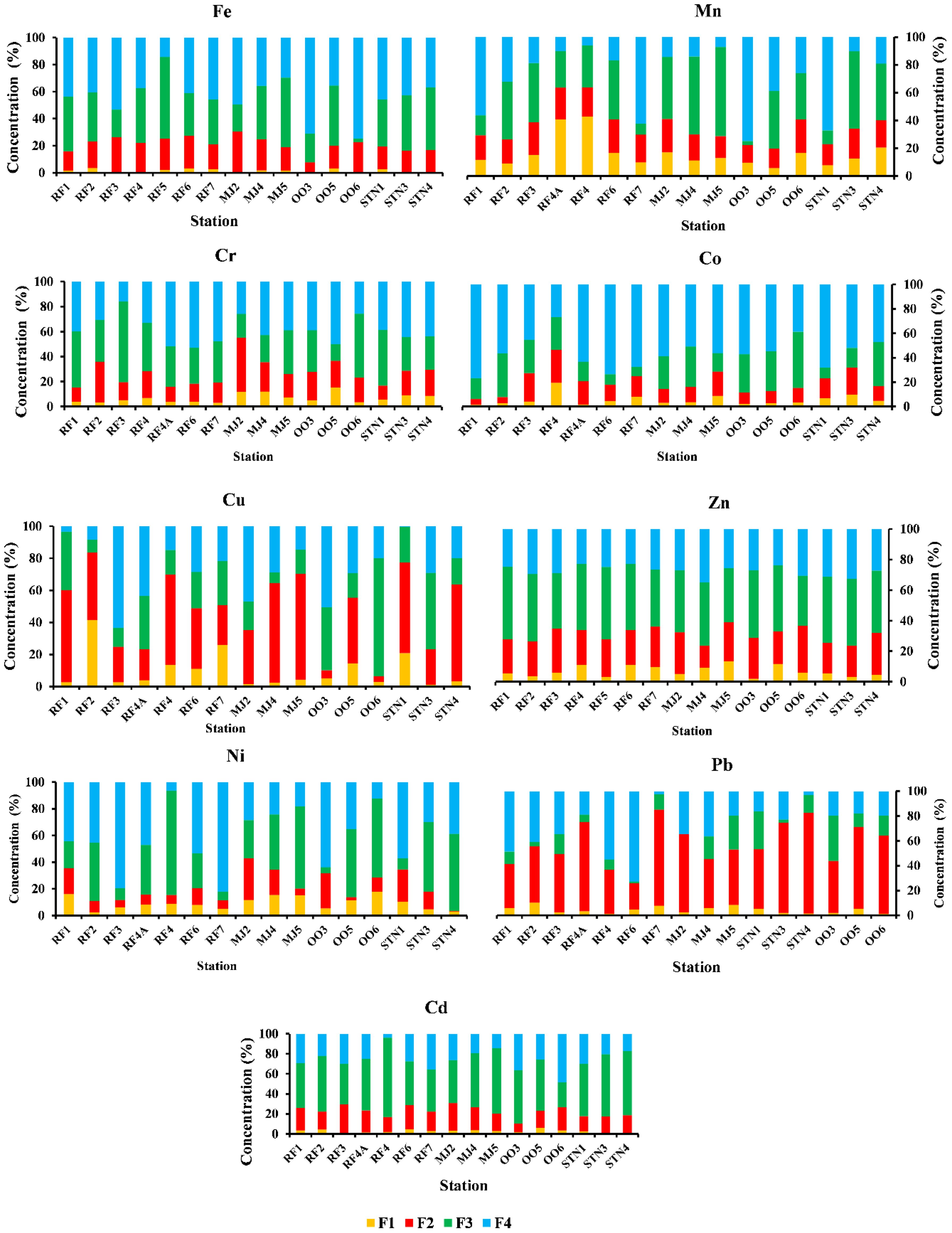
Figure 6. Fractionation of metals like Fe, Mn, Cr, Co, Cu, Zn, Ni, Pb, and Cd in different fractions (F1-Exchangeable and Carbonate, F2- Reducible, F3-Oxidisable and F4- Residual).
In Magdalenefjord, Fe, Cr, and Co were higher in the residual phase (F4). The average concentration of Fe, Mn, Cr, Co, Cu, Zn, Ni, Pb, and Cd in the F1 fraction was 1.16%, 13.71%, 10.32%, 5.19%, 2.72%, 9.25%, 14.02%, 5.56%, 3.23% respectively. In the F2 fraction, Fe, Mn, Cr, Co, Cu, Zn, Ni, Pb, and Cd had an average concentration of 23.54%,19.45%, 28.55%, 14.54%, 53.98%, 22.31%, 18.49%, 48.98%, 22.63% respectively. The F3 fraction had Fe (37.09%), Mn (54.93%), Cr (25.25%), Co (24.96%), Cu (13.15%), Zn (39.23%), Ni (43.81%), Pb (15.23) and Cd (54.19%) (Figure 6)
In St. Jonsfjord, Fe, Ni, Cr and Co were high in the residual phase (F4). The average concentration of Fe, Mn, Cr, Co, Cu, Zn, Ni, Pb, and Cd in the F1 fraction was 0.85%, 13.59%, 7.67%, 7.18%, 8.32%, 9.25%, 6.08%, 2.93%, 1.80%. In the F2 fraction Fe, Mn, Cr, Co, Cu, Zn, Ni, Pb, and Cd have an average concentration of 16.66%, 18.73%, 17.15%, 16.7546.43%, 22.47%, 12.52%, 67.16%, 16.15%. F3 fraction had Fe (40.71%), Mn (35.48%), Cr (32.97%), Co (20.28%), Cu (28.82%), Zn (42.66%), Ni (39.46%), Pb (15.91%) and Cd (59.57%) (Figure 6).
Thus, the overall order of availability of the analyzed element in different fractions is:
Fe- F4>F3>F2>F1
Mn-F3>F4>F2>F1
Cr- F4>F3>F2>F1
Co-F4>F3>F2>F1
Cu-F2>F4>F3>F1
Zn-F3>F4>F2>F1
Ni-F4>F3>F2>F1
Pb-F2>F4>F3>F1
Cd-F3>F4>F2>F1
3.5 Foraminiferal abundance and density
Total foraminiferal (dead and living) number per gram dry sediment i.e. Foraminiferal density decreases from the outer to inner regions of Raudfjord, Magdalenefjord, and St. Johnsfjord (Figure 7) The distribution of N.labradorica showed high abundance in the outer fjords and the open ocean stations with a decrease towards the inner fjord. Elphidium was more abundant in the inner fjords and decreased towards the outer fjord. The abundance of Cassidulina reniforme decreased from the outer fjords to the inner fjord. Cibicides showed higher abundance in the outer fjords and low abundance in the inner fjords.
