- Guangxi Key Laboratory for Polysaccharide Materials and Modifications, Guangxi Marine Microbial Resources Industrialization Engineering Technology Research Center, School of Marine Sciences and Biotechnology, Guangxi Minzu University, Nanning, China
Marine red yeast is a good feed additive for the aquaculture industry that can promote the growth of aquatic animals, and significantly improve their antioxidant capacity, survival rate, and nonspecific immune ability of the body. Our hypothesis is that dietary supplementation with marine red yeast could affect the serum biochemistry, muscle composition, muscle fatty acid composition, lipid metabolism enzyme activity, and expression of antioxidant and inflammatory genes in juvenile genetically improved farmed tilapia (GIFT, Oreochromis niloticus). Five diets with different levels of marine red yeast (0 %, 0.25 %, 0.50 %, 0.75 %, and 1.00 %) were used to feed juvenile GIFT (initial weight: 21.12 ± 0.86 g) for 60 days. The main results are as follows: Compared with the control group (0 % marine red yeast), the contents of total protein (TP), albumin (ALB), high-density lipoprotein (HDL), and free fatty acid (FFA) in the serum of juvenile GIFT tilapia significantly increased (P < 0.05), while the contents of glucose (GLU), triglyceride (TG), total cholesterol (T-CHO), low-density lipoprotein (LDL), alanine aminotransferase (ALT) significantly decreased (P < 0.05). The contents of crude protein, crude fat, and docosahexaenoic acid + eicosapentaenoic acid (DHA+EPA) in the muscle significantly increased (P < 0.05), while the contents of moisture and monounsaturated fatty acids (∑MUFAs) significantly decreased (P < 0.05). The activities of acetyl CoA carboxylase α (ACCα), glucose-6-phosphate dehydrogenase (G6PD), and stearoyl-CoA desaturase (SCD) in the liver significantly increased (P < 0.05). The expression levels of catalase (cat), alkaline phosphatase (alp), nuclear factor erythroid 2-related factor 2 (nrf2), lysozyme (lyz), glutathione S-transferase (gst), glutathione peroxidase (gsh-px), and superoxide dismutase (sod) genes in the liver significantly increased (P < 0.05). The expression levels of tumor necrosis factor α (tnf-α), interferon-γ (inf-γ), interleukin 6 (il-6), interleukin 8 (il-8), interleukin 1β (il-1β), and transforming growth factor-β (tgf-β1) genes in the liver, spleens, and head kidney significantly increased (P < 0.05), while the expression level of interleukin 10 (il-10) gene significantly decreased (P < 0.05). In conclusion, the addition of different levels of marine red yeast could significantly affect the serum biochemistry, muscle composition, muscle fatty acid composition, lipid metabolism enzyme activity, and expression of antioxidant and inflammatory genes in juvenile GIFT tilapia. Based on the results, the optimal dietary marine red yeast level was 0.50 %.
1 Introduction
Aquaculture has developed rapidly in the past few decades and has made significant contributions to meeting human demand for aquatic organisms. However, with the improvement of productivity, intensive aquaculture has exposed many shortcomings, such as the need to improve the quality of fish fry and strengthen the supply of feed nutrients. The most important problem is that the misuse of antibiotics has led to the destruction of the aquaculture water environment, excessive residue of chemical drugs in fish, increased drug resistance among bacteria and viruses, and frequent outbreaks of diseases resulting in numerous deaths of aquatic animals and immeasurable economic losses (Dawood and Koshio, 2016; Chen et al., 2019). Probiotics are considered a powerful candidate for solving problems due to their ability to colonize the intestines of aquatic animals, improve aquaculture water quality, enhance the growth performance of aquatic animals, and maintain stable aquaculture environments without generally having negative effects on consumers (Dawood et al., 2017; Wang et al., 2023). For example, probiotics can enhance the ability of aquatic animals to produce digestive enzymes, increase their feed utilization, and thus improve breeding efficiency (Hai, 2015). Probiotics can stimulate the immune system of aquatic animals, enhancing their immunity and producing antibacterial substances themselves, thereby increasing aquatic animals’ resistance to pathogenic bacteria (Zorriehzahra et al., 2016). Additionally, probiotics can produce antibacterial substances and bioactive compounds that reduce oxidative stress damage by inhibiting free radical production and activating antioxidant enzyme activity in fish bodies, ultimately improving fish health (Tseng et al., 2009).
Marine red yeast is a pigment yeast isolated from marine mud and animal digestive tracts, containing abundant nutrients such as proteins, vitamins, fatty acids, and carotenoids, mainly astaxanthin. It is a good feed additive for the aquaculture industry that can promote the growth of aquatic animals and significantly improve their antioxidant capacity, survival rate, and non-specific immune ability of the body (Zhang et al., 2014). Previous studies have shown that red yeast could not only improve the growth performance of aquatic organisms but also enhance their antioxidant capacity and immune function while increasing resistance to fish diseases (Wang et al., 2018), as well as improving the activity of digestive enzymes by affecting the composition of intestinal microorganisms in farmed fish (Waché et al., 2006).
Genetically improved farmed tilapia (GIFT, Oreochromis niloticus) is globally recognized as an economically important fish species. Over the past few decades, the population of GIFT has steadily increased (Prabu et al., 2019). GIFT exhibits excellent growth performance, rapid growth, tender meat, strong environmental tolerance, high nutritional value, and easy breeding characteristics (Richard et al., 2012). Currently, there is limited research on the impact of red yeast on tilapia. Therefore, this study aimed to investigate the effects of dietary marine red yeast supplementation on serum biochemistry, muscle composition, fatty acid profile, lipid metabolic enzyme activity, and antioxidant and inflammatory gene expression in juvenile GIFT. This study provides a reference for incorporating marine red yeast into the commercialized breeding production of tilapia.
2 Materials and methods
2.1 Experimental diets
The freeze-dried powder of marine red yeast (Rhodotorula mucilaginosa) was provided by China Guangzhou Xinhaili Biotechnology Co., Ltd. The concentration of freeze-dried marine red yeast cells exceeds 1 × 1010 cells per gram. Based on wet weight, the nutritional components of marine red yeast are as follows: moisture 81.17%, crude protein 9.27%, crude fat 4.45%, total sugar 4.2%, β-glucan 1.3%, β-carotene 1.4 mg/kg, astaxanthin 1.0 mg/kg, and vitamin E 172 mg/kg.
The number of viable bacteria in marine red yeast was determined by the plate method (Nikoskelainen et al., 2003). One gram of freeze-dried marine red yeast powder stored in a refrigerator (−80°C) was added to 9 mL of sterile saline. To ensure uniform distribution, the mixture was vortexed [Genie 2, SI-0246, Tuohe Electromechanical Technology (Shanghai) Co., Ltd., Shanghai, China] at 2,800 rpm for 1 min. Subsequently, the diluent with a concentration of 10−3–10−9 g/mL was prepared using the gradient dilution method. The obtained diluent was inoculated on a plate culture medium and activated at 30°C for 24 h. The plate culture medium contains 2.0% glucose, 1.0% peptone, 0.5% yeast extract, 2.0% agar, and 100 mL of seawater with a pH of 8.1. The number of live bacteria in the sample was determined based on the counting of marine red yeast colonies on a flat plate culture medium (Nikoskelainen et al., 2003). The count of live marine red yeast = B × f, where “B” is the total number of colonies on the plate medium and “f” is the dilution ratio. The results indicated that the viable count of marine red yeast was 7.33 × 109 cfu/g.
Two colonies were isolated from the above plate medium and transferred to a triangular conical flask with 80 mL of liquid medium (the total volume of the triangular conical flask was 500 mL). The seed culture medium was prepared by stirring on a constant temperature shaker (30°C, 160 rpm) for 24 h. Subsequently, 4.8 mL of seed medium was inoculated into a new conical flask containing 80 mL of liquid medium while keeping the culture conditions unchanged in a constant temperature for 48 h. The liquid culture medium contains 2.0% glucose, 1.0% peptone, 0.5% yeast extract, and 100 mL of seawater with a pH of 8.1. The count of living bacteria was determined by hematology (Nikoskelainen et al., 2003). The results indicated that the density of marine red yeast was 6.38 × 108 cfu/mL. The rapid growth of marine red yeast is the necessary condition for its application as a feed additive for aquatic animals.
The commercial feed was produced by Nanning Haida Feed Company in Nanning, China, and the feed formulas were unknown. The content of crude protein, crude fat, moisture, and ash in the commercial feed was determined according to the method of the Association of Official Analytical Chemists (AOAC, 2005). The proximate composition (dry basis) of the commercial feed was as follows: dry matter 90.6%, crude protein 33.9%, crude lipid 7.4%, and ash 7.0%.
Five dietary marine red yeast levels of 0% (control diet), 0.25%, 0.50%, 0.75%, and 1.00% were designed based on the study by Yang et al. (2010). All experimental diets were finely ground into a 60-mesh powder by a hammer mill. Then, the fine powder was supplemented with five classification levels of marine red yeast (0%, 0.25%, 0.50%, 0.75%, and 1.00%) and mixed in a drum mixer for 15 min, respectively. To form the consistency of hard dough, sterile distilled water (40% by mass) was added to the mixture. The dough was manually separated into many small balls, and a screen was used to select balls with a diameter of approximately 1.00–1.50 mm. All experimental diets were dried in airflow at 30°C until the water content reached below 100 g/kg. Finally, the dry diet was put into a sealed bag and stored at −20°C for later use. The experimental diets were prepared every week to maintain the quality and vitality of marine red yeast.
2.2 Experimental fish and culture
The same batch of 1,000 juvenile GIFT was produced by Guangxi Angui Aquaculture Company in Nanning, China. Before the start of the culture experiment, the juvenile GIFT was disinfected with 20 mg/L of potassium permanganate solution for 10 min, and then the juvenile GIFT was acclimatized in the culture pond for 2 weeks. The juvenile GIFT used in this experiment was approved by the Biomedical Ethics Committee of Guangxi University for Nationalities (approval number: GXMZU-2022–008). During the acclimatization, circulating water was used in the culture pond, and the water temperature was adjusted by using a heating rod to keep the water temperature at 26°C ± 1°C, the pH value was 7.0–8.0, and the dissolved oxygen was ≥8 mg/L. The juvenile GIFT was fed with the control diet at 9:00, 14:00, and 19:00 every day. Before feeding every morning, the feces from the reproductive system was removed and replaced with one-third of the total water.