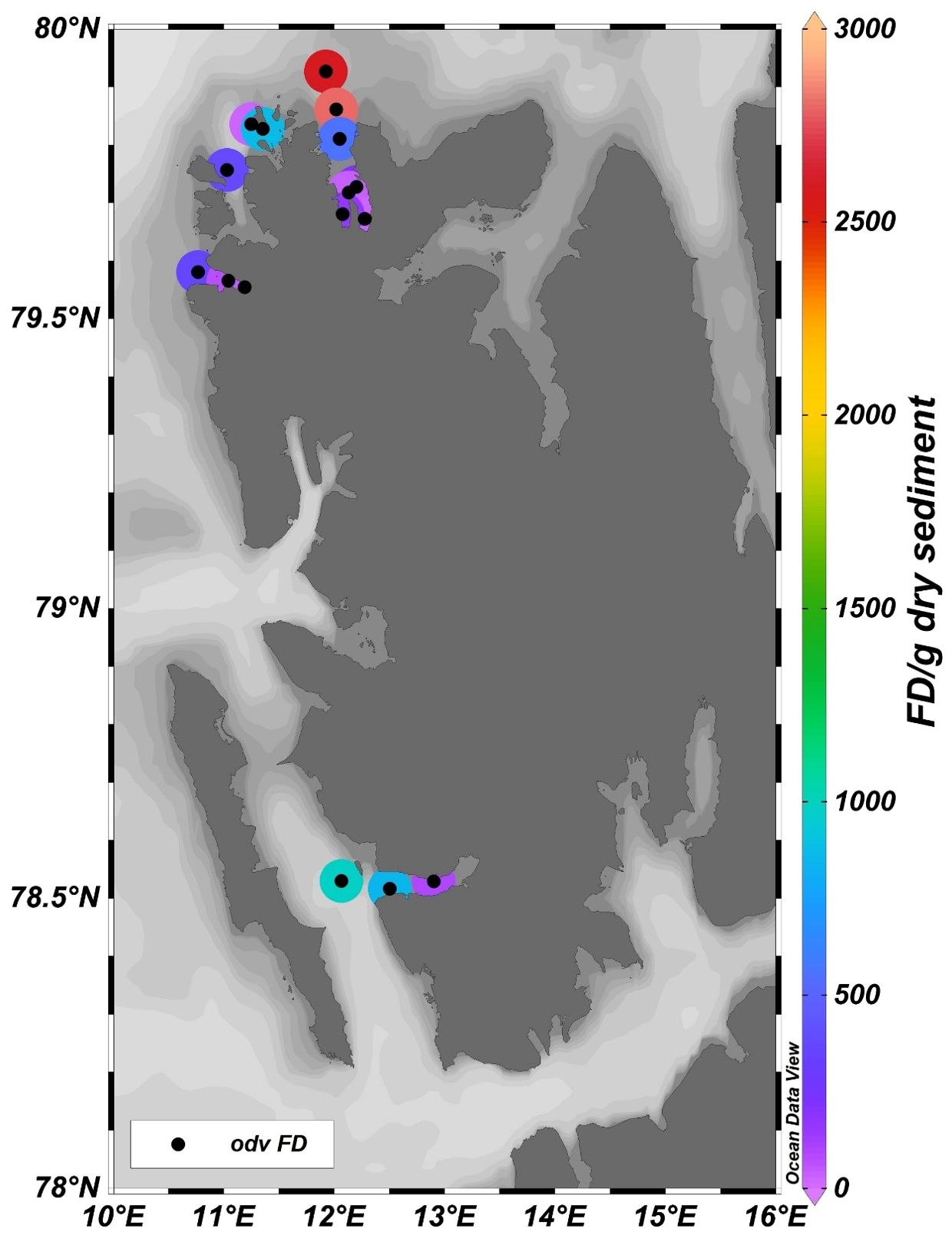
Figure 7. Spatial variations of Foraminiferal density/g dry sediment in Raudfjord, Magdalenefjord, St. Jonsfjord, and open ocean.
4 Discussion
4.1 Distribution and source of trace elements in fjords
Increased concentrations of terrigenous elements such as Al, Ti, Si, and K suggest high concentrations of siliciclastic materials in marine sediment having fluvial or aeolian origin (Chen et al., 2013). Major elements (Al, Ti, Fe, Mn, Mg, Ca, Na, and K) decreased from the inner fjord to the outer fjord in Raudfjord and St. Jonsfjord, suggesting that they have been derived from rock weathering. The significant positive correlation between the major elements is attributed to their common lithogenic origin and similar modes of transportation (Ganugapenta et al., 2018). The Al2O3/TiO2 ratio has been used to assess the source rock type of the sediments. Al2O3/TiO2 ratio <10 indicates mafic source rocks, ratios between 10 and 20 suggest intermediate, and >20 are indicators of felsic source rocks (Hayashi et al., 1997). The Al2O3/TiO2 ratio for all the samples is greater than 20 (22.06–59.96, avg. 35.50), suggesting that felsic rocks are the primary source rock types. The Al2O3/TiO2 ratio of samples from different fjords does not vary much, except for the samples from the open ocean, which have the highest Al2O3/TiO2 ratio. TiO2 showed a strong positive correlation with Fe2O3 (r=0.99), MnO (r=0.87), and MgO (r=0.79), suggesting that ilmenite is one of the minerals forming the source rock. Al and Ti are conservative elements and remain constant or become enriched in the weathered products compared to the parent sediments (Wei et al., 2006; Choudhary et al., 2018b). Al concentration ranged from 1.56% to 1.81%, indicating its uniform terrestrial source suggesting limited Al accumulation changes along the fjords. The average elemental concentrations were lower than the PAAS values and were in the range of other geochemical studies in the fjords of West Spitsbergen (Lu et al., 2013; Grotti et al., 2013; 2017; Singh et al., 2018; Choudhary et al., 2020). This implied that most of the elements have been derived from the weathering of catchment rocks. Potassium (K) is generally derived from K-feldspar or clay mineral illite and is least weathered by chemical weathering (Govin et al., 2012). In contrast, Na and Ca get easily removed from the parent sediment and depleted in the weathered product (residue) due to their higher mobility. Na content is low in the inner fjord and increases towards the outer fjords due to the seawater influx.
Trace elements such as Fe, Mn, Cr, Co, Cu, Zn, Cd, and Ba showed high concentrations in the inner fjords as Al and Ti, respectively (Table 1), suggesting their similar terrigenous source derived from the bedrock. Pb showed a significant positive correlation (r = 0.61, p = <0.05, n =16) with Cd, indicating their common source and post-depositional processes. Sr does not significantly correlate with other elements except Ca, indicative of calcareous rocks in the catchment. Sr/Ba ratio is often used as a geochemical indicator distinguishing between the sedimentary environments i.e. terrestrial and marine environments (Wang et al., 2021). Gradual increase in Sr/Ba ratio from the inner fjord to the outer fjord indicates the influence of seawater influx towards the outer fjord (Table 1). Sediment components like grain size and organic matter do not play any role in the distribution of metals in these fjords. Metals are regulated mainly by Fe-Mn oxyhydroxides and aluminosilicates. Other sources of these metals include atmospheric deposition, long-range transportation by currents, and discharge by glacial meltwater, sea ice, and terrestrial runoff (Rudnicka-Kępa and Zaborska, 2021).
4.2 Terrigenous (TI%) and non-terrigenous (excess) influence in the sediments
TI% (Terrigenous influence) and excess values are calculated to evaluate the terrigenous and non-terrigenous contributions of the elements. TI% decreases from the inner to the outer fjord in all three fjords as the sea water influx increases (Table 1; Figure 4).
Feexcess, Mnexcess, Coexcess, and Cdexcess values obtained (Figure 5) in most of the surface samples exhibited positive values, and the excess percentage was low, indicating less input of Fe and Mn from other sources in addition to terrigenous. Zn excess showed positive values with a high excess percentage varied from 40 to 80%, suggesting high input of Zn other than terrigenous. Cuexcess and Niexcess showed negative values indicating their detrital nature. Crexcess and Pbexcess showed higher excess values attributed to their additional source other than the catchment rocks. Ba showed high excess values for all the samples except MJ2, wherein almost all the metals showed negative excess values other than Cd. Thus, to assess the metal accumulation and metal-related ecological risks in the fjord, the bulk concentration of elements has been compared with Arctic sediment quality guidelines (ASQGs).
4.3 Assessment of sediment quality
The bulk concentration of elements was compared with Sediment Quality Guidelines available for the Arctic region (ASQGs) proposed by Lu and Kang (2018) to assess the contamination in marine and estuarine sediments (Hubner et al., 2009) and understand the impacts on the sediment-associated biota. It has been observed that Cu, Ni, and Zn contents are below ASQG-low in all three fjords suggesting that these elements can rarely cause adverse biological effects in the study area. Cr, Cd, and Pb were higher than ASQG-low but were lower than ASQG-high, indicating adverse biological effects in the study area. High metal accumulation and retention rates in the freshwater environment cause more adverse effects on the biota (Barik et al., 2022).
Further, the element contamination indices, such as the Pollution Load Index (PLI), have been used to assess the sediment quality of the fjords. This index helps evaluate the changes in sediment quality at different environmental conditions as they use the background elemental concentrations of the particular region (Sarkar, 2018). The pollution load index showed lower values (<1) at station RF1 and RF2 towards the mouth in Raudfjord. In Magdalenefjord, PLI was <1 at station MJ2, at station STN4 in St. Jonsfjord, and in the open ocean at all the stations indicating no pollution at these stations (Table 1). PLI was found above 1 in the inner fjord, indicating high elemental contamination compared to the outer fjord. The contamination levels are frequently assessed using bulk concentrations of metals. However, sometimes it may lead to an overestimation of the ecological risks associated. Thus, to avoid the overestimation of risk, the metal speciation has been carried out using the Bureau of Certified Reference (BCR) extraction method into four different fractions: 1. Exchangeable + Carbonate (F1-labile phases) 2. Fe-Mn (F2-Reducible) 3. Organic sulfide (F3-oxidisable), and 4. Residual phase. The F1, F2, and F3 fractions are known as bioavailable phases.
4.4 Risk assessment of metals in different fractions
Martins et al. (2013) suggested that the metals in the F1 fraction are loosely bound and are susceptible to release with changes in pH and redox potential. Exchangeable and carbonate fractions are highly mobile and readily available for biota uptake and enter the food chain, risking the lives of the organisms. Mn is available in significant concentration in F1 fraction along with Cu and Ni in considerable concentration leading to the toxicity in sediment-associated biota in these fjords (Figure 6).
Fe-Mn oxides and hydroxides (F2 fraction) are considered carriers of metals due to their large surface area-to-volume ratio and significantly impact the mobility of elements (Turner, 2000). Cu and Pb have significant concentrations in the Fe-Mn oxide phase (F2 fraction). Pb can form stable bonds with Fe–Mn hydroxides. These hydrous oxides act as scavengers of Pb in the marine environment (Ramos et al., 1994). A considerable amount of Fe, Mn, and Zn is also available in this phase (Figure 6). Metals in this fraction are bound to Fe-Mn oxides and hydroxides. Under the reduced conditions, they can be released in the aqueous environment (Okbah et al., 2020).
Significant Mn, Zn, and Cd concentrations are available in the organic/sulfide phase (F3) (Figure 6). An appreciable quantity of Fe is available in the organic/sulfide phase. Metals from F3 fractions can only be released by oxidizing agents causing bioaccumulation (Okbah et al., 2020). Due to their less mobile nature, organic matter and sulfides are associated with humic substances having high molecular weight. Generally, elements associated with the organic/sulfide phase are the least bioavailable due to the strong bonding between metal and organic matter.
Metals like Fe, Cr, Ni, and Co are available in the maximum concentration in the residual phase (F4 fraction, Figure 6), suggesting that these metals have been released by the weathering of rocks in the catchment region of a fjord. The metals in this fraction are immobile as they are bound to detrital minerals and a matrix of silicates, which are difficult for ingestion and accumulation in the sediment-associated biota. Almost 40 to 50% of metal concentrations are available in the F4 fraction, except for Mn (28.81%), Cu (26.14%), and Cd (25.22%), bound to primary and secondary minerals, which are immobile. Therefore, it is not available for the uptake of the organisms. The remaining 50 to 60% is distributed among the bioavailable phases and is potentially available for biota uptake. During the spring and early summer, oxygen deficiency in the fjords makes these fractions leachable into the water, posing a significant risk to the sediment-associated biota.
The metal bonding strength with the sediment fractions determines the bioavailability and risk associated with metals in aquatic systems (Lu and Kang, 2018). The metal association in the labile fraction (exchangeable and carbonate) suggests that these can be easily released into the water column posing a risk to the aquatic organisms due to slight changes in the pH and redox potential (Saleem et al., 2018). A risk assessment code (RAC) was used to evaluate the risk posed by releasing metals from the F1 fraction into the aquatic environment (Perin et al., 1985). <1% of any metal poses negligible risk to the environment, while >30% poses a high risk to the sediment-associated biota. It has been observed that Fe is under the safe range with RAC<1%. However, significant concentrations of Fe in the F2 fraction can adversely affect the biological community in a reducing environment. Ni, Cu, Pb, Cd, Cr, Zn, and Co pose a low risk to the sediment-associated biota. However, Cu and Ni have significant concentrations in the F1 fraction compared to other metals. Higher concentrations of Mn in labile phases pose a medium risk to the sediment-associated biota. Similar concentrations of Mn were found in the sediments of the Krossfjord-Kongsfjord system (Choudhary et al., 2020).
4.5 Effect of metals on benthic foraminifera in sediment
The density and abundance of foraminiferal assemblages can be affected by the trace metals (Bergamin et al., 2005; Debenay and Fernandez, 2009; Vilela et al., 2011). The variation in Foraminiferal density (FD) is an important tool for assessing pollution (Nigam et al., 2006). The inner fjord is drained by glacial meltwater and surface runoff, which does not support the benthic foraminifera population. Thus, the foraminiferal density (FD) showed an increasing trend towards the outer fjord in all three fjords (Figure 7). Inner fjords have high metal concentrations and, thus, high PLI (Figure 8), posing an ecological threat to the sediment-associated biota. The inner fjord region is dominated by the genus Elphidium. Elphidium tolerates most contaminants and effluents in polluted coastal environments (Pregnolato et al., 2018; Buckley et al., 1974; Schafer et al., 1991). Due to their high mobility, Elphidium flourishes in contaminated areas (Murray, 1991; Schafer and Young, 1977). Agglutinated foraminifera were also highly abundant in the inner fjord. The influence of tidewater glaciers and colder environments caused the dissolution of CaCO3 at inner fjords. Also, the higher sediment flux from the glacier mouth dilutes the calcitic microfossils in the inner fjords leading to increased foraminiferal density towards the outer fjord. The live/dead test ratio in these fjords is low (Figure 9) due to the high sedimentation rate increasing the turbidity affecting the living benthic foraminifera, resulting in a reduced benthic foraminifera population (Saraswat et al., 2018). Principal component analysis (Figure 10) showed that Ni and Cu in the bioavailable fraction are associated with Elphidium and agglutinated foraminifera. Cu contamination has a deleterious effect on foraminifera; particularly, high concentrations of Cu (>120 µg/l) lead to a lowering of foraminiferal density (Frontalini and Coccioni, 2011). Bioavailable fractions (F1 and F3) of Cd and Pb correlated well with Cibicides. Cadmium is detrimental to benthic foraminifera. Gradual increase in Cd concentration mainly affects normal growth and causes morphological abnormalities (Linshy et al., 2013). Pb is toxic, but due to its large ionic size, it is least absorbed by the foraminifera (Samir and El-Din, 2001; Barik et al., 2022). Metals like Mn, Fe, Cd, Ni, and Zn in F2 and F3 fractions are associated with N. Labradorica. Fe in F2 fraction and seasonal hypoxia causes a low benthic foraminiferal population (Gustafsson and Nordberg, 2000) and increases pyritization in the tests of foraminiferal species (Yanko et al., 1999). Mn can be easily incorporated into the calcite in reducing environments (Barras et al., 2018) but causes low toxicity. Mn, Co, Zn, Cr, Co, Cd, and Cr in bioavailable fractions are associated with Cassidulina. High concentrations of metals like Cu (73.86%), Ni (58.32%), Pb (71%), Cd (63.18%), and Cr (61.76%) are available in bioavailable fraction, suggesting their availability for uptake and accumulation in benthic fauna. Cr, Zn, and Cu are more readily absorbed in the shells of foraminifera (Banerji, 1991), affecting the growth and reproduction of benthic foraminifera in marginal zones (Coccioni et al., 2009). Therefore, it can be deciphered that higher concentration of metals and their accumulation in different bioavailable phases have affected the foraminiferal density in the Arctic fjords.