After a 2-week acclimatization period, the juvenile GIFT was subjected to a 24-h fasting regime. Subsequently, 450 juveniles (initial body weight of 21.12 ± 0.86 g) were randomly assigned into five groups with three replicates per group, resulting in a total of 15 breeding tanks. Each breeding tank had a capacity of 720 L (150 cm × 60 cm × 80 cm, L × W × H) and accommodated 30 juvenile GIFT.
As described in the acclimatization process, the juvenile tilapia was cultured in the same aquaculture system. The juveniles were fed three times a day at 9:00, 14:00, and 19:00. The control diet was not added with marine red yeast, and the other diets with different marine red yeast levels of 0.25%, 0.50%, 0.75%, and 1.00% were used as the experimental group diets, respectively. The daily feeding amount was 5% of the total wet weight of the fish. To adjust the feed quality, three fish were randomly weighed in each group every week. The feeding period lasted for 60 days.
2.3 Sampling
At the end of the 60-day experiment, the juvenile GIFT was starved for 24 h, and then nine fish were randomly selected from each group (three fish were extracted from each tank), and then the samples were anesthetized with 0.2 g/L of ethyl aminobenzoate mesylate (MS-222, Shanghai Adamas Reagent Co., Ltd., Shanghai, China). Blood was collected from the tail vein of the fish, and the collected blood sample was placed in a sterile EP tube, allowed to stand at 4°C for 24 h, and then centrifuged at 4°C and 3,000g for 15 min. The upper serum was taken, put in a new EP tube, and labeled accordingly. Muscle, liver, spleen, and head kidney samples were collected. All samples were put into labeled sample bags and stored in liquid nitrogen. After all the sampling procedures were completed, the samples were evenly stored in an ultra-low temperature refrigerator set at −80°C for subsequent analysis.
2.4 Determination of serum biochemical parameters and lipid metabolism enzyme activity
According to the method of Liu et al. (2023), the serum biochemical parameters and lipid metabolism enzyme activity were determined using an enzyme-linked immunosorbent assay (ELISA) analyzer (RT-6100, Rayto, Shenzhen, China) and assay kits. The content of total protein (TP, g/L) in the serum of juvenile tilapia was determined using the Coomassie blue staining method. The contents of albumin (ALB, g/L), triglyceride (TG, mmol/L), high-density lipoprotein (HDL, mmol/L), low-density lipoprotein (LDL, mmol/L), and free fatty acid (FFA, μmol/L) were determined using the microplate method. The content of globulin (GLB, g/L) was determined using the immunoturbidimetry method. The content of glucose (GLU, mmol/L) was determined by the glucose oxidase method. The content of total cholesterol (T-CHO, mmol/L) was determined using the cholesterol oxidase (COD-PAP) method. The activities of aspartate aminotransferase (AST, U/L), alanine aminotransferase (ALT, U/L), and lactate dehydrogenase (LDH, IU/L) were determined using the microplate method. The activities of carnitine palmityl transferase 1 (CPT-1, U/mgprot), acetyl CoA carboxylase α (ACCα, U/gprot), fatty acid synthase (FAS, U/mgprot), lipoprotein lipase (LPL, U/gprot), glucose-6-phosphate dehydrogenase (G6PD, U/gprot), malate dehydrogenase (MDH, U/gprot), isocitrate dehydrogenase (IDH, U/mgprot), and stearoyl-CoA desaturase (SCD, U/mgprot) were determined using the colorimetric method. All the assay kits were produced by Nanjing Jiancheng Bioengineering Research Institute (Nanjing, China), and all instructions can be found and downloaded at http://www.njjcbio.com/ (accessed on 1 April 2024).
2.5 Determination of muscle composition
The contents of crude fat, crude protein, moisture, and ash in the muscle of juvenile tilapia were determined according to the method of the Association of Official Analytical Chemists (AOAC, 2005). The crude fat was determined by Soxhlet extraction. The crude protein was determined by Kjeldahl nitrogen determination. The moisture was determined by oven drying at 105°C and by the constant weight method. The ash was determined by muffle furnace burning at 550°C.
2.6 Determination of fatty acid composition in the muscle
According to the method of Liu et al. (2023), fatty acids in the muscle were determined by gas chromatography (GC, Agilent Technologies, Inc., Santa Clara, California, USA). The determination of fatty acids was as follows: First, the lipids were extracted and purified; second, the lipids were saponified; third, the fatty acids were methylated; fourth, the extracted fatty acids underwent methyl esterification; and fifth, fatty acids were determined using a gas chromatograph. The mixed standard of fatty acid methyl esterification (number GLC 455) comes from China Shanghai Sigma-Aldridge (Shanghai) Trading Co., Ltd.
2.7 Determination of gene expression
The real-time quantitative polymerase chain reaction (RT-qPCR) method of Zhang et al. (2023) was applied to determine the expression of superoxide dismutase (SOD), catalase (CAT), glutathione peroxidase (GSH-PX), glutathione S-transferase (GST), nuclear factor erythroid 2-related factor 2 (Nrf2), lysozyme (LYZ), alkaline phosphatase (ALP), tumor necrosis factor α (TNF-α), interleukin 1β (IL-1β), interleukin 6 (IL-6), interleukin 8 (IL-8), interleukin 10 (IL-10), interferon-γ (INF-γ), transforming growth factor β1 (TGF-β1), and β-actin in juvenile GIFT. β-Actin was used as the internal reference gene. Forward and reverse primers for RT-qPCR were designed using the primer design software Primer Premier 6.0, based on the mRNA sequences of tilapia available in the National Center for Biotechnology Information (NCBI) database. These primers were synthesized by Shanghai Sangon Bioengineering Technology Co., Ltd., Shanghai, China. Detailed information about the primers can be found in Table 1. The brief steps of the RT-qPCR method are as follows.
First, total RNA was extracted from the liver of a juvenile GIFT by using the Takara MiniBEST Universal RNA Extraction Kit (universal type) produced by Takara Biomedical Technology Co., Ltd., Beijing, China. The instructions provided in the toolkit gave more information. The content and purity of RNA were determined using the NanoDrop-2000 spectrophotometer (Thermo, Waltham, MA, USA). The RNA content was determined by measuring the absorbance of the RNA solution at 260 nm. The RNA content ranged from 30 to 1,000 ng/mL. The purity of RNA was determined by measuring the absorbance ratio of the RNA solution at 260:280 nm. The ratio of 260:280 in the range of 1.9–2.1 shows that RNA purity is high and meets the experimental requirements. The integrity of RNA was evaluated by gel electrophoresis on 1% (w/v) agarose TAE gel stained with gel RedTM nucleic acid stain (UVP, Upland, CA, USA). Complete RNA usually shows three different bands on agarose gel electrophoresis, of which the 28S band is the brightest, followed by the 18S band, and the 5S band is the least bright. The brightness ratio between 28S and 18S bands can be used as a measure of RNA quality. When the brightness of the 28S band is more than twice that of the 18S band, it shows that the integrity of RNA is the best.
Second, 1,000 ng of total RNA was reverse-transcribed into cDNA using PrimeScript™ RT Master Mix (Perfect Real-Time) reverse transcription kit produced by Takara Biomedical Technology Co., Ltd., Beijing, China. According to the instructions of the kit, a reaction mixture with a total volume of 50 μL per reaction cycle was prepared, which contained the following components: 2× Taqman PCR mixture (25 μL), upstream primer (10 μM) (2.5 μL), downstream primer (10 μm) (2.5 μm), RNA template (1,000 ng), and ddH2O (adjusted to a total volume of 50 μL). After the PCR reaction, a cDNA sample was obtained. The process includes culturing at 30°C for 10 min, then at 42°C for 15 min, and finally at 95°C for 5 min and then cooling to 5°C for 5 min.
Third, the LightCycler 96 RT-qPCR detection system (Roche, Basel, Switzerland) and the TB Green Premix Ex Taq™ II (Tli RNase H Plus) RT-qPCR kit produced by Takara Biomedical Technology (Beijing) Co., Ltd., Beijing, China, were used for RT-qPCR determination. RT-qPCR reaction was carried out using 2 μL of gDNA Clean Reaction Mix Ver.2, 4 μL of 5 × Evo M-MLVRT Reaction Mix Ver.2, 1 μL of forward primer, 1 μL of reverse primer, 2 μL of cDNA, and 10 μL of RNase-free water, with a total reaction volume of 20 μL. The thermal cycling conditions of RT-qPCR reaction include an initial denaturation step at 95°C for 10 s and then amplification for 40 cycles, including denaturation at 95°C for 60 s and annealing at 60°C for 30 s and at 72°C for 90 s. The specificity of the reaction was confirmed by analyzing the melting curve obtained when heating to 95°C.
The 2−ΔΔCT method (Schmittgen and Livak, 2008) was applied to calculate the relative expression levels of SOD, CAT, GSH-PX, GST, Nrf2, LYZ, ALP, TNF-α, IL-1β, IL-6, IL-8, IL-10, INF-γ, and TGF-β1 genes in juvenile GIFT.
2.8 Data statistical analyses
The data underwent initial processing in Microsoft Excel 2023 (Version number: 16.78 [23100802], Microsoft Corporation, Washington, WA, USA). Subsequently, a one-way analysis of variance (ANOVA) was performed using the IBM SPSS 26 software package (International Business Machines Corporation, Armonk, NY, USA). Normality and homogeneity of variances among the groups were evaluated before conducting the statistical tests. For comparisons between groups, Duncan’s multiple-range test was utilized. Statistical significance was set at P <0.05 level, and the results were reported as mean ± standard error (mean ± SE).
3 Results
3.1 Effects of dietary marine red yeast supplementation on serum biochemistry parameters of juvenile GIFT
The contents of TP, ALB, HDL, and FFA in the serum of juvenile GIFT fed different dietary marine red yeast supplementation (0.25%, 0.50%, 0.75%, and 1.00% marine red yeast) were significantly higher (P < 0.05) than those fed with the control diet (0% marine red yeast). Among the groups, the highest TP was in the 1.00% dietary marine red yeast group, the highest ALB and HDL were in the 0.50% dietary marine red yeast group, and the highest FFA was in the 0.75% dietary marine red yeast group, as shown in Table 2.