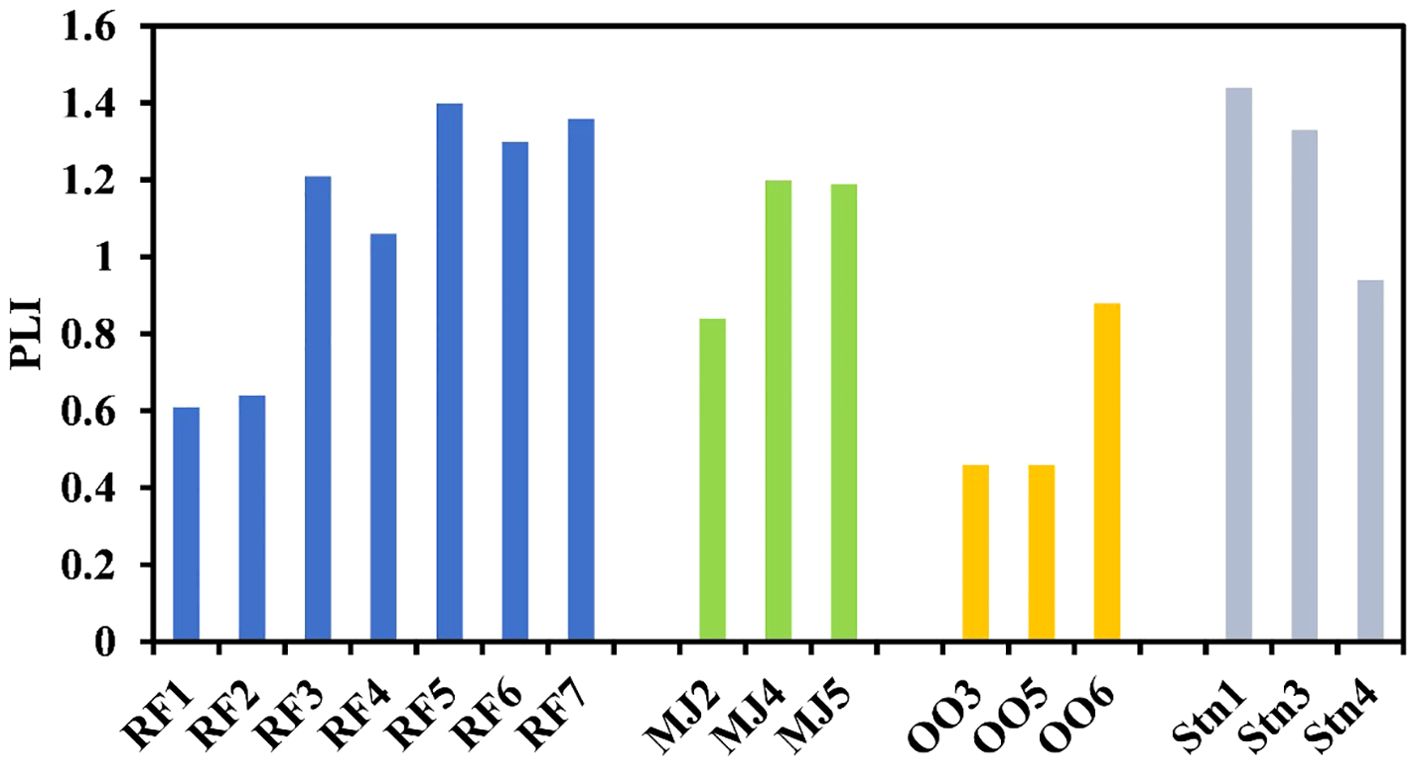
Figure 8. Pollution load index (PLI) in the sediment of Raudfjord, Magdalenefjord, St. Jonsfjord open ocean.
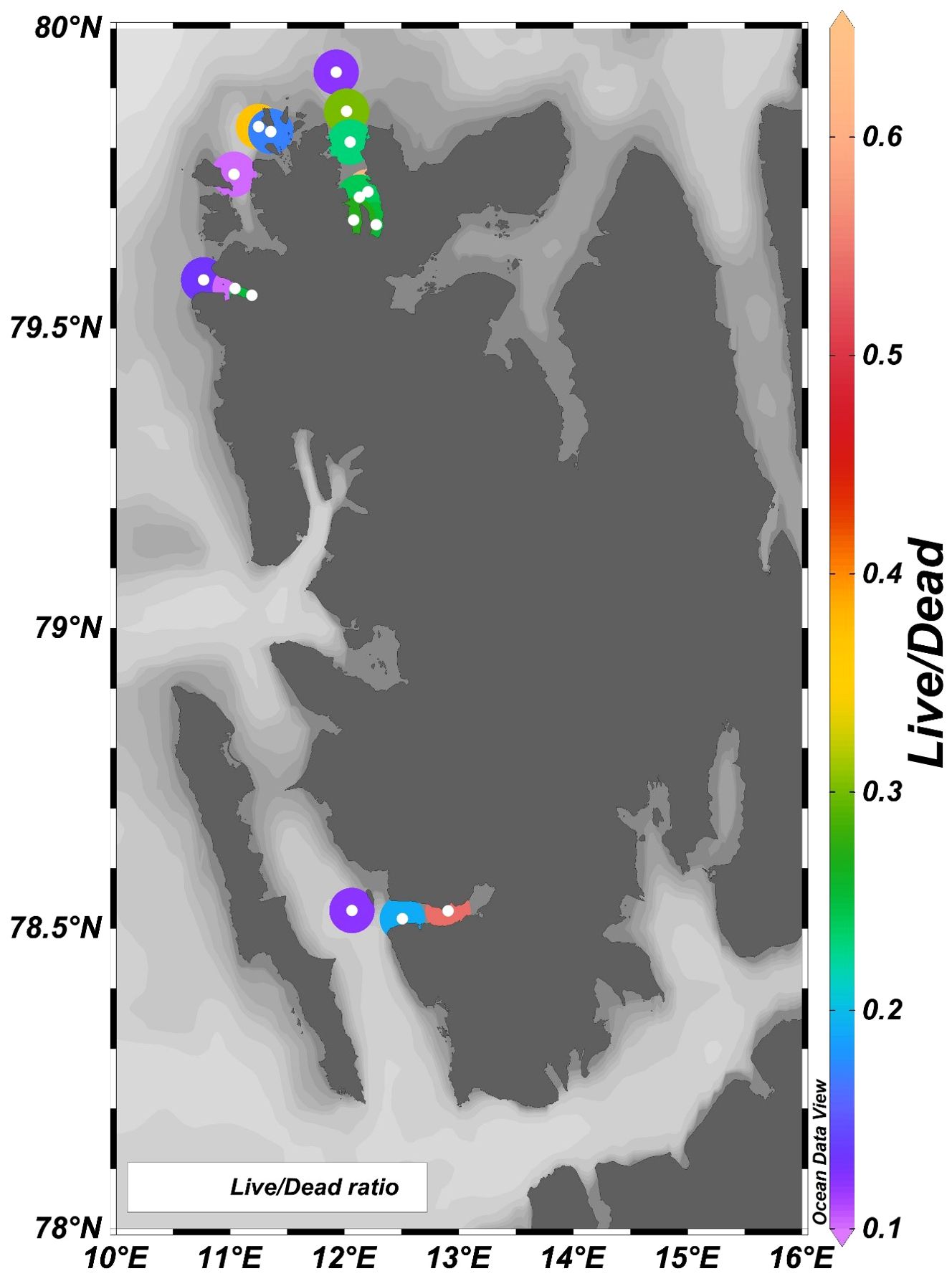
Figure 9. Spatial variations of Live/dead ratio of benthic foraminifera in sediments of Raudfjord, Magdalenefjord, St. Jonsfjord, and open ocean.