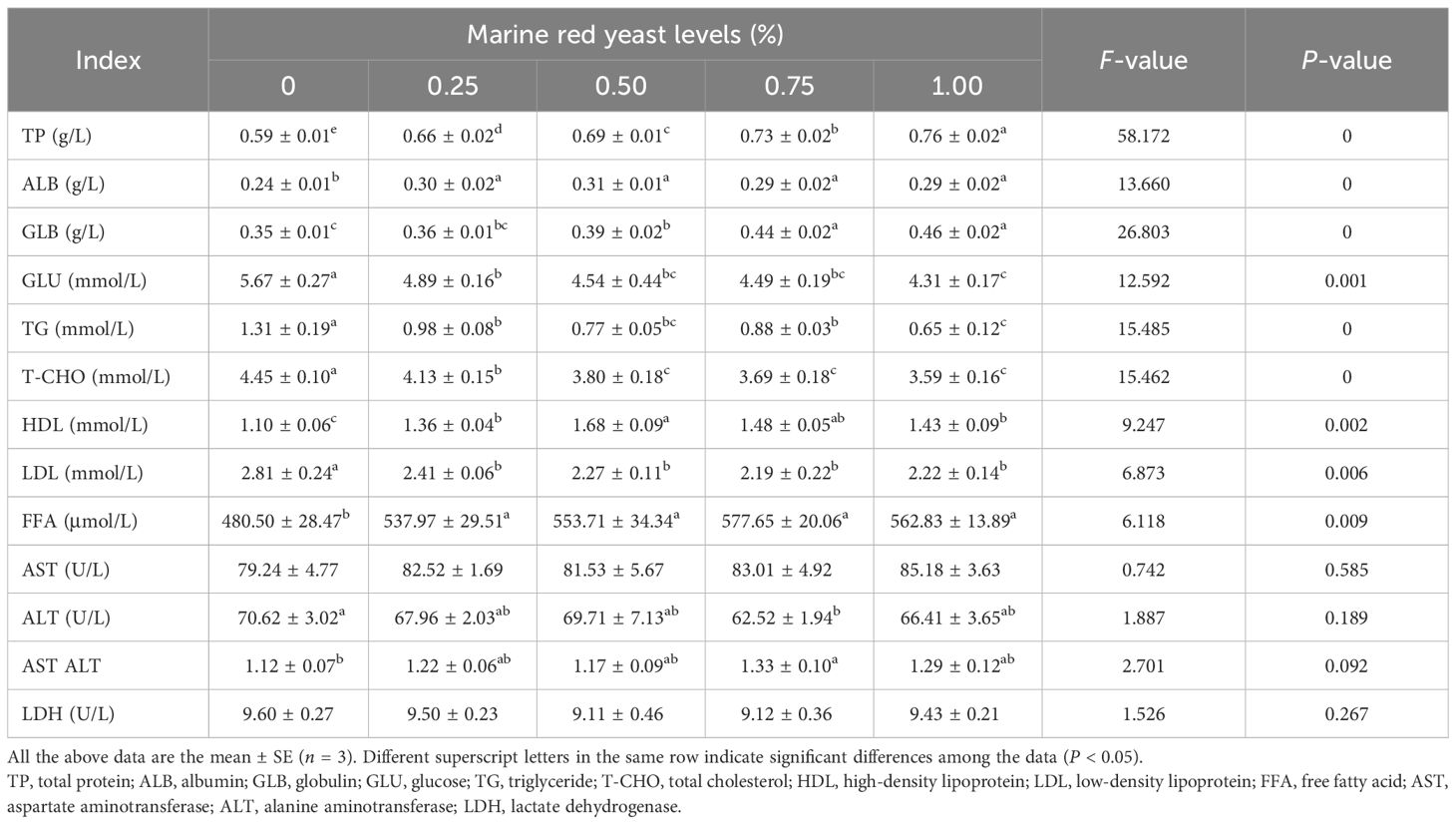
Table 2 Effect of dietary marine red yeast supplementation on serum biochemistry parameters of juvenile genetically improved farmed tilapia (GIFT).
The contents of GLU, TG, T-CHO, and LDL in the serum of juvenile GIFT fed different dietary marine red yeast supplementation (0.25%, 0.50%, 0.75%, and 1.00% marine red yeast) were significantly lower (P < 0.05) than those fed with the control diet (0% marine red yeast). Among the groups, the lowest GLU, TG, and T-CHO were in the 1.00% dietary marine red yeast group, and the lowest LDL was in the 0.75% dietary marine red yeast group, as shown in Table 2.
The content of GLB in the serum of juvenile GIFT fed different dietary marine red yeast supplementation (0.50%, 0.75%, and 1.00% marine red yeast) was significantly higher (P < 0.05) than those fed with the control diet (0% marine red yeast). The highest GLB was in the 1.00% dietary marine red yeast group. However, there was no significant difference (P > 0.05) in GLB between the 0.25% marine red yeast group and the control group, as shown in Table 2.
The activity of ALT in the serum of juvenile GIFT fed 0.75% marine red yeast supplementation was significantly lower (P < 0.05) than those fed with the control diet (0% marine red yeast). However, there was no significant difference (P > 0.05) in ALT between the 0.25%, 0.50%, and 1.00% marine red yeast groups and the control group, as shown in Table 2.
The AST/ALT in the serum of juvenile GIFT fed 0.75% marine red yeast supplementation was significantly higher (P < 0.05) than those fed with the control diet (0% marine red yeast). However, there was no significant difference (P > 0.05) in AST/ALT among the 0.25%, 0.50%, and 1.00% marine red yeast groups and the control group, as shown in Table 2.
There was no significant difference (P > 0.05) in the activities of AST and LDH in the serum of juvenile GIFT among the different dietary marine red yeast supplementation groups (0.50%, 0.75%, and 1.00% marine red yeast) and the control group, as shown in Table 2.
3.2 Effects of dietary marine red yeast supplementation on muscle composition of juvenile GIFT
The contents of crude protein and crude fat in the muscle of juvenile GIFT fed different dietary marine red yeast supplementation (0.25%, 0.50%, 0.75%, and 1.00% marine red yeast) were significantly higher (P < 0.05) than those fed with the control diet (0% marine red yeast). Among the groups, the highest crude protein was in the 0.50% dietary marine red yeast group, and the highest crude protein was in the 0.75% dietary marine red yeast group, as shown in Table 3.
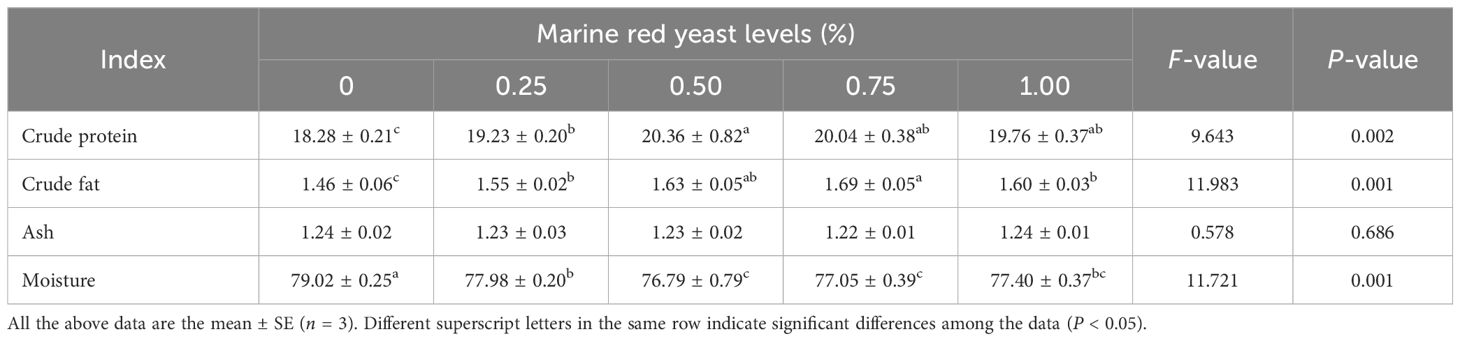
Table 3 Effects of dietary marine red yeast supplementation on muscle composition of juvenile GIFT (%/wet basis).
The content of moisture in the muscle of juvenile GIFT fed different dietary marine red yeast supplementation (0.25%, 0.50%, 0.75%, and 1.00% marine red yeast) was significantly lower (P < 0.05) than those fed with the control diet (0% marine red yeast). The lowest moisture was in the 0.50% dietary marine red yeast group, as shown in Table 3.
There was no significant difference (P > 0.05) in the content of ash in the muscle of juvenile GIFT among the different dietary marine red yeast supplementation groups (0.25%, 0.50%, 0.75%, and 1.00% marine red yeast) and the control group, as shown in Table 3.
3.3 Effects of dietary marine red yeast supplementation on muscle fatty acid composition of juvenile GIFT
The content of ∑MUFAs in the muscle of juvenile GIFT fed different dietary marine red yeast supplementation (0.25%, 0.50%, 0.75%, and 1.00% marine red yeast) was significantly lower (P < 0.05) than those fed with the control diet (0% marine red yeast). The lowest ∑MUFA was in the 0.50% dietary marine red yeast group, as shown in Table 4.