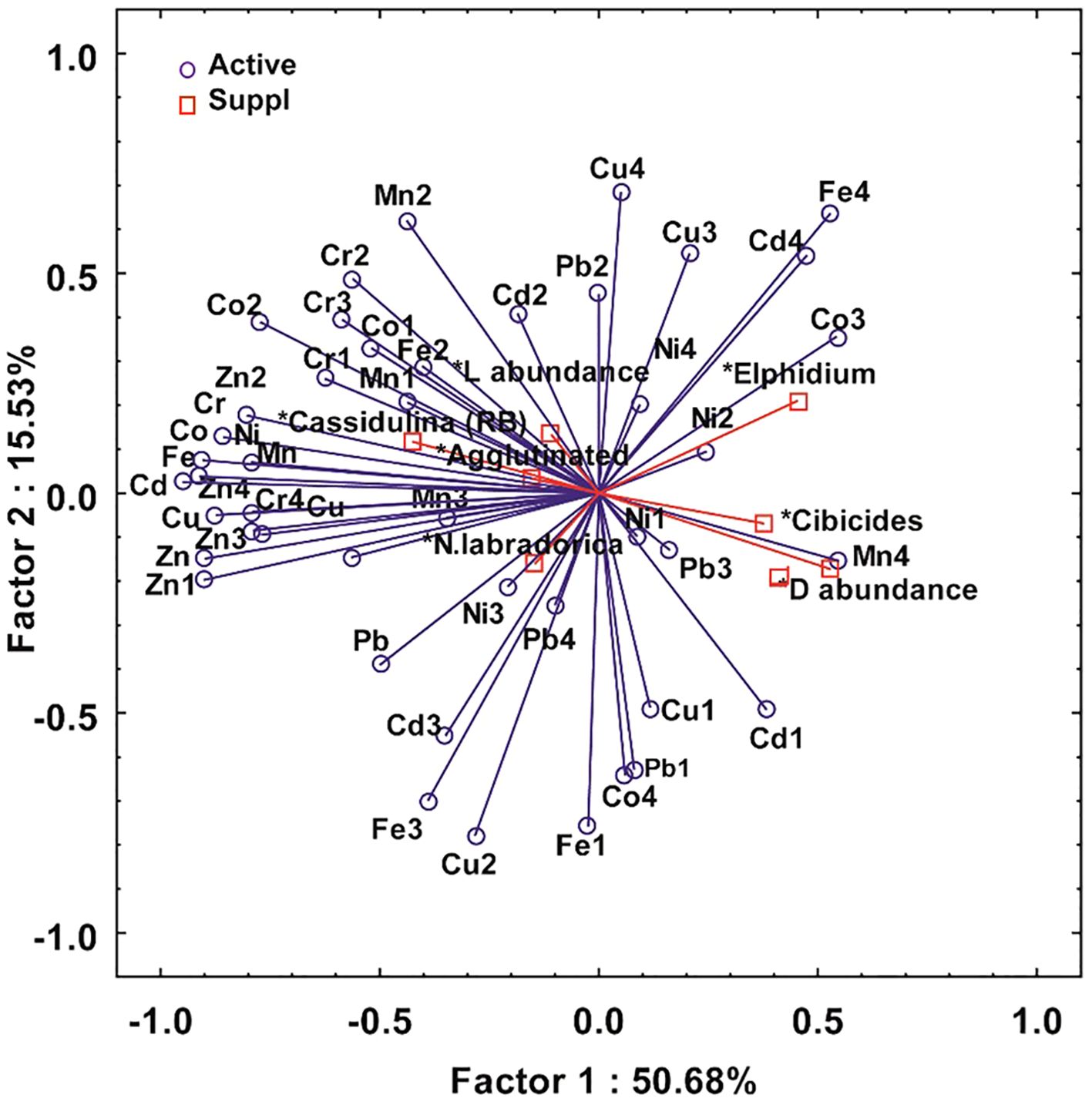
Figure 10. Principal component analysis (PCA) of bulk metals, metal concentration in different fractions (F1, F2, F3, and F4) along with foraminiferal genera and their living and dead abundance in sediments of Raudfjord, Magdalenefjord, St. Jonsfjord, and open ocean.
5 Conclusions
The study conducted on the surface sediments of Raudfjord, Magdalenefjord, and St. Jonsfjord showed considerable variations in metal accumulation within the fjords and are regulated mainly by Fe-Mn oxyhydroxides and aluminosilicates. The Al2O3/TiO2 ratio shows that felsic rocks are the primary source rock of the fjord sediments. Terrigenous influence (TI%) decreases from the inner to the outer fjord. Higher excess values for Cr and Pb are attributed to their additional source other than the catchment rocks. Further, the Speciation showed that Mn concentration poses a medium ecological risk to the sediment-associated biota. Although the overall concentration of metals in the bioavailable fractions (F1, F2, and F3) is low, they have affected the abundance of foraminifera. Besides the bioavailable fraction of metals, glacial meltwater, and terrigenous influx have affected the foraminiferal density in the fjords. Detailed studies on the bioavailability of contaminants are needed to comprehend their effects on fjord ecosystems, particularly on sediment-associated biota. Additionally, continuous and comprehensive monitoring is required to track the sources and levels of metal contamination over time, facilitating the implementation of targeted mitigation strategies.
Data availability statement
The original contributions presented in the study are included in the article/Supplementary Material, further inquiries can be directed to the corresponding author/s.
Author contributions
SC: Writing – review & editing, Writing – original draft, Visualization, Validation, Supervision, Software, Resources, Project administration, Methodology, Investigation, Funding acquisition, Formal analysis, Data curation, Conceptualization. SS: Writing – review & editing, Resources, Methodology, Data curation, Conceptualization. RM: Writing – review & editing, Supervision, Resources, Project administration, Investigation. MT: Writing – review & editing, Resources, Methodology.
Funding
The author (s) declare financial support was recieved for publication of this article. The authors are thankful to the Director of the National Centre for Polar and Ocean Research (NCPOR), Goa for his continuous support and encouragement throughout the study. One of the authors (SC) would like to thank the Science and Engineering Research Board (SERB), Department of Science and Technology (DST) for awarding a National Post-Doctoral Fellowship (NPDF-PDF/2020/000857).
Acknowledgments
The authors are also thankful to Dr. Parijat (Scientist), Deepak Aggarwal (Project Scientist), and Ms. Viola Rodrigues (Scientific Assistant), ESSO-NCPOR, Goa for extending the instrumental facility of XRF and ICP-OES, and their assistance in the elemental analysis. This is NIO contribution No. 7276.
Conflict of interest
The authors declare that the research was conducted in the absence of any commercial or financial relationships that could be construed as a potential conflict of interest.
Publisher’s note
All claims expressed in this article are solely those of the authors and do not necessarily represent those of their affiliated organizations, or those of the publisher, the editors and the reviewers. Any product that may be evaluated in this article, or claim that may be made by its manufacturer, is not guaranteed or endorsed by the publisher.
Supplementary material
The Supplementary Material for this article can be found online at: https://www.frontiersin.org/articles/10.3389/fmars.2024.1429998/full#supplementary-material
References
Akeredolu F. A., Barrie L. A., Olson M. P., Oikawa K. K., Pacyna J. (1996). Alpine lake related to temperature. Can. J. Fish Aquat. Sci. 53, 780–786.
Alve E. (1995). Benthic foraminiferal responses to estuarine pollution; a review. J. Foraminiferal Res. 25, 190–203.
Banerji R. K. (1991). “Heavy metals and benthic foraminiferal distribution along Bombay Coast, India,” in Studies in benthic foraminifera, proc. 4th int. Symp. Benthic foraminifera, sendai 1990. Eds. Takayanagi Y., Saito T. (Sendai: Tokai University Press), 151–157.