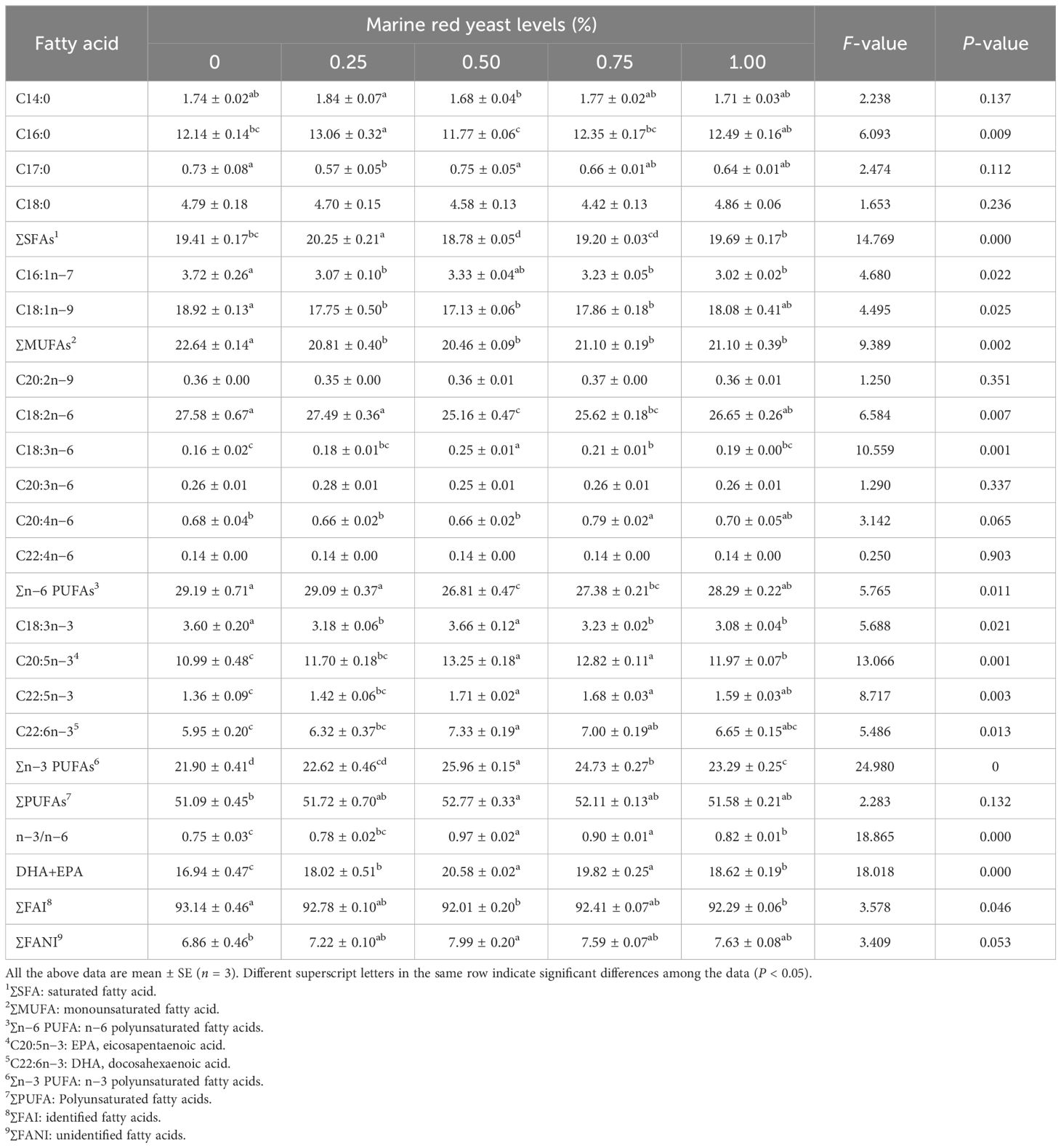
Table 4 Effects of dietary marine red yeast supplementation on muscle fatty acid composition of juvenile GIFT.
The content of DHA+EPA in the muscle of juvenile GIFT fed different dietary marine red yeast supplementation (0.25%, 0.50%, 0.75%, and 1.00% marine red yeast) was significantly higher (P < 0.05) than those fed with the control diet (0% marine red yeast). The highest DHA+EPA was in the 0.50% dietary marine red yeast group, as shown in Table 4.
The content of ∑SFAs in the muscle of juvenile GIFT fed 0.25% dietary marine red yeast supplementation was significantly higher (P < 0.05) than those fed with the control diet (0% marine red yeast). The content of ∑SFAs in juvenile GIFT fed 0.50% dietary marine red yeast supplementation was significantly lower (P < 0.05) than those fed with the control diet. However, there was no significant difference (P > 0.05) in ∑SFAs among the 0.75% and 1.00% marine red yeast groups and the control group, as shown in Table 4.
The content of ∑n−6 PUFAs in the muscle of juvenile GIFT fed 0.50% and 0.75% dietary marine red yeast supplementation was significantly lower (P < 0.05) than those fed with the control diet (0% marine red yeast). However, there was no significant difference (P > 0.05) in ∑n−6 PUFAs among the 0.25% and 1.00% marine red yeast groups and the control group, as shown in Table 4.
The contents of C20:5n−3 (EPA) and ∑n−3 PUFAs in the muscle of juvenile GIFT fed different dietary marine red yeast supplementation (0.50%, 0.75%, and 1.00% marine red yeast) were significantly higher (P < 0.05) than those fed with the control diet (0% marine red yeast). The highest C20:5n−3 and ∑n−3 PUFAs were in the 0.50% dietary marine red yeast group. However, there was no significant difference (P > 0.05) in C20:5n−3 and ∑n−3 PUFAs between the 0.25% marine red yeast group and the control group, as shown in Table 4.
The content of C22:6n−3 (DHA) in the muscle of juvenile GIFT fed 0.50% and 0.75% dietary marine red yeast supplementation was significantly higher (P < 0.05) than those fed with the control diet (0% marine red yeast). The highest C22:6n−3 was in the 0.50% dietary marine red yeast group. However, there was no significant difference (P > 0.05) in C22:6n−3 among the 0.25% and 1.00% marine red yeast groups and the control group, as shown in Table 4.
The content of ∑PUFAs in the muscle of juvenile GIFT fed 0.50% dietary marine red yeast supplementation was significantly higher (P < 0.05) than those fed with the control diet (0% marine red yeast). However, there was no significant difference (P > 0.05) in ∑PUFAs between the 0.25%, 0.75%, and 1.00% marine red yeast groups and the control group, as shown in Table 4.
3.4 Effects of dietary marine red yeast supplementation on lipid metabolism enzyme activity of juvenile GIFT
The activities of ACCα, G6PD, and SCD in the liver of juvenile GIFT fed different dietary marine red yeast supplementation (0.25%, 0.50%, 0.75%, and 1.00% marine red yeast) were significantly higher (P < 0.05) than those fed with the control diet (0% marine red yeast). The highest ACCα, G6PD, and SCD were in the 0.50% dietary marine red yeast group, as shown in Table 5.
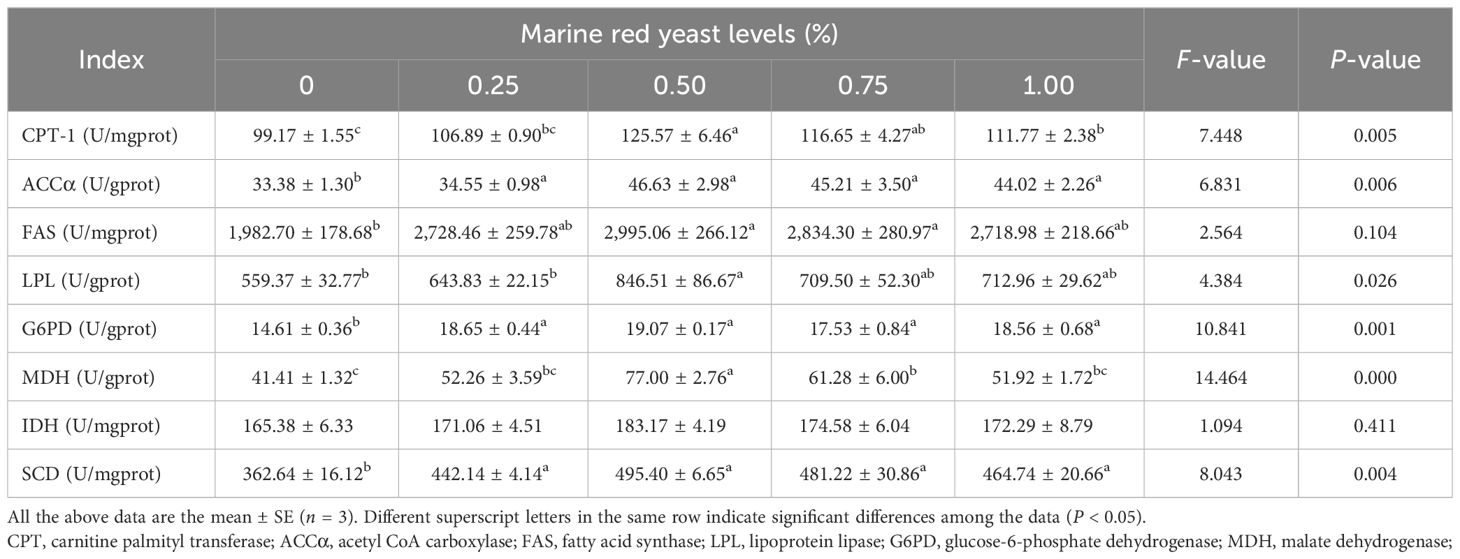
Table 5 Effects of dietary marine red yeast supplementation on lipid metabolism enzyme activity of juvenile GIFT.
The activity of CPT-1 in the liver of juvenile GIFT fed different dietary marine red yeast supplementation (0.50%, 0.75%, and 1.00% marine red yeast) was significantly higher (P < 0.05) than those fed with the control diet (0% marine red yeast). The highest CPT-1 was in the 0.50% dietary marine red yeast group. However, there was no significant difference (P > 0.05) in CPT-1 between the 0.25% marine red yeast group and the control group, as shown in Table 5.
The activities of FAS and MDH in the liver of juvenile GIFT fed 0.50% and 0.75% dietary marine red yeast supplementation were significantly higher (P < 0.05) than those fed with the control diet (0% marine red yeast). The highest FAS and MDH were in the 0.50% dietary marine red yeast group. However, there was no significant difference (P > 0.05) in FAS and MDH among the 0.25% and 1.00% marine red yeast groups and the control group, as shown in Table 5.
The activity of LPL in the liver of juvenile GIFT fed 0.50% dietary marine red yeast supplementation was significantly higher (P < 0.05) than those fed with the control diet (0% marine red yeast). However, there was no significant difference (P > 0.05) in LPL among the 0.25%, 0.75%, and 1.00% marine red yeast groups and the control group, as shown in Table 5.
There was no significant difference (P > 0.05) in the activity of IDH in the liver of juvenile GIFT among the different dietary marine red yeast supplementation groups (0.25%, 0.50%, 0.75%, and 1.00% marine red yeast) and the control group, as shown in Table 5.
3.5 Effects of dietary marine red yeast supplementation on the expression of antioxidant gene of juvenile GIFT
The relative expression levels of Nrf2, SOD, CAT, GSH-PX, GST, ALP, and LYZ genes in the liver of juvenile GIFT fed different dietary marine red yeast supplementation (0.25%, 0.50%, 0.75%, and 1.00% marine red yeast) were significantly higher (P < 0.05) than those fed with the control diet (0% marine red yeast). The highest Nrf2 and LYZ were in the 1.00% dietary marine red yeast group, the highest SOD and GSH-PX were in the 0.75% dietary marine red yeast group, and the highest CAT, GST, and ALP were in the 0.50% dietary marine red yeast group, as shown in Figure 1.