Barik S. S., Singh R. K., Jena P. S., Tripathy S., Sharma K., Prusty P. (2019). Spatio-temporal variations in ecosystem and CO2 sequestration in coastal lagoon: a foraminiferal perspective. Mar. Micropaleontology 147, 43–56. doi: 10.1016/j.marmicro.2019.02.003
Barik S. S., Singh R. K., Tripathy S., Farooq S. H., Prusty P. (2022). Bioavailability of metals in coastal lagoon sediments and their influence on benthic foraminifera. Sci. Total Environ. 825, 153986. doi: 10.1016/j.scitotenv.2022.153986
Barras C., Mouret A., Nardelli M. P., Metzger E., Petersen J., La C., et al. (2018). Experimental calibration of manganese incorporation in foraminiferal calcite. Geochimica Cosmochimica Acta 237, 49–64. doi: 10.1016/j.gca.2018.06.009
Barrie L. A. (1986). Background pollution in the Arctic air mass and its relevance to North American acid rain studies. Water Air Soil pollut. 30, 765–777. doi: 10.1007/BF00303343
Bergamin L., Romano E., Magno M. C., Ausili A., Gabellini M. (2005). Pollution monitoring of Bagnoli Bay (Tyrrhenian Sea, Naples, Italy), a sedimentological, chemical, and ecological approach. Aquat. Ecosystem Health Manage. 8, 293–302. doi: 10.1080/14634980500220866
Bergin F., Kucuksezgin F., Uluturhan E.S.İ.N., Barut I. F., Meric E., Avsar N.İ.Y.A.Z.İ., et al. (2006). The response of benthic foraminifera and ostracoda to heavy metal pollution in the Gulf of Izmir (Eastern Aegean Sea). Estuarine Coast. Shelf Sci. 66, 368–386. doi: 10.1016/j.ecss.2005.09.013
Bianchi T. S., Arndt S., Austin W. E., Benn D. I., Bertrand S., Cui X., et al. (2020). Fjords as aquatic critical zones (ACZs). Earth-Science Rev. 203, 103145. doi: 10.1016/j.earscirev.2020.103145
Buckley D. E., Owens E. H., Schafer C. T., Vilks G., Cranston R. E., Rashid M. A., et al. (1974). Canso Strait and Chedabucto Bay: a multidisciplinary study of the impact of man on the marine environment. Geol. Surv. Canada 1, 133 ± 160. doi: 10.4095/102510
Burton E. D., Phillips I. R., Hawker D. W. (2006). Factors controlling the geochemical partitioning of trace metals in estuarine sediments. Soil Sediment Contamination: Int. J. 15, .253–.276. doi: 10.1080/15320380600646290
Chen H., Yang Z., Chu R. K., Tolic N., Liang L., Graham D. E., et al. (2018). Molecular insights into Arctic soil organic matter degradation under warming. Environ. Sci. Technol. 52, 4555–4564. doi: 10.1021/acs.est.7b05469
Chen H. F., Yeh P. Y., Song S. R., Hsu S. C., Yang T. N., Wang Y., et al. (2013). The Ti/Al molar ratio as a new proxy for tracing sediment transportation processes and its application in aeolian events and sea level change in East Asia. J. Asian Earth Sci. 73, 31–38. doi: 10.1016/j.jseaes.2013.04.017
Choudhary S., Nayak G. N., Khare N. (2018a). Provenance, processes, and productivity through spatial distribution of the surface sediment from Kongsfjord to Krossfjord system, Svalbard. J. Indian Assoc. Sedimentologists 35, 47–56.
Choudhary S., Nayak G., Tiwari A. K., Khare N. (2018b). Sediment composition and its effect on the productivity in Larsemann Hills, East Antarctica. Arabian J. Geosciences 11, 1–17. doi: 10.1007/s12517-018-3755-4
Choudhary S., Nayak G. N., Khare N. (2020). Source, mobility, and bioavailability of metals in fjord sediments of Krossfjord-Kongsfjord system, Arctic, Svalbard. Environ. Sci. pollut. Res. 27, 15130–15148. doi: 10.1007/s11356-020-07879-1
Choudhary S., Nayak G. N., Khare N. (2023). Sedimentary processes, metal enrichment and potential ecological risk of metals in lacustrine sediments of Svalbard, Arctic. Environ. Sci. pollut. Res. 30, 106967–106981. doi: 10.1007/s11356-022-23600-w
Coccioni R., Frontalini F., Marsili A., Mana D. (2009). Benthic foraminifera and trace element distribution: a case study from the heavily polluted lagoon of Venice (Italy). Mar. pollut. Bull. 59, 257–267. doi: 10.1016/j.marpolbul.2009.08.009
Debenay J. P., Fernandez J. M. (2009). Benthic foraminifera records of complex anthropogenic environmental changes combined with geochemical data in a tropical bay of New Caledonia (SW Pacific). Mar. pollut. Bull. 59, 311–322. doi: 10.1016/j.marpolbul.2009.09.014
Dietz R., Letcher R. J., Aars J., Andersen M., Boltunov A., Born E. W., et al. (2022). A risk assessment review of mercury exposure in Arctic marine and terrestrial mammals. Sci. Total Environ. 829, 154445. doi: 10.1016/j.scitotenv.2022.154445
El Kateb A., Stalder C., Martínez-Colón M., Mateu-Vicens G., Francescangeli F., Coletti G., et al. (2020). Foraminiferal-based biotic indices to assess the ecological quality status of the Gulf of Gabes (Tunisia): Present limitations and future perspectives. Ecol. Indic. 111, 105962. doi: 10.1016/j.ecolind.2019.105962
Ferraro L., Sprovieri M., Alberico I., Lirer F., Prevedello L., Marsella E. (2006). Benthic foraminifera and heavy metals distribution: a case study from the Naples Harbour (Tyrrhenian Sea, Southern Italy). Environ. pollut. 142, 274–287. doi: 10.1016/j.envpol.2005.10.026
Frontalini F., Buosi C., Da Pelo S., Coccioni R., Cherchi, et al. (2009). Benthic foraminifera as bio-indicators of trace element pollution in the heavily contaminated Santa Gilla lagoon (Cagliari, Italy). Mar. pollut. Bull. 58, 858–877. doi: 10.1016/j.marpolbul.2009.01.015
Frontalini F., Coccioni R. (2008). Benthic foraminifera for heavy metal pollution monitoring: a case study from Italy’s central Adriatic Sea coast. Estuarine Coastal Shelf Sci. 76, .404–.417. doi: 10.1016/j.ecss.2007.07.024
Frontalini C., Coccioni R. (2011). Benthic foraminifera as bioindicators of pollution: a review of Italian research over the last three decades. Rev. Micropaleontol. 54, 115–127. doi: 10.1016/j.revmic.2011.03.001
Ganugapenta S., Nadimikeri J., Chinnapolla S. R. R. B., Ballari L., Madiga R., Nirmala K., et al. (2018). Assessment of heavy metal pollution from the sediment of Tupilipalem Coast, southeast coast of India. Int. J. Sediment Res. 33, 294–302. doi: 10.1016/j.ijsrc.2018.02.004
Geslin E., Debenay J. P., Duleba W., Bonetti C. (2002). Morphological abnormalities of foraminiferal tests in Brazilian environments: comparison between polluted and non-polluted areas. Mar. Micropaleontology 45, 151–168. doi: 10.1016/S0377-8398(01)00042-1
Gibson B. D., Ptacek C. J., Blowes D. W., Daugherty S. D. (2015). Sediment resuspension under variable geochemical conditions and implications for contaminant release. J. Soils Sediments 15, 1644–1656. doi: 10.1007/s11368-015-1106-6
Gildeeva O., Akita L. G., Biehler J., Frenzel P., Alivernini M. (2021). Recent brackish water Foraminifera and Ostracoda from two estuaries in Ghana, and their potential as (palaeo) environmental indicators. Estuarine Coast. Shelf Sci. 256, 107270. doi: 10.1016/j.ecss.2021.107270
Govin A., Holzwarth U., Heslop D., Ford Keeling L., Zabel M., Mulitza S., et al. (2012). Distribution of major elements in Atlantic surface sediments (36 N–49 S): Imprint of terrigenous input and continental weathering. Geochemistry Geophysics Geosystems 13, 1–23. doi: 10.1029/2011GC003785
Grotti M., Soggia F., Ardini F., Bazzano A., Moroni B., Vivani R., et al. (2017). Trace elements in surface sediments from Kongsfjorden, Svalbard: occurrence, sources, and bioavailability. Int. J. Environ. Anal. Chem. 97, 401–418. doi: 10.1080/03067319.2017.1317762
Grotti M., Soggia F., Ianni C., Magi E., Udisti R. (2013). Bioavailability of trace elements in surface sediments from Kongsfjorden, Svalbard. Mar. pollut. Bull. 77, 367–374. doi: 10.1016/j.marpolbul.2013.10.010
Gustafsson M., Nordberg K. (2000). Living (stained) benthic foraminifera and their response to the seasonal hydrographic cycle, periodic hypoxia and primary production in Havstens Fjord on the Swedish west coast. Estuarine Coast. Shelf Sci. 51, 743–761. doi: 10.1006/ecss.2000.0695
Hayashi K. I., Fujisawa H., Holland H. D., Ohmoto H. (1997). Geochemistry of∼ 1.9 Ga sedimentary rocks from northeastern Labrador, Canada. Geochimica cosmochimica Acta 61, 4115–4137. doi: 10.1016/S0016-7037(97)00214-7
Hubner R., Astin K. B., Herbert R. J. (2009). Comparison of sediment quality guidelines (SQGs) for the assessment of metal contamination in marine and estuarine environments. J. Environ. Monit. 11, 713–722. doi: 10.1039/b818593j
Jakimska A., Konieczka P., Skóra K., Namieśnik J. (2011). Bioaccumulation of metals in tissues of marine animals, Part II: metal concentrations in animal tissues. Polish J. Environ. Stud. 20, 1127–1146.