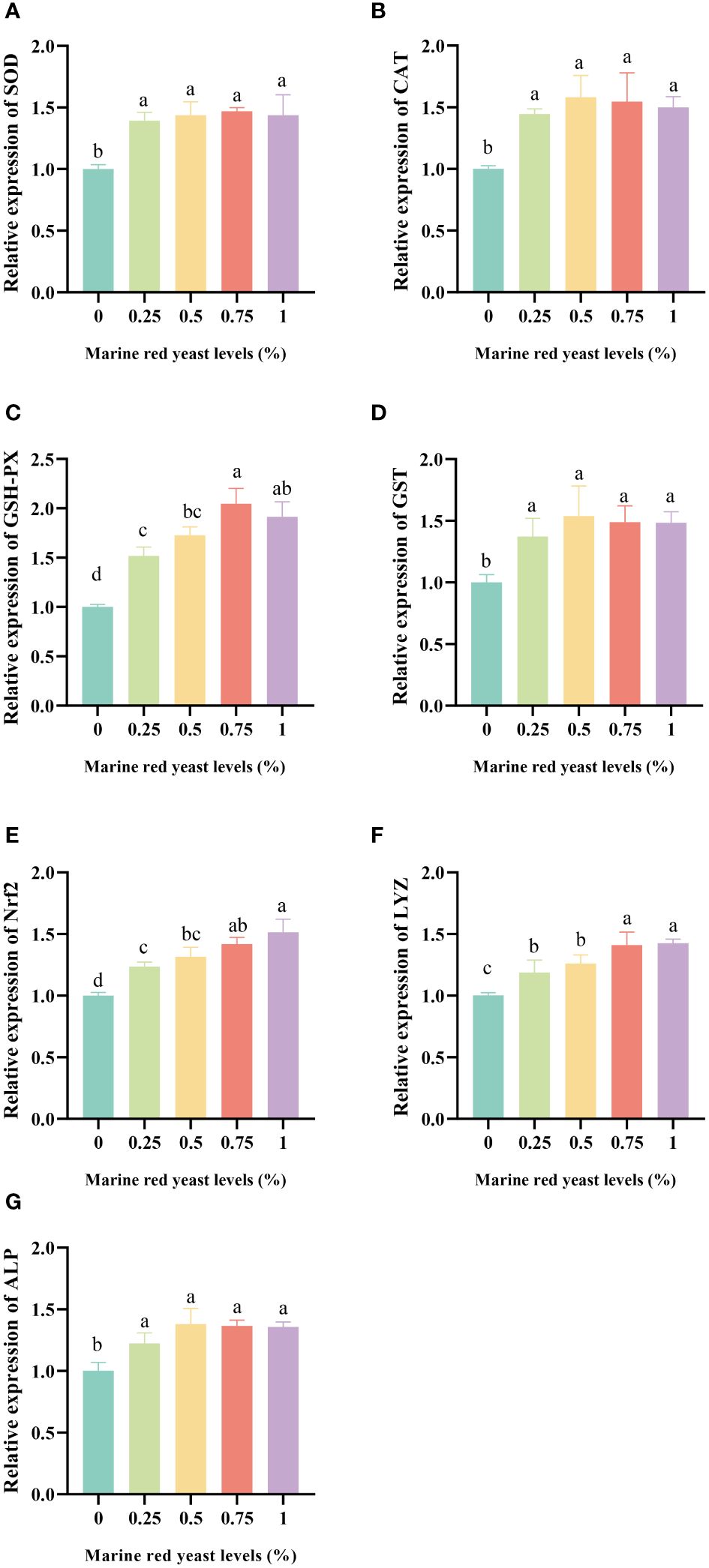
Figure 1 Effects of dietary marine red yeast supplementation on the relative expression levels of superoxide dismutase [SOD,(A)], catalase [CAT, (B)], glutathione peroxidase [GSH-PX, (C)], glutathione S-transferase [GST, (D)], nuclear factor erythroid 2-related factor 2 [Nrf2, (E)], lysozyme [LYZ, (F)], and alkaline phosphatase [ALP, (G)] genes in the liver of juvenile GIFT. All the above data are the mean ± SE (n = 3). Different superscript letters in the figure indicate significant differences among the data (P < 0.05).
3.6 Effects of dietary marine red yeast supplementation on the expression of inflammatory response gene of juvenile GIFT
The relative expression levels of TNF-α, INF-γ, IL-6, IL-8, IL-1β, and TGF-β1 genes in the liver, spleen, and head kidney of juvenile GIFT fed different dietary marine red yeast supplementation (0.25%, 0.50%, 0.75%, and 1.00% marine red yeast) were significantly higher (P < 0.05) than those fed with the control diet (0% marine red yeast). The highest TNF-α in the liver and spleen was in the 0.75% dietary marine red yeast group, and the highest TNF-α in the head kidney was in the 1.00% dietary marine red yeast group. The highest IL-1β in the liver was in the 1.00% dietary marine red yeast group, and the highest IL-1β in the spleen and head kidney was in the 0.75% dietary marine red yeast group. The highest IL-6 in the liver was in the 0.50% dietary marine red yeast group, and the highest IL-6 in the spleen and head kidney was in the 0.75% dietary marine red yeast group. The highest IL-8 in the liver was in the 1.00% dietary marine red yeast group, and the highest IL-8 in the spleen and head kidney was in the 0.75% dietary marine red yeast group. The highest TGF-β1 in the liver and spleen was in the 1.00% dietary marine red yeast group, and the highest TGF-β1 in the head kidney was in the 0.75% dietary marine red yeast group, as shown in Figure 2.
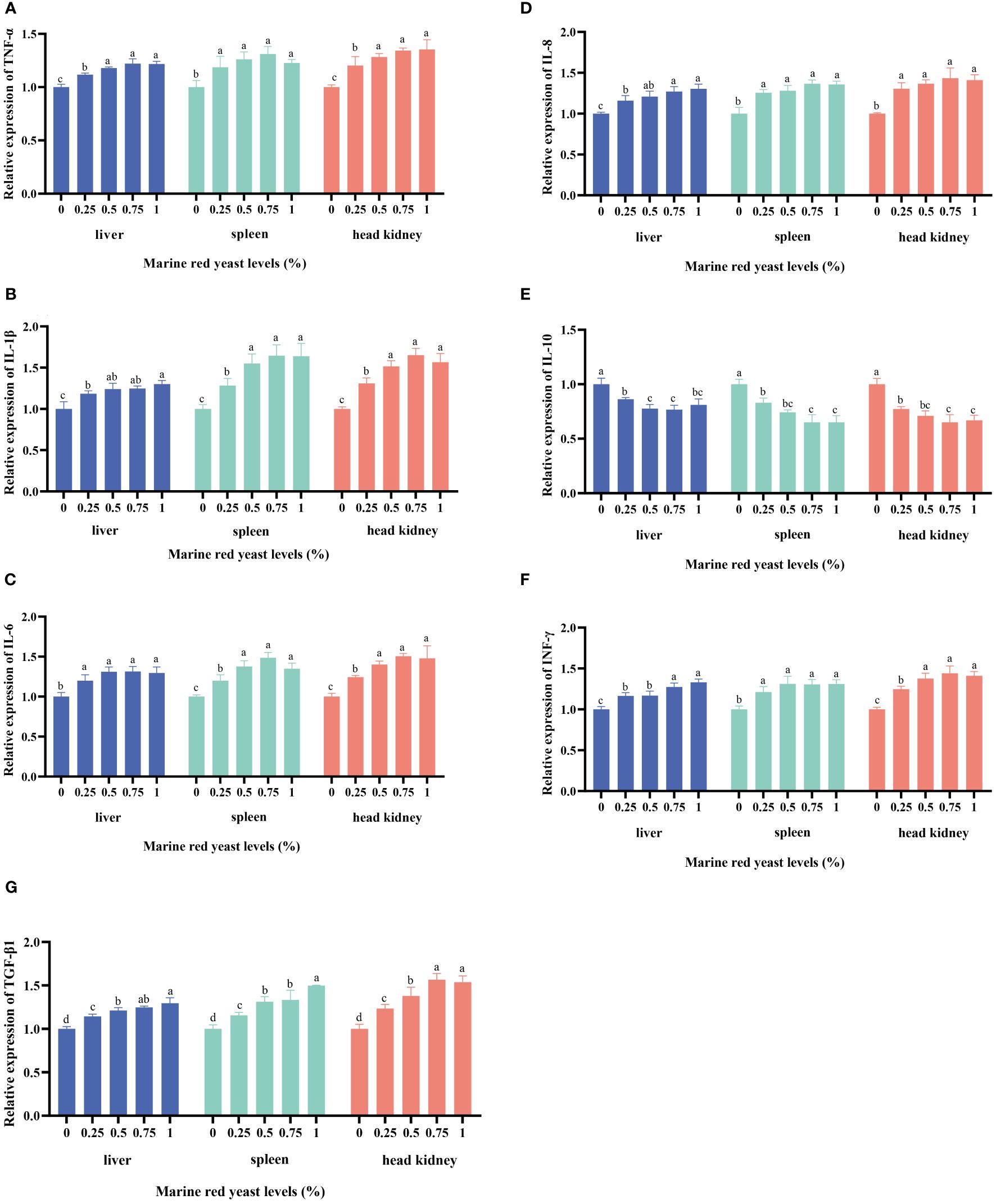
Figure 2 Effects of dietary marine red yeast supplementation on the relative expression levels of tumor necrosis factor α (TNF-α, [TNF-α, (A)], interleukin 1β [IL-1β, (B)], interleukin 6 [IL-6, (C)], interleukin 8 [IL-8, (D)], interleukin 10 [IL-10, (E)], interferon-γ [INF-γ, F], and transforming growth factor-β [TGF-β, (G)] genes in the liver, spleen, and head kidney of juvenile GIFT. All the above data are the mean ± SE (n = 3). Different superscript letters in the figure indicate significant differences among the data (P < 0.05).
The relative expression level of the IL-10 gene in the liver, spleen, and head kidney of juvenile GIFT fed different dietary marine red yeast supplementation (0.25%, 0.50%, 0.75%, and 1.00% marine red yeast) was significantly lower (P < 0.05) than those fed with the control diet (0% marine red yeast). The lowest IL-10 in the liver, spleen, and head kidney was in the 1.00% dietary marine red yeast group, as shown in Figure 2.
4 Discussion
Serum biochemical indicators are one of the standards used to measure the health and organ function of aquatic animals, and they are easily influenced by feed components (Dossou et al., 2018). Therefore, studying the changes in serum biochemical indicators in fish can help evaluate the nutritional value of marine red yeast added to feed. In this study, the levels of TP, GLB, and ALB in the serum of juvenile GIFT were significantly increased. This suggested that dietary marine red yeast supplementation could improve the metabolic rate, accelerate protein synthesis, and enhance immune function through the synthesis of astaxanthin and mannan-oligosaccharides in vivo. These compounds could improve nutrient utilization, rebalance intestinal flora microecology, promote nutrient absorption and utilization, and enhance fish resistance against pathogenic bacteria (Chen et al., 2019). In this study, the levels of HDL and FFA in the serum of juvenile GIFT were significantly increased, while the levels of LDL, TG, and T-CHO were significantly decreased. This indicated that marine red yeast could promote cholesterol metabolism and improve its lipid profile. The reasons may be as follows: First, marine red yeast could increase lipase activity and accelerate lipid metabolism (Castillo et al., 2014). Second, astaxanthin had a significant effect on increasing HDL concentration and reducing LDL concentration, thereby preventing arteriosclerosis and cardiovascular disease (Yoshida et al., 2009). Third, previous studies have shown a correlation between intestinal flora and plasma HDL levels, indicating that intestinal flora plays an important role in HDL metabolism (Hooman and Hazen, 2015). Therefore, the reason why marine red yeast could increase HDL content in this study may be due to its improvement of intestinal flora in juvenile GIFT, thereby promoting HDL metabolism within their bodies and increasing serum concentrations. In this study, the level of GLU in the serum of juvenile GIFT was significantly decreased, indicating that marine red yeast could improve glucose utilization in the body. This may be attributed to changes in intestinal microbial flora caused by marine red yeast, thereby promoting sugar substance absorption and utilization (Cheeranan et al., 2023). Additionally, mannan-oligosaccharides present in marine red yeast may stimulate insulin release and reduce blood glucose levels (Wang et al., 2022). In this study, the levels of ALT, AST, and LDH in the serum of juvenile GIFT were not significantly different among the marine red yeast supplementation groups and the control group, indicating that marine red yeast did not affect liver permeability or impact liver cells in juvenile GIFT. Similar findings were reported by Van et al., where adding marine red yeast to feed did not influence AST and ALT levels in juvenile GIFT serum (Van et al., 2022).
In this study, the contents of crude protein and crude fat in the muscle of juvenile GIFT were significantly increased. This may be because probiotics produce or improve nutrient absorption in the digestive tract endocrine system, which helps accumulate protein and fat in the body (Wu et al., 2021). It may also be due to a large amount of mannan-oligosaccharides in marine red yeast that could directly interact with intestinal cells, increasing nutrient exchange rate and improving digestibility of nutrients, feed utilization, and intestinal absorption of amino acids and minerals leading to changes in crude protein and crude fat content for juvenile GIFT (Chen et al., 2019). Similar results were found by Mukherjee et al. who believed Bacillus had a significant effect on crude protein, crude fat, and ash content of Rohu (Labeo rohita) (Mukherjee et al., 2019). Chen et al. found that adding 1% marine red yeast to feed could increase the crude protein content of tilapia (Chen et al., 2019). However, there were conflicting reports on the effect of probiotics on the muscle composition of aquatic animals. Some studies have shown that adding probiotics to feed did not significantly affect the crude protein, crude fat, and crude ash content of olive flounder (Paralichthys olivaceus) (Niu et al., 2019) and Javanese carp (Puntius gonionotus, Bleeker 1850) (Allameh et al., 2016). Inconsistent results from these studies may relate to different probiotic strains used as well as differences among fish species’ feeding environments and experimental periods among other factors.
Fatty acids are essential nutrients in fish feed, playing a significant role in the growth, development, and reproduction of fish (Xu et al., 2017). The lipid metabolism process in fish is influenced by various factors, such as breeding conditions, breeding modes, and nutrient levels in their feed (Zhao et al., 2018). In this study, the content of n−6 PUFAs in the muscle of juvenile GIFT was significantly decreased, while the content of n−3 PUFAs and the n−3/n-6 PUFAs ratio were significantly increased. The reason for this may be that n−3 and n−6 PUFAs shared a variety of fatty acid synthase enzymes, and there was a metabolic competition between them. These enzymes had a higher affinity for n−3 PUFAs. When excess n−3 PUFAs were ingested, they competed with n−6 PUFAs for △6 desaturase enzymes to inhibit arachidonic acid (C20:4n−6, AA) production, thereby reducing the total amount of n−6 PUFAs (Karapanagiotidis et al., 2007; Chen et al., 2011). Previous studies have shown the potential role of n−3 LC-PUFA in alleviating lipid deposition in fish such as grass carp (Ctenopharyngodon idellus) (Ji et al., 2011) and Atlantic salmon (Salmo salar) (Todorčević et al., 2008). Therefore, an increase in the concentration of n−3 LC-PUFA observed in this study indicated that red yeast promoted lipid accumulation in fish. In this study, unsaturated fatty acid content significantly increased in the muscle of juvenile GIFT. This could be attributed to marine red yeast being a lipid-rich probiotic containing various unsaturated fatty acids, which increased unsaturated fatty acid content after feeding it to fish (Gupta et al., 2012). Another possible reason was that astaxanthin prevented oxidation and reduction of unsaturated acids leading to an increase in their content within fish (Nakano et al., 1999). Additionally, the DHA+EPA content significantly increased may be due to marine red yeast providing large amounts of linoleic acid and linolenic acid promoting DHA and EPA production within fish (Chen et al., 2018). Studies have shown that the levels of EPA and DHA could directly affect the growth, molting, gonadal development, and immune capacity of aquatic organisms (Wu et al., 2011). Therefore, the increase in EPA and DHA observed in this study indicated that marine red yeast could promote the growth, antioxidant capacity, and immune response of tilapia. Additionally, it had been demonstrated that elevated levels of EPA and DHA facilitated the oxidation and utilization of certain fatty acids within tissue organs (Nakano et al., 1999). So, the results from this study suggested that marine red yeast played a role in promoting lipid accumulation in juvenile GIFT.
In this study, the activities of ACCα, FAS, G6PD, MDH, SCD, and IDH significantly increased in the liver of juvenile GIFT, while the activities of LPL and CPT-1 significantly decreased. The possible reason was that marine red yeast could improve the activity of digestive enzymes in tilapia, enhance nutrient digestion and utilization, and promote fish fat synthesis (Wang et al., 2015). Duan et al. fed the Pacific white shrimp with a diet supplemented with Clostridium butyricum for 56 days and found that the short-chain fatty acid content and digestive enzyme activity in the gut of the probiotic group increased (Duan et al., 2017). Zhao et al. discovered that adding 1 × 109 cfu/kg Clostridium butyricum to broiler chicken feed increased intramuscular fat content in the breast and leg muscles by affecting fatty acid synthase enzyme activity and lipid synthesis-related gene expression (Zhao et al., 2013). Similar results have also been observed in pigs, where long-term feeding with probiotic Lactobacillus reuteri could significantly affect its fatty acid metabolism level (Tian et al., 2020).
Fish have natural antioxidant and non-specific immune systems, in which SOD, CAT, GSH-PX, and other enzymes are the main antioxidant enzymes that scavenge free radicals in the body (Fattman et al., 2003). Nrf2 is an important transcription factor that regulates the expression of various antioxidant-related genes, such as SOD, CAT, and GSH-PX (Yuan et al., 2018). LYZ and ALP are important components of fish resistance to pathogen infection in the environment and can be used to reflect the strength of the immune function (Siti et al., 2015). In this study, the expression levels of CAT, Nrf2, GST, GSH-PX, SOD, ALP, and LYZ genes in the liver of juvenile GIFT were significantly increased, indicating that marine red yeast could improve the antioxidant and immune performance of tilapia. The reasons may be due to its ability to produce β-glucan, carotenoids, astaxanthin, and other substances with strong antioxidant activity. These substances could clear excess reactive oxygen species and free radicals in the body while protecting tissues against oxidative damage and enhancing immune performance in aquatic animals (Dou et al., 2023). Similar studies have shown that supplementing tilapia feed with yeast hydrolyzate increased GSH-PX, SOD, and CAT activities in tilapia while enhancing ALP gene expression (Andriamialinirina et al., 2020). In sea cucumbers (Apostichopus japonicus), adding red yeast significantly improved the SOD, LYZ, ACP, and AKP activities (Wang et al., 2015).
Probiotics can regulate immune response by stimulating cytokines, which in turn stimulate host resistance and promote the elimination of potential pathogens (Nayak, 2010). Inflammatory factors include IL-1β, IL-6, IL-8, and TNF-α. These factors are important inflammatory mediators in animals and play a significant role in immune regulation. Their expression levels are often used as indicators of the body’s inflammatory response (Liu et al., 2023). In this study, adding marine red yeast to feed significantly increased the expression of inflammatory response genes and improved the immune response of juvenile GIFT. The results may be attributed to marine red yeast acting as a probiotic, producing bactericidal substances such as bacteriocins and lysozymes, which had inhibitory effects on typical pathogenic bacteria in aquatic animals and stimulated cytokine expression (Verschuere et al., 2000). Red yeast contained β-glucan, which enhanced the body’s immunity by increasing lysozyme activity and phagocytic cell count and activating the complement pathway. It also stimulated cytokine expression to improve immune response (Liu et al., 2021). Nguyen et al. feeding β-glucan enhanced carp’s immune ability and increased LYS and IL-8 gene expression (Nguyen et al., 2019). Jami et al. found that adding β-glucan to salmon (Salmo trutta caspius) feed significantly increased TNF-α, IL-1, and IL-8 gene expression (Jami et al., 2019). The increase in inflammatory factor expression observed in tilapia during this experiment may also be due to improved antioxidant capacity leading to reduced ROS levels within their bodies. This enhancement strengthens non-specific immune capacity while stimulating gene expressions of TNF-α, IL-1β, IL-8, IL-6, and INF-γ (Siti et al., 2015). Similar studies have shown that in the study of tilapia, the addition of yeast hydrolysate to feed could significantly increase the expression of TNF-α, TGF-β, IL-1β, IL-10, and other genes in the liver (Andriamialinirina et al., 2020).