Jarvis I., Jarvis K. E. (1985). Rare-earth element geochemistry of standard sediments: a study using inductively coupled plasma spectrometry. Chem. Geology 53, 335–344. doi: 10.1016/0009-2541(85)90078-6
Jayaraju N., Sundara Raja Reddy B. C., Reddy K. R. (2008). The response of benthic foraminifera to various pollution sources: A study from Nellore Coast, East Coast of India. Environ. Monit. Assess. 142, 319–323. doi: 10.1007/s10661-007-9931-8
Kock G., Triendl M., Hofer R. (1996). Seasonal patterns of metal accumulation in arctic char (Salvelinus alpinus) from an oligotrophic alpine lake related to temperature. Can. J. Fisheries Aquat. Sci. 53, 780–786. doi: 10.1139/f95-243
Koziorowska K., Kuliñski K., Pempkowiak J. (2016). Sedimentary organic matter in two Spitsbergen fjords: Terrestrial and marine contributions based on carbon and nitrogen contents and stable isotopes composition. Continental Shelf Res. 113, 38–46. doi: 10.1016/j.csr.2015.11.010
Kumar V., Tiwari M., Nagoji S., Tripathi S. (2016). Evidence of Anomalously low δ13C of marine organic matter in an Arctic fjord. Sci. Rep. 6, 36192. doi: 10.1038/srep36192
Li L., Zheng H., Wang T., Cai M., Wang P. (2018). Perfluoroalkyl acids in surface seawater from the north pacific to the Arctic Ocean: contamination, distribution, and transportation. Environ. pollut. 238, 168–176. doi: 10.1016/j.envpol.2018.03.018
Limoges A., Ribeiro S., Weckström K., Heikkilä M., Zamelczyk K., Andersen T. J., et al. (2018). Linking the modern distribution of biogenic proxies in high Arctic Greenland shelf sediments to sea ice, primary production, and Arctic-Atlantic inflow. J. Geophysical Research: Biogeosciences 123, 760–786. doi: 10.1002/2017JG003840
Linshy V. N., Nigam R., Heinz P. (2013). “Response of shallow water benthic foraminifera to a 13C-labeled food pulse in the laboratory,” in Approaches to study living foraminifera: collection, maintenance, and experimentation (Springer Japan, Tokyo), 115–131.
Lintner M., Lintner B., Wanek W., Keul N., von der Kammer F., Hofmann T., et al. (2021). Effects of heavy elements (Pb, Cu, Zn) on algal food uptake by Elphidium excavatum (Foraminifera). Heliyon 7, 1–17. doi: 10.1016/j.heliyon.2021.e08427
Lu Z., Cai M., Wang J., Yin Z., Yang H. (2013). Levels and distribution of trace metals in surface sediments from Kongsfjorden, Svalbard, Norwegian Arctic. Environ. Geochemical Health 35, 257–269. doi: 10.1007/s10653-012-9481-z
Lu Z. B., Kang M. (2018). Risk assessment of toxic metals in marine sediments from the Arctic Ocean using a modified BCR sequential extraction procedure. J. Environ. Sci. Health Part A 53, 278–293. doi: 10.1080/10934529.2017.1397443
Maccali J., Hillaire-Marcel C., Carignan J., Reisberg L. C. (2013). Geochemical signatures of sediments documenting Arctic sea-ice and water mass export through Fram Strait since the Last Glacial Maximum. Quaternary Sci. Rev. 64, 136–151. doi: 10.1016/j.quascirev.2012.10.029
Martins M. V. A., Frontalini F., Tramonte K. M., Figueira R. C. L., Miranda P., Sequeira C., et al. (2013). Assessment of the health quality of Ria de Aveiro (Portugal): heavy metals and benthic foraminifera. Mar. pollut. Bull. 70, 18–33. doi: 10.1016/j.marpolbul.2013.02.003
Martins M. V. A., Yamashita C., e Sousa S. H. D. M., Koutsoukos E. A. M., Disaró S. T., Debenay J. P., et al. (2019). “Response of benthic foraminifera to environmental variability: importance of benthic foraminifera in monitoring studies,” in Monitoring of marine pollution (UK: IntechOpen).
McLennan S. M. (2001). Relationships between the trace element composition of sedimentary rocks and upper continental crust. Geochemistry Geophysics Geosystems 2, 2000GC000109. doi: 10.1029/2000GC000109
Mendes R., Garbeva P., Raaijmakers J. M. (2013). The rhizosphere microbiome: significance of plant beneficial, plant pathogenic, and human pathogenic microorganisms. FEMS Microbiol. Rev. 37, 634–663. doi: 10.1111/1574-6976.12028
Mortlock R. A., Froelich P. N. (1989). A simple method for the rapid deter-mination of biogenic opal in pelagic marine sediments. Deep-Sea Res i: Oceanogr Res Pap. 36, 1415–1426.
Muller P. J., Schneider R. (1993). An automated leaching method for thedetermination of opal in sediments and particulate matter. DeepSea Res i: Oceanogr Res Pap 40, 425–444. doi: 10.1016/0967-0637(93)90140-X
Murphy J., Riley J. P. (1962). A modified single solution method for thedetermination of phosphate in natural waters. Anal Chim Acta., 2731–3627. doi: 10.1016/S0003-2670(00)88444-5
Murray (1991). “Ecology and paleoecology of benthonic foraminifera,” in Longman scientific and technical/wiley, vol. 397. (UK, New York).
Murray R. W., Leinen M. (1996). Scavenged excess aluminum and its relationship to bulk titanium in biogenic sediment from the central equatorial Pacific Ocean. Geochimica Cosmochimica Acta 60, 3869–3878. doi: 10.1016/0016-7037(96)00236-0
Nigam R., Saraswat R., Kurtarkar S. R. (2006). Laboratory experiment to study the effect of salinity variations on benthic foraminiferal species-Pararotalia nipponica (Asano). J. Geological Soc. India 67, 41–46.
Okbah M. A., El-Gammal M. I., Ibrahim M. S., Waheshi Y. A. (2020). Geochemical speciation of trace metals in northern Nile Delta Lake sediments by sequential extraction technique. Chem. Ecol. 36, 236–255. doi: 10.1080/02757540.2020.1718118
Ottar B. (1989). Arctic air pollution: a Norwegian perspective. Atmospheric Environ. 23, 2349–2356. doi: 10.1016/0004-6981(89)90248-5
Perin G., Craboledda L., Lucchese M., Cirillo R., Dotta L., Zanette M. L., et al. (1985). “Heavy metal speciation in the sediments of northern adriatic sea. A new approach for environmental toxicity determination,” in Heavy Metals in the Environment International Conference, Edinburg, Vol. 2. 454–456.
Poland J. S., Riddle M. J., Barbara A. (2003). “Contaminants in the Arctic and the Antarctic: a comparison of sources, impacts, and remediation options,” in Polar record, (UK: Cambridge University Press), 39, 369–383.
Pregnolato L. A., Viana R. D. A., Passos C. C., Misailidis M. L., Duleba W. (2018). Ammonia-Elphidium index as a proxy for marine pollution assessment, Northeast Brazil. J. Sedimentary Environments 3, 176–186. doi: 10.12957/jse.2018.38001
Ramos L., Hernandez M., Gonzalez J. (1994). Sequential fractionation of copper, lead, cadmium and zinc in soils from or near Donana National Park. American Society of Agronomy, Crop Science Society of America, and Soil Science Society of America 23, 50–57.