5 Conclusion
In conclusion, the addition of different levels of marine red yeast (R. mucilaginosa) to the diet could significantly affect the serum biochemistry, muscle composition, muscle fatty acid composition, lipid metabolism enzyme activity, and the expression of antioxidant and inflammatory genes in juvenile GIFT. Based on the results, marine red yeast could be used as an aquatic animal feed additive, and the optimal dietary marine red yeast level was 0.50%. This study can provide a reference for incorporating marine red yeast into the commercialized breeding production of tilapia.
Data availability statement
The original contributions presented in the study are included in the article/supplementary material. Further inquiries can be directed to the corresponding authors.
Ethics statement
The animal study was approved by the Biomedical Ethics Committee of Guangxi Minzu University. The study was conducted in accordance with the local legislation and institutional requirements.
Author contributions
YL: Conceptualization, Data curation, Formal analysis, Funding acquisition, Investigation, Methodology, Project administration, Resources, Supervision, Validation, Visualization, Writing – review & editing, Writing – original draft. EH: Conceptualization, Data curation, Formal analysis, Investigation, Methodology, Resources, Software, Supervision, Validation, Visualization, Writing – original draft. XL: Investigation, Methodology, Validation, Visualization, Writing – original draft. YX: Investigation, Methodology, Validation, Visualization, Writing – original draft. LM: Investigation, Methodology, Validation, Visualization, Writing – original draft. DL: Investigation, Methodology, Validation, Visualization, Writing – original draft. TT: Investigation, Methodology, Validation, Visualization, Writing – original draft. JW: Conceptualization, Data curation, Formal analysis, Funding acquisition, Investigation, Methodology, Project administration, Resources, Supervision, Validation, Visualization, Writing – review & editing. QZ: Conceptualization, Data curation, Formal analysis, Funding acquisition, Investigation, Methodology, Project administration, Resources, Supervision, Validation, Visualization, Writing – review & editing.
Funding
The author(s) declare financial support was received for the research, authorship, and/or publication of this article. This research was supported by the Guangxi Key Research and Development Program (Guike: AB23075147), grants from the Scientific Research Foundation for the Introduced Talents of Guangxi Minzu University (2021KJQD17 and 2018KJQD14), Innovation-Driven Development Special Fund Project of Guangxi (AA17204044), Joint Funds of the National Natural Science Foundation of China (U20A2064), and Shandong Provincial Key Research and Development Programs (2019JZZY020710).
Conflict of interest
The authors declare that the research was conducted in the absence of any commercial or financial relationships that could be construed as a potential conflict of interest.
Publisher’s note
All claims expressed in this article are solely those of the authors and do not necessarily represent those of their affiliated organizations, or those of the publisher, the editors and the reviewers. Any product that may be evaluated in this article, or claim that may be made by its manufacturer, is not guaranteed or endorsed by the publisher.
References
Allameh S. K., Yusoff F. M., Ringø E., Daud H. M., Saad C. R., Ideris A. (2016). Effects of dietary mono- and multiprobiotic strains on growth performance, gut bacteria and body composition of J avanese carp (Puntius gonionotus, Bleeker 1850). Aquacult. Nutr. 22, 367–373. doi: 10.1111/anu.12265
Andriamialinirina H. J. T., Irm M., Taj S., Lou J. H., Jin M., Zhou Q. (2020). The effects of dietary yeast hydrolysate on growth, hematology, antioxidant enzyme activities and non-specific immunity of juvenile Nile tilapia, Oreochromis niloticus. Fish Shellfish Immun. 101, 168–175. doi: 10.1016/j.fsi.2020.03.037
AOAC (2005). Association of official analytical chemists, official methods of analysis. 16th (Artington, Virginia, USA: AOAC International).
Castillo S., Rosales M., Pohlenz C., Gatlin D. M. (2014). Effects of organic acids on growth performance and digestive enzyme activities of juvenile red drum Sciaenops ocellatus. Aquaculture 433, 6–12. doi: 10.1016/j.aquaculture.2014.05.038
Cheeranan S., Sirawich L., Jaksuma P., Tannatorn P., Amarin M., Naruemon S., et al. (2023). Effect of fed dietary yeast (Rhodotorula paludigena CM33) on shrimp growth, gene expression, intestinal microbial, disease resistance, and meat composition of Litopenaeus vannamei. Dev. Comp. Immunol. 147, 104896. doi: 10.1016/J.DCI.2023.104896
Chen C., Guan W., Xie Q., Chen G., He X., Zhang H., et al. (2018). N-3 essential fatty acids in Nile tilapia, Oreochromis niloticus: Bioconverting LNA to DHA is relatively efficient and the LC-PUFA biosynthetic pathway is substrate limited in juvenile fish. Aquaculture 495, 513–522. doi: 10.1016/j.aquaculture.2018.06.023
Chen X., Zhao W., Xie S., Xie J., Zhang Z., Tian L., et al. (2019). Effects of dietary hydrolyzed yeast (Rhodotorula mucilaginosa) on growth performance, immune response, antioxidant capacity and histomorphology of juvenile Nile tilapia (Oreochromis niloticus). Fish Shellfish Immunol. 90, 30–39. doi: 10.1016/j.fsi.2019.03.068
Chen J., Zhu X., Han D., Yang Y., Lei W., Xie S. (2011). Effect of dietary n-3 HUFA on growth performance and tissue fatty acid composition of gibel carp Carassius auratus gibelio. Aquacult. Nutr. 17, 476–485. doi: 10.1111/j.1365–2095.2010.00784.x
Dawood M. A. O., Koshio S. (2016). Recent advances in the role of probiotics and prebiotics in carp aquaculture: A review. Aquaculture 454, 243–251. doi: 10.1016/j.aquaculture.2015.12.033
Dawood M. A. O., Koshio S., Ishikawa M., Yokoyama S., Basuini M. F. E., Hossain M. S., et al. (2017). Dietary supplementation of β-glucan improves growth performance, the innate immune response and stress resistance of red sea bream, Pagrus major. Aquacult. Nutr. 23, 148–159. doi: 10.1111/anu.12376
Dossou S., Koshio S., Ishikawa M., Yokoyama S., Dawood M. A. O., Basuini M. F. E., et al. (2018). Effect of partial replacement of fish meal by fermented rapeseed meal on growth, immune response and oxidative condition of red sea bream juvenile, Pagrus major. Aquaculture 490, 228–235. doi: 10.1016/j.aquaculture.2018.02.010
Dou X., Huang H., Li Y., Deng J., Tan B. (2023). Effects of dietary β-glucan on growth rate, antioxidant status, immune response, and resistance against Aeromonas hydrophila in genetic improvement of farmed tilapia (GIFT, Oreochromis niloticus). Aquac. Rep. 29, 101480. doi: 10.1016/J.AQREP.2023.101480
Duan Y., Zhang Y., Dong H., Wang Y., Zheng X., Zhang J. (2017). Effect of dietary Clostridium butyricum on growth, intestine health status and resistance to ammonia stress in Pacific white shrimp Litopenaeus vannamei. Fish Shellfish Immunol. 65, 25–33. doi: 10.1016/j.fsi.2017.03.048
Fattman C. L., Schaefer L. M., Oury T. D. (2003). Extracellular superoxide dismutase in biology and medicine. Free Radical Bio. Med. 35, 236–256. doi: 10.1016/S0891–5849(03)00275–2
Gupta A., Vongsvivut J., Barrow C. J., Puri M. (2012). Molecular identification of marine yeast and its spectroscopic analysis establishes unsaturated fatty acid accumulation. J. Biosci. Bioeng. 114, 411–417. doi: 10.1016/j.jbiosc.2012.05.013
Hai N. (2015). The use of probiotics in aquaculture. J. Appl. Microbiol. 119, 917–935. doi: 10.1111/jam.12886
Hooman A., Hazen L. S. (2015). Contribution of gut bacteria to lipid levels: Another metabolic role for microbes? Circ. Res. 117, 750–754. doi: 10.1161/CIRCRESAHA.115.307409
Jami M. J., Kenari A. A., Paknejad H., Mohseni M. (2019). Effects of dietary b-glucan, mannan oligosaccharide, Lactobacillus plantarum and their combinations on growth performance, immunity and immune related gene expression of Caspian trout, Salmo trutta caspius (Kessler 1877). Fish Shellfish Immunol. 91, 202–208. doi: 10.1016/j.fsi.2019.05.024
Ji H., Li J., Liu P. (2011). Regulation of growth performance and lipid metabolism by dietary n-3 highly unsaturated fatty acids in juvenile grass carp, Ctenopharyngodon idellus. Comp. Biochem. Physiology Part B. 159, 49–56. doi: 10.1016/j.cbpb.2011.01.009
Karapanagiotidis I. T., Bell M. V., Little D. C., Yakupitiyage A. (2007). Replacement of dietary fish oils by alpha-linolenic acid-rich oils lowers omega 3 content in tilapia flesh. Lipids. 42, 547–559. doi: 10.1007/s11745–007-3057–1
Liu Y., Huang E., Xie Y., Meng L., Liu D., Zhang Z., et al. (2023). The Effect of Dietary Lipid Supplementation on the Serum Biochemistry, Antioxidant Responses, Initial Immunity and mTOR Pathway of Juvenile Tilapia (Oreochromis niloticus). Fishes 8, 535. doi: 10.3390/FISHES8110535
Liu Y., Wu Q., Wu X., Attia A., Gong F., Hu J., et al. (2021). Structure, preparation, modification, and bioactivities of β-glucan and mannan from yeast cell wall: A review. Int. J. Biol. Macromol. 173, 445–456. doi: 10.1016/J.IJBIOMAC.2021.01.125
Mukherjee A., Chandra G., Ghosh K. (2019). Single or conjoint application of autochthonous Bacillus strains as potential probiotics: Effects on growth, feed utilization, immunity and disease resistance in Rohu, Labeo rohita (Hamilton). Aquaculture 512, 734302. doi: 10.1016/j.aquaculture.2019.734302
Nakano T., Kanmuri T., Sato M., Takeuchi M. (1999). Effect of astaxanthin rich red yeast (Phaffia rhodozyma) on oxidative stress in rainbow trout. Biochim. Biophys. Acta (BBA) - Gen. Subjects. 1426, 119–125. doi: 10.1016/S0304–4165(98)00145–7
Nayak S. K. (2010). Probiotics and immunity: A fish perspective. Fish Shellfish Immunol. 29, 2–14. doi: 10.1016/j.fsi.2010.02.017
Nguyen T., Syaghalirwa N., Tran T., Larondelle Y., Mellery J., Mignolet E., et al. (2019). Growth performance and immune status in common carp Cyprinus carpio as affected by plant oil-based diets complemented with β-glucan. Fish Shellfish Immun. 92, 288–299. doi: 10.1016/j.fsi.2019.06.011
Nikoskelainen S., Ouwehand A. C., Bylund G., Salminen S., Lilius E. (2003). Immune enhancement in rainbow trout (Oncorhynchus mykiss) by potential probiotic bacteria (Lactobacillus rhamnosus). Fish Shellfish Immunol. 15, 443–452. doi: 10.1016/S1050-4648(03)00023-8
Niu K., Khosravi S., Kothari D., Lee W., Lim J., Lee B., et al. (2019). Effects of dietary multi-strain probiotics supplementation in a low fishmeal diet on growth performance, nutrient utilization, proximate composition, immune parameters, and gut microbiota of juvenile olive flounder (Paralichthys olivaceus). Fish Shellfish Immunol. 93, 258–268. doi: 10.1016/S1050–4648(03)00023–8
Prabu E., Rajagopalsamy C. B. T., Ahilan B., Jeevagan I. J. M. A., Renuhadevi M. (2019). Tilapia – an excellent candidate species for world aquaculture: A review. Annu. Res. Rev. Biol. 31, 1–14. doi: 10.9734/arrb/2019/v31i330052
Richard G., Michaelle R., Naoual A., Pierre C. J., Celine B., Helena D. C., et al. (2012). A high-resolution map of the Nile tilapia genome: A resource for studying cichlids and other percomorphs. BMC Genomics 13, 222. doi: 10.1186/1471–2164-13–222
Schmittgen T., Livak K. (2008). Analyzing real-time PCR data by the comparative C(T) method. Nat. Protoc. 3, 1101–1108. doi: 10.1038/nprot.2008.73
Siti H. N., Kamisah Y., Kamsiah J. (2015). The role of oxidative stress, antioxidants and vascular inflammation in cardiovascular disease (a review). Vasc. Pharmacol. 71, 40–56. doi: 10.1016/j.vph.2015.03.005
Tian Z., Cui Y., Lu H., Wang G., Ma X. (2021). Effect of long-term dietary probiotic Lactobacillus reuteri 1 or antibiotics on meat quality, muscular amino acids and fatty acids in pigs. Meat Sci. 171, 108234. doi: 10.1016/j.meatsci.2020.108234
Todorčević M., Kjær M. A., Djaković N., Vegusdal A., Torstensen B. E., Ruyter B. (2008). N-3 HUFAs affect fat deposition, susceptibility to oxidative stress, and apoptosis in Atlantic salmon visceral adipose tissue. Comp. Biochem. Physiology Part B. 152, 135–143. doi: 10.1016/j.cbpb.2008.10.009
Tseng D. Y., Ho P. L., Huang S. Y., Cheng S. C., Shiu Y. L., Chiu C. S., et al. (2009). Enhancement of immunity and disease resistance in the white shrimp, Litopenaeus vannamei, by the probiotic, Bacillus subtilis E20. Fish Shellfish Immunol. 26, 339. doi: 10.1016/j.fsi.2008.12.003
Van D., Wanaporn T., Thanongsak C., Eakapol W., Ruamruedee P., Nantaporn S. (2022). Effects of Red Yeast (Sporidiobolus pararoseus) on Growth, Innate Immunity, Expression of Immune-related Genes and Disease Resistance of Nile Tilapia (Oreochromis niloticus). Probiotics antimicrobial proteins. 15, 1312–1326. doi: 10.1007/S12602–022-09984–8
Verschuere L., Rombaut G., Sorgeloos P., Verstraete W. (2000). Probiotic bacteria as biological control agents in aquaculture. Microbiol. Mol. Biol. reviews: MMBR. 64, 655–671. doi: 10.1023/A:1024127100993
Waché Y., Auffray F., Gatesoupe F., Zambonino J., Gayet V., Labbé L., et al. (2006). Cross effects of the strain of dietary Saccharomyces cerevisiae and rearing conditions on the onset of intestinal microbiota and digestive enzymes in rainbow trout, Onchorhynchus mykiss, fry. Aquaculture 258, 470–478. doi: 10.1016/j.aquaculture.2006.04.002
Wang B., Liu Y., Luo K., Zhang S., Wei C., Wang L., et al. (2023). ‘Biotic’ potential of the red yeast Rhodotorula mucilaginosa strain JM-01 on the growth, shell pigmentation, and immune defense attributes of the shrimp, Penaeus vannamei. Aquaculture 572, 739543. doi: 10.1016/j.aquaculture.2023.739543
Wang C., Liu Y., Sun G., Li X., Liu Z. (2018). Growth, immune response, antioxidant capability, and disease resistance of juvenile Atlantic salmon (Salmo salar L.) fed Bacillus velezensis V4 and Rhodotorula mucilaginosa compound. Aquaculture. 500, 65–74. doi: 10.1016/j.aquaculture.2018.09.052
Wang T., Wu H., Li W., Xu R., Qiao F., Du Z., et al. (2022). Effects of dietary mannan oligosaccharides (MOS) supplementation on metabolism, inflammatory response and gut microbiota of juvenile Nile tilapia (Oreochromis niloticus) fed with high carbohydrate diet. Fish Shellfish Immunol. 130, 550–559. doi: 10.1016/J.FSI.2022.09.052
Wang J., Zhao L., Liu J., Wang H., Xiao S. (2015). Effect of potential probiotic Rhodotorula benthica D30 on the growth performance, digestive enzyme activity and immunity in juvenile sea cucumber Apostichopus japonicus. Fish Shellfish Immunol. 43, 330–336. doi: 10.1016/j.fsi.2014.12.028
Wu Z., Qi X., Qu S., Ling F., Wang G. (2021). Dietary supplementation of Bacillus velezensis B8 enhances immune response and resistance against Aeromonas veronii in grass carp. Fish Shellfish Immunol. 115, 14–21. doi: 10.1016/J.FSI.2021.05.012
Wu X., Wang Z., Cheng Y. X., Zeng C., Yang X., Lu J. (2011). Effects of dietary phospholipids and highly unsaturated fatty acids on the precocity, survival, growth and hepatic lipid composition of juvenile Chinese mitten crab, Eriocheir sinensis (H. Milne-Edwards). Aquac. Res. 42, 457–468. doi: 10.1111/j.1365–2109.2010.02643.x
Xu Y., Li W., Ding Z. (2017). Polyunsaturated fatty acid supplements could considerably promote the breeding performance of carp. Eur. J. Lipid Sci. Tech. 119, 1600183. doi: 10.1002/ejlt.201600183
Yang S., Wu Z., Jian J., Zhang X. (2010). Effect of marine red yeast Rhodosporidium paludigenum on growth and antioxidant competence of Litopenaeus vannamei. Aquaculture 309, 62–65. doi: 10.1016/j.aquaculture.2010.09.032
Yoshida H., Yanai H., Ito K., Tomono Y., Koikeda T., Tsukahara H., et al. (2009). Administration of natural astaxanthin increases serum HDL-cholesterol and adiponectin in subjects with mild hyperlipidemia. Atherosclerosis. 209, 520–523. doi: 10.1016/j.atherosclerosis.2009.10.012
Yuan J., Wang D., Liu Y., Chen X., Zhang H., Shen F., et al. (2018). Hydrogen-rich water attenuates oxidative stress in rats with traumatic brain injury via Nrf2 pathway. J. Surg. Res. 228, 238–246. doi: 10.1016/j.jss.2018.03.024
Zhang Q., Guo M., Li F., Qin M., Yang Q., Yu H., et al. (2023). Evaluation of fermented soybean meal to replace a portion fish meal on growth performance, antioxidant capacity, immunity, and mTOR signaling pathway of coho salmon (Oncorhynchus kisutch). Aquacult. Nutr. 2023, 2558173. doi: 10.1155/2023/2558173
Zhang R., Niu S., Zhang H., Yang Z. (2014). effects of rhodotorula benthicain diet with brown fish meal on growth performance of turbot scophthalmus maximus. Fisheries Sci. China 33, 1003–1111. doi: 10.16378/j.cnki.1003–1111.2014.10.004
Zhao X., Guo Y., Guo S. (2013). Effects of Clostridium butyricum and Enterococcus faecium on growth performance, lipid metabolism, and cecal microbiota of broiler chickens. Appl. Microbiol. Biotechnol. 97, 6477–6488. doi: 10.1007/s00253–013-4970–2
Zhao H., Xia J., Zhang X., He X., Li L., Tang R., et al. (2018). Diet affects muscle quality and growth traits of grass carp (Ctenopharyngodon idellus): A comparison between grass and artificial feed. Front. Physiol. 9. doi: 10.3389/fphys.2018.00283
Keywords: marine red yeast, lipid metabolism, antioxidant, inflammatory, tilapia
Citation: Liu Y, Huang E, Li X, Xie Y, Meng L, Liu D, Tong T, Wang J and Zhang Q (2024) Serum biochemistry, fatty acids, lipid metabolism, antioxidants, and inflammation response were significantly affected by feeding different marine red yeast supplementation in juvenile tilapia (GIFT strain, Oreochromis niloticus). Front. Mar. Sci. 11:1426848. doi: 10.3389/fmars.2024.1426848
Received: 02 May 2024; Accepted: 03 June 2024;
Published: 21 June 2024.
Edited by:
Jin Niu, Sun Yat-sen University, ChinaReviewed by:
Jun Wang, Chinese Academy of Fishery Sciences (CAFS), ChinaZhigang Zhou, Chinese Academy of Agricultural Sciences, China
Shuyan Chi, Guangdong Ocean University, China
Copyright © 2024 Liu, Huang, Li, Xie, Meng, Liu, Tong, Wang and Zhang. This is an open-access article distributed under the terms of the Creative Commons Attribution License (CC BY). The use, distribution or reproduction in other forums is permitted, provided the original author(s) and the copyright owner(s) are credited and that the original publication in this journal is cited, in accordance with accepted academic practice. No use, distribution or reproduction is permitted which does not comply with these terms.
*Correspondence: Jinzi Wang, d2FuZ2ppbnppQGd4dW4uZWR1LmNu; Qin Zhang, emhhbmdxaW5AZ3htenUuZWR1LmNu
†These authors have contributed equally to this work