Ribeiro S., Sejr M. K., Limoges A., Heikkilä M., Andersen T. J., Tallberg P., et al. (2017). Sea ice and primary production proxies in surface sediments from a High Arctic Greenland fjord: Spatial distribution and implications for palaeoenvironmental studies. Ambio 46, 106–118. doi: 10.1007/s13280-016-0894-2
Rudnicka-Kępa P., Zaborska A. (2021). Sources, fate and distribution of inorganic contaminants in the Svalbard area, representative of a typical Arctic critical environment–a review. Environ. Monit. Assess. 193, 724. doi: 10.1007/s10661-021-09305-6
Saalim S. M., Choudhary S., Mohan R. (2022a). Sources and fate of organic carbon in west spitsbergen fjord systems: A review. J. Geological Soc. India 98, 615–620. doi: 10.1007/s12594-022-2035-9
Saalim S. M., Saraswat R., Nigam R. (2022b). Ecological preferences of living benthic foraminifera from the Mahanadi river-dominated north-western Bay of Bengal: A potential environmental impact assessment tool. Mar. pollut. Bull. 175, 113158. doi: 10.1016/j.marpolbul.2021.113158
Saalim S. M., Saraswat R., Suokhrie T., Bhadra S. R., Kurtarkar S. R., Nigam R. (2017). Benthic foraminiferal response to changes in mining pattern: a case study from the Zuari estuary, Goa, India. Environ. Earth Sci. 76, 1–10. doi: 10.1007/s12665-017-7052-4
Salazar-Rojas T., Cejudo-Ruiz F. R., Gutiérrez-Soto M. V., Calvo-Brenes G. (2023). Assessing heavy metal pollution load index (PLI) in biomonitors and road dust from vehicular emission by magnetic properties modeling. Environmental Science and Pollution Research 30, 91248–91261. doi: 10.1007/s11356-023-28758-5
Saleem A., Fareed I., Irshad M., Mahmood Q., Eneji A. E., Shahzad M. (2018). Transformations of phosphorus and other plant nutrients in poultry litter composted with sugarcane and cabbage wastes. Compost Sci. Utilization 26, 114–127. doi: 10.1186/s44147-023-00177-w
Samir A. M., El-Din A. B. (2001). Benthic foraminiferal assemblages and morphological abnormalities as pollution proxies in two Egyptian bays. Mar. Micropaleontology 41, 193–227. doi: 10.1016/S0377-8398(00)00061-X
Saraswat R., Roy C., Khare N., Saalim S. M., Kurtarkar S. R. (2018). Assessing the environmental significance of benthic foraminiferal morpho-groups from the northern high latitudinal regions. Polar Sci. 18, 28–38. doi: 10.1016/j.polar.2018.08.002
Sarkar S. K. (2018). TraceMetals in a tropical mangrove wetland (Singapore: Springer). doi: 10.1007/978-981-10-2793-2.978-981
Schafer C. T., Collins E. S., Smith N. J. (1991). Relationship of foraminifera and thecamoebian distribution to sediments contaminated by pulp mill ef¯uent: Saguenary Fjord, Quebec, Canada. Mar. Micropaleontology 17, 255–283. doi: 10.1016/j.polar.2018.08.002
Schafer C. T., Young J. (1977). Experiments of mobility and transportability of some nearshore benthonic foraminifera species. Geol. Surv. Can. Paper 70, 77–1C, 27 ± 31. doi: 10.4095/102811
Schintu M., Buosi C., Galgani F., Marrucci A., Marras B., Ibba A., et al. (2015). Interpretation of coastal sediment quality based on trace metal and PAH analysis, benthic foraminifera, and toxicity tests (Sardinia, Western Mediterranean). Mar. pollut. Bull. 94, 72–83. doi: 10.1016/j.marpolbul.2015.03.007
Schlitzer R. (2023). Ocean data view. Available online at: https://odv.awi.de (accessed July 03, 2024).
Schroeder J. O., Murray R. W., Leinen M., Pflaum R. C., Janecek T. R. (1997). Barium in equatorial Pacific carbonate sediment: Terrigenous, oxide, and biogenic associations. Paleoceanography 12, .125–.146. doi: 10.1029/96PA02736
Sensarma S., Banerjee R., Satyanarayanan M., Mukhopadhyay S. (2018). Depositional environment of the surface sediments in Central Indian Basin (CIB), Indian Ocean, between 8–18 S latitude and 72–79 E longitude, based on their geochemical characteristics. Geological J. 53, 1586–1603. doi: 10.1002/gj.2978
Singh N., Sivaramakrishnan R., Choudhary S., Chikkamadaiah K. (2018). Spatial distribution and environmental assessment of heavy metals in the surface sediments of Kongsfjorden, Svalbard. Czech Polar Rep. 8, 1–23. doi: 10.5817/CPR2018-1-1
Taylor S. R., McLennan S. M. (1985). The continental crust: its composition and evolution. U.S. Department of Energy, U.S. Department of Energy, Office of Scientific and Technical Information, United states.
Taylor S. R., McLennan S. M. (1995). The geochemical evolution of the continental crust. Rev. Geophysics 33, 241–265. doi: 10.1029/95RG00262
Tomlinson D. L., Wilson J. G., Harris C. R., Jeffney D. W. (1980). Problems in the assessment of heavy metal levels in estuaries and the formation of a pollution index. Helgoländer Meeresun 33, 566–572. doi: 10.1007/BF02414780
Turner A. (2000). Trace metal contamination in sediments from UK estuaries: an empirical evaluation of the role of hydrous iron and manganese oxides. Estuarine Coast. Shelf Sci. 50, 355–371. doi: 10.1006/ecss.1999.0573
Vilela C. G., Batista D. S., Neto J. A. B., Ghiselli R. O. Jr. (2011). Benthic foraminifera distribution in a tourist lagoon in Rio de Janeiro, Brazil: A response to anthropogenic impacts. Mar. pollut. Bull. 62, 2055–2074. doi: 10.1016/j.marpolbul.2011.07.023
Wang, Wang A., Wang Z., Liu J., Xu N., Li H. (2021). The Sr/Ba ratio response to salinity in clastic sediments of the Yangtze River Delta. Chem. Geology 559, 119923. doi: 10.1016/j.chemgeo.2020.119923
Wei G., Li X. H., Liu Y., Shao L., Liang X. (2006). Geochemical record of chemical weathering and monsoon climate change since the early Miocene in the South China Sea. Paleoceanography 21, 1–11. doi: 10.1029/2006PA001300
Winkelmann D., Knies J. (2005). Recent distribution and accumulation of organic carbon on the continental margin west off Spitsbergen. Geochemistry Geophysics Geosystems 6, 1–22. doi: 10.1029/2005GC000916
Yanko V., Ahmad M., Kaminski M. (1998). Morphological deformities of benthic foraminiferal tests in response to pollution by heavy metals; implications for pollution monitoring. J. Foraminiferal Res. 28, 177–200.
Yanko V., Arnold A. J., Parker W. C. (1999). Effects of marine pollution on benthic foraminifera (Netherlands, Dordrech: Modern Foraminifera. Springer), 217–235.
Yanko V., Kronfeld J., Flexer A. (1994). Response of benthic foraminifera to various pollution sources: implications for pollution monitoring. J. Foraminiferal Res. 24, 1–17. doi: 10.2113/gsjfr.24.1.1
Keywords: fjord, metals, terrigenous influence, excess values, foraminifera, ecological risk, sediment-associated biota
Citation: Choudhary S, Syed Mohammad S, Mohan R and Tiwari M (2024) Source, bioavailability, and toxicity of metals in modern fjord sediments, west Spitsbergen, and their influence on sediment-associated biota. Front. Mar. Sci. 11:1429998. doi: 10.3389/fmars.2024.1429998
Received: 09 May 2024; Accepted: 07 August 2024;
Published: 02 September 2024.
Edited by:
Rachel Ann Hauser-Davis, Oswaldo Cruz Foundation (Fiocruz), BrazilCopyright © 2024 Choudhary, Syed Mohammad, Mohan and Tiwari. This is an open-access article distributed under the terms of the Creative Commons Attribution License (CC BY). The use, distribution or reproduction in other forums is permitted, provided the original author(s) and the copyright owner(s) are credited and that the original publication in this journal is cited, in accordance with accepted academic practice. No use, distribution or reproduction is permitted which does not comply with these terms.
*Correspondence: Shabnam Choudhary, c2hhYm5hbS5nZW9sb2d5QGdtYWlsLmNvbQ==